- 1Division of Applied Life Science (BK21 Four), Department of Horticulture, Graduate School, Gyeongsang National University, Jinju, South Korea
- 2Institute of Agriculture and Life Science, Gyeongsang National University, Jinju, South Korea
- 3Research Institute of Life Science, Gyeongsang National University, Jinju, South Korea
Silicon (Si) has been reported to benefit plant growth and stress resistance. This work aimed to find out an optimal method of Si application to enhance the resistance of strawberry (Fragaria × ananassa Duch.) transplants to high temperatures, commonly experienced in the summer when strawberries are propagated for greenhouse production in Korea. Plants of strawberry “Sulhyang”, “Maehyang”, and “Kuemsil” were subjected to one of five treatments before the cutting propagation: no treatment (control), substrate dressing of a water-soluble silicate fertilizer, substrate drench of 75 mg·L−1 Si (from potassium silicate) to the mother plants, or foliar spray of 75 mg·L−1 Si to either the mother plants or daughter plants. Half of the daughter plants in each Si treatment received continued application of Si through either substrate dressing of a water-soluble silicate fertilizer, substrate drench, or foliar spray after the cutting propagation. A high temperature (43°C) resistance test was conducted in plant growth chambers for 7 days with a 16-h photoperiod with a light intensity of 300 mmol·m−2·s−1 PPFD. During the high temperature test, the rate of decline in the photosynthesis was lower in plants treated with Si than in the control. After the high temperature test, it was observed that Si application significantly increased the shoot fresh weight of transplants. Moreover, the contents of sugars, proteins, and enzymatic (CAT, SOD, POD, and APX) and non-enzymatic (anthocyanin and proline) antioxidants were higher in plants treated with Si throughout the entire propagation period, compared to the control and plants only treated with Si before or after the cutting propagation. Overall, the Si application improved the growth of the transplants regardless of the application method used. Moreover, spraying the daughter plants with Si, and continually spraying the transplants were found to be the best and is recommended to increase the resistance of strawberries to high temperatures during propagation.
Introduction
Strawberry (Fragaria × ananassa, Duchesne) is a major economical fruit crop. In Korea, strawberry is cultivated on 6,057 hectares as of 2021. Of those, the open-field cultivation area was 519 hectares, while most of the strawberry cultivation was through facility cultivation (Korean Statistical Information Service, KOSIS, The Area of Facility Crop Cultivation https://kosis.kr/statHtml/statHtml.do?orgId=101&tblId=DT_1ET0017&conn_path=I2 accessed on 7 April 2022). Almost all strawberry plants are commercially propagated by using daughter plants from the runners (Caruana et al., 2018). The mother plants begin growing into unrooted runners in March, and the runner grows to daughter plants and are transferred to trays for rooting in June. Lastly, these plants are kept in a greenhouse until September, then established in the field or greenhouses (Takeda et al., 2004; Li et al., 2020). During this period, the temperature inside the greenhouse reaches 45°C with natural ventilation (Kim et al., 2020). In the last decade, abnormally high-temperature weather occurred from spring to late autumn in Korea (Korea Meteorological Administration, Report of Abnormal Climate http://www.climate.go.kr/home/bbs/list.php?code=93&bname=abnormal accessed on 7 April 2022). Furthermore, excessively high temperature frequently occurs due to global warming, and the Fifth Assessment Report presented by the Intergovernmental Panel on Climate Change (IPCC) suggests that there is a long-term increase in the global temperature (IPCC, 2014). Using cooling devices may be a heavy burden for farmers, therefore, researchers are recently trending toward improving the resistance of strawberries to high temperatures.
Silicon (Si) is the second most abundant element in the earth's crust (Epstein, 1994). It is present in the form of silicic acid (H4SiO4) in the soil solution and is absorbed by plants (Etesami and Jeong, 2018). Si has been demonstrated to be beneficial for the growth and development of plants, and the accumulation and deposition of Si can form a cuticle-Si double silicate layer in the leaves, decreasing the evapotranspiration and improving the photosynthesis rate (Park et al., 2018). Additionally, the variation can reinforce the morphological structures of the cell wall, and maintain the integrity of the cell membrane by creating a physical barrier (Nasircilar, 2021). It was reported that Si improves the N use efficiency and adjusts the absorption of P and micronutrients (Pavlovic et al., 2021). Besides, Si was involved in the stomatal regulation and the antioxidant enzyme system, and alleviated damages by preventing lipid peroxidation and hydrogen peroxide (H2O2) formation (Hu et al., 2020).
Many researchers have concluded that Si plays an important role in mitigating high temperature stresses in plants. The Si helps maintain photosynthesis in high-temperature environments by improving the abundance of photosynthetic proteins, especially the photosystem I P700 apoprotein A (PsaA) and photosystem II protein D1 (PsbA) (Soundararajan et al., 2014; Muneer et al., 2017; Moradtalab et al., 2019). Moreover, Si application can mitigate the electrolyte leakage of leaf tissues (Agarie et al., 1998), and alleviate heat-induced oxidative membrane damages by improving the antioxidant enzyme activities (Sattar et al., 2020). Furthermore, Si is the only element that can be translocated and accumulated in massive amounts in plants without toxic symptoms, and it is non-corrosive and pollution-free (Ma et al., 2001; Wang et al., 2016). Therefore, Si can be used as a profitable and effective fertilizer for strawberry facility cultivation.
However, Si fertilization in agricultural production is in its infancy, and it has yet to be determined how silicon affects specific internal mechanisms in plants. The concentration of Si in the shoots greatly varies among different plants. Plants are classified as either Si excluders or non-accumulators, Si intermediates, and Si accumulators according to the concentration of Si ranging from <0.5, 0.5–0.1, and more than 1.0% (v/v), respectively (Takahashi et al., 1990). All monocots are Si accumulators, and most dicots belong to the excluders or intermediates. The absorption of Si includes radial transport and release of Si. Firstly, it depends on a proteinaceous transporter for the Si transport to the cortical cells of the root from the exterior (Tamai and Ma, 2003). For instance, tomato, cucumber, and rice, respectively, represent Si non-accumulators, Si intermediates, and Si accumulators. Rice had the highest density of the Si transporter, followed by cucumbers. Therefore, the accumulation of Si in shoots depends on the ability of the roots to absorb Si (Mitani and Ma, 2005). Following absorption to the root, Si is transported into the xylem of the shoot as silicic acid. Those two processes are involved in two different types of Si transporters. Roots absorb Si into the symplast via the influx transporter low silicon rice 1 (Lsi1), and the efflux transporter Lsi2 loads the Si into the apoplast (Ma and Takahashi, 2002). In higher plants, rice Lsi1 was the first gene that was isolated by a low-Si rice mutant, and was identified to encode the Si transporter (Ma et al., 2006). In contrast to rice, little information is available in the research on Si transport in dicots, and the gene encoding the silicon transporter in strawberries was not identified until 2017 (Ouellette et al., 2017). In addition to transporter proteins, Si uptake is related to transpiration, and with the loss of water. The Si is deposited as the “phytoliths” in the leaves, stem, leaf sheath, and hull (Ma, 2003). And the “phytoliths” are able to recycle into the soil to form a soil-Si-plant mechanisms in ecosystems (Katz et al., 2021). Compared to non-agricultural soils, the agricultural soils showed lower Si concentrations due to desilication during the soil-Si-plant cycle (Puppe et al., 2021). Therefore, it is necessary to explore improved utilization of Si in limited sources. Researchers found that the method of Si application and plant conditions can greatly influence the benefits that strawberry can obtain from Si (Dallagnol et al., 2020). Combining soil and foliar Si application resulted in the greatest yield in wheat (Kowalska et al., 2021). Soil Si application was the most effective in alleviating cadmium stress (Howladar et al., 2018). Furthermore, it was reported that potassium silicate (K2SiO3) is more efficient than Na2SiO3 or CaSiO3 (Sivanesan et al., 2013). The commercial soluble silicate fertilizer ‘Keunson' also showed beneficial effects on plant growth (Vu et al., 2017; Song et al., 2021). Therefore, we are still working on finding the most suitable Si application method for enhancing the growth and stress tolerance in strawberry.
In this study, the three most common strawberry cultivars “Sulhyang”, “Maehyang”, and “Kuemsil” in Korea were investigated. “Sulhyang” was registered in 2005 and originated from the “Akihime” and “Red Pearl”, and its cultivation area occupies more than 80% of strawberries in Korea (Jun et al., 2017). “Maehyang” was bred from “Tochinomine” and “Akihime”, and showed high growth, yield, and quality (Kim et al., 2004). “Kuemsil” was derived from “Sulhyang” and “Maehyang” with the general characteristics of early flower bud differentiation and first harvest (Yoon et al., 2020). The optimum growth temperature range for strawberries is between 10 and 26°C (Strik, 1985). And it has been established that the critical temperature range for strawberry growth inhibition is 35–40°C (Kadir et al., 2006). Previous research has reported oxidative damages in “Sulhyang” and “Maehyang” under 33 and 41°C, and showed a decrease in the abundance of PSI-A core protein of PSI (PsaA) and D1 protein of PSII (PsbA) (Muneer et al., 2017). Moreover, “Sulhyang” showed necrotic leaves after subjection to 42°C (Li et al., 2020). Although there is no research on heat tolerance of “Kuemsil”, its antioxidative properties are lower than “Sulhyang” and “Maehyang” (Kim et al., 2012). Therefore, the three cultivars are thought to be sensitive to high temperatures. We applied Si for strawberry plants during the summer cutting propagation, and evaluated the growth parameters, antioxidant enzymes, and reactive oxygen species of transplants after Si application under high temperatures to preliminarily dissect the response of strawberry transplants incurred by high temperatures, and to find the optimal Si application method to enhance the resistance to high temperature stresses in strawberry transplants during hot summer seasons.
Materials and Methods
Plant Materials and Silicon Treatments
The strawberry cultivars used were “Sulhyang”, “Maehyang”, and “Kuemsil”. Daughter plants were selected from a strawberry farm (Sugok-myeon, Jinju, Gyeongsangnam-do, Korea) and transplanted in a hydroponic gutter system filled with the BVB medium (Bas Van Buuren Substrate, EN-12580, De Lier, Westland, The Netherlands) on January 10, 2021. The plants was drip-irrigated with a multipurpose nutrient solution composed of NH4NO3 80.0, KNO3 232.3, KH2PO4 272.0, K2SO4 17.4, Ca(NO3)2·4H2O 467.6, MgSO4·H2O 209.1, H3BO3 1.4, NaMoO4·2H2O 0.12, MnSO4·4H2O 2.10, ZnSO4·7H2O 0.80, and CuSO4·5H2O 0.20 (mg·L−1).
The axillary buds of mother plants developed into runners and gradually grew into daughter plants after 2 months. The Si treatments were started on March 10, 2021. Strawberry plants were subjected to one of five treatments before the cutting propagation: C, no treatments (control); SM, foliar spray of a Si solution (from K2SiO3 at a final concentration of 75 mg·L−1) to the mother plants; DM, substrate drenching with a Si solution to the mother plants; DSM, substrate dressing with a commercial soluble silicon fertilizer “Keunson” (equivalent to 0.075 g of pure Na2SiO3) (Saturn Bio Tech Co., Ltd., Gangwon-do, Korea) to the mother plants; or SD, foliar spraying of Si solution to the daughter plants. All daughter plants were stuck in 21-cell zigzag trays (21-Zigpot/21 cell tray, Daeseung, Jeonju, Korea) filled with the BVB medium (Bas Van Buuren Substrate, EN-12580, De Lier, Westland, The Netherlands) on April 30, 2020. The plants were then put into a fogging system (UH-303, JB Natural Co. Ltd., Gunpo, Korea) on a propagation bench for rooting. Two weeks later, the plants were moved out from the fogging tunnel, and the control group was separated into three groups: C-C, no Si application during cutting propagation; C-SD, no Si application before cutting propagation and the daughter plants sprayed with the Si solution after cutting propagation; C-DD, no Si application before cutting propagation and the daughter plants drenched with the Si solution after cutting propagation. The daughter plants from other treatments were separated into two groups and continued application Si: SM-C, the mother plants sprayed with the Si solution before cutting propagation and no Si application after cutting propagation; SM-SD, the mother plants sprayed with the Si solution before cutting propagation and the daughter plants sprayed with the Si solution after cutting propagation; DM-C, the mother plants drenched with the Si solution before cutting propagation and no Si application after cutting propagation; DM-DD, the mother plants drenched with the Si solution before cutting propagation and the daughter plants drenched with the Si solution; DSM-C, the mother plants dressed with soluble Si fertilizer before cutting propagation and no Si application after cutting propagation; DSM-DSD, the mother plants dressed with the soluble Si fertilizer before cutting propagation and the daughter plants dressed with the soluble Si fertilizer; SD-C, the daughter plants spray with the Si solution before cutting propagation and no Si application after cutting propagation; or SD-SD, the daughter plants sprayed with the Si solution before and after cutting propagation (Figure 1 and Table 1).
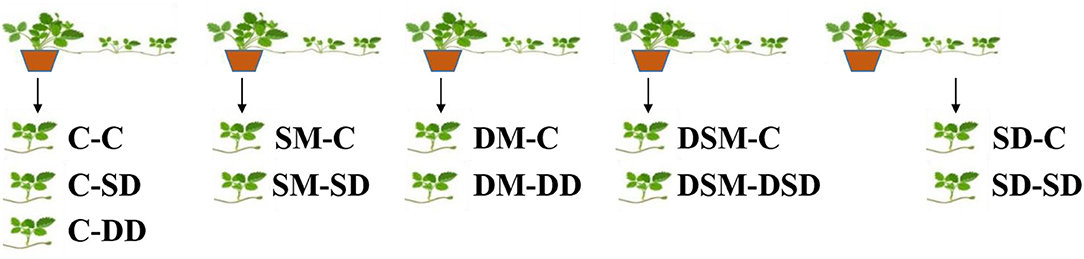
Figure 1. Methods of Si application for strawberries. Respectively: C-C, no Si application during cutting propagation; C-SD, no Si application before cutting propagation and the daughter plants sprayed with the Si solution after cutting propagation; C-DD, no Si application before cutting propagation and the daughter plants drenched with the Si solution after cutting propagation; SM-C, the mother plants sprayed with the Si solution before cutting propagation and no Si application after cutting propagation; SM-SD, the mother plants sprayed with the Si solution before cutting propagation and the daughter plants sprayed with the Si solution after cutting propagation; DM-C, the mother plants drenched with the Si solution before cutting propagation and no Si application after cutting propagation; DM-DD, the mother plants drenched with the Si solution before cutting propagation and the daughter plants drenched with the Si solution after cutting propagation; DSM-C, the mother plants dressed with the soluble Si fertilizer before cutting propagation and no Si application after cutting propagation; DSM-DSD, the mother plants dressed with the soluble Si fertilizer before cutting propagation and the daughter plants dressed with the soluble Si fertilizer after cutting propagation; SD-C, the daughter plants sprayed with the Si solution before cutting propagation and no Si application after cutting propagation; or SD-SD, the daughter plants sprayed the Si solution before and after cutting propagation.
High Temperature Stress Treatments and Measurements of the Chlorophyll Fluorescence Parameters
The daughter plants were transplanted into 10 cm plastic pots for high temperature experiments on 11 June 2021. Two weeks later, transplants were randomly placed in plant growth chambers (C1200H3, FC Poibe Co. Ltd., Seoul, Korea) for 1 week under a condition of 43°C, a 16-hour photoperiod with a light intensity of 300 mmol·m−2·s−1 PPFD, and 65% relative humidity. The plants were fertigated with 50 mL per plant of a multipurpose nutrient solution everyday. There were 3 replicates and each replicate had 3 transplants, a total of 9 plants for each treatment. All plants were randomly placed in the growth chambers. The chlorophyll fluorescence parameters were measured every 48 hours using a portable fluorometer (FluorPen FP110, Photon Systems Instruments, Drásov, Czech Republic).
Measurement of the Growth Parameters
The plant height, crown diameter, fresh and dry weights of the shoot, length and width and stomatal conductance of leaves of the transplants were measured. The stomatal conductance was determined using a Decagon Leaf Porometer SC-1 (Decagon Device Inc., Pullman, WA, USA). The fresh weight was measured with an electronic scale (EW 220-3NM, Kern and Sohn GmbH., Balingen, Germany), The dry weights of shoot and roots were measured after drying for 72 hours in a drying oven (Venticell-222, MMM Medcenter Einrichtungen GmbH., Munich, Germany) at 70°C. The relative water content (LRWC) was determined using the following formula (González and González-Vilar, 2001):
Determination of the Contents of Chlorophyll, Carotenoid, and Total Anthocyanin
The content of chlorophyll was measured according to Sükran et al. (1998). A 0.1 g leaf sample was submerged in 80% (v/v) acetone for 12 hours, which was then centrifuged (Centrifuge 5428, Eppendorf, Hamburg, Germany) at 13,000 rpm for 15 min at 4°C, and the absorbance of the liquid supernatant was measured at 645 and 663 nm using a UV-spectrophotometer (Libra S22, Biochrom Ltd., Cambridge, UK). The contents of chlorophyll a and b were determined using the following formulae:
(*V, the volume of the extraction solution. The pigment content was expressed as mg of chlorophyll a and b, and carotenoid per gram of fresh leaf weight).
The total anthocyanin content was determined according to Pirie and Mullins (1976). Briefly, 0.5 g leaf tissue was extracted in 2 mL of 1% (v/v) HCl-ethanol and centrifuged at 13,000 rpm for 20 min at 4°C. Then the liquid supernatant was acidified to approximately pH 1.0 using 0.1 N HCl, and the absorbance was measured at 530 nm.
Determination of the Contents of Micro- and Macro-Nutrients
The Si content was determined according to the methods of Zhang and Dotson (1994). Briefly, dried leaves and roots in an oven at 60°C and ground, then 0.5 g samples were ashed using a nabertherm muffle furnace (Model LV 5/11/B180, Lilienthal, Breman, Germany) at 525°C for 4 h. Afterward, the ash was dissolved into 5 mL 25% (v/v) HCl, and then diluted with 15 mL of warm distilled water and 10 mL of room temperature distilled water. The nutrient contents of Si, potassium (K), calcium (Ca), phosphorus (P), sulfur (S), magnesium (Mg), iron (Fe), zinc (Zn), copper (Cu), and manganese (Mn) were measured using the inductively coupled plasma spectrometer (Optima 4300DV/5300DV, Perkin Elmer, Germany).
Determination of the Hydrogen Peroxide (H2O2) Content and Lipid Peroxidation
To estimate the content of H2O2, 0.1 g leaf samples were homogenized and extracted in a 5 mL 10 mM phosphate buffer (pH 5.8) containing 0.1% (w/v) trichloroacetic acid (TCA), which was then centrifuged at 10,000 rpm for 30 min at 4°C. 2 mL of the liquid supernatant was mixed with a 0.2 mL 10 mM phosphate buffer (pH 5.8) and 0.2 mL 1 M potassium iodide (KI). After incubation in the dark for 30 min, the absorbance was measured at 350 nm (Junglee et al., 2014).
Lipid peroxidation was measured according to Sun et al. (2015). 0.5 g leaf samples were extracted in 5 mL 0.1% (w/v) TCA and then centrifuged at 10,000 g for 5 min. 1 mL of the liquid supernatant was mixed with 0.6% (w/v) thiobarbituric acid and 10% (w/v) TCA, which were subsequently placed in a boiling water bath for 30 min to react and rapidly cooled. The absorbance was measured at 450, 532, and 600 nm, and the lipid peroxidation was estimated by the malondialdehyde (MDA) level:
(The content of MDA was expressed as μmol per gram of fresh leaf weight).
Determination of Proline and Soluble Sugar Contents
To estimate the proline content, 1.0 g leaf samples were homogenized and extracted in 10 mL 3% (w/v) sulfosalicylic acid. 1 mL of the liquid supernatant was then mixed with 1 mL acid ninhydrin and 1 mL glacial acetic acid, and reacted in a 95°C water bath for 1 h. After natural cooling at room temperature, 2 mL cold toluene was added and the absorbance was measured at 520 nm (Bates et al., 1973).
To estimate the soluble sugar contents, 0.5 g leaf samples were homogenized and extracted in a 10 mL 20 mM phosphate buffer (pH 7.0), and centrifuged at 10,000 rpm for 30 min at 4°C. 0.2 mL of the liquid supernatant was then mixed with 5 mL H2SO4 and 1.8 mL distilled H2O, and reacted in a boiling water bath for 10 min. After natural cooling, the absorbance was measured at 620 nm (Sun et al., 2015).
Determination of the Total Soluble Protein Content and Antioxidant Enzyme Activities
To estimate the total soluble protein content, the 0.5 g leaf samples were homogenized with liquid nitrogen and extracted in a 1.5 mL ice-cold 50 mM phosphate buffer (pH 7.0) containing 1 mM ethylenediaminetetraacetic acid (EDTA), 0.05% (v/v) Triton X-100, and 1 mM polyvinylpyrrolidone (PVP), which was then centrifuged at 13,000 rpm for 20 min at 4°C, and the liquid supernatant was used in assaying the antioxidant enzyme activities, namely of superoxide dismutase (SOD), guaiacol peroxidase (GPX), ascorbate peroxidase (APX), and catalase (CAT) according to Soundararajan et al. (2019).
Data Collection and Analysis
The experimental results were subjected to an analysis of variance (ANOVA) and the Duncan's multiple range test (p ≤ 0.05) for comparison within a cultivar, and the F-test was calculated according to Fisher's least significant difference test at a threshold of p = 0.05. The Statistical Analysis Program (SAS 9.1, SAS Institute Inc., Cary, NC, USA) was used for the statistical analysis. Graphing was performed with the OriginPro software (version 9.0, OriginLab Co. Ltd., Northampton, MA, USA).
Results
Growth and Development of Strawberry Transplants
In the present experiment, the height, fresh and dry weights, and shoot crown diameter of the three strawberry cultivars all significantly increased with Si treatments when compared with the control (Table 2). The maximal shoot fresh and dry weights were observed when plants were drenched in the Si solution both before and after the cutting propagation (DM-DD). The F-test results revealed that the supplemental Si significantly affected the shoot height, crown diameter, and fresh weight. However, Si treatments had no significant effects on the leaf length. Furthermore, as shown in Figure 2, the morphophysiological state is affected by the Si treatments in ‘Sulhyang”, “Maehyang”, and “Kuemsil' strawberry transplants subjected to 43°C on different days. The leaves of transplants began to slightly roll from the third day of the high temperature stress. On the fifth day of the high temperature stress, varying degrees of wilting were observed on the transplants. It is noteworthy that the plants in the control were severely stressed and gradually died, while the Si-treated plants remained alive on the seventh day of the high temperature stress.
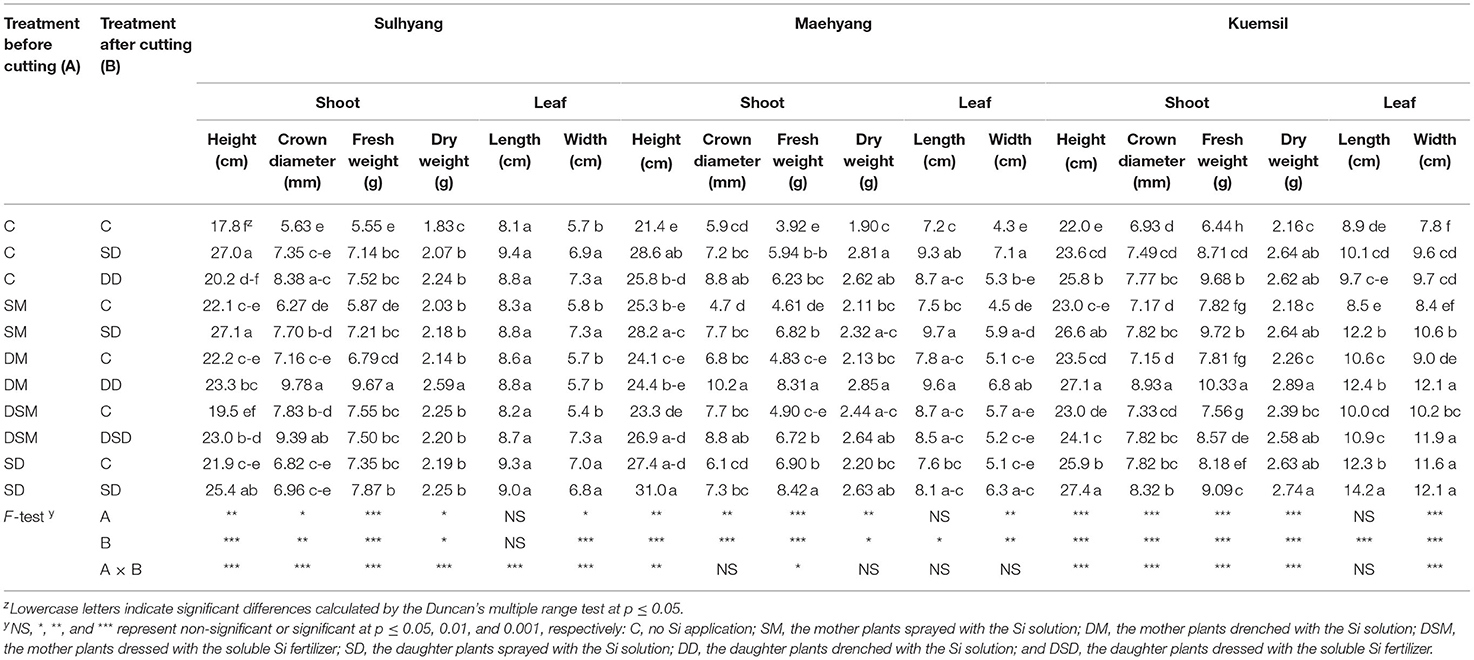
Table 2. The growth parameters as affected by the Si treatment in strawberry transplants after subjection to 43°C for 7 days.
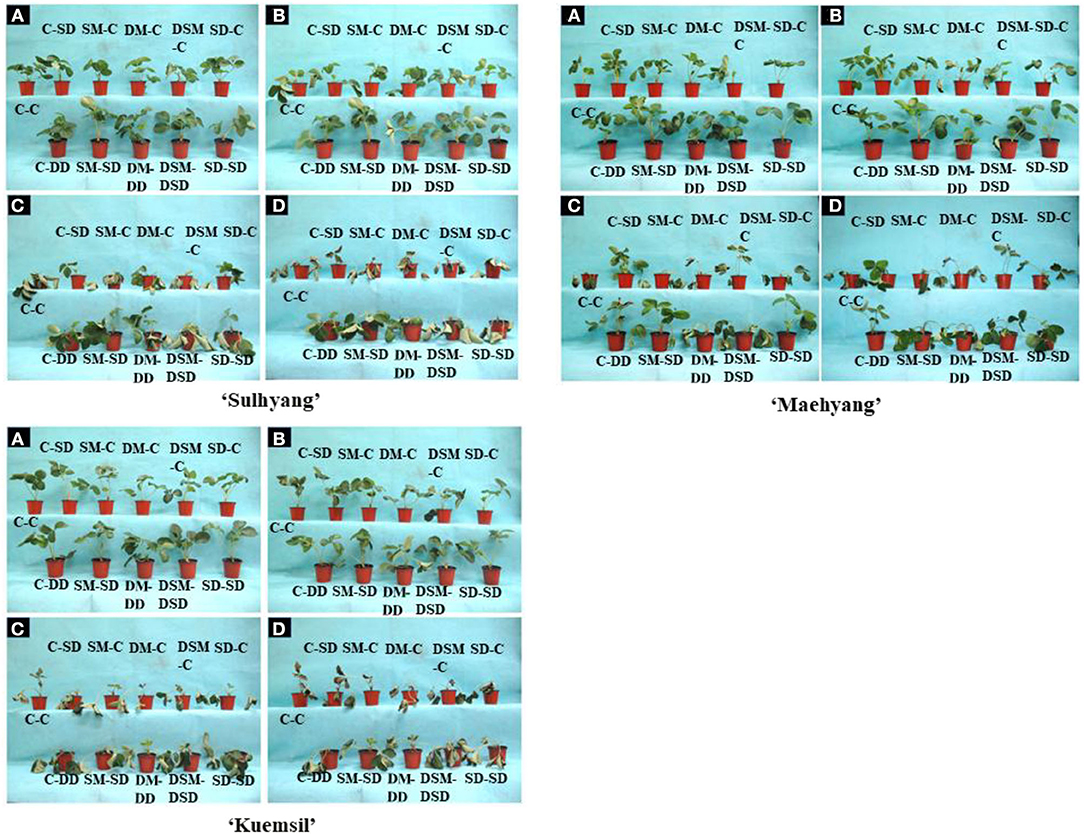
Figure 2. Morphophysiological state as affected by the Si treatment in strawberry transplants after subjection to 43°C for 1 (A), 3 (B), 5 (C), and 7 (D) days. See Figure 1 for descriptions of treatment codes.
Relative Water Content and Stomatal Conductance
The LRWC in leaves is considered as a degree of stress expressed under high temperatures. As compared with the control (Figure 3), the LRWC values increased by 26–45%, 56–75%, and 70–84% in “Sulhyang”, “Maehyang”, and “Kuemsil” cultivars, respectively, with Si (+, +) application. Meanwhile, we found that Si treatments increased the stomatal conductance of leaves compared to the control, and the greatest stomatal conductance was obtained with the SD-SD.
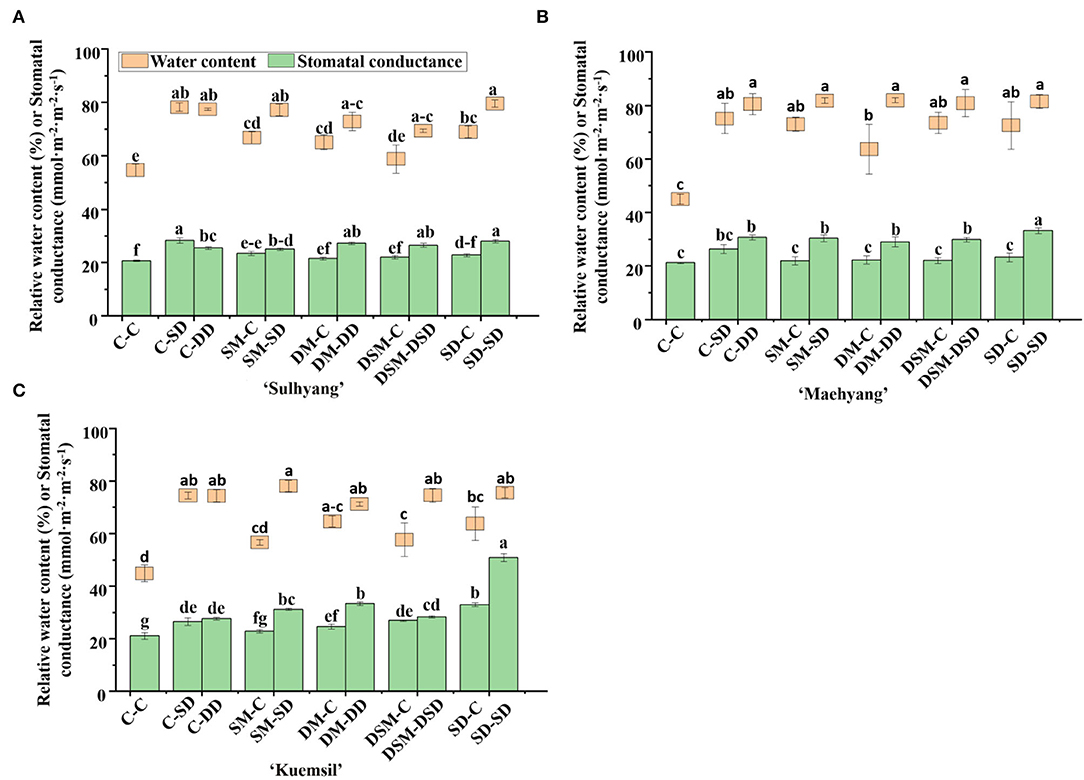
Figure 3. Leaves relative water content and stomatal conductance of strawberry transplants (A) “Sulhyang”; (B) “Maehyang”; and (C) “Kuemsil” as affected by the Si treatment after subjection to 43°C for 7 days. Lowercase letters indicate significant differences calculated by the Duncan's multiple range test at p ≤ 0.05. See Figure 1 for descriptions of treatment codes.
Chlorophyll Fluorescence Parameters and Photosynthetic Pigment Contents
We further studied the changes in the chlorophyll fluorescence parameters of the photosystem II (PS II) under high temperature stress (Figure 4). The maximum primary yield of PS II photochemistry (Fv/F0) and the chlorophyll fluorescence parameters of the maximum/potential quantum efficiency of PS II (Fv/Fm) significantly decreased. The Fv/F0 and Fv/Fm decreased relatively slowly for the first three days, and then rapidly decreased until the fifth day. Interestingly, there were no significant differences in the Fv/F0 and Fv/Fm values between the control and Si-treated transplants on the first and third day, whereafter, the Fv/F0 and Fv/Fm of the control transplants (0.92 and 0.43), were 58% and 48% lower than that of Si-treated transplants (1.42 and 0.64), respectively.
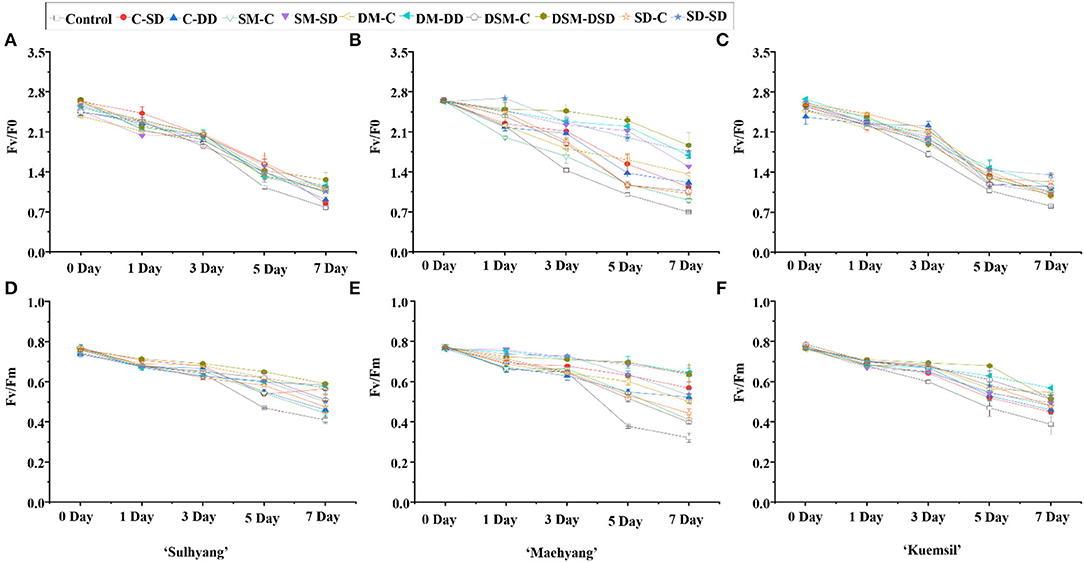
Figure 4. Chlorophyll fluorescence Fv/F0 and Fv/Fm of strawberry transplants (A,D) “Sulhyang”; (B,E) “Maehyang”; and (C,F) “Kuemsil” as affected by the Si treatment after subjection to 43°C for 0, 1, 3, 5, and 7 days. See Figure 1 for descriptions of treatment codes.
To further explore the influence of the Si and high temperature stress on photosynthesis, the chlorophyll contents of leaves were investigated in this study (Figure 5). The lowest total chlorophyll contents were observed in the control, regardless of the cultivar. Similarly, the chlorophyll a content in “Sulhyang” (0.39–0.91 mg·g−1), “Maehyang” (0.68–2.29 mg·g−1), and “Kuemsil” (0.54~0.95 mg·g−1) transplants with Si application were, respectively, 1.3–3.1, 1.3–4.5, and 1.2–2.0 times those in the control (0.29, 0.50, and 0.46 mg·g−1). In addition, the chlorophyll b content in “Sulhyang” and “Maehyang” leaves were found to be significantly affected by the Si application. However, for “Kuemsil” transplants exposed to the high temperature stress, there were no significant differences in the chlorophyll a content with or without the Si treatments.
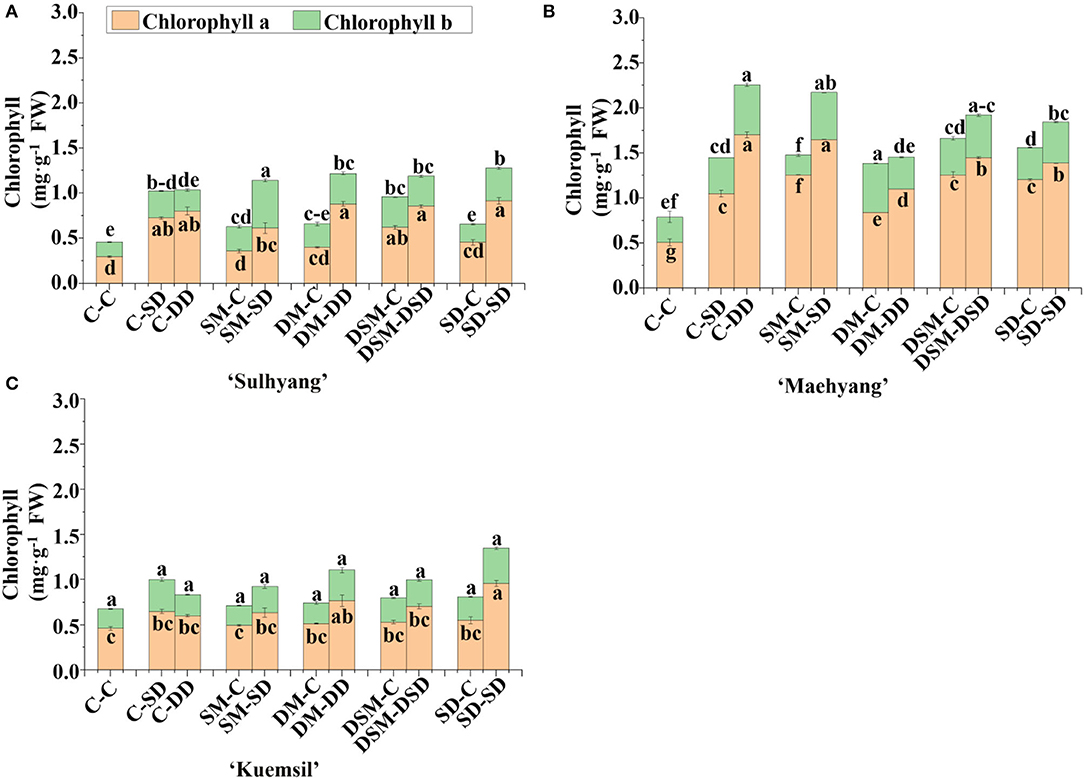
Figure 5. Chlorophyll content of strawberry transplants (A) “Sulhyang”; (B) “Maehyang”; and (C) “Kuemsil” as affected by Si treatment after subjection to 43°C for 7 days. Lowercase letters indicate significant differences calculated by the Duncan's multiple range test at p ≤ 0.05. See Figure 1 for descriptions of treatment codes.
Moreover, there were significant differences in the carotenoid content (Figure 6). In “Sulhyang”, “Maehyang”, and “Kuemsil”, the carotenoid content with Si (+, +) treatments (2.12, 2.24, and 2.07 mg·g−1) were, respectively, 1.1, 1.0, and 0.6 times higher than those of the control transplants (1.02, 1.09, and 1.26 mg·g−1). However, there were no significant differences in the content of carotenoids in transplants Si (+,–) and Si (–,–).
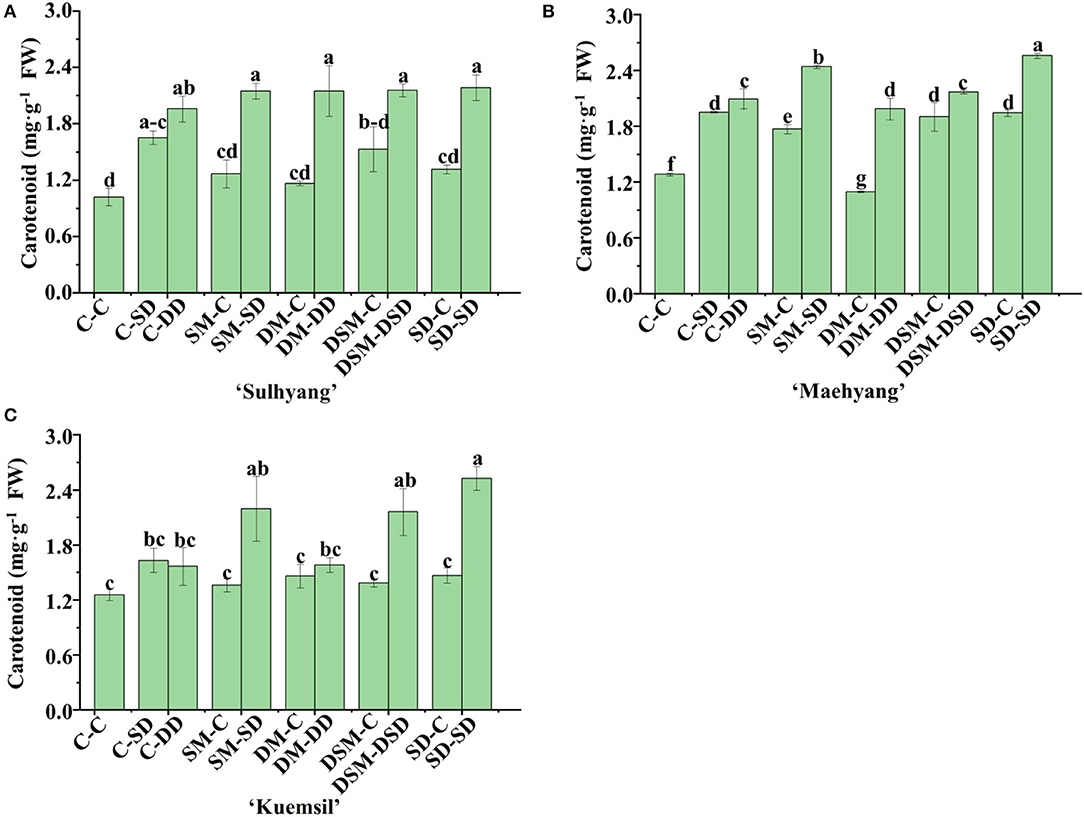
Figure 6. Carotenoid content of strawberry transplants (A) “Sulhyang”; (B) “Maehyang”; and (C) “Kuemsil” as affected by the Si treatment after subjection to 43°C for 7 days. Lowercase letters indicate significant differences calculated by the Duncan's multiple range test at p ≤ 0.05. See Figure 1 for descriptions of treatment codes.
Soluble Sugars and Soluble Proteins
The results related to the accumulation of soluble sugars and proteins showed significant effects of Si application (Figure 7). Si application promoted the soluble sugar contents, and SD-SD led to the highest soluble sugar contents in “Sulhyang” and “Kuemsil”. However, there were no significant differences in the content of soluble proteins in transplants treated with or without Si after subjection to the high temperature in “Maehyang”.
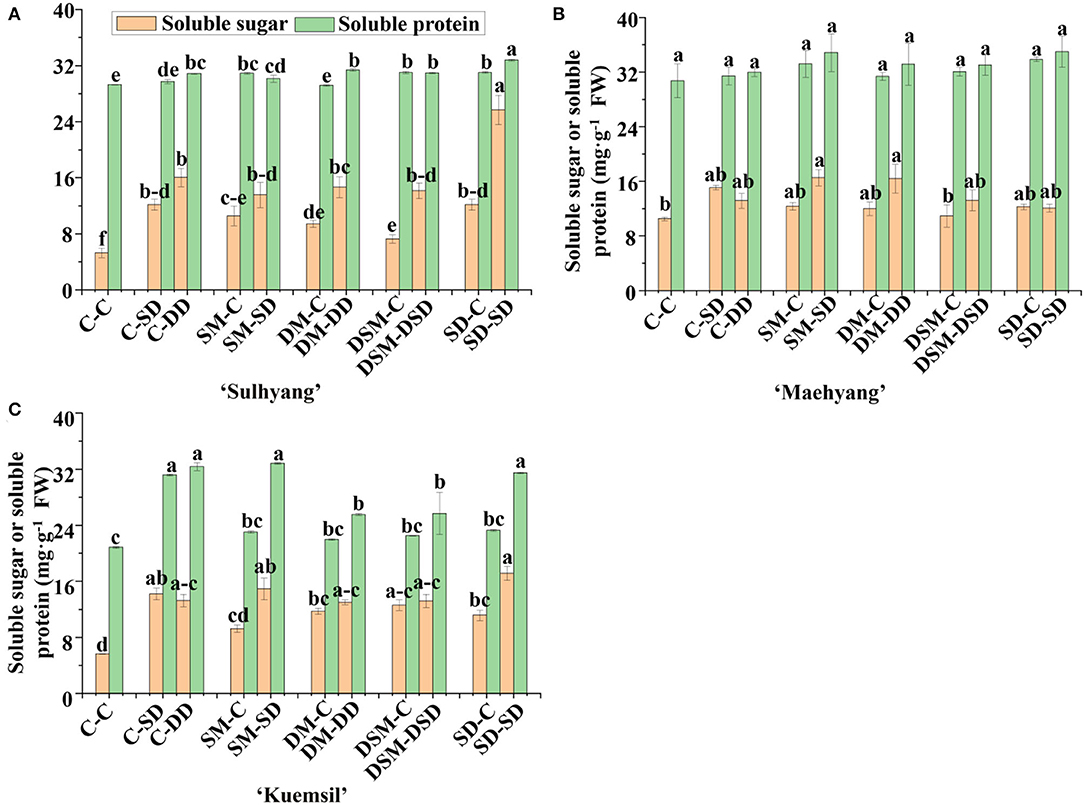
Figure 7. Soluble sugar and protein contents in leaves of strawberry transplants (A) “Sulhyang”; (B) “Maehyang”; and (C) “Kuemsil” as affected by the Si treatment after subjection to 43°C for 7 days. Lowercase letters indicate significant differences calculated by the Duncan's multiple range test at p ≤ 0.05. See Figure 1 for descriptions of treatment codes.
Contents of Micro- and Macro-Nutrients
The contents of micro- and macro-nutrients in leaves and roots were measured (Figures 8, 9 and Tables 3–5). Si application promoted the Si content both in leaves and roots, and the maximum Si content was obtained in DM-DD, followed by C-DD and SD-SD. Furthermore, the Si content in leaves was higher than that of roots in transplants treated with Si, for all three cultivars. However, in “Sulhyang”, the Si content of the roots was no differences compared with those in the control after the foliar spray. The results of F-test showed that Si application significantly affected the macro-nutrient contents in “Maehyang” and “Kuemsil”, and Si increased the absorption and transport of K, P, S, and Mg. For micro-nutrients, Si significantly affected Fe transport from roots to leaves.
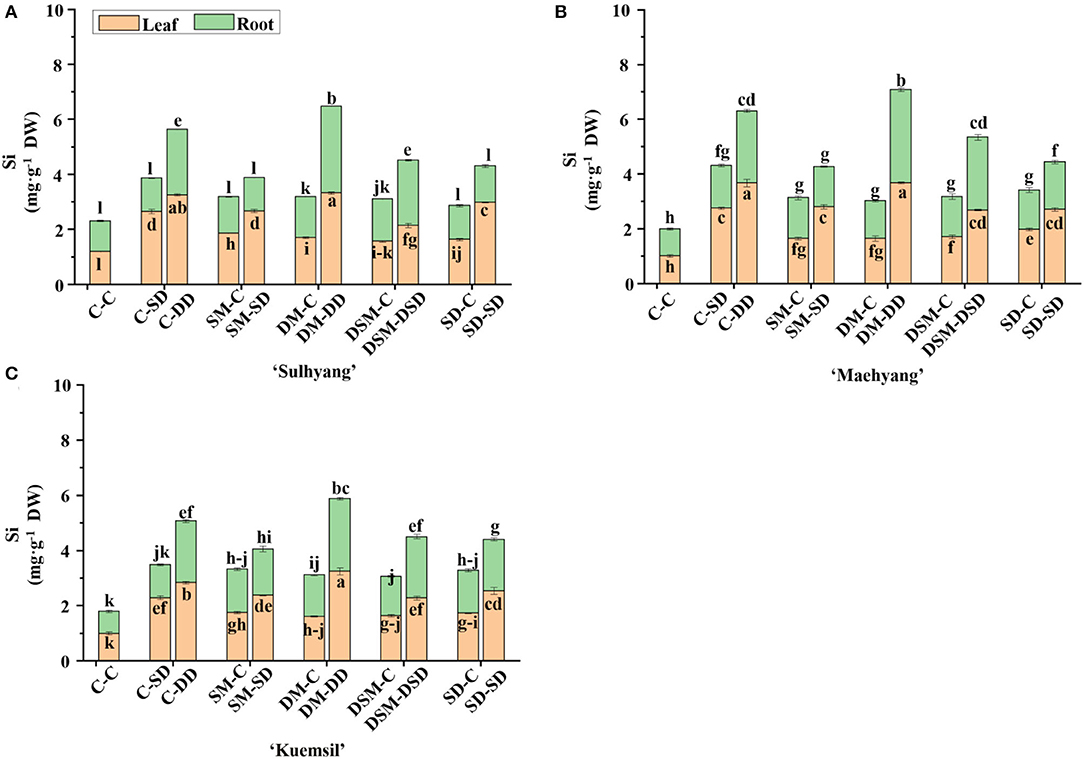
Figure 8. The Si content in leaves and root of strawberry transplants (A) “Sulhyang”; (B) “Maehyang”; and (C) “Kuemsil” as affected by the Si treatments subjected to 43°C for 7 days. Lowercase letters indicate significant differences calculated by the Duncan's multiple range test at p ≤ 0.05. See Figure 1 for descriptions of treatment codes.
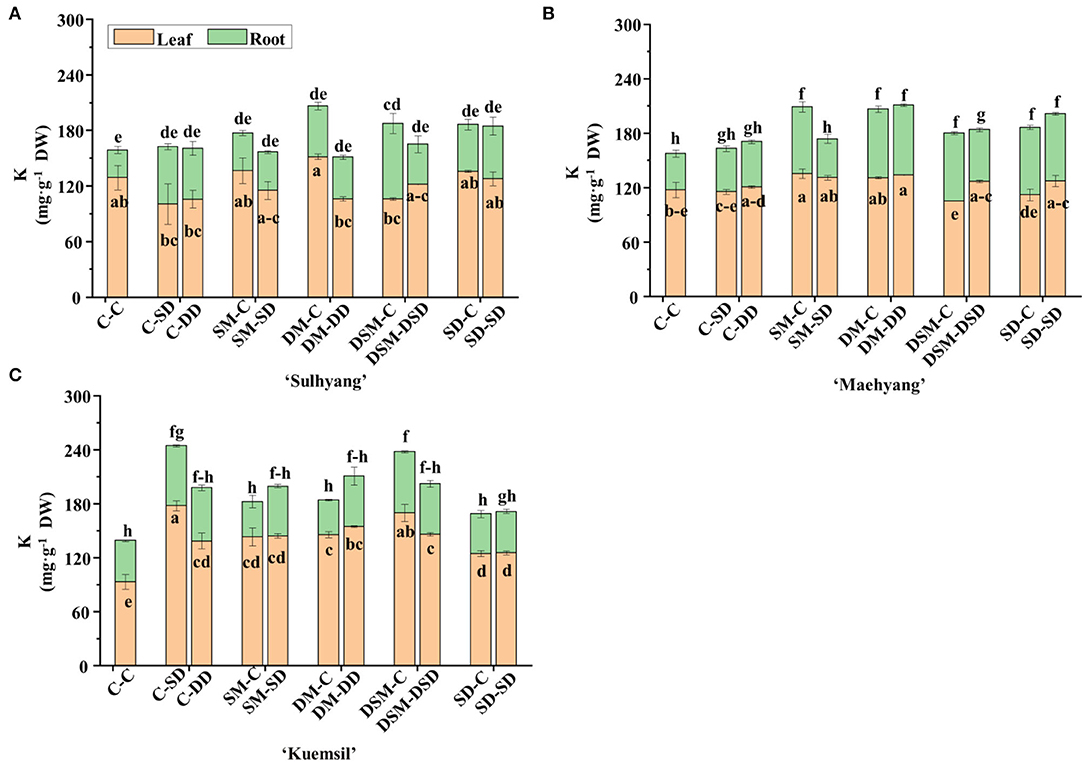
Figure 9. The K content in leaves and root of strawberry transplants (A) “Sulhyang”; (B) “Maehyang”; and (C) “Kuemsil” as affected by the Si treatments subjected to 43°C for 7 days. Lowercase letters indicate significant differences calculated by the Duncan's multiple range test at p ≤ 0.05. See Figure 1 for descriptions of treatment codes.
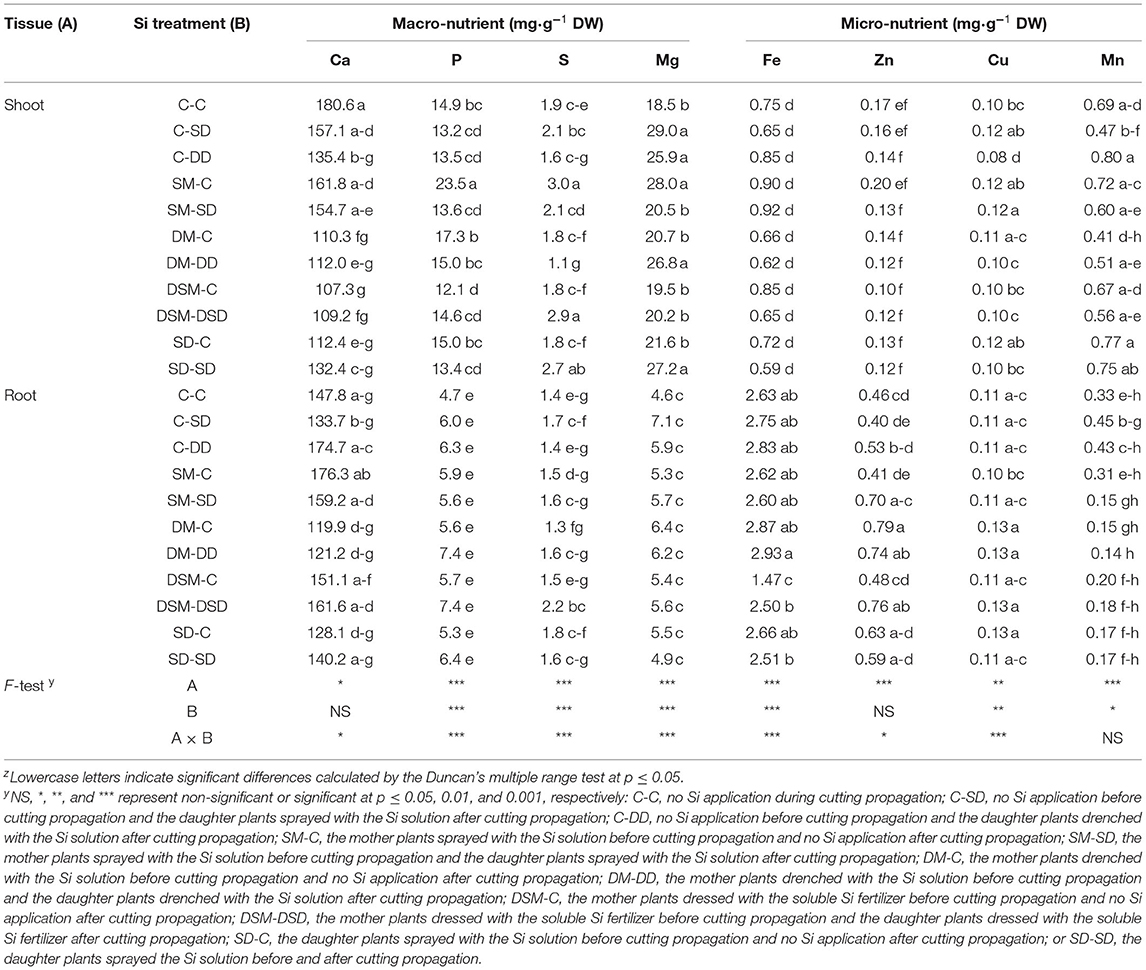
Table 3. Mineral contents in leaves and root of “Sulhyang” transplants as affected by different Si treatments.
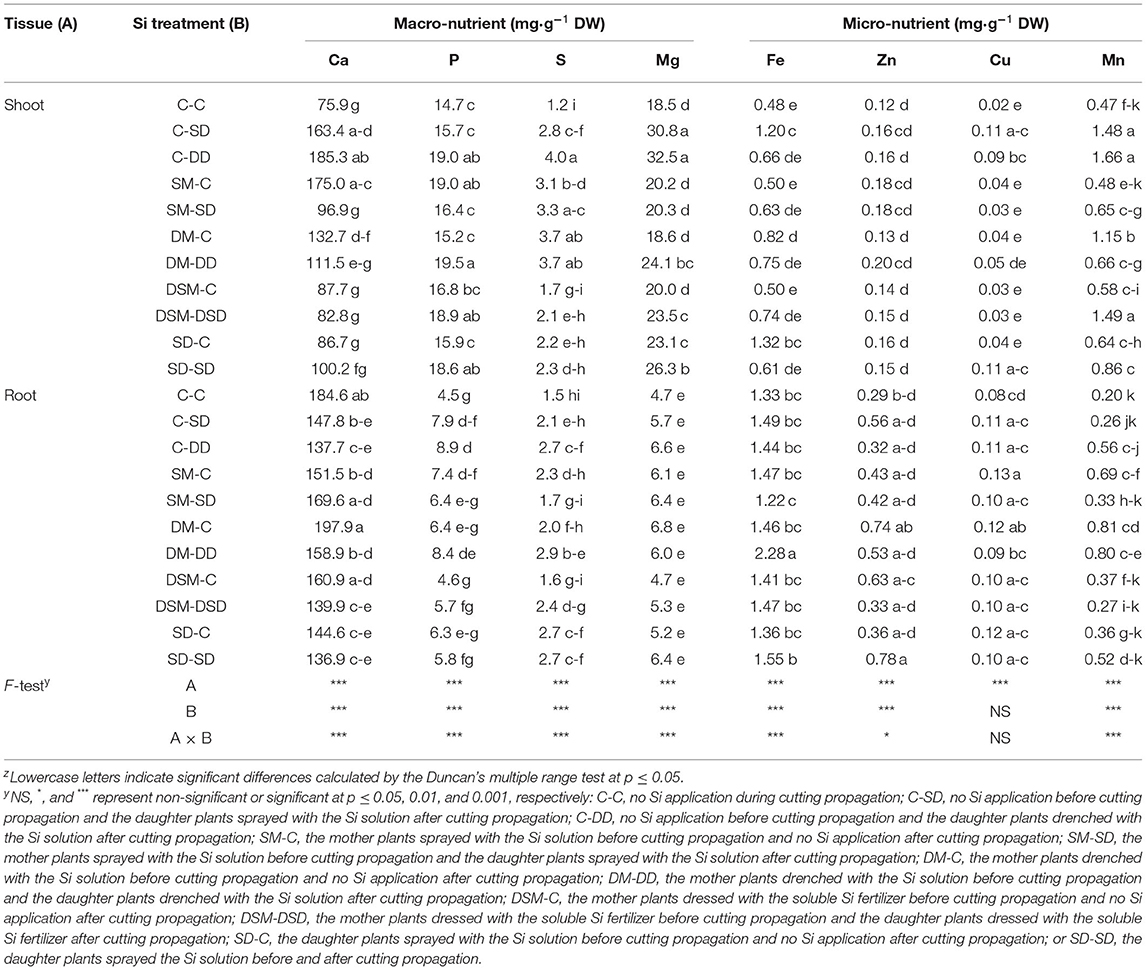
Table 4. Mineral contents in leaves and root of “Maehyang” transplants as affected by different Si treatments.
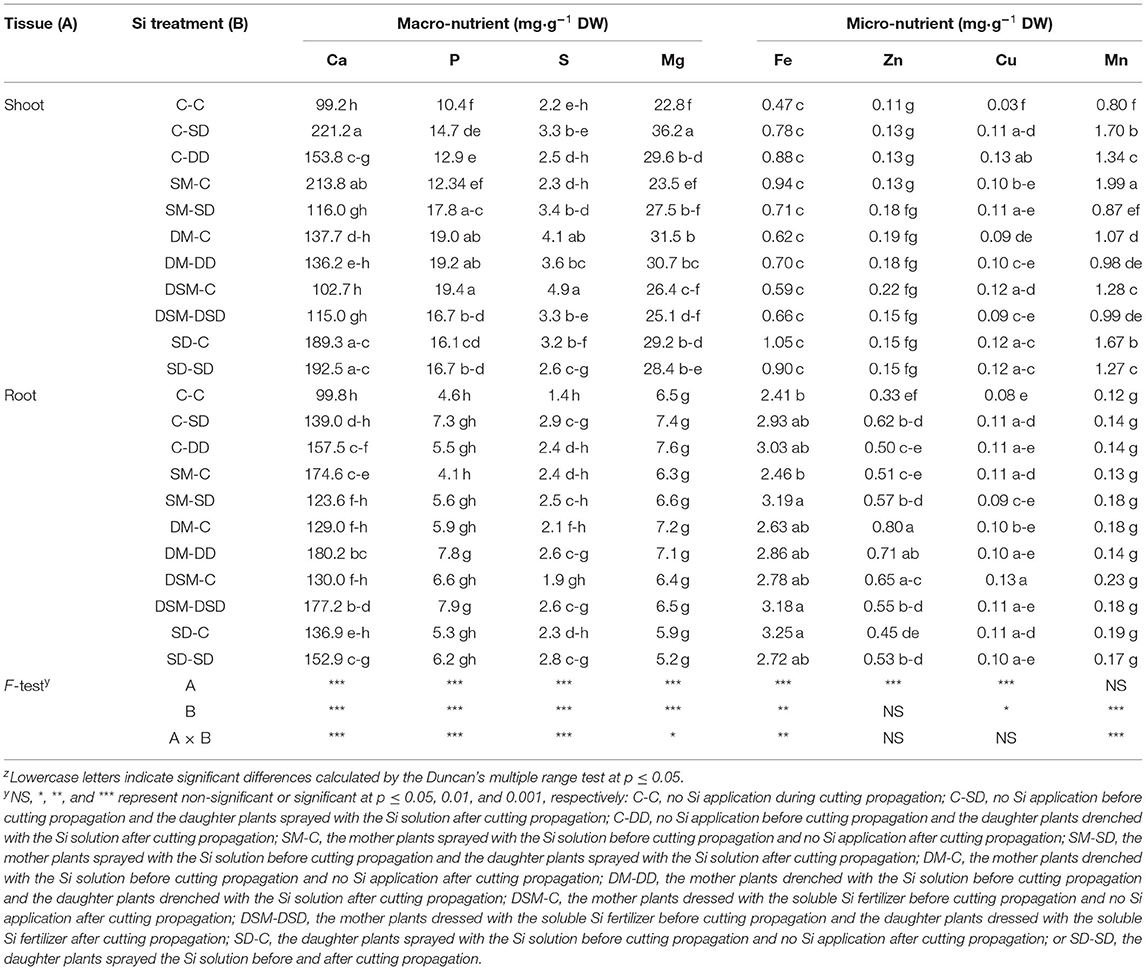
Table 5. Mineral contents in leaves and root of “Kuemsil” transplants as affected by different Si treatments.
Reactive Oxygen Species
ROS is closely related to the degree of damage caused by stresses. Figure 8 presents the H2O2 and MDA contents of the strawberry transplants measured after the high temperature stress. On average, Si application substantially reduced the MDA content of transplants (by about 85, 72, and 62%, respectively, compared to the control). Differently, although the H2O2 content decreased in the Si (+, +) treatments, there were no significant differences in the ROS contents between Si (+, −) and Si (−, −) treatments in “Sulhyang” and “Kuemsil”.
Antioxidant Enzyme Activities
Significant differences in the activities of certain key enzymes were found in the transplants with Si application (Figures 10–13). All Si treatments lead to significantly higher SOD activities than in the control (by 33–62%) in “Maehyang” (Figure 12D). Moreover, the CAT and APX activities as affected by the Si (+, +) treatments in “Sulhyang” (0.23 and 0.52 U·mg−1), “Maehyang” (1.05 and 0.37 U·mg−1), and “Kuemsil” (0.26 and 0.47 U·mg−1) were, respectively, 1.0 and 0.9, 3.8 and 1.65, and 0.9 and 0.76 times higher than those of the control (0.11 and 0.27, 0.21 and 0.14, and 0.14 and 0.26 U·mg−1) (Figures 11A,B). Overall, Si application resulted in higher activities of antioxidant enzymes.
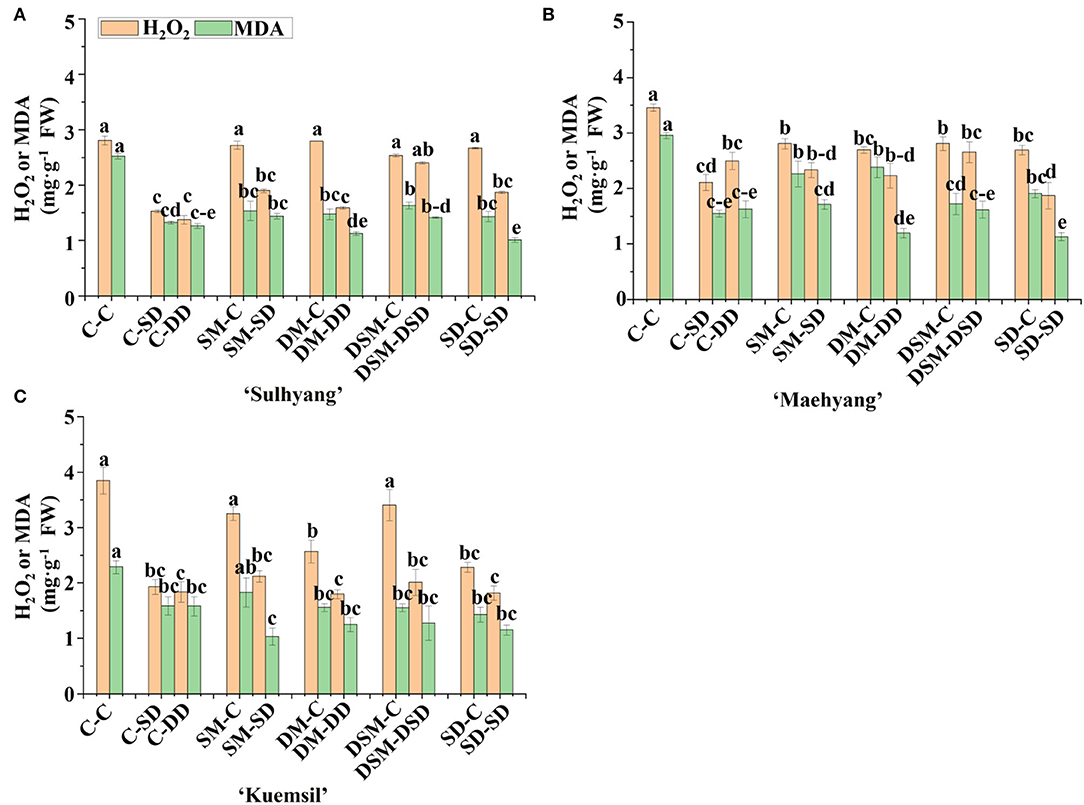
Figure 10. The H2O2 and MDA contents in leaves of strawberry transplants (A) “Sulhyang”; (B) “Maehyang”; and (C) “Kuemsil” as affected by the Si treatment after subjection to 43°C for 7 days. Lowercase letters indicate significant differences calculated by the Duncan's multiple range test at p ≤ 0.05. See Figure 1 for descriptions of treatment codes.
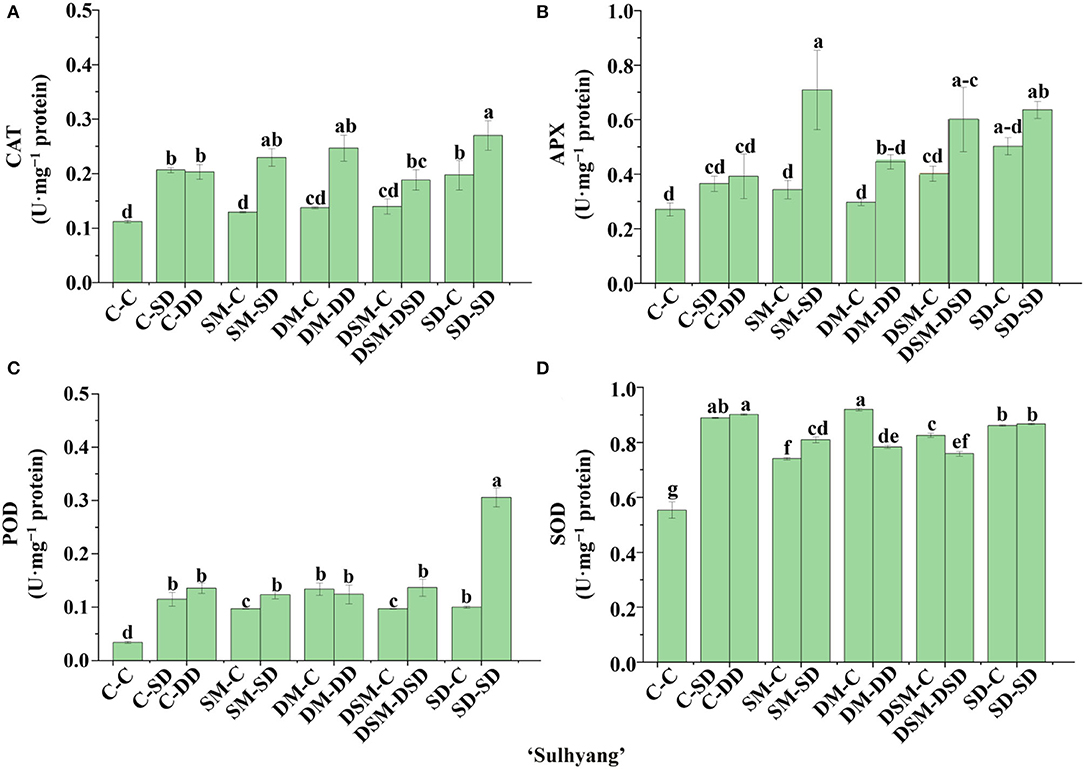
Figure 11. Activities of CAT (A), APX (B), POD (C), and SOD (D) in leaves of “Sulhyang” as affected by the Si treatment after subjection to 43°C for 7 days. Lowercase letters indicate significant differences calculated by the Duncan's multiple range test at p ≤ 0.05. See Figure 1 for descriptions of treatment codes.
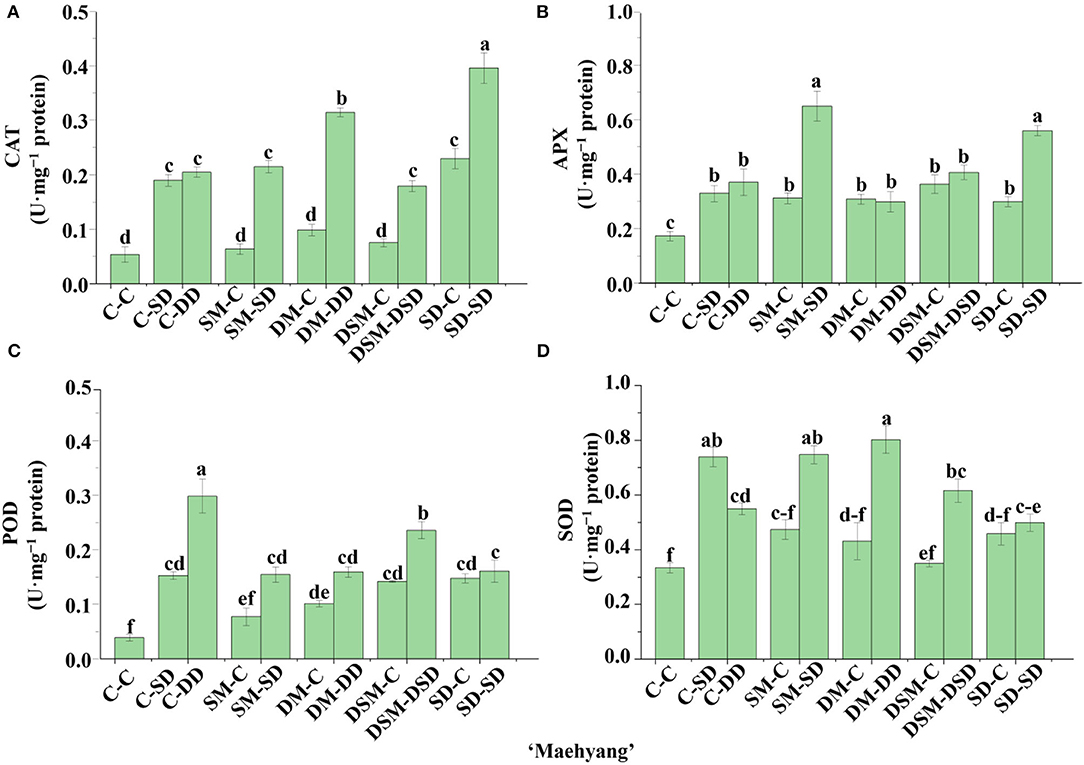
Figure 12. Activities of CAT (A), APX (B), POD (C), and SOD (D) in leaves of “Maehyang” as affected by the Si treatment after subjection to 43°C for 7 days. Lowercase letters indicate significant differences calculated by the Duncan's multiple range test at p ≤ 0.05. See Figure 1 for descriptions of treatment codes.
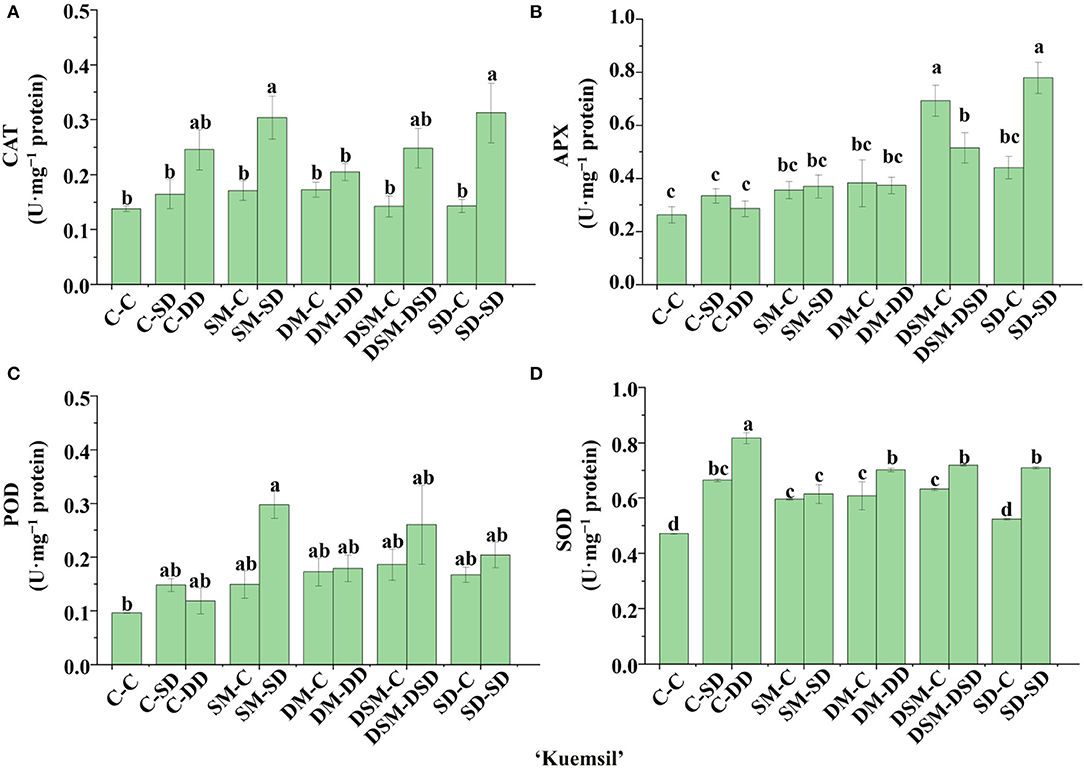
Figure 13. Activities of CAT (A), APX (B), POD (C), and SOD (D) in leaves of “Kuemsil” as affected by the Si treatment after subjection to 43°C for 7 days. Lowercase letters indicate significant differences calculated by the Duncan's multiple range test at p ≤ 0.05. See Figure 1 for descriptions of treatment codes.
Anthocyanin and Proline
The trend of anthocyanin and proline contents was opposite to that of H2O2 and MDA contents (Figures 14, 15). The anthocyanin content in Si (+, +) (2.74, 3.20, and 2.40 mg·g−1) were, respectively, 1.45, 1.34, and 0.53 times higher than that of the control (1.11, 1.37, and 1.57 mg·g−1). Similarly, the Si (+, +) treatments led to higher proline contents than the Si (+, –) treatments, and the SD-SD resulted in the maximum proline value for all three cultivars. However, the proline content of the treatments SM-C, SM-C, and DSM-C were significantly decreased than the control in “Sulhyang”.
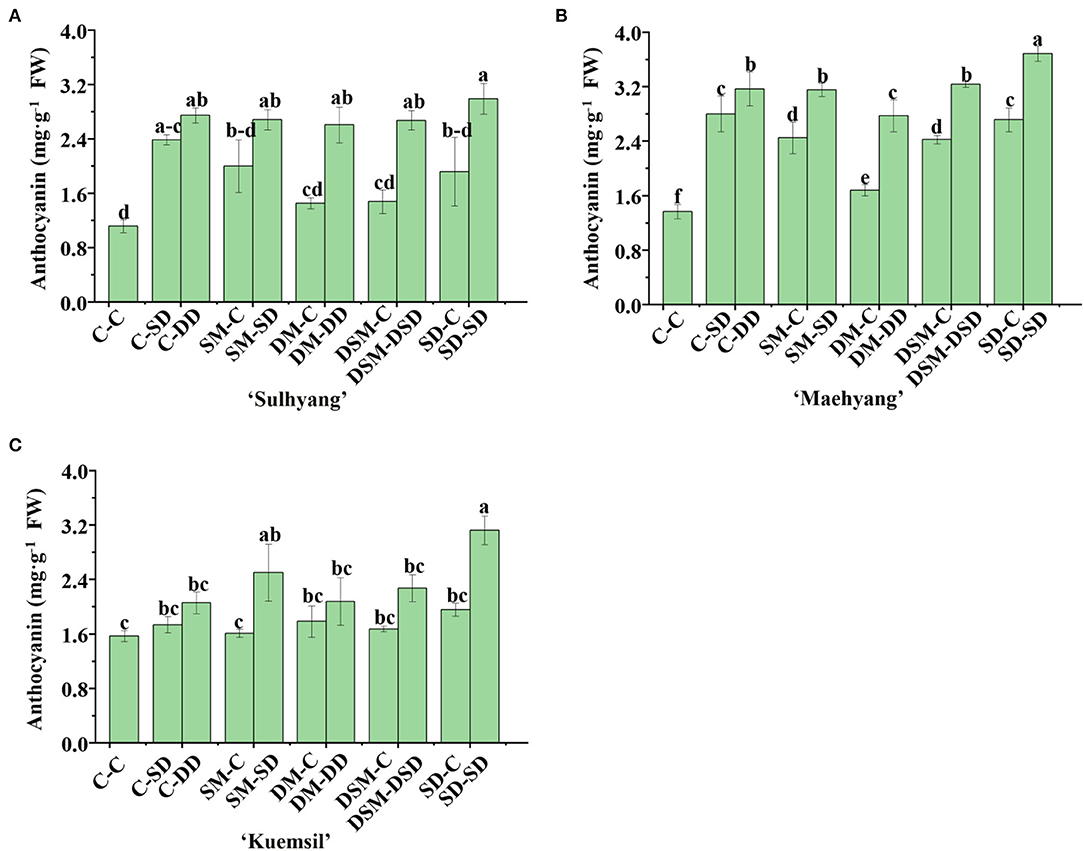
Figure 14. Anthocyanin content in leaves of strawberry transplants (A) “Sulhyang”; (B) “Maehyang”; and (C) “Kuemsil” as affected by the Si treatment after subjection to 43°C for 7 days. Lowercase letters indicate significant differences calculated by the Duncan's multiple range test at p ≤ 0.05. See Figure 1 for descriptions of treatment codes.
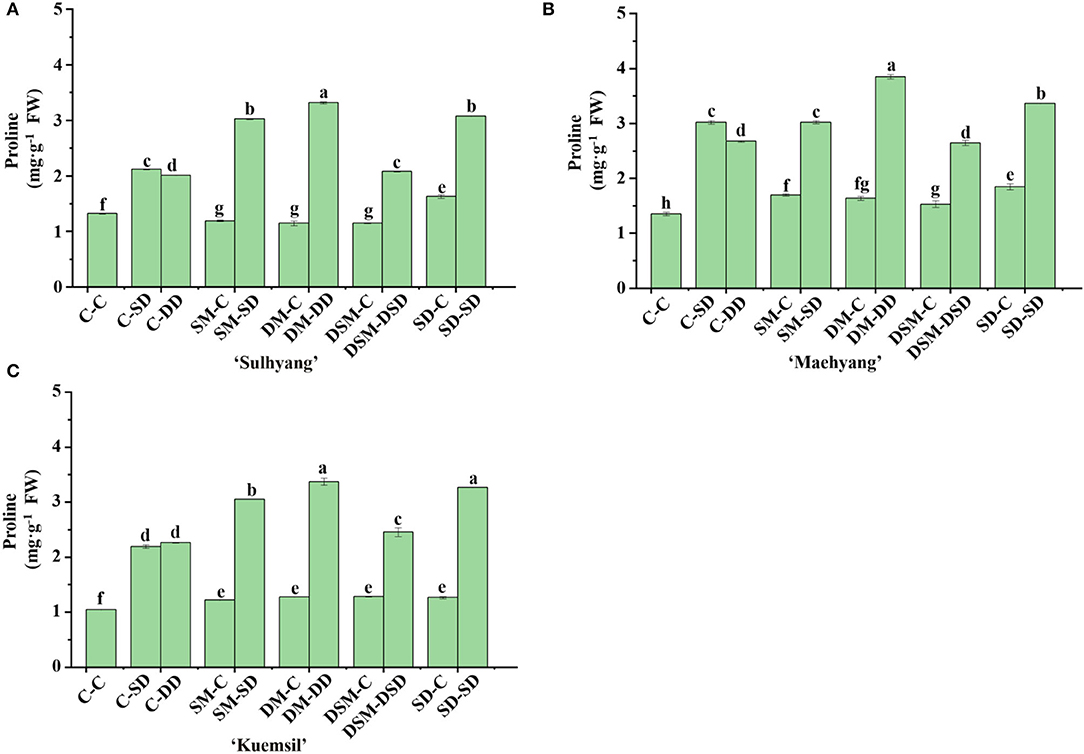
Figure 15. Proline content in leaves of strawberry transplants (A) “Sulhyang”; (B) “Maehyang”; and (C) “Kuemsil” as affected by the Si treatment after subjection to 43°C for 7 days. Lowercase letters indicate significant differences calculated by the Duncan's multiple range test at p ≤ 0.05. See Figure 1 for descriptions of treatment codes.
Discussion
Temperature affects the membrane properties, enzyme activity levels, and chemical reactions of life processes, and plants adapt to high temperature stresses by modifying photosynthesis, synthesis and accumulation of primary and secondary metabolites, and induction of stress proteins (Wahid et al., 2012). It is considered that Si induces high temperature stress tolerance by enhancing these abilities (Chen et al., 2011).
Analysis of the High Temperature and Si Application on Strawberry Photosynthesis, Growth, and Development
The growth, development, and survival of plants depend on the temperature. In general, high temperature damages the balance in photosynthesis and respiration, as the rate of photosynthesis decreases while the dark- and photo-respiration rates increase (Wahid et al., 2007). Extensive studies have shown that high temperature leads to a decrease in net photosynthesis (Pn) (Su and Liu, 2005). In cotton, high temperature inhibited the photosynthetic electron transport and Rubisco regeneration capacity, which simultaneously reduced chlorophyll biosynthesis (Wise et al., 2004). High temperature stress (38/28°C) for 14 days was observed to result in pigment loss and thylakoid membrane damage in soybean (Djanaguiraman et al., 2011). In this study, Si application increased the chlorophyll and carotenoid contents (Figures 5, 6). This demonstrated that Si effectively protects photosynthetic pigments. Similarly, in ryegrass (Lolium perenne L.), exogenous Si induced chlorophyll synthesis and reduced membrane injury under saline-alkali stresses. Photosynthetic apparatuses, especially PSII, water splitting, and oxygen-evolving complex (OEC) are highly susceptible to high temperature (Edwards and Walker, 1983). High temperature directly inactivates the OEC in PSII by dissociating the divalent Ca2+ and Mn2+ cations (Nash et al., 1985). This simultaneously dislodges PSII complexes from the thylakoid membrane by increasing the fluidity of thylakoid membranes (Mathur et al., 2014). Maize seedlings grown above 40°C had sharply decreased Fv/Fm and Pn was completely inhibited (Crafts-Brandner and Salvucci, 2002). Therefore, the Fv/Fm is regarded as a common tool to detect and quantify damages in photosynthesis (particularly PSII) in response to temperature stresses and has been widely used in various crops (Shangguan et al., 2000; Sinsawat et al., 2004). In this study, the Fv/Fm decreased with the duration of the high temperature stress, while from the fifth day, the Si (+) slowed down the decline of Fv/Fm (Figure 4). This is in good agreement well agreed with the report where Si application was useful to enhance photochemical efficiency in rice plants (Chen et al., 2011).
It is noteworthy that there is always a risk the high temperature effects get confounded with drought and light effects in high temperature experiments. Therefore, special attention to watering during this trial. In order to maintain normal leaf temperature, plants intensive transpiration and lose a number of water under high temperature (Sadok et al., 2021). In this study, the leaf relative water content and stomatal conductance analysis showed application Si both increased the LRWC and stomatal conductance (Figure 3), it help plants to increase the rate of photosynthesis evaporative cooling (Urban et al., 2017). Likewise, exogenous Ca or 2,4-epibrassinolide helps relieve high-temperature-induced inhibition of the stomatal conductance (Tan et al., 2011; Zhang et al., 2014).
Moreover, high temperature tolerance is also generally defined as the ability of the plant to grow and produce. High temperature reduced cell wall elongation and cell differentiation, and decreased the shoot dry weight and net assimilation rates (Potters et al., 2007). Si is involved in growth stimulation and increases plant vigor and stress resistance. In leaves, Si is deposited as amorphous silica and forms a cuticle-Si double layer, such as hemicellulose, pectin, and lignin (He et al., 2015). This cuticle-Si double layer is beneficial for cell wall synthesis and remodeling, and maintains the mesophyll cells relatively intact under stresses (Sheng and Chen, 2020). As expected, Si application increased the fresh and dry weights of strawberry shoots (Table 1). This agrees with many previous findings in grass and soybean (Eneji et al., 2008; Lee et al., 2010). It is a positive cycle for plants: the increased biomass accumulation contributed to increased photosynthesis (Frazão et al., 2020).
Analysis of Nutrients
A positive response of nutrients to Si application was observed. The K is able to induce the activation of enzymes and maintains the balance of ionic among the cells, in addition to increasing the mechanical strength of plants (Amtmann et al., 2008). The effect of Si application on K depends on the plant species (Rea et al., 2022). In lettuce, the content of K decreased with the application of Si, while Si application increased the K concentration in maize and rice (Greger et al., 2018). It is worth noting that the content of K in the root of the DSM-DSD is higher than the DSM-C, despite the Na2SiO3 being the main component of soluble Si fertilizer. It is illustrated that the Si application increased the absorption of K by the root. As expected, the application of Si increased the accumulation of P in the root of transplants, and it was reported that Si binds with Fe and Mn, and increased the availability of P (Owino and Gascho, 2005). Overall, Si is beneficial for nutrient utilization and enhances absorption by the root.
Analysis of High Temperature and Si Application on Strawberry ROS Regulation
The chemical energy expended in a mass metabolic processes is originates from photosynthesis (Ashraf and Harris, 2013). ROS is an inevitable result of electron transport chains during normal metabolic processes. In fact, the chloroplast and mitochondria are the main sites for ROS production, and stress leads to excessive ROS production and accumulation (Bhattacharjee, 2005). For example, superoxide radicals () are produced by NADPH oxidases and cell wall peroxidases, then SOD turns into H2O2 through the disproportionation reaction. Because H2O2 can freely diffuse through the biological membranes, it is cell-damaging and relatively long-living (Plaine, 1955; Møller et al., 2007). Individual aldehydic lipids break down into MDA and alter proteins, DNA, RNA, and other biomolecules (Alscher et al., 1997). Overall, ROS are reactive and can cause the peroxidation of membrane lipids and the destruction of pigments (Sewelam et al., 2016). It is noteworthy that MDA and H2O2 play important signaling roles in stress response pathways. Several studies have indicated that H2O2 interacted with other signaling molecules such as abscisic acid (ABA), calcium (Ca2+), nitric oxide, salicylic acid (SA), as well as the ethylene signaling pathways in plants (Saxena et al., 2016). On the other hand, MDA signaling does not require jasmonic acid (JA), SA, or ethylene due to MDA is able to directly induce a series of stress relative genes such as cell wall metabolism, beta-oxidation, drought stress, and lipid metabolism (Weber et al., 2004). In general, according to these signaling molecules, plants are able to maintain a dynamic equilibrium of the production and scavenging of ROS with osmoregulation, phytohormones, and antioxidant enzymes (Huang et al., 2019). ROS scavenging mechanisms can be classified as enzymatic and non-enzymatic antioxidant defense systems (Apel and Hirt, 2004). In this study, the control group had higher ROS contents and lower antioxidant enzyme activities (Figures 10–13). The enzymatic antioxidant systems increased with the Si application (Figure 8). It was reported that Si played a role not only as a mechanical barrier in the resistance process (Samuels et al., 1991). Likewise, it was frequently observed that Si application induced higher activities of antioxidant enzymes in plants such as barely, rice, cucumber, and strawberry (Liang et al., 2003; Zhu et al., 2004; Farooq et al., 2019; Xiao et al., 2022). Therefore, Si is able to regulate ROS with antioxidant proteins.
Although the regulation of ROS by Si focuses on enzymatic systems, it was reported that Si application is beneficial to osmoregulation and production of secondary metabolites subjection to abiotic stresses (Maghsoudi et al., 2019; El-Beltagi et al., 2020). Proline is an important amino acid and considered to be an osmo-protectant (Abdelaal et al., 2020). It can generate turgor, scavenge ROS, and regulate the cytosolic pH (Smirnoff and Cumbes, 1989). Anthocyanin in plants has also been proposed as an effective antioxidant (Yamasaki et al., 1996). In this study, Si application increased the proline and total anthocyanin contents (Figures 14, 15). Similar results were observed in wheat, where Si application alleviates the losses of yield caused by high temperatures through improvements in the production of proline and anthocyanin (Mustafa et al., 2021). Interestingly, the proline content in plants treated with Si (+, –) approached that of the control (Figure 13), which leads to the speculation that the effects of Si on the proline will decrease over time.
Method of Si Application Affects the Quality of Strawberry Transplants
Although the beneficial effects of Si fertilization are observed in agriculture, a common use for Si application is its infancy because many plant species are unable to benefit from Si application, and optimizing Si fertilization methods is still controversial (Tubana et al., 2016). Previous studies have shown that the transfer direction of Si in strawberry was unidirectional, there are only two ways: from the root to the shoot or from the mother plants to the daughter plants (Li et al., 2020). The results of this study show that the Si contents in the roots did not show differences between the control and after the foliar spray in “Sulhyang” (Figure 8). Interestingly, although strawberry plants absorbed more Si through root drench than with foliar spray, the Si content in the roots of foliar-sprayed plants was higher than in the control in “Maehyang” and “Kuemsil”. It has been shown that Si must be transported by specific Lsi6 transporters in shoots. Furthermore, some tissues with low transpiration can accumulate high levels of Si despite the fact that Si transport in the xylem is driven by transpiration (Yang et al., 2018). Therefore, it is not excluded that Si is redistributed from the leaf to the shoot and root before deposition. Far more research is needed to understand whether or not there are any transport mechanisms after foliar Si spray. Moreover, organosilicon is a nonionic surfactant and has been found to enhance stomatal infiltration (Fernández and Eichert, 2009). In this study, the stomatal conductance in SD-SD was higher than in DM-DD (Figure 3) in “Maehyang” and “Kuemsil”. Furthermore, compared with the substrate Si drench, foliar spray increased the plant resistance to wilting; this was not replicated when foliar Si spray was combined with substrate Si drench (Tebow et al., 2021). Trials on a variety of crops have led to the “Silicic Acid Agro Technology (SAAT)”, which indicates that foliar Si sprays are only effective when they start early in the vegetative stage and follow a specific spray schedule (Laane, 2017). Moreover, the amount of Si in the edible parts of higher plants varies widely. For fruits and vegetables, the trichomes or ultrafine hairs of fruits are the potential location for Si depositions (Powell et al., 2005). However, the Si hardly accumulates in edible parts of strawberries and the Si rather enhanced the vegetative growth and fruit quality by stimulating growth and modifying the phenolic metabolism (Hajiboland et al., 2018). It was reported that the Si concentration of the fruit never reached the level of detection and was undetectable after subjection to Si treatment for 5 months in six strawberry cultivars (Ouellette et al., 2017). Furthermore, Si increased the shelf-life of berries (Peris et al., 2020). Therefore, the absorption and efficiency of Si are affected by the species, plant age, application method, and frequency.
Conclusion
The significant effects of Si application on plants have been extensively investigated, but researchers have rarely studied the optimal method for better growth, development, and resistance to high temperature during strawberry cutting propagation. Our results suggest that Si increased the plant growth during the cutting propagation. DM-DD (the mother plants drenched with the Si solution before cutting propagation and the daughter plants drenched with the Si solution after cutting propagation) and SD-SD (the daughter plants sprayed with the Si solution before and after cutting propagation) were especially effective, compared to applying Si only before or after the cutting propagation. SD-SD (the daughter plants sprayed with the Si solution before and after cutting propagation) was the best for enhancing the resistance to high temperature stresses in strawberry transplants because photosynthesis and stomatal conductance were less affected by the high temperature, and the strawberry transplants were able to accumulate more sugars, proteins, and enzymatic and non-enzymatic antioxidants.
Data Availability Statement
The original contributions presented in the study are included in the article/supplementary material, further inquiries can be directed to the corresponding author/s.
Author Contributions
JX, YL, and BRJ conceived and designed this study. JX performed the experiments, analyzed the data, and drafted the manuscript. JX and BRJ edited and finalized the manuscript. All authors read and approved the submitted version.
Funding
JX and YL were supported by the BK21 Four Program, Ministry of Education, Republic of Korea.
Conflict of Interest
The authors declare that the research was conducted in the absence of any commercial or financial relationships that could be construed as a potential conflict of interest.
Publisher's Note
All claims expressed in this article are solely those of the authors and do not necessarily represent those of their affiliated organizations, or those of the publisher, the editors and the reviewers. Any product that may be evaluated in this article, or claim that may be made by its manufacturer, is not guaranteed or endorsed by the publisher.
References
Abdelaal, K. A., Attia, K. A., Alamery, S. F., ElAfry, M. M., Ghazy, A. I., and Tantawy, D. S. (2020). Exogenous application of proline and salicylic acid can mitigate the injurious impacts of drought stress on barley plants associated with physiological and histological characters. Sustainability. 12, 1736. doi: 10.3390/su12051736
Agarie, S., Hanaoka, N., Ueno, O., Miyazaki, A., Kubota, F., and Agata, W. (1998). Effects of silicon on tolerance to water deficit and heat stress in rice plants (Oryza sativa L.), monitored by electrolyte leakage. Plant. Prod. Sci. 1, 96–103. doi: 10.1626/pps.1.96
Alscher, R. G., Donahue, J. L., and Cramer, C. L. (1997). Reactive oxygen species and antioxidants: relationships in green cells. Physiol. Plant. 100, 224–233. doi: 10.1111/j.1399-3054.1997.tb04778.x
Amtmann, A., Troufflard, S., and Armengaud, P. (2008). The effect of potassium nutrition on pest and disease resistance in plants. Physiol. Plant. 133, 682–691. doi: 10.1111/j.1399-3054.2008.01075.x
Apel, K., and Hirt, H. (2004). Reactive oxygen species: Metabolism, oxidative stress, and signal transduction. Annu. Rev. Plant Biol. 55, 373–399. doi: 10.1146/annurev.arplant.55.031903.141701
Ashraf, M., and Harris, P. J. (2013). Photosynthesis under stressful environments: An overview. Photosynthetica. 51, 163–190. doi: 10.1007/s11099-013-0021-6
Bates, L. S., Waldren, R. P., and Teare, I. (1973). Rapid determination of free proline for water-stress studies. Plant Soil. 39205–207. doi: 10.1007/BF00018060
Bhattacharjee, S. (2005). Reactive oxygen species and oxidative burst: Roles in stress, senescence and signal transducation in plants. Curr. Sci. 11, 13–1121.
Caruana, J. C., Sittmann, J. W., Wang, W., and Liu, Z. (2018). Suppressor of runnerless encodes a DELLA protein that controls runner formation for asexual reproduction in strawberry. Mol. Plant. 11, 230–233. doi: 10.1016/j.molp.2017.11.001
Chen, W., Yao, X., Cai, K., and Chen, J. (2011). Silicon alleviates drought stress of rice plants by improving plant water status, photosynthesis and mineral nutrient absorption. Biol. Trace Elem. Res. 142, 67–76. doi: 10.1007/s12011-010-8742-x
Crafts-Brandner, S. J., and Salvucci, M. E. (2002). Sensitivity of photosynthesis in a C4 plant, maize, to heat stress. Plant Physiol. 129, 1773–1780. doi: 10.1104/pp.002170
Dallagnol, L. J., Ramos, A. E. R., and da Rosa Dorneles, K. (2020). Silicon use in the integrated disease management of wheat: Current knowledge. Curr. Trends Wheat Res. 10, 95285.
Djanaguiraman, M., Prasad, P., and Al-Khatib, K. (2011). Ethylene perception inhibitor 1-MCP decreases oxidative damage of leaves through enhanced antioxidant defense mechanisms in soybean plants grown under high temperature stress. Environ. Exp. Bot. 71, 215–223. doi: 10.1016/j.envexpbot.2010.12.006
Edwards, G., and Walker, D. (1983). “The reduction pentose phosphate pathway and associated reaction,” in C3, C4. Mechanisms, Cellular and Environmental Regulation of Photosynthesis, eds G. Edwards, and D. Walker (Berkeley: University of California Press), 107–156.
El-Beltagi, H. S., Sofy, M. R., Aldaej, M. I., and Mohamed, H. I. (2020). Silicon alleviates copper toxicity in flax plants by up-regulating antioxidant defense and secondary metabolites and decreasing oxidative damage. Sustainability. 12, 4732. doi: 10.3390/su12114732
Eneji, A. E., Inanaga, S., Muranaka, S., Li, J., Hattori, T., and An, P. (2008). Growth and nutrient use in four grasses under drought stress as mediated by silicon fertilizers. J. Plant Nutr. 31, 355–365. doi: 10.1080/01904160801894913
Epstein, E. (1994). The anomaly of silicon in plant biology. Proc. Natl. Acad. Sci. 91, 11–17. doi: 10.1073/pnas.91.1.11
Etesami, H., and Jeong, B. R. (2018). Silicon (Si): Review and future prospects on the action mechanisms in alleviating biotic and abiotic stresses in plants. Ecotoxicol. Environ. Saf. 147, 881–896. doi: 10.1016/j.ecoenv.2017.09.063
Farooq, M. A., Saqib, Z. A., Akhtar, J., Bakhat, H. F., Pasala, R.-K., et al. (2019). Protective role of silicon (Si) against combined stress of salinity and boron (B) toxicity by improving antioxidant enzymes activity in rice. Silicon. 11, 2193–2197. doi: 10.1007/s12633-015-9346-z
Fernández, V., and Eichert, T. (2009). Uptake of hydrophilic solutes through plant leaves: current state of knowledge and perspectives of foliar fertilization. Crit. Rev. Plant Sci. 28, 36–68. doi: 10.1080/07352680902743069
Frazão, J. J., de Mello Prado, R., de Souza Júnior, J. P., and Rossatto, D. R. (2020). Silicon changes C: N: P stoichiometry of sugarcane and its consequences for photosynthesis, biomass partitioning and plant growth. Sci. Rep. 10, 1–10. doi: 10.1038/s41598-020-69310-6
González, L., and González-Vilar, M. (2001). “Determination of relative water content,” in Handbook of Plant Ecophysiology Techniques, ed M. J. R. Roger (New York: Springer), 207–212. doi: 10.1007/0-306-48057-3_14
Greger, M., Landberg, T., and Vaculík, M. (2018). Silicon influences soil availability and accumulation of mineral nutrients in various plant species. Plants. 7, 41. doi: 10.3390/plants7020041
Hajiboland, R., Moradtalab, N., Eshaghi, Z., and Feizy, J. (2018). Effect of silicon supplementation on growth and metabolism of strawberry plants at three developmental stages. N. Z. J. Crop Hortic. Sci. 46, 144–161. doi: 10.1080/01140671.2017.1373680
He, C., Ma, J., and Wang, L. (2015). A hemicellulose-bound form of silicon with potential to improve the mechanical properties and regeneration of the cell wall of rice. New Phytol. 206, 1051–1062. doi: 10.1111/nph.13282
Howladar, S. M., Al-Robai, S. A., Alzahrani, F. S., Howladar, M. M., and Aldhebiani, A. Y. (2018). Silicon and its application method effects on modulation of cadmium stress responses in Triticum aestivum (L.) through improving the antioxidative defense system and polyamine gene expression. Ecotoxicol. Environ. Saf. 159, 143–152. doi: 10.1016/j.ecoenv.2018.05.004
Hu, J., Li, Y., and Jeong, B. R. (2020). Silicon alleviates temperature stresses in poinsettia by regulating stomata, photosynthesis, and oxidative damages. Agronomy. 10, 1419. doi: 10.3390/agronomy10091419
Huang, H., Ullah, F., Zhou, D. X., Yi, M., and Zhao, Y. (2019). Mechanisms of ROS regulation of plant development and stress responses. Front. Plant Sci. 10, 800. doi: 10.3389/fpls.2019.00800
IPCC (2014). “Climate Change 2014: Synthesis Report,” in Proceedings of the Contribution of Working Groups I, II and III to the Fifth Assessment Report of the Intergovernmental Panel on Climate Change, eds Core Writing Team, R. K. Pachauri, and L. A. Meyer (Geneva: IPCC), 151.
Jun, H., Jung, H., and Imai, K. (2017). Gas exchange characteristics of a leading cultivar of Korean strawberry (Fragaria × ananassa,”Sulhyang”). Sci. Hortic. 221, 10–15. doi: 10.1016/j.scienta.2017.04.009
Junglee, S., Urban, L., Sallanon, H., and Lopez-Lauri, F. (2014). Optimized assay for hydrogen peroxide determination in plant tissue using potassium iodide. Am. J. Anal. Chem. 5, 730. doi: 10.4236/ajac.2014.511081
Kadir, S., Sidhu, G., and Al-Khatib, K. (2006). Strawberry (Fragaria × ananassa Duch.) growth and productivity as affected by temperature. HortScience. 41, 1423–1430. doi: 10.21273/HORTSCI.41.6.1423
Katz, O., Puppe, D., Kaczorek, D., Prakash, N. B., and Schaller, J. (2021). Silicon in the soil-plant continuum: intricate feedback mechanisms within ecosystems. Plants 10, 652. doi: 10.3390/plants10040652
Kim, D. E., Kwon, J. K., Hong, S. J., Lee, J. W., and Woo, Y. H. (2020). The effect of greenhouse climate change by temporary shading at summer on photo respiration, leaf temperature and growth of cucumber. Protected Hortic. Plant Fact. 29, 306–312. doi: 10.12791/KSBEC.2020.29.3.306
Kim, S. K., Bae, R., Na, H., Song, J. H., Kang, H. J., and Chun, C. (2012). Changes in fruit physicochemical characteristics by fruit clusters in June-bearing strawberry cultivars. Kor. J. Sci. Technol. 30, 378–384. doi: 10.7235/hort.2012.12027
Kim, T. I., Jang, W. S., Choi, J. H., Nam, M. H., Kim, W. S., and Lee, S. S. (2004). Breeding of strawberry ‘Maehyang' for forcing culture. Horticul. Sci. Technol. 22, 434–437.
Kowalska, J., Tyburski, J., Jakubowska, M., and Krzymińska, J. (2021). Effect of different forms of silicon on growth of spring wheat cultivated in organic farming system. Silicon. 13, 211–217. doi: 10.1007/s12633-020-00414-4
Laane, H. (2017). The effects of the application of foliar sprays with stabilized silicic acid: an overview of the results from 2003-2014. Silicon 9, 803–807. doi: 10.1007/s12633-016-9466-0
Lee, S., Sohn, E., Hamayun, M., Yoon, J., and Lee, I. (2010). Effect of silicon on growth and salinity stress of soybean plant grown under hydroponic system. Agrofor. Syst. 80, 333–340. doi: 10.1007/s10457-010-9299-6
Li, Y., Xiao, J., Hu, J., and Jeong, B. R. (2020). Method of silicon application affects quality of strawberry daughter plants during cutting propagation in hydroponic substrate system. Agronomy. 10, 1753. doi: 10.3390/agronomy10111753
Liang, Y., Chen, Q., Liu, Q., Zhang, W., and Ding, R. (2003). Exogenous silicon (Si) increases antioxidant enzyme activity and reduces lipid peroxidation in roots of salt-stressed barley (Hordeum vulgare L.). J. Plant Physiol. 160, 1157–1164. doi: 10.1078/0176-1617-01065
Ma, J. F. (2003). “Functions of silicon in higher plants,” in Silicon biomineralization, ed. E. G. Werner (New York: Springer), 127–147.
Ma, J. F., Miyake, Y., and Takahashi, E. (2001). Silicon as a beneficial element for crop plants. Stud. Plant Sci. 8, 17–39. doi: 10.1016/S0928-3420(01)80006-9
Ma, J. F., and Takahashi, E. (2002). “Silicon uptake and accumalation in plants,” in Soil, Fertilizer, and Plant Silicon Research in Japan, eds. J. F. Ma, and E. Takahashi (Amsterdam: Elsevier), 73–100.
Ma, J. F., Tamai, K., Yamaji, N., Mitani, N., Konishi, S., and Katsuhara, M. (2006). A silicon transporter in rice. Nature. 440, 688–691. doi: 10.1038/nature04590
Maghsoudi, K., Emam, Y., Ashraf, M., Pessarakli, M., and Arvin, M. J. (2019). Silicon application positively alters pollen grain area, osmoregulation and antioxidant enzyme activities in wheat plants under water deficit conditions. J. Plant Nutr. 42, 2121–2132. doi: 10.1080/01904167.2019.1648677
Mathur, S., Agrawal, D., and Jajoo, A. (2014). Photosynthesis: response to high temperature stress. J. Photochem. Photobiol. B. 137, 116–126. doi: 10.1016/j.jphotobiol.2014.01.010
Mitani, N., and Ma, J. F. (2005). Uptake system of silicon in different plant species. J. Exp. Bot. 56, 1255–1261. doi: 10.1093/jxb/eri121
Møller, I. M., Jensen, P. E., and Hansson, A. (2007). Oxidative modifications to cellular components in plants. Annu. Rev. Plant Biol. 58, 459–481. doi: 10.1146/annurev.arplant.58.032806.103946
Moradtalab, N., Hajiboland, R., Aliasgharzad, N., Hartmann, T. E., and Neumann, G. (2019). Silicon and the association with an arbuscular-mycorrhizal fungus (Rhizophagus clarus) mitigate the adverse effects of drought stress on strawberry. Agronomy. 9, 41. doi: 10.3390/agronomy9010041
Muneer, S., Park, Y. G., Kim, S., and Jeong, B. R. (2017). Foliar or subirrigation silicon supply mitigates high temperature stress in strawberry by maintaining photosynthetic and stress-responsive proteins. J. Plant Growth Regul. 36, 836–845. doi: 10.1007/s00344-017-9687-5
Mustafa, T., Sattar, A., Sher, A., Ul-Allah, S., Ijaz, M., and Irfan, M. (2021). Exogenous application of silicon improves the performance of wheat under terminal heat stress by triggering physio-biochemical mechanisms. Sci. Rep. 1, 11–12. doi: 10.1038/s41598-021-02594-4
Nash, D., Miyao, M., and Murata, N. (1985). Heat inactivation of oxygen evolution in photosystem II particles and its acceleration by chloride depletion and exogenous manganese. Biochim. Biophys. Acta. Bioenerg. 807, 127–133. doi: 10.1016/0005-2728(85)90115-X
Nasircilar, A. P. D. A. G. (2021). “The role of silicone applications in tolerance to abiotic stress conditions,” in Highly Interconnected and Endless Puzzle: Agriculture, ed A. B. Özçinar (Ankara: Iksad), 23–44.
Ouellette, S., Goyette, M. H., Labb,é, C., Laur, J., Gaudreau, L., and Gosselin, A. (2017). Silicon transporters and effects of silicon amendments in strawberry under high tunnel and field conditions. Front. Plant Sci. 8, 949. doi: 10.3389/fpls.2017.00949
Owino, G. C., and Gascho, G. J. (2005). Effect of silicon on low pH soil phosphorus sorption and on uptake and growth of maize. Commun. Soil Sci. Plant Anal. 35, 2369–2378. doi: 10.1081/LCSS-200030686
Park, Y. G., Muneer, S., Kim, S., Hwang, S. J., and Jeong, B. R. (2018). Silicon application during vegetative propagation affects photosynthetic protein expression in strawberry. Hortic. Environ. Biotechnol. 59, 167–177. doi: 10.1007/s13580-018-0022-2
Pavlovic, J., Kostic, L., Bosnic, P., Kirkby, E. A., and Nikolic, M. (2021). Interactions of silicon with essential and beneficial elements in plants. Front. Plant Sci. 12, 1224. doi: 10.3389/fpls.2021.697592
Peris, F. J., Benavent-Gil, Y., and Hernández-Apaolaza, L. (2020). Silicon beneficial effects on yield, fruit quality and shelf-life of strawberries grown in different culture substrates under different iron status. Plant Physiol. Biochem. 152, 23–31. doi: 10.1016/j.plaphy.2020.04.026
Pirie, A., and Mullins, M. G. (1976). Changes in anthocyanin and phenolics content of grapevine leaf and fruit tissues treated with sucrose, nitrate, and abscisic acid. Plant Physiol. 58468–472. doi: 10.1104/pp.58.4.468
Plaine, H. L. (1955). The effect of oxygen and of hydrogen peroxide on the action of a specific gene and on tumor induction in Drosophila melanogaster. Genetics. 40, 268. doi: 10.1093/genetics/40.2.268
Potters, G., Pasternak, T. P., Guisez, Y., Palme, K. J., and Jansen, M. A. (2007). Stress-induced morphogenic responses: growing out of trouble? Trends Plant Sci. 12, 98–105. doi: 10.1016/j.tplants.2007.01.004
Powell, J. J., McNaughton, S. A., Jugdaohsingh, R., Anderson, S. H. C., Dear, J., Khot, F., et al. (2005). A provisional database for the silicon content of foods in the United Kingdom. Br. J. Nutr. 94, 804–812. doi: 10.1079/BJN20051542
Puppe, D., Kaczorek, D., Schaller, J., Barkusky, D., and Sommer, M. (2021). Crop straw recycling prevents anthropogenic desilication of agricultural soil-plant systems in the temperate zone–results from a long-term field experiment in NE Germany. Geoderma. 403, 115187. doi: 10.1016/j.geoderma.2021.115187
Rea, R. S., Islam, M. R., Rahman, M. M., Nath, B., and Mix, K. (2022). Growth, nutrient accumulation, and drought tolerance in crop plants with silicon application: a review. Sustainability. 14, 4525. doi: 10.3390/su14084525
Sadok, W., Lopez, J. R., and Smith, K. P. (2021). Transpiration increase under high-temperature stress: Potential mechanisms, trade-offs and prospects for crop resilience in a warming world. Plant Cell Environ. 44, 2102–2116. doi: 10.1111/pce.13970
Samuels, A., Glass, A., Ehret, D., and Menzies, J. (1991). Distribution of silicon in cucumber leaves during infection by powdery mildew fungus (Sphaerotheca fuliginea). Can. J. Bot. 69, 140–146. doi: 10.1139/b91-020
Sattar, A., Cheema, M. A., Sher, A., Ijaz, M., Wasaya, A., and Yasir, T. A. (2020). Foliar applied silicon improves water relations, stay green and enzymatic antioxidants activity in late sown wheat. Silicon. 12, 223–230. doi: 10.1007/s12633-019-00115-7
Saxena, I., Srikanth, S., and Chen, Z. (2016). Cross talk between H2O2 and interacting signal molecules under plant stress response. Front. Plant Sci. 7, 570. doi: 10.3389/fpls.2016.00570
Sewelam, N., Kazan, K., and Schenk, P. M. (2016). Global plant stress signaling: reactive oxygen species at the cross-road. Front. Plant Sci. 7, 187. doi: 10.3389/fpls.2016.00187
Shangguan, Z., Shao, M., and Dyckmans, J. (2000). Effects of nitrogen nutrition and water deficit on net photosynthetic rate and chlorophyll fluorescence in winter wheat. J. Plant Physiol. 156, 46–51. doi: 10.1016/S0176-1617(00)80271-0
Sheng, H., and Chen, S. (2020). Plant silicon-cell wall complexes: Identification, model of covalent bond formation and biofunction. Plant Physiol. Biochem. 155, 13–19. doi: 10.1016/j.plaphy.2020.07.020
Sinsawat, V., Leipner, J., Stamp, P., and Fracheboud, Y. (2004). Effect of heat stress on the photosynthetic apparatus in maize (Zea mays L.) grown at control or high temperature. Environ. Exp. Bot. 52, 123–129. doi: 10.1016/j.envexpbot.2004.01.010
Sivanesan, I., Son, M. S., Soundararajan, P., and Jeong, B. R. (2013). Growth of chrysanthemum cultivars as affected by silicon source and application method. Horticul. Sci. Technol. 31, 544–551. doi: 10.7235/hort.2013.13046
Smirnoff, N., and Cumbes, Q. J. (1989). Hydroxyl radical scavenging activity of compatible solutes. Phytochemistry. 28, 1057–1060. doi: 10.1016/0031-9422(89)80182-7
Song, J., Li, Y., Hu, J., Lee, J., and Jeong, B. R. (2021). Pre-and/or postharvest silicon application prolongs the vase life and enhances the quality of cut peony (Paeonia lactiflora Pall.) flowers. Plants. 10, 1742. doi: 10.3390/plants10081742
Soundararajan, P., Manivannan, A., Ko, C. H., Park, J. E., and Jeong, B. R. (2019). Evaluation of relative toxicity caused by deicing agents on photosynthesis, redox homeostasis, and the osmoregulatory system in creeper-type plants. Hortic. Environ. Biotechnol. 60, 175–186. doi: 10.1007/s13580-018-0117-9
Soundararajan, P., Sivanesan, I., Jana, S., and Jeong, B. R. (2014). Influence of silicon supplementation on the growth and tolerance to high temperature in Salvia splendens. Hortic. Environ. Biotechnol. 55271–279. doi: 10.1007/s13580-014-0023-8
Su, P., and Liu, X. (2005). Photosynthetic characteristics of linze jujube in conditions of high temperature and irradiation. Sci. Hortic.104, 339–350. doi: 10.1016/j.scienta.2004.08.012
Sükran, D., GÜNES, T., and Sivaci, R. (1998). Spectrophotometric determination of chlorophyll-a, b and total carotenoid contents of some algae species using different solvents. Turk. J. Bot. 2213–18.
Sun, C., Li, X., Hu, Y., Zhao, P., Xu, T., and Sun, J. (2015). Proline, sugars, and antioxidant enzymes respond to drought stress in the leaves of strawberry plants. Hortic. Sci. Biotechnol. 33625–632. doi: 10.7235/hort.2015.15054
Takahashi, E., Ma, J., and Miyake, Y. (1990). The possibility of silicon as an essential element for higher plants. Comments Agric. Food Chem. 2, 99–122.
Takeda, F., Hokanson, S. C., and Enns, J. M. (2004). Influence of daughter plant weight and position on strawberry transplant production and field performance in annual plasticulture. HortSci. 39, 1592–1595. doi: 10.21273/HORTSCI.39.7.1592
Tamai, K., and Ma, J. F. (2003). Characterization of silicon uptake by rice roots. New Phytol. 158, 431–436. doi: 10.1046/j.1469-8137.2003.00773.x
Tan, W., wei Meng, Q., Brestic, M., Olsovska, K., and Yang, X. (2011). Photosynthesis is improved by exogenous calcium in heat-stressed tobacco plants. J. Plant Physiol. 168, 2063–2071. doi: 10.1016/j.jplph.2011.06.009
Tebow, J. B., Houston, L. L., and Dickson, R. W. (2021). Silicon foliar spray and substrate drench effects on plant growth, morphology, and resistance to wilting with container-grown edible species. Horticulturae. 7, 263. doi: 10.3390/horticulturae7090263
Tubana, B. S., Babu, T., and Datnoff, L. E. (2016). A review of silicon in soils and plants and its role in US agriculture: history and future perspectives. Soil Sci. 181, 393–411. doi: 10.1097/SS.0000000000000179
Urban, J., Ingwers, M., McGuire, M. A., and Teskey, R. O. (2017). Stomatal conductance increases with rising temperature. Plant Signaling Behav. 12, e1356534. doi: 10.1080/15592324.2017.1356534
Vu, N. T., Tran, A. T., Le, T. T. C., Na, J. K., Kim, S. H., Park, J. M., et al. (2017). Improvement of tomato seedling quality under low temperature by application of silicate fertilizer. J. Bio-Environ. Control. 26, 158–166. doi: 10.12791/KSBEC.2017.26.3.158
Wahid, A., Farooq, M., Hussain, I., Rasheed, R., and Galani, S. (2012). “Responses and management of heat stress in plants,” in Environmental Adaptations and Stress Tolerance of Plants in The Era of Climate Change, ed A. Parvaiz (New York: Springer), 135–157. doi: 10.1007/978-1-4614-0815-4_6
Wahid, A., Gelani, S., Ashraf, M., and Foolad, M. R. (2007). Heat tolerance in plants: an overview. Environ. Exp. Bot. 61199–223. doi: 10.1016/j.envexpbot.2007.05.011
Wang, Y., Hu, Y., Duan, Y., Feng, R., and Gong, H. (2016). Silicon reduces long-term cadmium toxicities in potted garlic plants. Acta Physiol. Plant. 381–9. doi: 10.1007/s11738-016-2231-6
Weber, H., Chételat, A., Reymond, P., and Farmer, E. E. (2004). Selective and powerful stress gene expression in Arabidopsis in response to malondialdehyde. Plant J. 37, 877–888. doi: 10.1111/j.1365-313X.2003.02013.x
Wise, R., Olson, A., Schrader, S., and Sharkey, T. (2004). Electron transport is the functional limitation of photosynthesis in field-grown Pima cotton plants at high temperature. Plant Cell Environ. 27, 717–724. doi: 10.1111/j.1365-3040.2004.01171.x
Xiao, J., Li, Y., and Jeong, B. R. (2022). Foliar silicon spray before summer cutting propagation enhances resistance to powdery mildew of daughter plants. Int. J. Mol. Sci. 23, 3803. doi: 10.3390/ijms23073803
Yamasaki, H., Uefuji, H., and Sakihama, Y. (1996). Bleaching of the red anthocyanin induced by superoxide radical. Arch. Biochem. Biophys. 332, 183–186. doi: 10.1006/abbi.1996.0331
Yan, C., Nikolic, M., Ye, M., Xiao, Z., and Liang, Y. (2018). Silicon acquisition and accumulation in plant and its significance for agriculture. J. Integr. Agric. 17, 2138–2150. doi: 10.1016/S2095-3119(18)62037-4
Yoon, H. S., Jin, H. J., and Oh, J. Y. (2020). “Kuemsil”, a strawberry variety suitable for forcing culture. Kor. Soc. Breed. Sci. 52, 184–189. (In Korean) doi: 10.9787/KJBS.2020.52.2.184
Zhang, H., and Dotson, P. (1994). The use of microwave muffle furnace for dry ashing plant tissue samples. Commun. Soil Sci. Plant Anal. 25, 1321–1327. doi: 10.1080/00103629409369118
Zhang, Y., He, J., Yang, S., and Chen, Y. (2014). Exogenous 24-epibrassinolide ameliorates high temperature-induced inhibition of growth and photosynthesis in Cucumis melo. Biol. Plant. 58, 311–318. doi: 10.1007/s10535-014-0395-8
Keywords: abiotic stress, anthocyanin, antioxidant enzyme, chlorophyll fluorescence, photosynthesis pigment, reactive oxygen species
Citation: Xiao J, Li Y and Jeong BR (2022) Foliar Silicon Spray to Strawberry Plants During Summer Cutting Propagation Enhances Resistance of Transplants to High Temperature Stresses. Front. Sustain. Food Syst. 6:938128. doi: 10.3389/fsufs.2022.938128
Received: 07 May 2022; Accepted: 02 June 2022;
Published: 27 June 2022.
Edited by:
Joerg Schaller, Leibniz Center for Agricultural Landscape Research (ZALF), GermanyReviewed by:
Lyudmila Petrova Simova-Stoilova, Institute of Plant Physiology and Genetics (BAS), BulgariaOu Sheng, Guangdong Academy of Agricultural Sciences (GDAAS), China
Copyright © 2022 Xiao, Li and Jeong. This is an open-access article distributed under the terms of the Creative Commons Attribution License (CC BY). The use, distribution or reproduction in other forums is permitted, provided the original author(s) and the copyright owner(s) are credited and that the original publication in this journal is cited, in accordance with accepted academic practice. No use, distribution or reproduction is permitted which does not comply with these terms.
*Correspondence: Byoung Ryong Jeong, YnJqZW9uZ0BnbnUuYWMua3I=