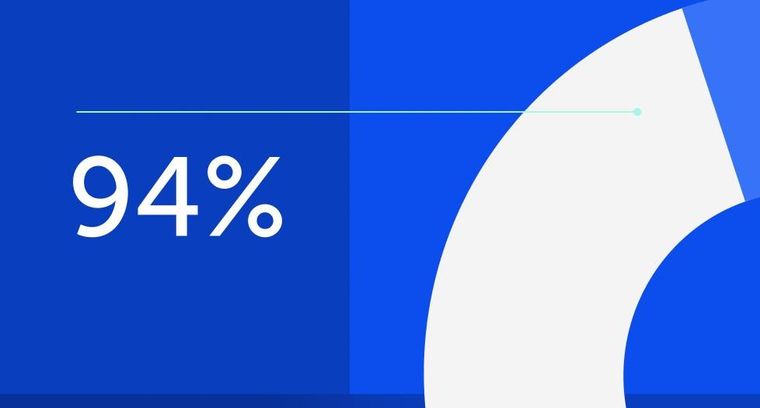
94% of researchers rate our articles as excellent or good
Learn more about the work of our research integrity team to safeguard the quality of each article we publish.
Find out more
REVIEW article
Front. Sustain. Food Syst., 15 September 2022
Sec. Agroecology and Ecosystem Services
Volume 6 - 2022 | https://doi.org/10.3389/fsufs.2022.921216
This article is part of the Research TopicEcological Nutrient Management as a Pathway to Zero HungerView all 13 articles
Soil degradation is widespread in smallholder agrarian communities across the globe where limited resource farmers struggle to overcome poverty and malnutrition. This review lays out the scientific basis and practical management options for an ecologically based approach to sustainably managing soil fertility, with particular attention to smallholder subsistence systems. We seek to change the trajectory of development programs that continue to promote inorganic fertilizers and other high input strategies to resource constrained smallholders, despite ample evidence that this approach is falling short of food security goals and contributing to resource degradation. Ecological nutrient management (ENM) is an agroecological approach to managing the biogeochemical cycles that govern soil ecosystem services and soil fertility. The portfolio of ENM strategies extends beyond reliance on inorganic fertilizers and is guided by the following five principles: (1) Build soil organic matter and other nutrient reserves. (2) Minimize the size of N and P pools that are the most susceptible to loss. (3) Maximize agroecosystem capacity to use soluble, inorganic N and P. (4) Use functional and phylogenetic biodiversity to minimize bare fallows and maximize presence of growing plants. (5) Construct agroecosystem and field scale mass balances to track net nutrient flows over multiple growing seasons. Strategic increases in spatial and temporal plant species diversity is a core ENM tactic that expands agroecosystem multifunctionality to meet smallholder priorities beyond soil restoration and crop yields. Examples of ENM practices include the use of functionally designed polycultures, diversified rotations, reduced fallow periods, increased reliance on legumes, integrated crop-livestock production, and use of variety of soil amendments. These practices foster soil organic matter accrual and restoration of soil function, both of which underpin agroecosystem resilience. When ENM is first implemented, short-term yield outcomes are variable; however, over the long-term, management systems that employ ENM can increase yields, yield stability, profitability and food security. ENM rests on a solid foundation of ecosystem and biogeochemical science, and despite the many barriers imposed by current agricultural policies, successful ENM systems are being promoted by some development actors and used by smallholder farmers, with promising results.
Smallholder subsistence farming systems provide food to almost half the global human population. Limited access to resources makes it challenging for farmers to replenish soil nutrient reserves and compensate for harvested removals, leading to soil organic matter1 (hereafter, SOM) depletion and soil erosion (Tittonell and Giller, 2013; Tully et al., 2015; Barbier and Hochard, 2018). Time lags exacerbate the downward trend in soil fertility because management is oriented toward annual food production, whereas soil degradation accrues over decadal timescales. As soil fertility and yields decline, smallholders often respond by intensifying cropping systems in ways that undermine soil fertility and food security, creating a downward spiral of malnutrition and poverty (Vanek et al., 2016; Barbier and Hochard, 2018).
Ecological nutrient management (hereafter, ENM) is a comprehensive, ecologically based approach to sustaining soil fertility (Drinkwater and Snapp, 2007a; Drinkwater et al., 2017). Ecological nutrient management aims to restore and maintain SOM/nutrient reservoirs, achieve acceptable yields, balance nutrient additions with exports, and minimize nutrient/soil losses. Our initial paper introduced ENM and focused on how this approach could reduce environmental nutrient losses in high-input, industrial farming systems (Drinkwater and Snapp, 2007a). Here, we shift our emphasis to smallholder farming systems where nutrient mining and degraded soils are pervasive. We first present the guiding principles of ENM and highlight features which distinguish ENM from conventional nutrient management. We then review the current understanding of SOM pools and their functions incorporating new understanding of the mechanisms regulating SOM dynamics and discuss the roles of plants and decomposers in governing elemental cycling processes. Then we discuss how ENM creates positive feedbacks that increase agroecosystem adaptive capacity and resilience and discuss core management strategies using three case studies of ENM in smallholder systems in Sub-Saharan Africa. Lastly, we briefly examine the obstacles that privilege the use of synthetic fertilizers while discouraging implementation of ENM strategies and present ideas about how these barriers can be overcome.
Conventional nutrient management aims to maximize inorganic fertilizer (Fi) use efficiency by reducing temporal asynchrony and spatial separation between Fi applications and crop uptake (Table 1; Cassman et al., 2002). To achieve this goal, a collection of best management practices designated as “4Rs Nutrient Management” (4R-NM) is widely promoted. The 4R-NM system seeks to maximize crop uptake of Fi using “the Right Fi source, at the Right rate, at the Right time, with the Right placement” (Vollmer-Sanders et al., 2016; Fixen, 2020). Compared to earlier strategies that often focused on one aspect of Fi management, 4R-NM is more comprehensive; however, the focus is still on a single growing season, and 4R-NM does not attempt to manage SOM reserves or the long-term trajectory of SOM levels. Thus, crop yield improvements can be rapidly apparent under 4R-NM due to high doses of Fi or “rescue” Fi applications, while the slower process of SOM decline continue (Ladha et al., 2011). Over the long-term, simplified rotations of crops bred to be highly responsive to Fi combined with SOM declines and diminished nutrient recycling act together to reinforce Fi dependency. This creates what has been termed a fertilizer treadmill (Drinkwater and Snapp, 2007a,b; Houser and Stuart, 2020).
ENM extends an ecological conceptual framework to agricultural management (Table 1). The nitrogen (N) saturation hypothesis was developed to explain changes in forest ecosystem biogeochemistry resulting from chronic anthropogenic N deposition and provides the theoretical foundation for ENM (Agren and Bosatta, 1988; Aber et al., 1989). According to this hypothesis, ecosystems are N saturated when primary productivity is no longer limited by N, and N additions exceed the capacity of the ecosystem to cycle or store N internally. Nitrogen flows are governed largely biotic processes, so retention of N depends on plant and microbial assimilation while microbial N transformations (nitrification/denitrification) drive N losses from ecosystems. More recently, the N saturation conceptual framework has been extended to P (Deng et al., 2017; Chen et al., 2021) with some modifications due to differences in N and P cycles. While N sinks and transformations are primarily controlled by plant- and microbially-mediated processes, P cycling processes include several abiotic mechanisms, including precipitation-dissolution, sorption-desorption, and occlusion. These geochemically mediated sinks compete with biological assimilation for the small amounts of soluble, inorganic P (hereafter Pi) which are typically present in the soil solution (Attiwill and Adams, 1993). Under P saturation, excess soluble P can be captured by geochemical processes (“fixed”) essentially removing P from the biological P cycle.
There are two key aspects of this ecosystem-based framework that inform ENM. First is the recognition that time lags and cycling processes occurring across spatial/temporal scales influence ecosystem-scale accrual or loss of carbon (C), N and phosphorus (P). Second, the framework highlights the importance of interactions among elemental cycles and clarifies the role of C-N-P coupling in determining whether conservation or loss pathways dominate.
Thus, the overarching goal of ENM is to manage agroecosystems to reach dynamic steady states where SOM formation equals decomposition, nutrient additions equal harvested exports, and yields and SOM/nutrient reserves are maintained. The scope of ENM extends beyond promoting fast, single season nutrient supply to consider all processes governing biogeochemical cycling across spatial and temporal scales (Figure 1). ENM aims to recouple elemental cycling processes at multiple temporal and spatial scales to restore soil nutrient reserves that can be accessed by plants. As a result, the ENM portfolio includes a diverse array of management practices that seek to recouple elemental cycles, promote conservation pathways and gradually rebuild SOM and regenerate biogeochemical resilience (Drinkwater and Snapp, 2007a).
Figure 1. ENM expands the focus of nutrient management to SOM pools and mineral P reserves. Flows, inputs, and pools that are increased under ENM are outlined in black. Arrow colors indicate dominant elemental fluxes as follows: N only (blue), P only (green), N and P (blue green), and C (brown). Modified after Drinkwater and Snapp, 2007a.
1. Build SOM and other nutrient reserves. Because plants can access many forms of N and P though partnerships with beneficial microorganisms living in the rhizosphere, ENM targets the full range of nutrient reservoirs. The basic strategy is to conserve and build nutrient reserves that are less vulnerable to loss which can be accessed through rhizosphere and microbially mediated processes. These reserves include labile and stabilized SOM, microbial biomass, and sparingly soluble plus some forms of adsorbed P.
2. Minimize the size of N and P pools that are the most susceptible to loss. A central objective of ENM is to reduce loss pathways by minimizing standing pools of soluble inorganic N (hereafter, Ni) and Pi in soil, fostering plant and microbial assimilation, and reducing Ni and Pi additions. While the loss mechanisms differ, greater concentrations of Ni and Pi promote nutrient losses. As the concentration of Ni increases, leaching and denitrification increase. Phosphorus is less mobile, but most soils, particularly highly weathered soils commonly found in the Global South, “fix” excess soluble P though adsorption, precipitation, and occlusion. Reducing Fi rates is a powerful lever for significantly reducing Ni and Pi losses.
3. Maximize agroecosystem capacity to use soluble, Ni and Pi. Plant and microbial acquisition increase internal cycling, removing soluble nutrients from the soil solution, preventing their loss from the soil, and diverting Ni and Pi flows into SOM reserves. The greater abundance of soil C relative to Ni and Pi fuels the growth of soil organisms, increasing SOM formation.
4. Use functional and phylogenetic biodiversity to minimize bare fallows and maximize presence of growing plants. Plant and microbial species differ in their capacity to carry out elemental cycling processes. Diversifying crop rotations and reducing use of bare fallows by adding cover crops or perennials in conjunction with legume N sources are effective strategies for recoupling elemental cycles (Figure 1). Using plant diversity to maximize niche occupancy and promote a more functionally diverse soil biota can enhance overall productivity and nutrient cycling capacity of belowground communities. Integrated crop-livestock farming systems expand opportunities for diversification of plants and implementation of ENM.
5. Construct agroecosystem and field scale mass balances to track net nutrient flows over multiple growing seasons. Using simple mass balances ensures that soil mining, where crop harvests remove greater amounts of N and P compared to additions, can be detected, and addressed. Long-term removal of nutrients that exceeds nutrient additions undermines soil fertility and leads to soil degradation. This is the situation in many smallholder subsistence systems. On the other end of the spectrum, limiting the degree to which nutrient additions exceed harvested removals reduces the risk of environmental losses.
Successful application of ENM principles is grounded in a basic understanding of the biological and geochemical processes governing soil elemental cycles. Ecosystem state factors (climate, parent material, topography, potential biota, time, and human activities) are the master regulators of SOM levels (Jenny, 1941). The legacy of these factors provides the context for agricultural management to affect elemental cycles and SOM reserves (Amundson and Jenny, 1997; Kleber et al., 2015). Thus, the environmental context, management history and the current management regime determine the balance between decomposition and SOM formation/ stabilization. Over the past decade, major discoveries have fundamentally altered our understanding of SOM biogeochemistry (Kuzyakov and Xu, 2013; Lavallee et al., 2020; Liang et al., 2020; Daly et al., 2021).
The diverse functions of SOM reflect the heterogeneous composition of SOM reserves (Figure 2). Specifically, SOM pools differ in terms of turnover rate, nutrient density, the degree to which they are associated with mineral elements and their response to management practices (Lehmann and Kleber, 2015; Williams et al., 2018; Sokol et al., 2022). These distinct SOM reserves act together to support soil ecosystem integrity and plant growth.
Figure 2. Soil OM pools differ in composition and function. Compared to occluded particulate OM (POM) and mineral associated OM (MAOM), free POM is more accessible to decomposers, more energy rich but nutrient poor with a relatively fast turnover time (Liang et al., 2019; Lavallee et al., 2020). Occluded POM and MAOM are protected through their association with mineral constituents, and while they are nutrient rich, C limitation may also hinder decomposer access. As a result, plant driven priming of decomposition is most pronounced for oPOM and MAOM (Daly et al., 2021).
Most SOM is present as mineral associated organic matter (MAOM) which has turnover times ranging from decades to millennia. MAOM accounts for >90% of soil organic C (hereafter, Corg, refers to elemental C content of SOM) and plays a significant role in Corg sequestration, nutrient supply to plants and decomposers, and stabilization of small aggregates (Kleber et al., 2015). It is largely composed of microbial-derived monomers and polymers produced during decomposition and stabilized through protective associations with mineral components (von Lutzow et al., 2006; Schmidt et al., 2011; Liang et al., 2019). Rhizodeposition, litter composition, microbial C use efficiency, and soil mineralogy, all govern MAOM accrual (Puget and Drinkwater, 2001; Kallenbach et al., 2015; Lavallee et al., 2018). Interactions with minerals limit decomposer access to MAOM constituents, however, MAOM can still be accessed by decomposers and, as a result, decomposition of MAOM can dominate cycling of micro- and macro-nutrients due to the sheer size of this reserve (Lavallee et al., 2020).
Particulate organic matter (POM), defined as OM particles >53 um, is less abundant than MAOM but far more dynamic. POM consists of plant litter and microbial residues in varying stages of decay and is divided into two distinct pools that are either free from mineral interactions (free POM, hereafter fPOM), or are protected by soil minerals, usually by occlusion inside of aggregates (occluded POM, hereafter oPOM). Compared to MAOM, these labile pools which have shorter turnover times are more sensitive to management changes and accumulate C and N more quickly (Wander et al., 1994; John et al., 2005; Lavallee et al., 2020). Free POM is the raw material that is transformed into oPOM and MAOM during decomposition (Puget and Drinkwater, 2001).
While fPOM acts primarily as a food source for decomposers, oPOM contributes to a broader array of soil functions. Compared to fPOM, oPOM has a narrower C:N ratio, serves as an important source of mineralizable N and plays a significant role in aggregate stability (von Lutzow et al., 2006; Bu et al., 2015). Accordingly, increases in nutrient rich oPOM stocks contribute to soil fertility while also improving soil structural properties dependent on soil aggregation. These processes of organic N and P (hereafter Norg and Porg refer to organic forms of these elements) storage and soil aggregation in turn support plant nutrient acquisition and improve agroecosystem resiliency to extreme variation in precipitation. The sensitivity of oPOM to management changes, combined with its central role in key soil functions, makes oPOM particularly useful as an early indicator of the trajectory of SOM levels and soil health (Wander et al., 1994; Schmidt et al., 2011).
The rhizosphere is a site of plant-microbial interactions and compared to bulk soil, microbial metabolism in the rhizosphere is an order of magnitude greater due to root exudates that support microbial growth (Philippot et al., 2009). Labile exudates and other rhizodeposits alleviate energetic constraints on microbial extracellular enzyme production (Averill and Finzi, 2011; Dijkstra et al., 2013), leading to mineralization of nutrient-rich SOM (Hamilton and Frank, 2001; Weintraub et al., 2007; Kuzyakov, 2010). By supplying amino acids, sugars and other organic compounds, plants can cultivate distinct communities of microbes that facilitate decomposition of nutrient rich SOM reserves which can in turn increase the amount of N and P available to plants (Reynolds et al., 2003; Panke-Buisse et al., 2015; Jilling et al., 2018; Dijkstra et al., 2021). The significance of this process is supported by field studies where despite substantial Fi additions, Norg reserves supply a majority of grain crop N (Gardner and Drinkwater, 2009; Yan et al., 2020). The stimulation of decomposition spurs microbial growth and turnover promoting SOM formation and soil aggregation (Alami et al., 2000; Atkinson and Urwin, 2012). Thus, these plant-microbial interactions occurring in the rhizosphere contribute to the disproportionate accrual of root-derived C into oPOM and MAOM (Fulton-Smith and Cotrufo, 2019; Sokol et al., 2019a).
Greater species richness and increased plant functional diversity go together, expanding the influence of plants on a wide range of belowground processes (Cadotte et al., 2011; Cardinale et al., 2011). Examples of key plant functional traits include life history and nutrient acquisition strategies, the quantity/composition of litter and rhizodeposits, and root turnover rate (Haynes and Beare, 1997; Bardgett et al., 2014; Li et al., 2014; Poirier et al., 2018). Rhizosphere microbiome composition and function vary with plant species/cultivar and are linked to plant ecological niche and nutrient requirements (Peiffer et al., 2013; Turner et al., 2013; Emmett et al., 2017). Plant-microbial partnerships jointly control processes such as aggregate formation, N cycling dynamics and the net balance between SOM decomposition and formation in the rhizosphere (Figure 3; Briones et al., 2003; Philippot et al., 2013; Emmett et al., 2020). For example, fast growing plant species, including crops such as maize, tend to be net mineralizers and accelerate decomposition and N cycling rates (Castro-Diez et al., 2014). In contrast, many legume species build SOM because the rate of oPOM and MAOM formation in the rhizosphere exceeds decomposition (Puget and Drinkwater, 2001; Garland et al., 2018).
Figure 3. In the rhizosphere, decomposition and stabilization/formation of SOM occur simultaneously. Plants exchange C substrates for nutrients released by the decomposers. Organic compounds and ions are secreted by plant roots into the rhizosphere providing energy to decomposers and enabling them to produce expensive exoenzymes needed to access nutrient rich oPOM and MAOM fueling microbial growth. The resulting microbial biomass supports grazers and predators, and these trophic interactions release N and P that can then be taken up by roots. Growth and turnover of decomposers and soil food web organisms increases the flow of necromass and replenishes oPOM and MAOM reserves. Modified after Liang et al., 2017 and Valadares et al., 2020.
While all SOM originates from plant inputs and other organic residues (e.g., manures, composts), the bulk of stabilized SOM is composed of microbial biomass or “necromass” (Liang et al., 2017). In the same way that plant species effects on elemental cycling processes depend on life history strategies and corresponding plant functional traits, decomposers differ in their impact on C, N and P cycling processes. Microbial C use efficiency (hereafter CUE), is defined as the proportion of organic C taken up that is allocated to microbial growth (Kallenbach et al., 2019). Life history strategy determines the ecological and physiological characteristics that govern microbial allocation of energy to growth, resource acquisition, respiration, and survival (Roller et al., 2016; Malik et al., 2020). Microbial CUE ultimately determines the efficiency of microbial conversion of plant-derived C into stabilized, nutrient rich SOM reserves (Kallenbach et al., 2015; Barnett et al., 2021). Thus, the growth dynamics of soil microorganisms are another determinant of SOM accrual (Caruso et al., 2018; Sokol et al., 2019b).
Liebman and Gallandt (1997) used the phrase “many little hammers” to describe the benefits of orchestrating many practices to achieve effective weed control without the use of herbicides. This view captures the fundamental nature of all agroecological management strategies, including ENM. To achieve the short-term goals of a single growing season while being mindful of the trajectory of slower processes requires coordinated use of multiple practices while also recognizing that each practice can affect many cycling processes. Ultimately, shifts in fast cycling processes, the resulting changes in slower processes, and the ensuing feedbacks favoring conservation pathways increase the capacity of agroecosystems to supply crop nutrients (Drinkwater and Snapp, 2007a). Tolerance to stressors can also improve over the long-term as SOM accrual alters the soil environment and the soil community undergoes changes in composition (Petersen-Rockney et al., 2021). Here we first elaborate on interactions among fast and slow processes and highlight resulting feedbacks that promote soil restoration. We then discuss four core management strategies, focusing on practices that are likely to be most compatible with smallholder farming systems: (1) Diversification of plant species, (2) Inclusion of legumes and perennials, (3) Crop-livestock integration and (4) Nutrient sources and soil amendments.
There are two distinct types of feedbacks governing the positive cycle of soil restoration under ENM (Figure 4). Reinforcing (positive) feedbacks amplify change and result in transitions to different steady states. An example of a reinforcing feedback loop is the effect of management practices that increase Corg levels which in turn impact soil microbiome composition and function. Greater C abundance relative to Ni and Pi increases microbial growth and the flow of necromass derived C, N and P into SOM reserves. Greater SOM levels favor microbial communities that channel C into growth over maintenance favoring SOM accrual (McDaniel et al., 2014; Kallenbach et al., 2015; Buckeridge et al., 2020). Stablilizing (negative) feedbacks slow processes and favor dynamic steady states and greater stability. Downregulation of biological N fixation in legumes in response to increases in soil N supply capacity is an example of a stabilizing feedback loop. Greater reliance on legumes to supply N increases oPOM N reserves and soil N supply capacity resulting in legume downregulation of N2 fixation and reduces legume N additions and the potential for N surpluses and N losses over the long-term (Blesh and Drinkwater, 2013; Blesh, 2019).
Figure 4. ENM shifts soil processes to favor a positive trajectory of SOM accrual and soil restoration. The diagram depicts changes resulting from implementation of ENM in a degraded soil with a history of inadequate Fi additions and meager returns of senescent crop residues, a situation commonly found in limited resource, smallholder systems. In the short term, cycling processes and microbial communities are altered to favor conservation pathways (A) which have cascading effects on SOM accrual, nutrient retention, and microbial community composition/function (B) and lead to feedbacks that ultimately impact yields, yield stability/resilience and management (C). BNF, biological N fixation.
Under ENM, key shifts in elemental cycling, soil environmental conditions and C/N/P stocks in the short term (Figure 4A) set the stage for conservation pathways and changes in microbial community composition and function (Figure 4B) creating reinforcing feedbacks that tighten nutrient cycles and increase soil fertility leading to improved crop yields, yield stability, and permit further management adjustments (Figure 4C). Soils cannot accrue unlimited SOM or nutrient stocks, so ultimately a new steady state condition is reached. Ecosystem state factors (e.g., climate, soil texture/parent material) and management determine these limits to SOM accrual and the extent of soil restoration that can be achieved under ENM regimes.
ENM cannot be effectively implemented without strategic increases in spatial and temporal plant species diversity. Polycultures (intercropping and agroforestry), diversified rotations, and cover cropping are diversification practices used by farmers to improve yields (Figure 5). In polycultures, beneficial plant-plant interactions, including complementarity, resource partitioning and facilitation increase nutrient acquisition and improve fertilizer and water use efficiency (Iverson et al., 2014; Brooker et al., 2015; Duchene et al., 2017). Likewise, diversification of annual rotations by adding species with complementary phenology to minimize bare fallows, increases nutrient retention and promotes SOM accrual (Blesh, 2019; Hallama et al., 2019; Blanco-Canqui and Ruis, 2020; Kim et al., 2020; Beillouin et al., 2021). Maximizing belowground ecosystem services requires attention to the ecological niche and the capacity of functional groups or species to promote desirable processes. For example, while all legumes can fix N, there is considerable variation in rhizosphere effects on elemental cycling processes across species. Lupinus sp. can mobilize sparingly soluble P though rhizosphere acidification while other legume species such as Vicia sp. increase soil phosphatase activity and mineralization of Porg (Balota et al., 2014; Hallama et al., 2019). Creating polycultures through purposeful diversification with companion species offers the greatest potential to maximize complex belowground ecosystem services. In paddy rice systems, use of Azolla as a companion intercrop increases N use efficiency, reduces N losses and can provide yield benefits (Yao et al., 2018; Yang et al., 2021). Other examples are discussed in the case studies below.
Figure 5. ENM practices provide multiple ecosystem services. Management effects on desirable ecosystem services are indicated by color, with green indicating positive effects and orange indicating mixed or inconsistent effects. Dark green indicates the evidence is strong and reflects consistent results from multiple meta-analytical reviews while medium green indicates moderate evidence (i.e., ecosystem services that were considered in a limited number of meta-analyses). The lightest shade of green indicates limited evidence, including management effects where results are from the primary literature or a single meta-analysis. The split cell for cover cropping effects on nutrient retention indicates that the effect varies with cover crop composition; grasses (Gr) consistently increase nutrient retention while legumes (Leg) have inconsistent effects. Blank cells indicate ecosystem services for which there were insufficient data points to be included in the meta-analytical reviews. aLegume-grass intercrops dominate this literature; bPlant available and Norg, Porg; cNUE=Nutrient use efficiency; dPathogen and herbivore control; eCompared to Fi only.
Among the immense diversity of plants, legumes and perennial species stand out because of their exceptional capacity to expedite SOM formation/stabilization, N and P recycling efficiency, and soil restoration. Reliance on biological N fixation reduces Fi dependance and as the proportion of legume derived N increases, agroecosystem-scale N use efficiency increases, reducing the potential for environmental N losses (Blesh and Drinkwater, 2013; Blesh, 2019). Diversification with legumes can mobilize mineral P reserves and promote accrual of SOM increasing Norg/Porg stocks that are accessible to cash crops (Hallama et al., 2019; Jian et al., 2020). Furthermore, compared to annuals, perennials have a greater capacity to restore soil functions and SOM reserves (Crews et al., 2016; Crews and Rumsey, 2017). Compared to cropping systems composed of annuals, adding perennial forage species to rotations or as intercrops promotes soil restoration through reduced erosion, SOM/Norg/Porg accrual, and aggregate formation (Garland et al., 2018; Paul et al., 2020; Drinkwater et al., 2021). In agroforestry and alley cropping systems, belowground benefits increase in concert with the abundance of trees included with high value cash crops such as coffee and annual food crops (Tully et al., 2012; Cristobal-Acevedo et al., 2019).
Expansion of Fi usage as a remedy for degraded soils in smallholder systems continues to be widely promoted through government programs and development agencies (Mitchell et al., 2018). Besides the emphasis on short term outcomes such as yield improvements, the assumption is that by supplying the major limiting nutrients, yields and biomass production will be greater, and the increased crop residues will reverse soil degradation and rebuild SOM (Hickman et al., 2020; Tiefenbacher et al., 2021). Compared to organic amendments, Ni has the smallest impact on Corg accrual (Han et al., 2016; Luo et al., 2018). In cases where greater Corg is detected in Fi treatments compared to zero input controls is often due to a reduced rate of SOM loss rather than net SOM accrual (Ladha et al., 2011; Ndung'u et al., 2021; Tiefenbacher et al., 2021). Use of organic amendments, either alone or paired with Fi, accelerates SOM accrual, and provides other benefits including increases in microbial abundance/activity, including beneficials such as arbuscular mycorrhizae fungi (Figure 5; Jiang et al., 2021). While fertilizers often improve crop yields in smallholder systems, sole reliance on Fi does not provide expected yield boosts (Jayne et al., 2018) or deliver substantive soil health benefits (Ndung'u et al., 2021; Young et al., 2021). A study of over one thousand smallholder fields in Malawi is a case in point; management factors associated with soil Corg levels included crop diversity, weeds and organic residue incorporation, but not fertilizers (Tu et al., 2022).
In conjunction with additions of these nutrient sources, simple mass balances can be constructed using all inputs and harvested removals. To be of use, mass balances of N, P and K can be calculated for at least an entire rotation cycle to capture year to year variation in nutrient additions and removals. Simple input-output calculations do not include environmental losses, which can drive significant removals, particularly for N though biotic transformations or for P and K in erosion prone situations (Vanek and Drinkwater, 2013). Nevertheless, negative balances indicate that soil nutrients are being mined, while situations where N surpluses are large indicate vulnerability to denitrification and leaching losses (Tamagno et al., 2022).
The coordinated production of food crops, forages and livestock (used here to include poultry/fish/edible invertebrates) can improve family nutrition, food security and income and are the backbone of many smallholder farming systems (Lindahl et al., 2020; Paul et al., 2020; Bezner-Kerr et al., 2021). Integrated crop-livestock systems encompass all these core ENM strategies and are highly compatible with ENM because the pairing of livestock with arable food crops enables farmers to include perennial species, particularly perennial legumes either through intercropping with annual food crops or alternating pastures/forage production with annual food crops in rotation. Likewise in paddy rice production, production of poultry, fish or edible crustaceans is highly compatible with use of Azolla green manures (Sivakumar and Solaimalai, 2003; Chen et al., 2017). Compared to annual grain production systems, integrated crop-livestock systems build SOM, including oPOM and MAOM and conserve nutrients through recycling (Nayak et al., 2015; Chmelikova et al., 2021; Rui et al., 2022). In Sub-Saharan Africa, the integration of forge production with food crops significantly reduced soil loss and increased SOM as well as grain yield (Khan et al., 2014; Paul et al., 2020; Drinkwater et al., 2021). Studies of complex paddy rice systems in China that co-produce ducks or fish find multiple benefits including improved nutrient use efficiency and reduced GHG emissions compared to rice monocultures (Nayak et al., 2015).
There are a number of examples where agroecological practices and ENM have been successfully implemented to improve yields and alleviate poverty in smallholder subsistence agriculture (Pretty et al., 2006). Here, we highlight three exemplary farming systems that integrate ENM strategies.
The groundbreaking push-pull (PP) polyculture system was developed by the International Center for Insect Physiology and Ecology (icipe) in western Kenya and uses plant biodiversity to solve the complex, interrelated constraints that limit maize yields in the region (Khan et al., 2008a, 2014). Push-pull polyculture is an inexpensive win-win technology that improves grain yields, livelihoods and human wellbeing while restoring SOM reserves and agroecosystem resilience (Khan et al., 2014; Diiro et al., 2021; Drinkwater et al., 2021). Originally developed to deter lepidopteran pests that damage cereal crops in the region, 20 years of research aimed at meeting the needs of smallholder farmers have yielded an integrated system that delivers a wide range of ecosystem services.
Development of the PP system started with screening hundreds of species to find plants that could either repel key herbivores (push) or serve as trap plants (pull). The resulting PP system consists of Desmodium sp. (push) intercropped with maize or sorghum in fields surrounded by trap crop borders (pull). Volatiles released by desmodium repel stemborers and other lepidopterans while the trap crop border simultaneously attracts them out of the field preventing damage to grain crops (Khan et al., 2014). The diversified plant community also attracts natural enemies adding another prevention mechanism to reduce herbivores (Khan et al., 2000).
Push-Pull polycultures provide important belowground ecosystem services. By a stroke of luck, desmodium root exudates induce abortive germination of Striga (Striga hermonthica), an endemic parasitic weed which can reduce maize yields by as much as 80%. Desmodium intercrops eliminate striga from infested fields (Khan et al., 2000; Hamilton et al., 2012) and, despite shading from maize, Desmodium grows vigorously and fixes N, promoting SOM accrual and increasing the capacity of soils to supply N and P (Kifuko-Koech et al., 2012; Drinkwater et al., 2021). Push-pull intercropping leads to substantial increases in Norg and plant available P. Gains in Norg are divided between MaOM and oPOM pools with oPOM N accounting for >60% of Norg accrual (Drinkwater et al., 2021).
A diverse assortment of farmer-centered strategies has been employed to support adoption of PP polycultures. In the context of intensive livestock systems, which creates a demand for high quality forage, adoption has occurred on over 250,000 farms across E. Africa, in part because PP delivers yield and economic benefits within a short timeframe (Khan et al., 2014; Murage et al., 2015). The comprehensive suite of ecosystem services increases maize yields by two- to three-fold compared to maize grown under the typical farmer practices (Khan et al., 2008a, 2014; Midega et al., 2015). Moreover, both Desmodium and border plantings provide high-quality fodder enabling farmers to venture into dairy cattle and goat keeping (Khan et al., 2008b). Lastly, while establishing PP polycultures requires initial investments of seeds and labor, once established labor requirements are modest and income increases, enabling investments in child education and household goods (Diiro et al., 2021).
Parkland agroforestry is an indigenous land use system developed by farmers which allows them to grow annual crops in combination with useful trees and shrubs which are scattered among cultivated fields at varying densities (Boffa, 1999; Masters, 2021). In addition to cereals, farmers draw on a rich legacy of ethnobotanical knowledge to selectively retain native trees within fields and farmland that provide a range of medicinal, cultural, and livelihood functions. For example, Masters (2021) found that parklands systems of four cultures occupying the Aswa River catchment of northern Uganda included 88 indigenous edible plants used as sources of leafy vegetables, roots and tubers, fruits, oils, and seeds.
These systems are most common in arid and semi-arid climates where trees grow as sparse, mixed stands within rainfed staple crop and intercropped fields, frequently in or near riverine areas to tap into deep water sources and support leafing out of trees in the off season. The potential for tree-crop moisture competition is mediated through farmer management that uses intensive pruning and/or burning of lower limbs in tree species. Another common practice involves “reverse phenology” trees such as Faidherbia trees that leaf out during the off season when crops have been harvested (Ndoli et al., 2017). This is often (but not always) associated with higher crop yields and improved nutrient efficiency compared with other tree-crop combinations (Ndoli et al., 2017).
Participatory research in Gambia has shown the multifunctional nature of the parkland system, including a variety of ecosystem services valued by farmers (Stoate and Jarju, 2008). The trees generally increase SOM, and N and P availability which can be as much as two-fold higher in cultivated land near tree canopies, compared to non-tree areas. Biological N fixation is an important process supported by inclusion of leguminous trees, which is common in Parkland systems. Acacia and Sesbania species produce biomass N in excess of 150 kg N ha−1, with the exception of very sandy sites where growth is more limited (Chikowo et al., 2006). In this Zimbabwe study, legume leaf mulches increased N supply and maize yields while limiting nitrous oxide emissions. A southern Malawi trial showed accumulation of 12–15% soil Corg and POM-N over a decade in a maize-Gliricidia sepium agroforestry system (Beedy et al., 2010).
Integration of Fi with organic nutrient sources and the effect of trees on nutrient acquisition and nutrient use efficiency have been the subject of recent research in parkland agroforestry (Diallo et al., 2021). Judicious doses of external nutrients integrated with agroforestry balances nutrient supply and demand resulting in improved nutrient efficiency, as shown in millet (above) and maize fields. On-farm studies of N and P fertilizer use efficiency in Faidherbia parklands in Ethiopia and Rwanda suggest that improved nutrient use efficiency is one mechanism contributing to improved grain yields (Sida et al., 2020). In this study, four fertility treatments (0 fertilizer, +N, +P, +N&P at 30 kg ha−1 P, 64 kg ha−1 N) were compared in open fields and under Faidherbia canopies. In general, both crop yields and nutrient use efficiency were greater under tree canopies compared to open fields (Sida et al., 2020).
Parkland agroforestry is an example of a traditional, farmer-developed system which is adapted to local environmental conditions and has been improved by ENM practices that build SOM and improve production and nutrient use efficiency.
Smallholder farmers have limited access to land, labor, and large animals. Thus, plants grown strictly for cover and green manure purposes are rarely feasible to adopt. This can lead to resource degradation, as soil is left bare and residues are minimal in simplified cropping systems with reliance on short growth duration, annual crops. On-farm research has shown that while intercropping systems have yield and nutrition benefits, diversification with annual crops is rarely sufficient to ameliorate soil fertility or restore SOM (Yusuf et al., 2009; Snapp et al., 2010; Nezomba et al., 2015).
One way out of this dilemma is diversification with semi-perennial and indeterminant growth habit crops. Such plant types deliver ENM services while fitting into farming system constraints of small parcels of land. Examples include shrubs such as pigeonpea and spreading forms of cowpea, soybean, and groundnut. These require modest levels of investment in terms of seed, and they can be grown as intercrops and boundary plantings, to be compatible with staple food crops (Bezner-Kerr et al., 2007; Snapp et al., 2019). Growth types are often viney or tall, and produce grain to sell or consume, as well as providing ENM benefits through copious vegetation and deep root systems. Soil organic matter is stabilized through aggregate formation, which protects against physical and biologically mediate degradation processes (Six et al., 2006; Garland et al., 2018); thus it is promising to see evidence of soil aggregation associated with pigeonpea root systems (Spaccini et al., 2004; Garland et al., 2018). Biochemical diversity of aboveground litter is provided through mixed planting of two legumes, which supports ENM function through conserving topsoil, and providing a moist, nutrient rich environment for biological activity, along with enhanced macro pores in some cases (da Silva et al., 2022).
ENM requires attention to enhancing rhizosphere diversity for microbial function, for example, biological N fixation, N mineralization and mobilizing sparingly soluble P. Legumes are universally acknowledged to be an important plant family in such diversification efforts, yet they are often grown at very low intensity within farming systems. Grain legumes are generally sown at low population densities and over limited areas (Mhango et al., 2013), highlighting the need for expanding the legume varieties available, improving agronomy and developing market opportunities. Species such as pigeonpea, groundnut and lupin, have phosphorus-releasing traits such as specialized root exudates and microbial-assisted solubilization, which could be screened to provide improved options for ENM (Ae and Shen, 2002; Tomasi et al., 2008; Garland et al., 2018).
The doubled-up legume system is designed to enhance the presence of legumes, combining tall statured pigeonpea with short-statured groundnut varieties. The species are phenologically complementary, where the rapid early growth of the latter is not suppressed by the former, with its slow initial growth (Snapp et al., 2013). Both species enhance organic pools of soil N and P, although evidence of accrual of total carbon is variable (Witcomb, 2021). Other variations include pigeonpea-soybean and pigeonpea-cowpea doubled-up legumes, grown in rotation with maize (Kalasa et al., 2018). A recent review of innovative sustainable agriculture practices in Africa highlighted this technology as enhancing quality and quantity of grain yield while contributing to integrated nutrient management (Kuyah et al., 2021).
Agricultural systems are complex, nested social-ecological systems and farm management reflects the surrounding environmental and social context (Liu et al., 2007; Houser and Stuart, 2020). The current system of markets and government policies co-evolved with the dominant input driven, yield focused paradigm and is therefore at odds with agroecological management systems (Deguine et al., 2021). Many recommendations for changes to remove barriers have been proposed with limited success (Bettles et al., 2021; Calo et al., 2021; Vermunt et al., 2022).
Under agroecological practices, including ENM, short-term yield outcomes can vary, and sometimes yields are initially reduced (Ponisio et al., 2015). However, over the long-term, agroecological management will contribute to achieving the UN Sustainable Development Goals (SDGs) by delivering win-win outcomes for farmers and the broader society. ENM promotes diversification at multiple scales, an essential strategy for achieving Sustainable Development Goal #2 (SDG2), which aims to eliminate hunger and malnutrition. Meta-analytical studies find that compared to conventional management regimes, farm-scale diversification increases yields and profitability, improves yield stability and food security, and reduces risk (Himmelstein et al., 2017; Rosa-Schleich et al., 2019; Bezner-Kerr et al., 2021). Furthermore, a recent analysis of FAO data found that cash crop diversification at the national level increases temporal stability of the total national harvest, demonstrating that simply increasing food crop diversity provides a broad societal benefit (Renard and Tilman, 2019).
We consider three aspects of ENM that present challenges to broad adoption of ENM within the existing socio-economic context. First, ENM delivers ecosystem services beyond crop yield; these outcomes receive less support for scientific research and are not incentivized for farmers. Second, some ENM benefits, such as the restoration of the SOM pools, are incompatible with the short timeframe of market and policy incentives which are not equipped to deal with time lags extending beyond a single growing season. Lastly, ENM involves systems approaches to management of SOM dynamics and feedbacks and requires new knowledge and options, such as ENM recommendations and plants with ENM-facilitating traits. Here we discuss essential changes that will advance agricultural diversification and ENM implementation.
The current socio-ecological matrix can be shifted to implement new, diversification-friendly policies and programs to promote ENM though pathways involving both governmental and non-state actors. In the short-term, modifications to existing policies could fundamentally shift the landscape to favor diversification and ENM. For example, existing fertilizer subsides and other narrow programs could be expanded to promote farmer access to inputs needed to implement diversification and ENM [e.g., seeds and seedlings for planting multiple-purpose and soil-improving species, and livestock; (Khan et al., 2014)]. Subsidies and credit access to reduce risk such as short-term reductions in crop yields would remove one prevalent barrier to ENM implementation. Market failures can also be countered by promoting farmer access to price and market information for a wide range of products beyond commodity crops. Diversification-friendly government policies are needed, including microfinance institutions and policies directed at farmers who want to implement ENM and entrepreneurs who are interested in establishing new enterprises to supply plant materials needed for diversification. Supporting formation of farmer associations for greater market power can also help diversification (Bettles et al., 2021). These actions could provide immediate benefits for smallholder communities.
More fundamental changes to the government policies that shape agricultural development and market forces will require longer timeframes (Calo et al., 2021). One important ENM enabling policy involves the development of markets and funding mechanisms to reward farmers for provision of ecosystem services. This has proven to be controversial and challenging to implement (Kosoy and Corbera, 2010; Kolinjivadi et al., 2015). The difficulty in quantifying ecosystem services such as reduced GHG emissions, SOC sequestration or improved water quality is a major barrier to monetizing ecosystem services beyond yields per hectare. To circumvent the need to monitor actual outcomes at the farm or field scale, policy efforts could target proven management systems and practices using the large body of research linking management to these desirable outcomes. For example, direct payments for agricultural diversification, is one way to incentivize ENM and related management systems that promote ecosystem services (Renard and Tilman, 2019; Tamburini et al., 2020). While the multidimensional benefits of diversification are well-documented, criteria for specific management systems that meet policy goals need to be developed to ensure that diversification schemes are suited for specific environmental and social contexts (Bettles et al., 2021). For example, diversification of cash crops is sufficient for progress with goals related to reduced risk of crop failure and yield stabilization (Renard and Tilman, 2019). However, to promote SOM accrual and deliver a broader suite of ecosystem services that will sustainably improve food security and human wellbeing requires a broader approach to diversification that considers the full range of ecological actors and their ecosystem functions including companion plants that do not have a cash value per se as well as integration of plant and animal production (Figure 5).
Massive public investments in fertilizer subsidies and a narrow range of crop varieties has come at the expense of investments in knowledge-based ecological management (Ariga et al., 2019). Research priorities follow this lead and concentrate on Fi management with the goal of maximizing yields per hectare, while paying limited attention to the multilayered priorities of farmers and the biological processes governing nutrient cycling in agroecosystems. For example, in a review of more than 200 sustainable intensification studies on smallholder farms, >70% used yield as the primary metric to evaluate technology performance (Reich et al., 2021) and a meta-analysis of N management publications found that only 12% of the data points were from studies of Norg sources (Yan et al., 2020).
Several modest course corrections to research will start the process of realigning research to advance agroecological technologies and ENM that are both effective and compatible with smallholder farming systems. On-farm research is now a normative strategy and improved engagement of farmers is essential for meeting the UN Sustainable Development Goals. Farmers possess both experience-based and experimental knowledge of their farming systems, and this perspective adds to scientific knowledge systems and increases the likelihood of successful agroecological cropping system redesigns (Doré et al., 2011). Above all, farmer engagement ensures that performance assessments include farmer indicators and household priorities including yield oriented metrics that are useful to farmers. Yield can be contextualized by expanding yield metrics to reflect farmer priorities such as yield output relative to limited resources such as labor, purchased inputs or water used in irrigation (Avendano-Reyes et al., 2020; Diiro et al., 2021). Research is also needed to address socio-economic barriers to implementing ENM practices, including labor access and land tenure (Calo et al., 2021).
In conjunction with farmer input, better understanding of soil ecological processes and management effects on these processes will reduce the trial and error of cropping systems development and promote development of new agroecological management systems that will improve food security, resilience, and sustainability for smallholder communities across the globe. Research geared toward optimizing management to meet the five ENM guiding principles is urgently needed, such as a better understanding of SOM cycling, plant-microbiome interactions, and organic-nutrient replenishment pathways governing oPOM and MAOM reserves. In particular, management of soil N supply through replenishing SOM reserves deserves more research, as does extension messaging around this approach. Synchrony of N supply, as well as managing fresh residues, partially decomposed and Norg pools are all areas critical to ENM.
In the short-term, focusing this research on soil biogeochemical cycling in successful diversification/ENM systems such as those highlighted in the case studies would be a good starting point. To support and improve ENM, research on soil food webs, and delineation of trophic interactions along the lines of the new multichannel model recently proposed is needed (Potapov, 2022). In addition, considering the prevalence of highly weathered acidic soils in regions dominated by smallholder systems, research targeting plant-mycorrhizal associations that can enhance Pi access and facilitate the movement of Pi into Porg pools should be a priority (Gianinazzi et al., 2010; Koskey et al., 2021).
Crop breeding programs can advance ENM by intensifying plant selection on several fronts to expand plant traits compatible with ENM regimes. There has been a loss of traits that allow plants to maintain yields under non-saturating nutrient conditions and plant selection approaches may have led to disruption of plant-microbial mutualisms (Perez-Jaramillo et al., 2016; Jaiswal et al., 2020; Isaac et al., 2021). Over the near-term improvements could be made in breeding for ENM. This includes profiling existing cultivars and companion species for their belowground traits to jump-start breeding efforts. Substantive differences in belowground traits occur across existing cultivars and characterizing the impact of species/cultivars on key SOM pools will identify cultivars that have greater potential to perform well under ENM and enable more targeted EMN strategies. For example, rhizosphere priming of SOM varied from 8 to 18 μg C g−1 soil across maize lines (Gowda et al., 2021) and stabilization of root-derived C varied by 70% among barley cultivars (Mwafulirwa et al., 2021). Inclusion of neglected crop species, landraces and cultivars that may have superior adaptations to local conditions can expand belowground traits and genetic resources available for breeding programs.
Over the longer-term, investments are needed in the development of cultivars that possess a wider range of nutrient acquisition strategies, and that benefit from plant-microbial partnerships. Cultivars of important food crops need to be bred for improved performance in diversification schemes ranging from cultivar mixtures to polycultures and agroforestry systems (Bourke et al., 2021; Wuest et al., 2021). Development of improved cover crops and other companion species is clearly needed, with traits that maximize belowground ecosystem services such as oPOM and MAOM accrual. Expanding the companion species toolbox will broadly support diversification beyond ENM implementation and provide multiple benefits including improved food security and resilience (Tamburini et al., 2020; Bezner-Kerr et al., 2021). Bringing farmers to the table to expand the knowledge base and engage in participatory breeding programs has proven to be beneficial for developing genotypes that are better adapted to farmers' needs (Alves et al., 2018). In the long-term, development of perennial and semi-perennial staple crops would provide tremendous advantages over annuals in terms of soil restoration and sustainability (Crews et al., 2016).
Lack of ecological literacy among agronomists, extensionists and farmers is another barrier to agroecological management systems such as ENM and marked expansion of education on agroecology is needed at all levels (Deguine et al., 2021). Universities, public educational institutions, and government extension could all be important sources of agroecology training if this were the focus of education efforts. In wealthy nations, universities are offering graduate programs in agroecology and this trend needs to be promoted more broadly in developing nations (Eksvard et al., 2014). ENM can be enhanced by extension education that promotes understanding of SOM functions, nutrient cycling processes and biodiversity-ecosystem function concepts. A well-rounded ecological knowledge base is informed by formal education but includes informal learning and indigenous knowledge (Occelli et al., 2021). For example, farmers understand that legumes increase soil N fertility, but they often lack knowledge of key factors that govern N fixation rates as reflected by the high rates of N fertilizers some farmers apply to legume-grain intercrops (Drinkwater et al., 2021). For ENM to be adaptable to local conditions, peer knowledge is important, and can be facilitated through the support of networks for farmer to farmer exchange and curricula that build on local knowledge (Bezner-Kerr et al., 2019). Farmer-farmer learning, participatory extension and farmer field schools are all effective strategies for fostering farmer agency and agroecological management (Doré et al., 2011).
Another key area of investment is tools and training in on-farm problem solving for adaptive management, an essential element of ENM and all agroecological management systems (Lin, 2011). Farmers develop their own systems for evaluating performance and troubleshooting, and these strategies can be supplemented with technologies that can be used in the field (Falkowski and Drinkwater, 2020). Newly developed handheld sensors and digital tools that measure soil Corg levels are one example; feedback on soil Corg accrual is valuable to farmers who practice ENM and for implementing payments for C sequestration (Tieszen et al., 2004). For instance, in Malawi, inexpensive handheld sensors enabled extension educators to visit with farmers and provide reliable information on real-time soil Corg status and crop N response (Ewing et al., 2021).
Under the dominant agricultural production regime, farmers use fertilizers and other agrochemicals to maximize yields and to compensate for loss of the ecosystem services once provided by species diversity. This choice of crop yield as the primary performance indicator is particularly untenable given the limited resources and widespread abject poverty of rural communities in the developing world. In fact, the promotion of resource intensive agricultural technologies combined with the constraints imposed by poverty and food insecurity have fueled pervasive soil degradation. Farmers are caught in a vicious cycle where declining soil function requires intensification and increases the need for purchased inputs to produce crops, which only reinforces the trend of soil degradation—in essence, a fertilizer treadmill.
Reversing this downward spiral in the face of a changing climate coupled with unfettered intensification and widespread ecosystem degradation requires a change in strategy. Agroecological approaches and the use of agricultural diversification to restore ecological integrity provide the most promising pathway for advancing sustainable poverty alleviation and food security in regions where malnutrition and hunger are endemic. Ecological nutrient management falls within the portfolio of ecologically based management and offers a comprehensive approach to soil fertility. It recouples elemental cycles, promotes conservation pathways and gradually rebuilds SOM for resilience. ENM rests on a solid foundation of ecosystem and biogeochemical scientific understanding, and despite the many barriers imposed by current agricultural policies, successful ENM systems are being used by smallholder farmers with promising results. Likewise, there is progress in some development organizations in recognizing the value of agroecological management systems including ENM; however, policy changes that promote an enabling environment are essential for implementation of ENM and sustainable solutions to malnutrition and food insecurity.
LD and SS jointly developed the structure and content of the review and jointly conceptualized the pathways section. LD conceptualized and drafted the ENM guidelines, ecosystem processes and ecological nutrient management sections, the push-pull case study, figures, and tables. SS drafted the pathways section and the parkland and doubled up legumes case studies. Both authors contributed to drafting the conclusions and editing the entire manuscript.
The authors declare that the research was conducted in the absence of any commercial or financial relationships that could be construed as a potential conflict of interest.
All claims expressed in this article are solely those of the authors and do not necessarily represent those of their affiliated organizations, or those of the publisher, the editors and the reviewers. Any product that may be evaluated in this article, or claim that may be made by its manufacturer, is not guaranteed or endorsed by the publisher.
1. ^Refers to soil organic matter in its entirety including all elements.
Aber, J. D., Nadelhoffer, K. J., Steudler, P., and Melillo, J. M. (1989). Nitrogen saturation in northern forest ecosystems. Bioscience 39, 378–386. doi: 10.2307/1311067
Ae, N., and Shen, R. F. (2002). Root cell-wall properties are proposed to contribute to phosphorus (P) mobilization by groundnut and pigeonpea. Plant Soil 245, 95–103. doi: 10.1023/A:1020669326602
Agren, G. I., and Bosatta, E. (1988). Nitrogen saturation of terrestrial ecosystems. Environ. Pollut. 54, 185–197. doi: 10.1016/0269-7491(88)90111-X
Alami, Y., Achouak, W., Marol, C., and Heulin, T. (2000). Rhizosphere soil aggregation and plant growth promotion of sunflowers by an exopolysaccharide-producing Rhizobium sp strain isolated from sunflower roots. Appl. Environ. Microbiol. 66, 3393–3398. doi: 10.1128/AEM.66.8.3393-3398.2000
Alves, M. L., Belo, M., Carbas, B., Brites, C., Paulo, M., Mendes-Moreira, P., et al. (2018). Long-term on-farm participatory maize breeding by stratified mass selection retains molecular diversity while improving agronomic performance. Evol. Appl. 11, 254–270. doi: 10.1111/eva.12549
Amundson, R., and Jenny, H. (1997). Thinking of biology: on a state factor model of ecosystems. Bioscience 47, 536–543. doi: 10.2307/1313122
Ariga, J., Mabaya, E., Waithaka, M., and Wanzala-Mlobela, M. (2019). Can improved agricultural technologies spur a green revolution in Africa? A multicountry analysis of seed and fertilizer delivery systems. Agric. Econ. 50, 63–74. doi: 10.1111/agec.12533
Atkinson, N. J., and Urwin, P. E. (2012). The interaction of plant biotic and abiotic stresses: from genes to the field. J. Exp. Bot. 63, 3523–3543. doi: 10.1093/jxb/ers100
Attiwill, P. M., and Adams, M. A. (1993). Nutrient cycling in forests. New Phytol. 124, 561–582. doi: 10.1111/j.1469-8137.1993.tb03847.x
Avendano-Reyes, L., Robinson, P. H., Hernandez-Rivera, J. A., Correa-Calderon, A., Lopez-Lopez, A., Mellado, M., et al. (2020). Characterization of small-scale dairy farms and its relation to water use efficiency in the Mexicali Valley, Mexico. Trop. Anim. Health Prod. 52, 1141–1148. doi: 10.1007/s11250-019-02109-4
Averill, C., and Finzi, A. (2011). Plant regulation of microbial enzyme production in situ. Soil Biol. Biochem. 43, 2457–2460. doi: 10.1016/j.soilbio.2011.09.002
Balota, E. L., Calegari, A., Nakatani, A. S., and Coyne, M. S. (2014). Benefits of winter cover crops and no-tillage for microbial parameters in a Brazilian oxisol: a long-term study. Agric. Ecosyst. Environ. 197, 31–40. doi: 10.1016/j.agee.2014.07.010
Barbier, E. B., and Hochard, J. P. (2018). Land degradation and poverty. Nat. Sustain. 1, 623–631. doi: 10.1038/s41893-018-0155-4
Bardgett, R. D., Mommer, L., and De Vries, T. F. (2014). Going underground: root traits as drivers of ecosystem processes. Trends Ecol. Evol. 29, 692–699. doi: 10.1016/j.tree.2014.10.006
Barnett, S. E., Youngblut, N. D., Koechli, C. N., and Buckley, D. H. (2021). Multisubstrate DNA stable isotope probing reveals guild structure of bacteria that mediate soil carbon cycling. Proc. Natl. Acad. Sci. USA. 118. doi: 10.1073/pnas.2115292118
Beedy, T. L., Snapp, S. S., Akinnifesi, F. K., and Sileshi, G. W. (2010). Impact of Gliricidia sepium intercropping on soil organic matter fractions in a maize-based cropping system. Agric. Ecosyst. Environ. 138, 139–146. doi: 10.1016/j.agee.2010.04.008
Beillouin, D., Ben-Ari, T., Malezieux, E., Seufert, V., and Makowski, D. (2021). Positive but variable effects of crop diversification on biodiversity and ecosystem services. Glob. Chang. Biol. 27, 4697–4710. doi: 10.1111/gcb.15747
Bettles, J., Battisti, D. S., Cook-Patton, S. C., Kroeger, T., Spector, J. T., Wolff, N. H., et al. (2021). Agroforestry and non-state actors: a review. For. Policy Econ. 130, 102538. doi: 10.1016/j.forpol.2021.102538
Bezner-Kerr, R., Madsen, S., Stuber, M., Liebert, J., Enloe, S., Borghino, N., et al. (2021). Can agroecology improve food security and nutrition? A review. Global Food Sec. Agric. Policy Econ. Environ. 29, 100540. doi: 10.1016/j.gfs.2021.100540
Bezner-Kerr, R., Snapp, S., Chirwa, M., Shumba, L., and Msachi, R. (2007). Participatory research on legume diversification with malawian smallholder farmers for improved human nutrition and soil fertility. Exp. Agric. 43, 437–453. doi: 10.1017/S0014479707005339
Bezner-Kerr, R., Young, S. L., Young, C., Santoso, M. V., Magalasi, M., Entz, M., et al. (2019). Farming for change: developing a participatory curriculum on agroecology, nutrition, climate change and social equity in Malawi and Tanzania. Agric. Human Values 36, 549–566. doi: 10.1007/s10460-018-09906-x
Blanco-Canqui, H., and Ruis, S. J. (2020). Cover crop impacts on soil physical properties: a review. Soil Sci. Soc. Am. J. 84, 1527–1576. doi: 10.1002/saj2.20129
Blesh, J. (2019). Feedbacks between nitrogen fixation and soil organic matter increase ecosystem functions in diversified agroecosystems. Ecol. Appl. 29, e01986. doi: 10.1002/eap.1986
Blesh, J., and Drinkwater, L. E. (2013). The impact of nitrogen source and crop rotation on nitrogen mass balances in the Mississippi River Basin. Ecol. Appl. 23, 1017–1035. doi: 10.1890/12-0132.1
Bourke, P. M., Evers, J. B., Bijma, P., van Apeldoorn, D. F., Smulders, M. J. M., Kuyper, T. W., et al. (2021). Breeding beyond monoculture: putting the “intercrop” into crops. Front. Plant Sci. 12. doi: 10.3389/fpls.2021.734167
Briones, A. M., Okabe, S., Umemiya, Y., Ramsing, N. B., Reichardt, W., and Okuyama, H. (2003). Ammonia-oxidizing bacteria on root biofilms and their possible contribution to N use efficiency of different rice cultivars. Plant Soil 250, 335–348. doi: 10.1023/A:1022897621223
Brooker, R. W., Bennett, A. E., Cong, W.-F., Daniell, T. J., George, T. S., Hallett, P. D., et al. (2015). Improving intercropping: a synthesis of research in agronomy, plant physiology and ecology. New Phytol. 206, 107–117. doi: 10.1111/nph.13132
Bu, R. Y., Lu, J. W., Ren, T., Liu, B., Li, X. K., and Cong, R. H. (2015). Particulate organic matter affects soil nitrogen mineralization under two crop rotation systems. PLoS ONE 10, e0143835. doi: 10.1371/journal.pone.0143835
Buckeridge, K. M., La Rosa, A. F., Mason, K. E., Whitaker, J., McNamara, N. P., Grant, H. K., et al. (2020). Sticky dead microbes: rapid abiotic retention of microbial necromass in soil. Soil Biol. Biochem. 149, 107929. doi: 10.1016/j.soilbio.2020.107929
Cadotte, M. W., Carscadden, K., and Mirotchnick, N. (2011). Beyond species: Functional diversity and the maintenance of ecological processes and services. J. Appl. Ecol. 48, 1079–1087. doi: 10.1111/j.1365-2664.2011.02048.x
Calo, A., McKee, A., Perrin, C., Gasselin, P., McGreevy, S., Sippel, S. R., et al. (2021). Achieving food system resilience requires challenging dominant land property regimes. Front. Sustain. Food Syst. 5. doi: 10.3389/fsufs.2021.683544
Cardinale, B. J., Matulich, K. L., Hooper, D. U., Byrnes, J. E., Duffy, E., Gamfeldt, L., et al. (2011). The functional role of producer diversity in ecosystems. Am. J. Bot. 98, 572–592. doi: 10.3732/ajb.1000364
Caruso, T., De Vries, F. T., Bardgett, R. D., and Lehmann, J. (2018). Soil organic carbon dynamics matching ecological equilibrium theory. Ecol. Evol. 8, 11169–11178. doi: 10.1002/ece3.4586
Cassman, K. G., Dobermann, A., and Walters, D. T. (2002). Agroecosystems, nitrogen-use efficiency, nitrogen management. AMBIO. 31, 132–140. doi: 10.1579/0044-7447-31.2.132
Castro-Diez, P., Godoy, O., Alonso, A., Gallardo, A., and Saldana, A. (2014). What explains variation in the impacts of exotic plant invasions on the nitrogen cycle? A meta-analysis. Ecol. Lett. 17, 1–12. doi: 10.1111/ele.12197
Chen, H., Tang, J. J., Sun, X. B., Ma, K. Y., Chen, H. H., and Li, D. J. (2021). Topography modulates effects of nitrogen deposition on microbial resource limitation in a nitrogen-saturated subtropical forest. For. Ecosyst. 8, 68. doi: 10.1186/s40663-021-00341-9
Chen, Y., Qiu, S., Xu, Z., Li, J., Wu, Y., Qi, G., et al. (2017). Research progresses on rice-duck-azolla integrated farming, a novel ecological agriculture model. Anim. Husbandry Feed Sci. 38, 90–92.
Chikowo, R., Mapfumo, P., Leffelaar, P. A., and Giller, K. E. (2006). Integrating legumes to improve N cycling on smallholder farms in sub-humid Zimbabwe: resource quality, biophysical and environmental limitations. Nutr. Cycl. Agroecosystems 76, 219–231. doi: 10.1007/s10705-005-2651-y
Chmelikova, L., Schmid, H., Anke, S., and Hulsbergen, K. J. (2021). Nitrogen-use efficiency of organic and conventional arable and dairy farming systems in Germany. Nutr. Cycl. Agroecosystems 119, 337–354. doi: 10.1007/s10705-021-10126-9
Crews, T. E., Blesh, J., Culman, S. W., Hayes, R. C., Jensen, E. S., Mack, M. C., et al. (2016). Going where no grains have gone before: from early to mid-succession. Agric. Ecosyst. Environ. 223, 223–238. doi: 10.1016/j.agee.2016.03.012
Crews, T. E., and Rumsey, B. E. (2017). What agriculture can learn from native ecosystems in building soil organic matter: a review. Sustainability 9, 578. doi: 10.3390/su9040578
Cristobal-Acevedo, D., Tinoco-Rueda, J. A., Prado-Hernandez, J. V., and Hernandez-Acosta, E. (2019). Soil carbon and nitrogen in tropical montane cloud forest, agroforestry and coffee monoculture systems. Revista Chapingo Serie Ciencias Forestales Y Del Ambiente 25, 169–184. doi: 10.5154/r.rchscfa.2018.09.070
da Silva, J. P., Teixeira, R. D., da Silva, I. R., Soares, E. M. B., and Lima, A. M. N. (2022). Decomposition and nutrient release from legume and non-legume residues in a tropical soil. Eur. J. Soil Sci. 73. doi: 10.1111/ejss.13151
Daly, A. B., Jilling, A., Bowles, T. M., Buchkowski, R. W., Frey, S. D., Kallenbach, C. M., et al. (2021). A holistic framework integrating plant-microbe-mineral regulation of soil bioavailable nitrogen. Biogeochemistry 154, 211–229. doi: 10.1007/s10533-021-00793-9
Daryanto, S., Fu, B. J., Zhao, W. W., Wang, S., Jacinthe, P. A., and Wang, L. X. (2020). Ecosystem service provision of grain legume and cereal intercropping in Africa. Agric. Syst. 178. doi: 10.1016/j.agsy.2019.102761
De Stefano, A., and Jacobson, M. G. (2018). Soil carbon sequestration in agroforestry systems: a meta-analysis. Agrofor. Syst. 92, 285–299. doi: 10.1007/s10457-017-0147-9
Deguine, J. P., Aubertot, J. N., Flor, R. J., Lescourret, F., Wyckhuys, K. A. G., and Ratnadass, A. (2021). Integrated pest management: good intentions, hard realities. A review. Agron. Sustain. Dev. 41, 38. doi: 10.1007/s13593-021-00689-w
Deng, Q., Hui, D. F., Dennis, S., and Reddy, K. C. (2017). Responses of terrestrial ecosystem phosphorus cycling to nitrogen addition: a meta-analysis. Glob. Ecol. Biogeogr. 26, 713–728. doi: 10.1111/geb.12576
Diallo, M. B., Akponikpe, P. B. I., Abasse, T., Fatondji, D., and Agbossou, E. K. (2021). Combined effect of trees and soil fertility management practices on millet yields in the Sahel. Agrofor. Syst. 95, 717–730. doi: 10.1007/s10457-021-00624-8
Diiro, G. M., Fisher, M., Kassie, M., Muriithi, B. W., and Muricho, G. (2021). How does adoption of labor saving agricultural technologies affect intrahousehold resource allocations? The case of push-pull technology in Western Kenya. Food Policy 102, 102114. doi: 10.1016/j.foodpol.2021.102114
Dijkstra, F. A., Carrillo, Y., Pendall, E., and Morgan, J. A. (2013). Rhizosphere priming: a nutrient perspective. Front. Microbiol. 4. doi: 10.3389/fmicb.2013.00216
Dijkstra, F. A., Zhu, B., and Cheng, W. X. (2021). Root effects on soil organic carbon: a double-edged sword. New Phytol. 230, 60–65. doi: 10.1111/nph.17082
Doré, T., Makowski, D., Malezieux, E., Munier-Jolain, N., Tchamitchian, M., and Tittonell, P. (2011). Facing up to the paradigm of ecological intensification in agronomy: revisiting methods, concepts and knowledge. Eur. J. Agron. 34, 197–210. doi: 10.1016/j.eja.2011.02.006
Drinkwater, L. E., Midega, C. A. O., Awuor, R., Nyagol, D., and Khan, Z. R. (2021). Perennial legume intercrops provide multiple belowground ecosystem services in smallholder farming systems. Agric. Ecosyst. Environ. 320, 107566. doi: 10.1016/j.agee.2021.107566
Drinkwater, L. E., Schipanski, M., Snapp, S. S., and Jackson, L. E. (2017). “Ecologically based nutrient management,” in Agricultural Systems: Agroecology and Rural Innovation for Development, eds S. S. Snapp, and B. Pounds (Cambridge, MA: Academic Press), p. 203–257.
Drinkwater, L. E., and Snapp, S. S. (2007a). “Nutrients in agroecosystems: rethinking the management paradigm,” in Advances in Agronomy, Vol. 92, eds Sparks DL (San Diego: Elsevier Academic Press Inc).
Drinkwater, L. E., and Snapp, S. S. (2007b). “Understanding and managing the rhizosphere in agroecosystems,” in The Rhizosphere-An Ecological Perspective, eds Z. Cardon and J. Whitbeck (Amsterdam: Elsevier Press).
Du, Y. D., Cui, B. J., Zhang, Q., Wang, Z., Sun, J., and Niu, W. Q. (2020). Effects of manure fertilizer on crop yield and soil properties in China: a meta-analysis. Catena 193, 104617. doi: 10.1016/j.catena.2020.104617
Duchene, O., Vian, J. F., and Celette, F. (2017). Intercropping with legume for agroecological cropping systems: complementarity and facilitation processes and the importance of soil microorganisms. A review. Agric. Ecosyst. Environ. 240, 148–161. doi: 10.1016/j.agee.2017.02.019
Eksvard, K., Salomonsson, L., Francis, C., Tesfay, G., Abraha, Z., Kiggundu, S. T., et al. (2014). Narrowing the gap between academia and practice through agroecology: designing education and planning for action. NACTA J. 58, 148–154.
Emmett, B. D., Buckely, D. H., and Drinkwater, L. E. (2020). Plant growth rate and nitrogen uptake shape rhizosphere bacterial community composition and activity in an agricultural field. New Phytol. 225, 960–973. doi: 10.1111/nph.16171
Emmett, B. D., Youngblut, N. D., Buckley, D. H., and Drinkwater, L. E. (2017). Plant phylogeny and life history shape rhizosphere bacterial microbiome of summer annuals in an agricultural field. Front. Microbiol. 8. doi: 10.3389/fmicb.2017.02414
Ewing, P. M., TerAvest, D., Tu, X., and Snapp, S. S. (2021). Accessible, affordable, fine-scale estimates of soil carbon for sustainable management in sub-Saharan Africa. Soil Sci. Soc. Am. J. 85, 1814–1826. doi: 10.1002/saj2.20263
Falkowski, T., and Drinkwater, L. E. (2020). Problem Solving and Innovation on the Farm: A How-to Manual. Burlington, VT: Northeast Sustainable Agriculture Research and Education Program, p. 95.
Fixen, P. E. (2020). A brief account of the genesis of 4R nutrient stewardship. Agron. J. 112, 4511–4518. doi: 10.1002/agj2.20315
Fulton-Smith, S., and Cotrufo, M. F. (2019). Pathways of soil organic matter formation from above and belowground inputs in a Sorghum bicolor bioenergy crop. Glob. Change Biol. Bioenergy 11, 971–987. doi: 10.1111/gcbb.12598
Gardner, J. B., and Drinkwater, L. E. (2009). The fate of nitrogen in grain cropping systems: a meta-analysis of 15N field experiments. Ecol. Appl. 19, 2167–2184. doi: 10.1890/08-1122.1
Garland, G., Bunemann, E. K., Oberson, A., Frossard, E., Snapp, S., Chikowo, R., et al. (2018). Phosphorus cycling within soil aggregate fractions of a highly weathered tropical soil: a conceptual model. Soil Biol. Biochem. 116, 91–98. doi: 10.1016/j.soilbio.2017.10.007
Gianinazzi, S., Gollotte, A., Binet, M. N., van Tuinen, D., Redecker, D., and Wipf, D. (2010). Agroecology: the key role of arbuscular mycorrhizas in ecosystem services. Mycorrhiza 20, 519–530. doi: 10.1007/s00572-010-0333-3
Gowda, M., Cairns, J. E., Mwafulirwa, L., Daniell, T. J., Thierfelder, C., Paterson, E., et al. (2021). Evidence of a plant genetic basis for maize roots impacting soil organic matter mineralization. Soil Biol. Biochem. 161, 108402. doi: 10.1016/j.soilbio.2021.108402
Gu, C. F., Bastiaans, L., Anten, N. P. R. D., and Makowski, and W., van der Werf. (2021). Annual intercropping suppresses weeds: a meta-analysis. Agric. Ecosyst. Environ. 322, 107658. doi: 10.1016/j.agee.2021.107658
Hallama, M., Pekrun, C., Lambers, H., and Kandeler, E. (2019). Hidden miners - the roles of cover crops and soil microorganisms in phosphorus cycling through agroecosystems. Plant Soil 434, 7–45. doi: 10.1007/s11104-018-3810-7
Hamilton, E. W., and Frank, D. A. (2001). Can plants stimulate soil microbes and their own nutrient supply? Evidence from a grazing tolerant grass. Ecology 82, 2397–2402. doi: 10.1890/0012-9658(2001)082(2397:CPSSMA)2.0.CO;2
Hamilton, M. L., Kuate, S. P., Brazier-Hicks, M., Caulfield, J. C., Rose, R., Edwards, R., et al. (2012). Elucidation of the biosynthesis of the di-C-glycosylflavone isoschaftoside, an allelopathic component from Desmodium spp. that inhibits Striga spp. development. Phytochemistry 84, 169–176. doi: 10.1016/j.phytochem.2012.08.005
Han, P. F., Zhang, W., Wang, G. C., Sun, W. J., and Huang, Y. (2016). Changes in soil organic carbon in croplands subjected to fertilizer management: a global meta-analysis. Sci. Rep. 6. doi: 10.1038/srep27199
Haynes, R., and Beare, M. (1997). Influence of six crop species on aggregate stability and some labile organic matter fractions. Soil Biol. Biochem. 29, 1647–1653. doi: 10.1016/S0038-0717(97)00078-3
Hickman, J. E., Zingore, S., Galy-Lacaux, C., Kihara, J., Bekunda, M., and Palm, C. A. (2020). “Assessing synergies and trade-offs from nitrogen use in Africa,” in Just Enough Nitrogen: Perspectives on How to Get There for Regions With Too Much and Too Little Nitrogen, eds Sutton MA (Cham: Springer International Publishing).
Himmelstein, J., Ares, A., Gallagher, D., and Myers, J. (2017). A meta-analysis of intercropping in Africa: impacts on crop yield, farmer income, and integrated pest management effects. Int. J. Agric. Sustain. 15, 1–10. doi: 10.1080/14735903.2016.1242332
Houser, M., and Stuart, D. (2020). An accelerating treadmill and overlooked contradiction in industrial agriculture: climate change and nitrogen gertilizer. J. Agrar. Chang. 20, 215–237. doi: 10.1111/joac.12341
Isaac, M. E., Nimmo, V., Gaudin, A. C. M., Leptin, A., Schmidt, J. E., Kallenbach, C. M., et al. (2021). Crop domestication, root trait syndromes, and soil nutrient acquisition in organic agroecosystems: a systematic review. Front. Sustain. Food Syst. 5, 716480. doi: 10.3389/fsufs.2021.716480
Iverson, A. L., Marin, L. E., Ennis, K. K., Gonthier, D. J., Connor-Barrie, B. T., Remfert, J. L., et al. (2014). Do polycultures promote win-wins or trade-offs in agricultural ecosystem services? A meta-analysis. J. Appl. Ecol. 51, 1593–1602. doi: 10.1111/1365-2664.12334
Jaiswal, A. K., Mengiste, T. D., Myers, J. R., Egel, D. S., and Hoagland, L. A. (2020). Tomato domestication attenuated responsiveness to a beneficial soil microbe for plant growth promotion and induction of systemic resistance to foliar pathogens. Front. Microbiol. 11. doi: 10.3389/fmicb.2020.604566
Jayne, T. S., Mason, N. M., Burke, W. J., and Ariga, J. (2018). Review: Taking stock of Africa's second-generation agricultural input subsidy programs. Food Policy 75, 1–14. doi: 10.1016/j.foodpol.2018.01.003
Jian, J. S., Du, X., Reiter, M. S., and Stewart, R. D. (2020). A meta-analysis of global cropland soil carbon changes due to cover cropping. Soil Biol. Biochem. 143. doi: 10.1016/j.soilbio.2020.107735
Jiang, S. T., An, X. R., Shao, Y. D., Kang, Y. L., Chen, T. S., Mei, X. L., et al. (2021). Responses of arbuscular mycorrhizal fungi occurrence to organic fertilizer: a meta-analysis of field studies. Plant Soil. 469, 89–105. doi: 10.1007/s11104-021-05153-y
Jilling, A., Keiluweit, M., Contosta, A. R., Frey, S., Schimel, J., Schnecker, J., et al. (2018). Minerals in the rhizosphere: overlooked mediators of soil nitrogen availability to plants and microbes. Biogeochemistry 139, 103–122. doi: 10.1007/s10533-018-0459-5
John, B., Yamashita, T., Ludwig, B., and Flessa, H. (2005). Storage of organic carbon in aggregate and density fractions of silty soils under different types of land use. Geoderma 128, 63–79. doi: 10.1016/j.geoderma.2004.12.013
Kalasa, J. B., Mhango, W. G., Kanyama-Phiri, G. Y., and Lowole, M. W. (2018). Maize response to doubled-up legumes, compost manure and inorganic fertiliser on smallholder farms in Ntcheu district of Malawi. Afr. J. Agric. Res. 13, 1101–1110. doi: 10.5897/AJAR2017.12917
Kallenbach, C. M., Grandy, A. S., Frey, S. D., and Diefendorf, A. F. (2015). Microbial physiology and necromass regulate agricultural soil carbon accumulation. Soil Biol. Biochem. 91, 279–290. doi: 10.1016/j.soilbio.2015.09.005
Kallenbach, C. M., Wallenstein, M. D., Schipanksi, M. E., and Grandy, A. S. (2019). Managing agroecosystems for soil microbial carbon use efficiency: ecological unknowns, potential outcomes, and a path forward. Front. Microbiol. 10. doi: 10.3389/fmicb.2019.01146
Khan, Z. R., Midega, C. A. O., Amudavi, D. M., Hassanali, A., and Pickett, J. A. (2008a). On-farm evaluation of the 'push-pull' technology for the control of stemborers and striga weed on maize in western Kenya. Field Crops Res. 106, 224–233. doi: 10.1016/j.fcr.2007.12.002
Khan, Z. R., Midega, C. A. O., Njuguna, E. M., Arnudavi, D. M., Wanyama, J. M., and Pickett, J. A. (2008b). Economic performance of the 'push-pull' technology for stemborer and Striga control in smallholder farming systems in western Kenya. Crop Prot. 27, 1084–1097. doi: 10.1016/j.cropro.2008.01.005
Khan, Z. R., Midega, C. A. O., Pittchar, J. O., Murage, A. W., Birkett, M. A., Bruce, T. J. A., et al. (2014). Achieving food security for one million sub-Saharan African poor through push-pull innovation by 2020. Philos. Trans. R. Soc B-Biol. Sci. 369, 20120284. doi: 10.1098/rstb.2012.0284
Khan, Z. R., Pickett, J. A., van den Berg, J., Wadhams, L. J., and Woodcock, C. M. (2000). Exploiting chemical ecology and species diversity: stem borer and striga control for maize and sorghum in Africa. Pest Manag. Sci. 56, 957–962. doi: 10.1002/1526-4998(200011)56:11<957::AID-PS236>3.0.CO;2-T
Kifuko-Koech, M., Pypers, P., Okalebo, J. R., Othieno, C. O., Khan, Z. R., Pickett, J. A., et al. (2012). The impact of Desmodium spp. and cutting regimes on the agronomic and economic performance of Desmodium-maize intercropping system in western Kenya. Field Crops Res. 137, 97–107. doi: 10.1016/j.fcr.2012.08.007
Kim, D. G., and Isaac, M. E. (2022). Nitrogen dynamics in agroforestry systems. A review. Agron Sustain. Dev. 42. doi: 10.1007/s13593-022-00791-7
Kim, N., Zabaloy, M. C., Guan, K. Y., and Villamil, M. B. (2020). Do cover crops benefit soil microbiome? A meta-analysis of current research. Soil Biol. Biochem. 142, 107701. doi: 10.1016/j.soilbio.2019.107701
King, A. E., and Blesh, J. (2018). Crop rotations for increased soil carbon: perenniality as a guiding principle. Ecol. Appl. 28, 249–261. doi: 10.1002/eap.1648
Kleber, M., Eusterhues, K., Keiluweit, M., Mikutta, C., Mikutta, R., and Nico, P. S. (2015). Mineral-organic associations: formation, properties, and relevance in soil environments. Adv. Agron. 130, 1–140. doi: 10.1016/bs.agron.2014.10.005
Kolinjivadi, V., Grant, A., Adamowski, J., and Kosoy, N. (2015). Juggling multiple dimensions in a complex socio-ecosystem: the issue of targeting in payments for ecosystem services. Geoforum 58, 1–13. doi: 10.1016/j.geoforum.2014.10.004
Koskey, G., Mburu, S. W., Awino, R., Njeru, E. M., and Maingi, J. M. (2021). Potential use of beneficial microorganisms for soil amelioration, phytopathogen biocontrol, and sustainable crop production in smallholder agroecosystems. Front. Sustain. Food Syst. 5. doi: 10.3389/fsufs.2021.606308
Kosoy, N., and Corbera, E. (2010). Payments for ecosystem services as commodity fetishism. Ecol. Econ. 69, 1228–1236. doi: 10.1016/j.ecolecon.2009.11.002
Kuyah, S., Weldesemayat Sileshi, G., Nkurunziza, L., Chirinda, N., Ndayisaba, P. C., Dimobe, K., et al. (2021). Innovative agronomic practices for sustainable intensification in sub-Saharan Africa. A review. Agron Sustain. Dev. 41. doi: 10.1007/s13593-021-00673-4
Kuzyakov, Y. (2010). Priming effects: Interactions between living and dead organic matter. Soil Biol. Biochem. 42, 1363–1371. doi: 10.1016/j.soilbio.2010.04.003
Kuzyakov, Y., and Xu, X. (2013). Competition between roots and microorganisms for nitrogen: mechanisms and ecological relevance. New Phytol. 198, 656–669. doi: 10.1111/nph.12235
Ladha, J. K., Reddy, C. K., Padre, A. T., and van Kesse, C. (2011). Role of nitrogen fertilization in sustaining organic matter in cultivated soils. J. Environ. Qual. 40, 1756–1766. doi: 10.2134/jeq2011.0064
Lavallee, J. M., Conant, R. T., Paul, E. A., and Cotrufo, M. F. (2018). Incorporation of shoot versus root-derived C-13 and N-15 into mineral-associated organic matter fractions: results of a soil slurry incubation with dual-labelled plant material. Biogeochemistry 137, 379–393. doi: 10.1007/s10533-018-0428-z
Lavallee, J. M., Soong, J. L., and Cotrufo, M. F. (2020). Conceptualizing soil organic matter into particulate and mineral-associated forms to address global change in the 21st century. Glob. Chang. Biol. 26, 261–273. doi: 10.1111/gcb.14859
Lehmann, J., and Kleber, M. (2015). The contentious nature of soil organic matter. Nature 528, 60–68. doi: 10.1038/nature16069
Li, X., Rui, J., Xiong, J., Li, J., He, Z., Zhou, J., et al. (2014). Functional potential of soil microbial communities in the maize rhizosphere. PLoS ONE 9, e112609. doi: 10.1371/journal.pone.0112609
Liang, C., Amelung, W., Lehmann, J., and Kastner, M. (2019). Quantitative assessment of microbial necromass contribution to soil organic matter. Glob. Chang. Biol. 25, 3578–3590. doi: 10.1111/gcb.14781
Liang, C., Kastner, M., and Joergensen, R. G. (2020). Microbial necromass on the rise: the growing focus on its role in soil organic matter development. Soil Biol. Biochem. 150, 108000. doi: 10.1016/j.soilbio.2020.108000
Liang, C., Schimel, J. P., and Jastrow, J. D. (2017). The importance of anabolism in microbial control over soil carbon storage. Nat. Microbiol. 2. doi: 10.1038/nmicrobiol.2017.105
Liebman, M., and Gallandt, E. (1997). “Many little hammers: ecological management of crop-weed interactions,” in Ecology and Agriculture, ed L. E. Jackson (Cambridge, MA: Academic Press).
Lin, B. B. (2011). Resilience in agriculture through crop diversification: adaptive management for environmental change. Bioscience 61, 183–193. doi: 10.1525/bio.2011.61.3.4
Lindahl, J. F., Mutua, F., and Grace, D. (2020). Evaluating farm-level livestock interventions in low-income countries: a scoping review of what works, how, and why. Animal Health Res. Rev. 21, 108–121. doi: 10.1017/S1466252320000146
Liu, J., Dietz, T., Carpenter, S., Folke, C., Alberti, M., Redman, C., et al. (2007). Coupled human and natural systems. Ambio 36, 639–649. doi: 10.1579/0044-7447(2007)36(639:CHANS)2.0.CO;2
Liu, R., Thomas, B., Shi, X. J., Zhang, X. L., Wang, Z. C., and Zhang, Y. T. (2021). Effects of ground cover management on improving water and soil conservation in tree crop systems: a meta-analysis. Catena 199, 105085. doi: 10.1016/j.catena.2020.105085
Luo, G. W., Li, L., Friman, V. P., Guo, J. J., Guo, S. W., Shen, Q. R., et al. (2018). Organic amendments increase crop yields by improving microbe-mediated soil functioning of agroecosystems: a meta-analysis. Soil Biol. Biochem. 124, 105–115. doi: 10.1016/j.soilbio.2018.06.002
Malik, A. A., Martiny, J. B. H., Brodie, E. L., Martiny, A. C., Treseder, K. K., and Allison, S. D. (2020). Defining trait-based microbial strategies with consequences for soil carbon cycling under climate change. Isme. J. 14, 1–9. doi: 10.1038/s41396-019-0510-0
Masters, E. T. (2021). Traditional food plants of the upper Aswa River catchment of northern Uganda-a cultural crossroads. J. Ethnobiol. Ethnomed. 17. doi: 10.1186/s13002-021-00441-4
McDaniel, M. D., Tiemann, L. K., and Grandy, A. S. (2014). Does agricultural crop diversity enhance soil microbial biomass and organic matter dynamics? A meta-analysis. Ecol. Appl. 24, 560–570. doi: 10.1890/13-0616.1
Mhango, W. G., Snapp, S. S., and Phiri, G. Y. K. (2013). Opportunities and constraints to legume diversification for sustainable maize production on smallholder farms in Malawi. Renew. Agric. Food Syst. 28, 234–244. doi: 10.1017/S1742170512000178
Midega, C. A. O., Bruce, T. J. A., Pickett, J. A., Pittchar, J. O., Murage, A., and Khan, Z. R. (2015). Climate-adapted companion cropping increases agricultural productivity in East Africa. Field Crops Res. 180, 118–125. doi: 10.1016/j.fcr.2015.05.022
Mitchell, S., Gelman, A., Ross, R., Chen, J., Bari, S., Huynh, U. K., et al. (2018). The millennium villages project: a retrospective, observational, endline evaluation. Lancet Global Health 6, E500–E513. doi: 10.1016/S2214-109X(18)30065-2
Murage, A. W., Midega, C. A. O., Pittchar, J. O., Pickett, J. A., and Khan, Z. R. (2015). Determinants of adoption of climate-smart push-pull technology for enhanced food security through integrated pest management in eastern Africa. Food Secur. 7, 709–724. doi: 10.1007/s12571-015-0454-9
Mwafulirwa, L., Baggs, E. M., Russell, J., Hackett, C. A., Morley, N., Canto, C. D., et al. (2021). Identification of barley genetic regions influencing plant-microbe interactions and carbon cycling in soil. Plant Soil 468, 165–182. doi: 10.1007/s11104-021-05113-6
Nayak, D., Saetnan, E., Cheng, K., Wang, W., Koslowski, F., Cheng, Y. F., et al. (2015). Management opportunities to mitigate greenhouse gas emissions from Chinese agriculture. Agric. Ecosyst. Environ. 209, 108–124. doi: 10.1016/j.agee.2015.04.035
Ndoli, A., Baudron, F., Schut, A. G. T., Mukuralinda, A., and Giller, K. E. (2017). Disentangling the positive and negative effects of trees on maize performance in smallholdings of Northern Rwanda. Field Crops Res. 213, 1–11. doi: 10.1016/j.fcr.2017.07.020
Ndung'u, M., Ngatia, L. W., Onwonga, R. N., Mucheru-Muna, M. W., Fu, R., Moriasi, D. N., et al. (2021). The influence of organic and inorganic nutrient inputs on soil organic carbon functional groups content and maize yields. Heliyon 7, e07881. doi: 10.1016/j.heliyon.2021.e07881
Nezomba, H., Mtambanengwe, F., Tittonell, P., and Mapfumo, P. (2015). Point of no return? Rehabilitating degraded soils for increased crop productivity on smallholder farms in eastern Zimbabwe. Geoderma 239, 143–155. doi: 10.1016/j.geoderma.2014.10.006
Occelli, M., Mantino, A., Ragaglini, G., Dell'Acqua, M., Fadda, C., Pe, M. E., et al. (2021). Traditional knowledge affects soil management ability of smallholder farmers in marginal areas. Agron. Sustain. Dev. 41. doi: 10.1007/s13593-020-00664-x
Osipitan, O. A., Dille, A., Assefa, Y., Radicetti, E., Ayeni, A., and Knezevic, S. Z. (2019). Impact of cover crop management on level of weed suppression: a meta-analysis. Crop Sci. 59, 833–842. doi: 10.2135/cropsci2018.09.0589
Panke-Buisse, K., Poole, A. C., Goodrich, J. K., Ley, R. E., and Kao-Kniffin, J. (2015). Selection on soil microbiomes reveals reproducible impacts on plant function. Isme. J. 9, 980–989. doi: 10.1038/ismej.2014.196
Paul, B. K., Koge, J., Maass, B. L., Notenbaert, A., Peters, M., Groot, J. C. J., et al. (2020). Tropical forage technologies can deliver multiple benefits in Sub-Saharan Africa. A meta-analysis. Agron. Sustain. Dev. 40. doi: 10.1007/s13593-020-00626-3
Peiffer, J. A., Spor, A., Koren, O., Jin, Z., Tringe, S. G., Dangl, J. L., et al. (2013). Diversity and heritability of the maize rhizosphere microbiome under field conditions. Proc. Natl. Acad. Sci. USA. 110, 6548–6553. doi: 10.1073/pnas.1302837110
Perez-Jaramillo, J. E., Mendes, R., and Raaijmakers, J. M. (2016). Impact of plant domestication on rhizosphere microbiome assembly and functions. Plant Mol. Biol. 90, 635–644. doi: 10.1007/s11103-015-0337-7
Petersen-Rockney, M., Baur, P., Guzman, A., Bender, S. F., Calo, A., Castillo, F., et al. (2021). Narrow and brittle or broad and nimble? comparing adaptive capacity in simplifying and diversifying farming systems. Front. Sustain. Food Syst. 5. doi: 10.3389/fsufs.2021.564900
Peterson, C. A., Deiss, L., and Gaudin, A. C. M. (2020). Commercial integrated crop-livestock systems achieve comparable crop yields to specialized production systems: a meta-analysis. PLoS ONE. 15, e0231840. doi: 10.1371/journal.pone.0231840
Philippot, L., Hallin, S., Borjesson, G., and Baggs, E. M. (2009). Biochemical cycling in the rhizosphere having an impact on global change. Plant Soil. 321, 61–81. doi: 10.1007/s11104-008-9796-9
Philippot, L., Raaijmakers, J. M., Lemanceau, P., and van der Putten, H. W. (2013). Going back to the roots: the microbial ecology of the rhizosphere. Nat. Rev. Micro. 11, 789–799. doi: 10.1038/nrmicro3109
Poirier, V., Roumet, C., and Munson, A. D. (2018). The root of the matter: Linking root traits and soil organic matter stabilization processes. Soil Biol. Biochem. 120, 246–259. doi: 10.1016/j.soilbio.2018.02.016
Ponisio, L. C., M'Gonigle, L. K., Mace, K. C., Palomino, J., de Valpine, P., and Kremen, C. (2015). Diversification practices reduce organic to conventional yield gap. Proc. Biol. Sci. 282:20141396. doi: 10.1098/rspb.2014.1396
Potapov, A. M. (2022). Multifunctionality of belowground food webs: resource, size and spatial energy channels. Biol. Rev. 97, 1691–1711. doi: 10.1111/brv.12857
Pretty, J. N., Noble, A. D., Bossio, D., Dixon, J., Hine, R. E., de Vries, F., et al. (2006). Resource-conserving agriculture increases yields in developing countries. Environ. Sci. Technol. 40, 1114–1119. doi: 10.1021/es051670d
Puget, P., and Drinkwater, L. E. (2001). Short-term dynamics of root- and shoot-derived carbon from a leguminous green manure. Soil Sci. Soc. Am. J. 65, 771–779. doi: 10.2136/sssaj2001.653771x
Reich, J., Paul, S. S., and Snapp, S. S. (2021). Highly variable performance of sustainable intensification on smallholder farms: a systematic review. Glob. Food Sec. Agric. Policy Econ. Environ. 30, 100553. doi: 10.1016/j.gfs.2021.100553
Renard, D., and Tilman, D. (2019). National food production stabilized by crop diversity. Nature 571, 257–260. doi: 10.1038/s41586-019-1316-y
Reynolds, H. L., Packer, A., Bever, J. D., and Clay, K. (2003). Grassroots ecology: plant-microbe-soil interactions as drivers of plant community structure and dynamics. Ecology 84, 2281–2291. doi: 10.1890/02-0298
Roller, B. R. K., Stoddard, S. F., and Schmidt, T. M. (2016). Exploiting rRNA operon copy number to investigate bacterial reproductive strategies. Nat. Microbiol. 1. doi: 10.1038/nmicrobiol.2016.160
Rosa-Schleich, J., Loos, J., Musshoff, O., and Tscharntke, T. (2019). Ecological-economic trade-offs of diversified farming systems - a review. Ecol. Econ. 160, 251–263. doi: 10.1016/j.ecolecon.2019.03.002
Rui, Y. C., Jackson, R. D., Cotrufo, M. F., Sanford, G. R., Spiesman, B. J., Deiss, L., et al. (2022). Persistent soil carbon enhanced in Mollisols by well- managed grasslands but not annual grain or dairy forage cropping systems. Proc. Natl. Acad. Sci. USA. 119. doi: 10.1073/pnas.2118931119
Schmidt, M. W. I., Torn, M. S., Abiven, S., Dittmar, T., Guggenberger, G., Janssens, I. A., et al. (2011). Persistence of soil organic matter as an ecosystem property. Nature 478, 49–56. doi: 10.1038/nature10386
Shackelford, G. E., Kelsey, R., and Dicks, L. V. (2019). Effects of cover crops on multiple ecosystem services: ten meta-analyses of data from arable farmland in California and the Mediterranean. Land Use Policy 88, 104204. doi: 10.1016/j.landusepol.2019.104204
Sida, T. S., Baudron, F., Ndoli, A., Tirfessa, D., and Giller, K. E. (2020). Should fertilizer recommendations be adapted to parkland agroforestry systems? Case studies from Ethiopia and Rwanda. Plant Soil 453, 173–188. doi: 10.1007/s11104-019-04271-y
Sivakumar, C., and Solaimalai, A. (2003). Weed management in rice-fish-azolla farming system - a review. Agric. Rev. 24, 190–196.
Six, J., Frey, S. D., Thiet, R. K., and Batten, K. M. (2006). Bacterial and fungal contributions to carbon sequestration in agroecosystems. Soil Sci. Soc. Am. J. 70, 555–569. doi: 10.2136/sssaj2004.0347
Snapp, S., Kerr, R. B., Smith, A., Ollenburger, M., Mhango, W., Shumba, L., et al. (2013). Modeling and participatory farmer-led approaches to food security in a changing world: A case study from Malawi. Secheresse 24, 350–358. doi: 10.1684/sec.2014.0409
Snapp, S., Roge, P., Okori, P., Chikowo, R., Peter, B., and Messina, J. (2019). Perennial grains for Africa: possibility or pipedream? Exp. Agric. 55, 251–272. doi: 10.1017/S0014479718000066
Snapp, S. S., Blackie, M. J., Gilbert, R. A., Bezner-Kerr, R., and Kanyama-Phiri, G. Y. (2010). Biodiversity can support a greener revolution in Africa. Proc. Natl. Acad. Sci. USA. 107, 20840–20845. doi: 10.1073/pnas.1007199107
Sokol, N. W., Kuebbing, S. E., Karlsen-Ayala, E., and Bradford, M. A. (2019a). Evidence for the primacy of living root inputs, not root or shoot litter, in forming soil organic carbon. New Phytol. 221, 233–246. doi: 10.1111/nph.15361
Sokol, N. W., Sanderman, J., and Bradford, M. A. (2019b). Pathways of mineral-associated soil organic matter formation: Integrating the role of plant carbon source, chemistry, and point of entry. Glob. Chang. Biol. 25, 12–24. doi: 10.1111/gcb.14482
Sokol, N. W., Slessarev, E., Marschmann, G. L., Nicolas, A., Blazewicz, S. J., Brodie, E. L., et al. (2022). Life and death in the soil microbiome: how ecological processes influence biogeochemistry. Nat. Rev. Microbiol. 20, 415–430. doi: 10.1038/s41579-022-00695-z
Spaccini, R., Mbagwu, J. S. C., Igwe, C. A., Conte, P., and Piccolo, A. (2004). Carbohydrates and aggregation in lowland soils of Nigeria as influenced by organic inputs. Soil Tillage Res. 75, 161–172. doi: 10.1016/S0167-1987(03)00158-2
Stoate, C., and Jarju, A. K. (2008). A participatory investigation into multifunctional benefits of indigenous trees in West African savanna farmland. Int. J. Agric. Sustain. 6, 122–132. doi: 10.3763/ijas.2008.0299
Tamagno, S., Eagle, A. J., McLellan, E. L., van Kessel, C., Linquist, B. A., Ladha, J. K., et al. (2022). Quantifying N leaching losses as a function of N balance: a path to sustainable food supply chains. Agric. Ecosyst. Environ. 324, 107714. doi: 10.1016/j.agee.2021.107714
Tamburini, G., Bommarco, R., Wanger, T. C., Kremen, C., M., van der Heijden, G. A., et al. (2020). Agricultural diversification promotes multiple ecosystem services without compromising yield. Sci. Adv. 6, 45. doi: 10.1126/sciadv.aba1715
Tang, X. Y., Zhang, C. C., Yu, Y., Shen, J. B., van der Werf, W., and Zhang, F. S. (2021). Intercropping legumes and cereals increases phosphorus use efficiency; a meta-analysis. Plant Soil 460, 89–104. doi: 10.1007/s11104-020-04768-x
Tiefenbacher, A., Sanden, T., Haslmayr, H. P., Miloczki, J., Wenzel, W., and Spiegel, H. (2021). Optimizing carbon sequestration in croplands: a synthesis. Agronomy 11, 882. doi: 10.3390/agronomy11050882
Tieszen, L. L., Tappan, G. G., and Toure, A. (2004). Sequestration of carbon in soil organic matter in senegal: an overview. J. Arid Environ. 59, 409–425. doi: 10.1016/j.jaridenv.2004.04.002
Tittonell, P., and Giller, K. E. (2013). When yield gaps are poverty traps: the paradigm of ecological intensification in African smallholder agriculture. Field Crops Res. 143, 76–90. doi: 10.1016/j.fcr.2012.10.007
Tomasi, N., Weisskopf, L., Renella, G., Landi, L., Pinton, R., Varanini, Z., et al. (2008). Flavonoids of white lupin roots participate in phosphorus mobilization from soil. Soil Biol. Biochem. 40, 1971–1974. doi: 10.1016/j.soilbio.2008.02.017
Tonitto, C., David, M. B., and Drinkwater, L. E. (2006). Replacing bare fallows with cover crops in fertilizer-intensive cropping systems: a meta-analysis of crop yield and N dynamics. Agric. Ecosyst. Environ. 112, 58–72. doi: 10.1016/j.agee.2005.07.003
Tu, X., Snapp, S., and Viens, F. (2022). A Bayesian approach to understand controls on total and labile soil carbon in cultivated soils of Central and Southern Malawi. Geoderma. 413, 115746. doi: 10.1016/j.geoderma.2022.115746
Tully, K., Sullivan, C., Weil, R., and Sanchez, P. (2015). The state of soil degradation in sub-saharan africa: baselines, trajectories, and solutions. Sustainability 7, 6523–6552. doi: 10.3390/su7066523
Tully, K. L., Lawrence, D., and Scanlon, T. M. (2012). More trees less loss: nitrogen leaching losses decrease with increasing biomass in coffee agroforests. Agric. Ecosyst. Environ. 161, 137–144. doi: 10.1016/j.agee.2012.08.002
Turner, T. R., Ramakrishnan, K., Walshaw, J., Heavens, D., Alston, M., Swarbreck, D., et al. (2013). Comparative metatranscriptomics reveals kingdom level changes in the rhizosphere microbiome of plants. ISME J. 7, 2248–2258. doi: 10.1038/ismej.2013.119
Valadares, R. V., Costa, M. D., Neves, J. C. Netto L. J., da Silva, I. R., Moro, E., et al. (2020). Rhizosphere microbiological processes and eucalypt nutrition: synthesis and conceptualization. Sci. Total Environ. 746, 141305. doi: 10.1016/j.scitotenv.2020.141305
Vanek, S. J., and Drinkwater, L. E. (2013). Environmental, social, and management drivers of soil nutrient mass balances in an extensive Andean cropping system. Ecosystems 16, 1517–1535. doi: 10.1007/s10021-013-9699-3
Vanek, S. J., Jones, A. D., and Drinkwater, L. E. (2016). Coupling of soil regeneration, food security, and nutrition outcomes in Andean subsistence agroecosystems. Food Secur. 8, 727–742. doi: 10.1007/s12571-016-0598-2
Vermunt, D. A., Wojtynia, N., Hekkert, M. P., Van Dijk, J., Verburg, R., Verweij, P. A., et al. (2022). Five mechanisms blocking the transition towards 'nature-inclusive' agriculture: a systemic analysis of Dutch dairy farming. Agric. Syst. 195, 103280. doi: 10.1016/j.agsy.2021.103280
Vollmer-Sanders, C., Allman, A., Busdeker, D., Moody, L. B., and Stanley, W. G. (2016). Building partnerships to scale up conservation: 4R nutrient stewardship certification program in the Lake Erie watershed. J. Great Lakes Res. 42, 1395–1402. doi: 10.1016/j.jglr.2016.09.004
von Lutzow, M., Kogel-Knabner, I., Ekschmitt, K., Matzner, E., Guggenberger, G., Marschner, B., et al. (2006). Stabilization of organic matter in temperate soils: mechanisms and their relevance under different soil conditions - a review. Eur. J. Soil Sci. 57, 426–445. doi: 10.1111/j.1365-2389.2006.00809.x
Wander, M. M., Traina, S. J., Stinner, B. R., and Peters, S. E. (1994). Organic and conventional management effects on biologically-active soil organic-matter pools. Soil Sci. Soc. Am. J. 58, 1130–1139. doi: 10.2136/sssaj1994.03615995005800040018x
Weintraub, M. N., Scott-Denton, L. E., Schmidt, S. K., and Monson, R. K. (2007). The effects of tree rhizodeposition on soil exoenzyme activity, dissolved organic carbon, and nutrient availability in a subalpine forest ecosystem. Oecologia 154, 327–338. doi: 10.1007/s00442-007-0804-1
Weisberger, D., Nichols, V., and Liebman, M. (2019). Does diversifying crop rotations suppress weeds? A meta-analysis. PLoS ONE 14, e0219847. doi: 10.1371/journal.pone.0219847
Williams, E. K., Fogel, M. L., Berhe, A. A., and Plante, A. F. (2018). Distinct bioenergetic signatures in particulate versus mineral-associated soil organic matter. Geoderma 330, 107–116. doi: 10.1016/j.geoderma.2018.05.024
Witcomb, A. (2021). Nutrient cycling on smallholder farms in Uganda and Malawi (PhD Dissertation). Michigan State University, East Lansing, MI. Available online at: https://d.lib.msu.edu/etd/50085/datastream/OBJ/View/
Wuest, S. E., Peter, R., and Niklaus, P. A. (2021). Ecological and evolutionary approaches to improving crop variety mixtures. Nat. Ecol. Evol. 5, 1068–1077. doi: 10.1038/s41559-021-01497-x
Yan, M., Pan, G. X., Lavallee, J. M., and Conant, R. T. (2020). Rethinking sources of nitrogen to cereal crops. Glob. Chang. Biol. 26, 191–199. doi: 10.1111/gcb.14908
Yang, G. Y., Ji, H. T., Liu, H. J., Feng, Y. F., Zhang, Y. F., Chen, L. G., et al. (2021). Nitrogen fertilizer reduction in combination with Azolla cover for reducing ammonia volatilization and improving nitrogen use efficiency of rice. Peerj 9, e11077. doi: 10.7717/peerj.11077
Yao, Y. L., Zhang, M., Tian, Y. H., Zhao, M., Zeng, K., Zhang, B. W., et al. (2018). Azolla biofertilizer for improving low nitrogen use efficiency in an intensive rice cropping system. Field Crops Res. 216, 158–164. doi: 10.1016/j.fcr.2017.11.020
Young, M. D., Ros, G. H., and de Vries, W. (2021). Impacts of agronomic measures on crop, soil, and environmental indicators: a review and synthesis of meta-analysis. Agric. Ecosyst. Environ. 319, 107551. doi: 10.1016/j.agee.2021.107551
Yusuf, A. A., Abaidoo, R. C., Iwuafor, E. N. O., Olufajo, O. O., and Sanginga, N. (2009). Rotation effects of grain legumes and fallow on maize yield, microbial biomass and chemical properties of an Alfisol in the Nigerian savanna. Agric. Ecosyst. Environ. 129, 325–331. doi: 10.1016/j.agee.2008.10.007
Keywords: agricultural diversification, soil organic matter, nutrient use efficiency, nutrient cycling, decomposition, agroecology, biological N fixation, soil restoration
Citation: Drinkwater LE and Snapp SS (2022) Advancing the science and practice of ecological nutrient management for smallholder farmers. Front. Sustain. Food Syst. 6:921216. doi: 10.3389/fsufs.2022.921216
Received: 15 April 2022; Accepted: 26 July 2022;
Published: 15 September 2022.
Edited by:
Marney E. Isaac, University of Toronto, CanadaReviewed by:
Krista Jacobsen, University of Kentucky, United StatesCopyright © 2022 Drinkwater and Snapp. This is an open-access article distributed under the terms of the Creative Commons Attribution License (CC BY). The use, distribution or reproduction in other forums is permitted, provided the original author(s) and the copyright owner(s) are credited and that the original publication in this journal is cited, in accordance with accepted academic practice. No use, distribution or reproduction is permitted which does not comply with these terms.
*Correspondence: Laurie E. Drinkwater, bGVkMjRAY29ybmVsbC5lZHU=
Disclaimer: All claims expressed in this article are solely those of the authors and do not necessarily represent those of their affiliated organizations, or those of the publisher, the editors and the reviewers. Any product that may be evaluated in this article or claim that may be made by its manufacturer is not guaranteed or endorsed by the publisher.
Research integrity at Frontiers
Learn more about the work of our research integrity team to safeguard the quality of each article we publish.