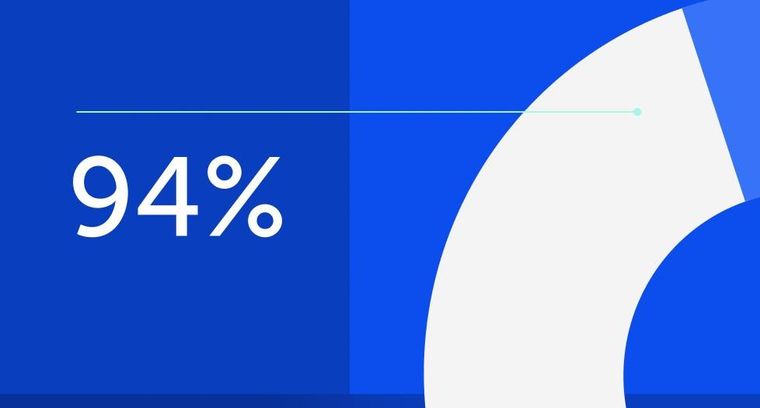
94% of researchers rate our articles as excellent or good
Learn more about the work of our research integrity team to safeguard the quality of each article we publish.
Find out more
ORIGINAL RESEARCH article
Front. Sustain. Food Syst., 29 August 2022
Sec. Sustainable Food Processing
Volume 6 - 2022 | https://doi.org/10.3389/fsufs.2022.918772
This article is part of the Research TopicProduction, Downstreaming, and Utilization of Proteins and Exopolysaccharides from Single Cells in Food MatricesView all 6 articles
Microalgae, and particularly the cyanobacterium Arthrospira platensis (spirulina), have attracted much attention due to their wide range of uses. The potential use of spirulina in food is mainly driven by its high content of macro and micronutrients including proteins, γ-linolenic acid, sulfated polysaccharides, minerals, vitamins, and the natural pigment phycocyanin. Despite these potential benefits, spirulina is still not widely used in the food industry due to numerous technological challenges during manufacturing or specific sensory issues in the final product. This research deals with the feasibility of integrating spirulina into the brewing process to create a tasty beer with high consumer acceptance. In the novel recipes, 5% (w/w) of the malt was replaced by spirulina powder. The first part of the study investigated inclusion at different time points throughout manufacturing of a reference beer style (mild pale ale). Compared to the control, alcoholic fermentation was slightly influenced by cyanobacterial biomass but resulted in a beer with a typical beer-like character. Sensory evaluations including a simple descriptive test, a popularity analysis, and Just-About-Right-Questions, indicated a complex alteration in the sensory properties. This includes a dominant algal taste that disturbs the character of the pale ale beers but also a deep blue color of the beer, if spirulina was included during the wort cooling phase. Based on these results, another set of beers with a higher original extract concentration and increased hop dosages was produced. These beers had a high popularity (6.0 original TESTSCORE; 7.12 and 6.64 optimized TESTSCORE), and also exhibited a deep blue color due to the natural pigment phycocyanin from spirulina. Further, bitterness and algal taste was rated by most of the panelists to be “just right” and the simple descriptive test indicated “sweetness” as important attribute which was not expected for this hoppy beer style.
Microalgae and cyanobacteria are ubiquitous aquatic microorganisms, that grow in lakes, oceans, and even wastewater. They can cope with unfavorable environmental conditions such as high temperatures, high light intensities, or nutrient depletion (Khan et al., 2018). The resultant stress response usually leads to a concerted alteration of the algal cell physiology and biomass composition, which is often intended in biotechnological applications, such as creation of renewable energy, sustainable food, and bioactive ingredients (Sandmann et al., 2018, 2021; Arun et al., 2020; Levasseur et al., 2020). The worldwide phototrophic production of microalgal biomass in 2021 was around 50,000 tons, with demand increasing (Verdelho et al., 2022). A broad overview of the algae production industry in Europe was recently presented by Araújo et al. (2021). The cyanobacterium Arthrospira platensis (commercially known as spirulina) is consumed and processed as human food. With up to 70% protein based on dry weight, it is known as valuable source of microbial protein and also includes minerals, γ-linolenic acid, and vitamins (Niccolai et al., 2020). Spirulina is classified by the FDA as GRAS [Generally Recognized As Safe (FDA, 2018)] and is authorized as a food for the European Union market (Niccolai et al., 2019). Additionally, cyanobacteria produce different biologically active substances. Spirulina is known to synthetize a complex sulfated polysaccharide, for example, that exhibits antiviral activity against enveloped viruses (Lee et al., 2001, 2007; Rechter et al., 2006). Another industrial application of spirulina biomass is derived from its intrinsic light harvesting protein complexes including the protein phycocyanin. Extracted phycocyanin exhibits a brilliant blue color and can be used as a fluorescent label in biomedical research or a natural food dye, for example (Eriksen, 2008; Manirafasha et al., 2016). Phycocyanin also exhibits a high antioxidant capacity, which seems to be beneficial in oxidative stress-induced diseases and inflammatory processes. These are major reasons for its common use as a nutraceutical (Fernández-Rojas et al., 2014; Chentir et al., 2018). Spirulina biomass is also increasingly incorporated as a food ingredient in some products. This includes bakery products (Batista et al., 2019), gluten-free pasta (Fradinho et al., 2020), soft drinks, yogurt, and candies (Christaki et al., 2011; Niccolai et al., 2019). Different authors have investigated the effect of incorporated spirulina biomass on the fermentation process based on lactic acid bacteria and the storage behavior of the resultant products. Examples include yogurt, cheese, fermented milk, and soybean drinks (Mazinani et al., 2016; Yamaguchi et al., 2019; Martelli et al., 2020b; Niccolai et al., 2020). Despite the clear potential of including spirulina as a food ingredient to generate new products with higher nutritional quality for athletes and the elderly (Lafarga, 2019), different obstacles can arise. First, the level of spirulina included in most of the products on the market is very low, ranging typically from 0.25 to 2% (Barkallah et al., 2017; Lafarga, 2019; Francezon et al., 2021). One major reason for this is a complex change in the sensory properties of common products after inclusion of spirulina. This may include major changes in color and odor which ultimately results in low consumer acceptance (Lafarga, 2019; Francezon et al., 2021). Other unfavorable factors may also develop, such as an increased sensitivity of the product to oxygen or a need to modify manufacturing technologies (Lafarga, 2019; Francezon et al., 2021). Microalgae and spirulina are also known to contain compounds with antimicrobial properties (El-Sheekh et al., 2014; Martelli et al., 2020a; Hensel et al., 2021; Tavakoli et al., 2021). This potentially interferes with typical processing steps in food technology that are based on fermentation and thus intended microbial growth processes (Ozdemir et al., 2004; Abedin and Taha, 2008; Niccolai et al., 2020).
One of these industrially important fermentation-driven processes is the brewing of beer. The complex process typically begins with barley seeds being malted using a process that involves controlled partial germination (Stewart, 2013). Malting facilitates the de novo synthesis and activation of intrinsic enzymes in the barley seeds needed for enzymatic digestion of storage compounds during germination. The malt is then milled and mixed with water in a process called mashing. Mashing can be described as enzymatic digestion of high-molecular weight storage compounds from the malt to generate soluble sugars, amino acids, and short peptides that act as resources for yeast growth (Stewart, 2013; Kerr et al., 2021). A typical mashing process consist of a defined temperature profile usually with several temperature stages (Kerr et al., 2021). The product of mashing is called wort, which corresponds to the soluble fraction of the mash. Wort is finally boiled with hops to introduce aromatic flavors and bitterness, stop enzymatic activity, and for sterilization (Barth, 2013; Stewart, 2013). Fermentation is then started by introducing yeast into the cooled wort (Figure 1). Yeast cells then consume sugars, peptides, and amino acids, while producing carbon dioxide, ethanol, and flavor compounds such as higher alcohols and esters.
Figure 1. Process scheme. (A) Addition of spirulina biomass in the reference recipe (mild pale ale). (B) Further product optimization within India pale ale brew style.
The aim of this study was to investigate the suitability of A. platensis biomass as a substrate for yeast-driven fermentation during the process of beer brewing to create a beer with high consumer acceptance. This includes identification of possible adverse effects that might alter the fermentation process itself or the product properties of the final beer. Analyses based on physicochemical characterization and sensory evaluations of the brewed beers were performed. Phycocyanin was used as a lead substance to monitor possible influences of the microbial biomass on beer color. To the best of our knowledge, no work has been published to date investigating the possible influences of spirulina biomass on the beer brewing process, including any necessary technological optimization to manage altered sensory properties of the final product. This also holds true for the three commercial algal beers that were identified (Table 1).
Six different top-fermented beers were brewed. The first four beers (brew 1 to brew 4) were based on a mild pale ale (intended original extract: 12°P, bitterness: 33 IBU). Brew 1 represents the control, with no spirulina biomass. The recipe for the control consists of 20 L main cast with 4.74 kg “Pilsner malt” (Weyermann GmbH & Co. KG, Bamberg, Germany). Three different hops varieties (type 90 pellets) were used and dosed at different time points during boiling: “Hallertau Blanc” (α-acids: 8.8%, 20 g at the start of boiling), “Amarillo” (α-acids: 9.4%, 10 g after 35 min of boiling), and “Relax” (α-acids: 0.8%, 8 g after 50 min of boiling). The type of hops in brews 1–4 have been chosen to create a slightly aromatic mild pale ale as starting point for the evaluation of the influence of spirulina. All hops were purchased from the online shop Hopfen der Welt (Ellingen, Germany). In brews 2–4, 5% (w/w) of the malt was replaced by spirulina powder (organic spirulina powder, MySuperfoods, Kent, England). The recipe thus consists of 20 L main cast with 4.503 kg “Pilsner malt” and 0.237 kg spirulina powder. Spirulina powder was added at different times in the brewing process (Figure 1): together with the malt at the start of mashing (brew 2), with the first hops addition (brew 3), and during wort cooling at <40°C (brew 4). This allows the influence of the algae powder to be examined in all process steps and the optimal addition time to be determined.
Based on the findings from the first four experiments, brews 5 and 6 were produced differently. These two brews corresponded to the characteristics of an India pale ale (IPA, intended original extract: 13°P, bitterness: 60 IBU). This was intended to determine whether a more bitter and hop-accentuated beer influences the dominant algal taste. The same sort and quantity of malt was used for both (for 20 L main cast: 5.13 kg “Pilsner malt”) while the varieties and quantities of hops differed. The time of additions was analogous to the first four beers: brew 5—“El Dorado” (α-acids: 13.3%, 22 g at the start of boiling), “Hallertauer Comet” (α-acids: 9.5%, 21 g after 35 min of boiling, 10 g after 50 min of boiling); brew 6 “Magnum” (α-acids: 12.7%, 29 g at the start of boiling), “Tradition” (α-acids: 5.4%, 21 g after 35 min of boiling, 10 g after 50 min of boiling). Additionally, whirlpool hopping was performed with both brews: brew 5 (“Mistral”, α-acids: 5.1%, 25 g, and “Hüll Melon” α-acids: 6.8%, 25 g,) to develop a highly fruity flavor and brew 6 (“Relax” α-acids: 0.8%, 50 g) to develop an earthy-spicy flavor in the beers. For brews 5 and 6, spirulina was dosed during wort cooling identical to brew 4.
The same top-fermenting yeast “Safale US-05” (Fermentis, Marcq-en-Barœul, France) was used in all beers (0.575 g (dry weight)/L wort).
An overview about raw materials utilized to produce the beers is given in Supplementary Table S2.
A general overview of the processing steps, including mashing, boiling, cooling, and the addition of spirulina biomass, is shown in Figure 1. Apart from the addition of algae, all beers were produced in the same way using the same micro brewing plant BRAXONIA (Rosoma GmbH, Rostock, Germany). Ground malt was mixed with distilled water in the mash tun and heated to 50°C (Figure 1). In case of brew 2, ground malt (4.503 kg), spirulina powder (0.237 kg) and 20 L distilled water was used. A traditional three rest mashing scheme was used (Kühbeck et al., 2005; Back and Narziß, 2009). The first rest was at 50°C for 10 min, second started at 62°C for 45 min, and third at 72°C for 20 min. For lautering in the lauter tun, the mash temperature was raised to 78°C beforehand. After the first lautering, recast water (normal tap water with a hardness of 17°dH) was added to reach a volume of 23 L. 0.5 L was taken from the first wort, autoclaved at 121°C, and used to rehydrate dry yeast after cooling to pitch temperature. Wort was then boiled for 60 min. Hops (plus spirulina powder for brew 3) were added according to the recipes section Recipes (see also Supplementary Table S2). The whirlpool process was then performed. For the brewed pale ale beers, post-isomerization time was determined as time to transfer into the whirlpool plus the stand time within the whirlpool (Table 2). The IPAs were brewed utilizing whirlpool hopping (Kaltner, 2000), in these cases wort was cooled until the temperature reached to 80°C, then additional hops were added (see section Recipes) and whirlpool was started afterwards. For the IPAs, post-isomerization time is decreased because it's just the timespan between end of boiling until cooling to 80°C.
During cooling of the wort to pitch temperature (20°C), the original extract was adjusted to 12°P (brews 1–4) or 13°P (brews 5–6) by dosing demineralized water. For brews 4–6, spirulina powder was added to wort below 40°C.
Cornelius style fermentation tanks (19 L volume, Thielmann Ucon GmbH, Hausach, Germany), equipped with spunging valves (KOMET, Barby + Kühner GmbH, Untersiemau, Germany) were used for fermentation. Yeast (Safale US-05) was added to the wort at a pitch temperature of 20°C and an aliquot (250 mL) was taken for lab-scale fermentation done in parallel to the actual fermentation (Thesseling et al., 2019). This lab-scale fermentation was done during first seven days of fermentation at 18°C. This avoids drawing of samples from the 19 L fermentation tank and supported microbial safety within the tank. After transfer to the fermentation tank, yeasts were sparged with sterile compressed air for 10 min and then fermentation was carried out for 7 days at 18°C. After 7 days, the green beer was filled into a new 19 L tank with removal of sedimented trub for post-fermentation and stored at the same temperature. After seven more days, the beer was again transferred into new tanks with a further removal of sedimented trub and the beer was matured for additional 4 weeks at 4°C. The whole procedure, including fermentation and maturation, took 42 days.
All chemicals used were supplied by Carl Roth GmbH (Karlsruhe, Germany) and by Merck KGaA (Darmstadt, Germany). pH value was determined with a WTW 330i pH meter (Weilheim, Germany) from wort and final beer. Original extract was determined with a beer wort spindle (MHV Brauhaushop, Borstendorf, Germany) also from wort and final beer. Free amino nitrogen (FAN) was quantified with the ninhydrin method B-400.11.111 (MEBAK, 2020a). First, a color reagent consisting of disodium hydrophosphate dodecahydrate (100 g/L), potassium dihydrogen phosphate (60 g/L), ninhydrin (5 g/L) and D(-)-Fructose (3 g/L) was prepared. For this, all solid materials were dissolved in bi-distilled water and pH-value of this solution was set between 6.6 and 6.8 with sodium hydroxide (4 M). Second, a solution of potassium iodate (2 g), dissolved in bi-distilled water (600 mL) and 96 % (v/v) Ethanol (400 mL) was prepared. For the analysis, 2 mL of the samples (recommendation: wort 1:100 dilution; beer 1:50 dilution with bi-distilled water) was placed in a 10 mL test tube, mixed with 1 mL of color reagent solution, and heated for 16 min at 100°C. The tubes were then cooled in a water bath at 20°C for 20 min and finally 5 mL of the potassium iodate solution was added to the mixture. Free amino nitrogen was determined with a UV/VIS spectral photometer ULC 1703008 (VWR International, Leuven, Belgium) at 570 nm after 30 min. Glycine (1.072 g/L) was dissolved in bi-distilled water. One milliliters of this solution was taken and filled up to 100 ml with bi-distilled water to create the calibration substance (2 mg amino nitrogen/L). Total sugar was estimated using a reducing-end method based on a reaction with dinitrosalicylic acid (DNSA) (Miller, 1959): 3,5-Dinitrosalicylic acid (10 g/L), sodium hydroxide (10 g/L), sodium sulfite (0.5 g/L), and potassium sodium tartrate (300 g/L) were dissolved together in bi-distilled water and then filtered to create the final reagent solution. For the analysis, 1 mL of the samples (recommendation: wort 1:100 dilution; beer 1:50 dilution with bi-distilled water) was placed in a 20 mL test tube, mixed with 2 mL of reagent solution, and heated for 15 min at 100°C. The tubes were then cooled in a water bath at 20°C for 20 min and finally 17 mL bi-distilled water was added to the mixture. The sugar content was determined with a UV/VIS spectral photometer ULC 1703008 (VWR International, Leuven, Belgium) at 505 nm. D(+)-Glucose was used as calibration substance (1 g/L). Both analytics were performed from wort and different samples along the fermentation process (Figure 2). Alcohol content was determined in the final beers with distillation and pycnometric determination using methods B-590.07.902 and B-590.10.024 (MEBAK, 2020b,c). After degassing and setting the pH-value to 7.0 with sodium hydroxide (2 M), 100 mL of the prepared sample was transferred to the distillation apparatus and slowly heated to 90°C. Prior, 2–3 boiling stones and a few drops of silicone-containing defoamer were added. The evaporated alcohol flowed into a 100 mL volumetric flask. The volumetric flask was then filled up with bi-distilled water, tempered to 20.0°C and the liquid transferred to an empty pycnometer. After further incubation for 5 min at 20.0°C, the mass of the filled pycnometer was determined, and the “dip weight ratio” (d20/20) was calculated according to equation (1):
m1 = mass of the empty pycnometer (g)
m2 = mass of the water-filled pycnometer at 20.0°C (g)
m3 = mass of the sample-filled pycnometer at 20.0°C (g)
Figure 2. Development of main yeast substrates during fermentation until final beer. Total sugar content (A) and free amino nitrogen (B). Mean and standard deviation shown for wort and final beer (n = 3).
With d20/20 the alcohol content can be determined based on the table collection of Rauscher et al. (1986). Color of the final beers was characterized using a Color Flex EZ spectral photometer (HunterLAB FMS Jansen GmbH & Co. KG, Murnau, Germany) using the -L*a*b*- color system. To determine the phycocyanin content, the final beer was degassed in an ultrasonic bath, centrifuged at 1,900 g-force for 10 min at room temperature, the liquid 10-times diluted with bi-distilled water, filtered and finally the extinction at the wavelengths of 620, 650, and 800 nm was determined with an ULC 1703008 UV/VIS spectral photometer (VWR International, Leuven, Belgium). The total phycocyanin content calculated using equation (2) modified from Böcker et al. (2019) whereas the original method was established from Yoshikawa and Belay (2008). The extinction values of the two wavelengths were corrected by the value at 800 nm to minimize disturbances caused by the turbidity in the beer samples:
herein tPC is the total phycocyanin content (g/L), A620 is the extinction at 620 nm; A650 is the extinction at 650 nm, and A800 is the extinction at 800 nm.
The statistical analysis was conducted with XLSTAT (version 2020.1.1.64431). To examine the statistical significance a one-way analysis of variance (ANOVA) followed by a post-hoc test (Tukey) was implemented (α = 0.050). This statistical analysis was used to check the significant differences of reducing sugar, FAN, alcohol-, phycocyanin content, and sensory results of the popularity test and the Just-About-Right-Questions against the reference brew. Brew 1 was the reference for brews 2–4, and brew 4 was the reference for brews 5 and 6.
To determine significant differences between the L*a*b* color values, ΔE* was used and calculated following Equation (3) (Gierling, 2006):
ΔE* is a measure of the perceived color distance between two products in the color space. A ΔE* value of larger than 5 means that the two colors are different.
During sensory evaluation, all six beers were tasted across two tastings (brews 1–4 and brews 5–6) with partially different panelists due to the different production times of the beers. The samples were prepared, and the tasting was implemented according to Busch-Stockfisch (2014). The sensory laboratory was equipped with 12 cabins according to the standard (DIN 10962:1997-10, 1997) under standardized conditions, including fixed room temperature (20 ± 0.3°C), a relative humidity between 40 and 50%, and the drinking temperature of the beers of 7°C. This also includes sample preparation, and sample presentation like e.g., giving the panelists the opportunity for “neutralization” with still mineral water and crisp bread (Busch-Stockfisch, 2014). Samples were tasted one by one without retasting to measure the first impression. The tasting panel was able to see the color of the beers. The size of the panel was 19 for brews 1–4, and 25 for brews 4–6.
First, a popularity test was carried out using a hedonic nine-point scale, with nine symbolizing the highest (likes hugely) and one the worst (displeased hugely) grading. The scale is categorized into two ranges: 9–6 (like) and 4–1 (dislike), with number 5 (neutral) symbolizing the turning point. The evaluation was made with the help of the group protocol by Fliedner and Wilhelmi (1993). Based on this, a beer can be classified as popular if 80% of all panelists have evaluated a beer in the range from 9 to 6.
Second, a simple descriptive test was done in which the panelists have been asked to note the most characteristic attributes (DIN 10964:2014-11, 2014).
Third, an evaluation with the aid of Just-About-Right-Questions (JAR) was carried out. The intensity of pre-chosen characteristics (“algal taste” and “bitterness”) was examined in a five-point-scale with the criteria “much too much,” “little too much,” “just right,” “little too little,” and “much too little.” The intensity of the given product characteristics were examined for all six beers and depicted in a bar chart (Stone et al., 2012).
Over the last few decades, microalgae, and cyanobacteria such as Arthrospira platensis have attracted much attention due to their wide range of uses. This also includes their use as a component of human food. Despite their potential benefits, numerous difficulties such as disturbed sensory properties of the final products may arise. The antimicrobial properties of Arthrospira may also interfere with subsequent microbial fermentation steps intended for final product manufacturing. The aim of this study was to investigate the suitability of A. platensis biomass as a further substrate for yeast-driven fermentation during the process of beer brewing to create a tasty beer with high consumer acceptance. The biomass composition of the spirulina powder is shown in Supplementary Table S1. The major constituents are 64% protein, 9.1% carbohydrate, and 8.2% lipids.
The first four beers (brews 1–4) were brewed to investigate putative influences of spirulina biomass on the subsequent fermentation process and the product properties of the final beer. The beer style was a mild pale ale, with brew 1 acting as the control with no spirulina biomass. Spirulina was added to brews 2–4 to replace 5% (w/w) of the malt. The spirulina was added at different processing steps during mashing, resulting in different thermal stresses for the cyanobacterial constituents (Figure 1). In brew 4 spirulina was added during wort cooling (below 40°C) which correlates to the lowest thermal stress. Whereas, the original extract of the wort was very similar in all four brews, the original extract in the final beer of brew 4 was increased by 25% compared to the control beer (Table 2). The primary parameters of the final beers, the pH value, total sugar content, and FAN, changed depending on when the spirulina was added (Table 2). The pH value increased with later inclusion of spirulina biomass by up to 1 pH unit for brew 4. Compared to the control, the final beer for brew 4 exhibited an increase in the total sugar content and FAN of around 25 and 13%, respectively. A more detailed analysis of time-dependent changes for these major yeast substrates is shown in Figure 2. Except for the first day of fermentation, the general decrease in FAN and sugars was very similar in the four brews. Approximately 90% of total sugars and 60% of FAN were depleted over the course of fermentation in all four beers (Figure 2). These results show that the general yeast-driven fermentation is successful in all four brews but in more detail, a slightly higher FAN and total sugar content is given which is most pronounced in brew 4. This likely results from extracted but unfermentable protein and sugar compounds of the spirulina powder in the beer. This conclusion is based on the increased pH and the increased original extract of the final beers, which relate to the inclusion time of the spirulina biomass. Additionally, the original extract of the final beer of brew 4 was increased by 25%.
In brew 4, the inclusion of spirulina resulted in a blue color of the final beer. This was shown by significant changes in the L*a*b* color scheme (Supplementary Table S3). Component a* shifted from slightly positive values to around −7. Component b* shifted from around 30 to −11, which finally produces a blue color with a slight cyan-blue touch. Quantification of the phycocyanin (PC) content in brew 4 indicated a concentration of 0.69 g PC/L beer, whereas brews 1–3 showed no noticeable PC (Figure 3). The PC content of brews 5 and 6 was similarly high. The minor PC content in the control could be related to chlorophyll-derived components from the hops or may originate from light scattering due to residual particles that are still in suspension. Please note that the method was not optimized for PC quantification in beer. It was established to quantify PC content in spirulina supplements, like multivitamin tablets spiked with spirulina (Yoshikawa and Belay, 2008) which represent also a complex matrix. Nevertheless, observed differences in the PC content are covered by L*a*b* measurements and by photos of the beers (Figure 3B).
Figure 3. Phycocyanin content of the beers. Brews 1–4: mild pale ale style. Brews 5 and 6: India pale ale style. Boxplot and data shown (circles, n = 3) (A). Box plots compile the median and quartiles. In addition, standard deviation was added as error bar (thick line). Asterisk indicates significant difference between brew 4 and brew 1 (reference for brew 4) (α = 0.050). Brews 5 and 6 have not been significantly different from brew 4 (α = 0.050). Photographs of the final beers (B).
For brews 1–4, the control (brew 1) with no algae was rated highest by the panelists (Table 3). Ninety-five percentage of the panelists liked the beer from the control brew 1 (range 9–6) (Table 3). Brews 2–4 shared a significant lower popularity of around six with a higher standard deviation (Table 3). For example, brew 4 was characterized with 63% (like) and 31% (dislike) and 5% neutral. The decision was also justified by the panelists with specific attributes derived from a simple descriptive test. For brews 2–4 more negative attributes were noted, including a unpleasant algal taste (Table 3). In addition, these beers were characterized as being sweeter than the control.
The used Just-About-Right analysis was applied only for the specific characteristics “algal taste” and “bitterness” (Figure 4). Regardless of the popularity of the beer, the panelists should state using a five-point-scale if the characteristic is present “much too much,” “little too much,” “just right,” “little too little,” or “much too little” in the beer. For brews 1–4, JAR analysis with a focus on “algal taste” showed an increase of the algal taste with a later inclusion time for the spirulina. In the control beer, a small proportion of the panelists perceived an algal taste. In contrast, 95% of the panelists perceived the algal taste with at least “just right” up to “much too much” in brew 4. In terms of the intensity of the bitterness, brew 1 was classified primarily with “just right” ratings. For brews 2–4, the heterogeneity in the ratings becomes larger and the perceived bitterness is more pronounced for the panelists with later inclusion times of the spirulina biomass. Regarding “algal taste”, the means of brews 2–4 were significantly different from brew 1. In case of the attribute “bitterness”, no significant difference was found between brews 2–4 and the reference (brew 1).
Figure 4. Just-About-Right (JAR) frequencies for all brews for the attributes algal taste (A) and bitterness (B). Brews 1–4: n = 19. Brews 5 and 6: n = 25. *Significant difference of averages to reference brew 1 (α = 0.050). **Significant difference of averages to reference brew 4 (α = 0.050).
In summary, the inclusion of spirulina in the mild pale ale resulted in a relatively non-popular beer with an unpleasant algal taste and a more intensively perceived bitterness. Nevertheless, the blue color of brew 4 was perceived as an interesting feature. The following parts of the work focus on the improvement of the sensory properties.
Based on the evaluation of brews 1–4, it was shown that the production of the beers and explicitly the fermentation step was not significantly disturbed. On the other hand, the inclusion of spirulina at different times during the processing resulted in greatly altered sensory properties for the final product (strong color change and a dominant algal taste). The development of the algal taste in particular compromises consumer acceptance. In the following steps, a further evaluation was carried out to determine how the dominant algal taste can be diminished without decreasing the algal content of the recipe while retaining the blue color of the beer. The additional two brews corresponded to the general characteristics of an India pale ale (IPA). With 60 IBU, this beer type is known to be more bitter and very hoppy. Two different IPA styles were brewed (Figure 1). The basic process parameters and characteristics of the two different IPA are shown in Table 2. Inclusion of spirulina in brews 5 and 6 resulted in a very similar blue color to that of brew 4. The L*a*b* color analysis as well as the PC content exhibited very similar values for the last three brews (Supplementary Table S3; Figure 3). In contrast to this, the sensory evaluation exhibited strongly different properties. Brews 5 and 6 shared a similarly high popularity compared to the control beer (Table 3). Eighty-eight and Eighty percentage of the panelists rated the beer positively (between 9 and 6) for brews 5 and 6, respectively. The attributes that were noted to explain the decisions made by the panelists were much more positive compared to the previous algae-containing beers (brews 2–4). Listed attributes are sweetness, pleasant bitterness, pleasant algal taste, and the positively evaluated blue color. Compared to brew 4, the JAR analysis showed a less intense algal taste in the altered brew variants (Figure 4). Nevertheless, the difference of the means from the JAR distributions were not significantly different for the attribute “algal taste”. Surprisingly, the perceived bitterness of the new brews (5 and 6) was strongly diminished and was not in proportion to the very high bitterness of around 60 IBU of the IPA brew style (Figure 4). In case of the attribute “bitterness”, the mean of the JAR distribution of brew 6 was significantly different in relation to the reference (brew 4) and for both IPAs, around 80% of the panelists rated the bitterness with “just right”. Finally, the more hop-accentuated beer with fruity hops (brew 5) was more compatible with the introduced algal taste.
Industrial utilization of microalgae and cyanobacteria is a rather new business, which started just a few decades ago (Khan et al., 2018). Nowadays, these microorganisms are increasingly recognized as a sustainable source of protein-rich foodstuffs, lipids, and antioxidants (Smetana et al., 2017; Sandmann et al., 2018, 2021; Arun et al., 2020; Levasseur et al., 2020; Verdelho et al., 2022). They exhibit much higher growth rates than plant crops, which is a major reason driving ongoing research. Additionally, algal cells and cyanobacteria grow in suspension but need less water than terrestrial crops, and their cultivation does not need pesticides or herbicides. Despite numerous benefits, there are still different challenges for large-scale production of the biomasses. There is still a lack of knowledge about how to transfer the high growth rates known from lab experiments to large-scale production photobioreactors (PBR) (Khan et al., 2018). For this reason, intense research efforts have been made to improve either the understanding of the complex interaction of cells within the PBR or to improve the PBR performance itself (Enfors et al., 2001; Huang et al., 2017; Wurm and Sandmann, 2021; Sandmann et al., 2022).
Despite open questions about PBR design, there are other bottlenecks at the application level. First, inclusion of algal or cyanobacterial biomasses in “classical” products often interferes with the sensory properties of the known products and ultimately results in lower consumer acceptance (Lafarga, 2019; Francezon et al., 2021). An example would be the inclusion of spirulina or Chlorella biomass into bread, which introduces a strong color change and might look like moldy bread. Second, other unfavorable factors might appear, such as the need to modify manufacturing technologies or an increased sensitivity of the product to oxygen (Lafarga, 2019; Francezon et al., 2021). Third, microalgae and spirulina are also known to exhibit antimicrobial properties (El-Sheekh et al., 2014; Martelli et al., 2020a; Hensel et al., 2021; Tavakoli et al., 2021). This might interfere with typical processing steps in food technology that are based on fermentation and thus on intended microbial growth processes (Ozdemir et al., 2004; Abedin and Taha, 2008; Niccolai et al., 2020). The aim of this study was to investigate the suitability of spirulina biomass as a substrate for yeast-driven fermentation during the beer brewing process. Additionally, putative adverse effects on sensory properties of the beer should be optimized to create a tasty beer with high consumer acceptance. The replacement of 5 % (w/w) malt by spirulina was the same as in the commercially available Chlorella beer (Table 1). Evaluation done by including spirulina at different processing steps during the brewing process, which results in different thermal stresses for the cyanobacterial constituents (Figure 1). In brew 4 the spirulina was added during wort cooling (below 40°C) which correlates with the lowest thermal stress for the cyanobacterial biomass. The recent study showed that the parameters for yeast-driven fermentation were altered but were still in the target range to create a beer with typical properties. This can be shown by the alcohol content as the main product of the fermentation and the general time-dependent FAN and total sugar decrease during fermentation (Table 2; Figure 2). A summary of the influence of spirulina biomass on brew 4 (mild pale ale style and inclusion of spirulina during wort cooling phase) is shown in Figure 5. These parameters include an increased pH of the final beers, which was more pronounced with later inclusion of spirulina biomass. Additionally, the original extract in the final beer of brew 4, which corresponds to the latest inclusion time, was increased by 25% relative to the control beer. A similar and significant increase was also observed in case of the sugar content of the final beer (brew 4). Brew 4 also showed significant changes in the color and exhibited a deep blue color (Supplementary Table S3; Figure 3). This temperature was chosen because it has been shown that PC is comparatively stable below 40°C and at pH values between 5.0 and 6.0 (Wu et al., 2016; Böcker et al., 2020). All spirulina-containing beers had such a beneficial pH value, but the reason remains unclear. Usually, the pH of beers is around 4.5 like in the control (brew 1) which would likely cause an instability of phycocyanin during storage. In brews 2 and 3, it is very likely that PC was denatured and lost its spectral properties during mashing and boiling of the wort. Additionally, inclusion of spirulina at different times during the processing introduced a dominant algal taste (Supplementary Table S3; Figure 3) which compromised the popularity of the beers (Table 3). Despite the algal taste, the panelists noticed a stronger sweetness and an increased bitterness (Table 3; Figure 4). Brew 4 was the basis for further changes in the brewing technology and the recipe to cover the dominant algal taste. The alternative beer type was an India pale ale style (IPA). With 60 IBU this beer type is very hoppy which was expected to diminish the algal taste. Furthermore, two different hop compositions were used to evaluate better potential for covering the algal taste. First, inclusion of spirulina in brews 5 and 6 also resulted in a deep blue color with very similar color characteristics and PC contents similar to brew 4. Second, the new beers exhibited a much higher popularity than the other algae containing beers and can be designated as popular (Fliedner and Wilhelmi, 1993). Third, spirulina seems to cover the bitter impression in more bitter and more aromatic beers according to the results of JAR. Fourth, a sweeter impression was described, even for the very hoppy IPA beers. Such relationships between inclusion of spirulina in beers and a coupling of the biomass inclusion onto distinct sensory properties of the beers have not been described before. The spirulina containing beers exhibited higher FAN contents, resulting from the high protein content of the cyanobacterial biomass (Supplementary Table S1). On one hand, the residual FAN seems not to be fermentable. On the other hand, the increased FAN had no pronounced adverse effects on the brewing process itself, as the product was a beer with high consumer acceptance and typical characteristics. Addition of extracted phycocyanin to the brewing process could diminish the increase of FAN and may also result in a blue beer. Nevertheless, the costs of the beer will increase significantly because the extraction and purification of the PC is a multistep procedure which still must be optimized on industrial scale. Technological modification and a change in the recipe of the beers, including more hops, resulted in a unique beer with high popularity (brews 5 and 6). For an industrial application, stability of the flavor components and the blue color would be of high importance. Stability tests should be carried out in future studies, to ensure a stability over several months. In addition, pasteurization on the product, which is routinely done in the brewing industry, should be considered. Continuous high-temperature short-time treatments of phycocyanin revealed a high temperature sensitivity starting at 50°C, which was also supported by Wu et al. (2016) and Böcker et al. (2020). Flash pasteurization (e.g., for 20 s at 72°C) which is frequently used in the brewing industry may minimizes the loss of spectral properties of the PC. On the other side the inclusion of spirulina biomass in beers will increase costs and may be suitable only for small breweries with focus on specialty beers. These small breweries often produce unpasteurized beer, which has only a shelf life of 1 week with up to 2 months. In these unpasteurized beers the blue color might be relatively stable, because the created beers in this study still exhibited a deep blue color even after the 6 weeks until full maturation. However, this remains speculative and should be studied in future. Another critical aspect that must be investigated in further studies is the putative introduction of spoilage microorganisms from spirulina biomass, affecting taste and shelf life of the beers. Three aspects may support such potential microbial contaminations. First, if spirulina is added during the wort cooling phase at temperatures below 40°C. Second, increased pH value in the spirulina containing beers will likely not inhibit growth of disturbing microorganisms as in classical beers. Third, if no pasteurization is conducted to keep the blue color stable. On the other hand, the high amount of hop in the used IPA beers will help to inhibit microbial growth (Vaughan et al., 2005).
Figure 5. Summarized influence of spirulina biomass on a beer belonging to the mild pale ale style if biomass inclusion done during wort cooling (brew 4). Photograph of the blue beer derived from brew 4.
Finally, the question may arise about whether such a blue spirulina-containing beer would be marketable. There are at least two aspects. First, there is strongly growing interest from consumers for “bio-functional foods” which contain bioactive compounds such as polyphenols or other antioxidant compounds that might be beneficial for human health (Bagchi, 2006; Hafting et al., 2012). On the other hand, the deep blue color can be used as an outstanding marketing feature. Nevertheless, the described blue beers would likely remain a niche product in future.
Any relation between the introduced spirulina biomass and a possible slight inhibition of the fermentation step cannot be explained without much deeper investigations. Even in conventional beers, there are still numerous open questions about exactly how the ingredients in the wort are consumed by the yeast and interact with signaling pathways of the yeast during fermentation. These questions can only be answered in the future with modern analytical tools and techniques or even systems biology approaches (Kerr et al., 2021). On the other hand, more detailed biochemical analyses of the novel beers are needed to investigate putatively introduced bioactive functions due to the incorporated spirulina.
Over the last 5 years another strategy for utilizing single-cell protein has appeared which is not focused on adding microbes to “classical” products but aims instead to utilize such single-cell proteins as a major constituent of new products. This includes the use of isolated spirulina protein in high moisture extrusion processing to create meat analogs (Palanisamy et al., 2019). Depending on the distinct cultivation conditions, such algal or cyanobacterial proteins could be one of the most sustainable protein sources in future (Smetana et al., 2017).
It is possible to integrate spirulina biomass [5% (w/w) of the malt] into the brewing process to create a tasty beer with high consumer acceptance. The parameters for yeast-driven fermentation were slightly altered but in general resulted in a beer with typical beer-like character. Depending on the time of addition in the mild pale ale manufacturing process, the beers exhibit a deep blue color that was caused by the microbial protein phycocyanin. On the other hand, there were major alterations in odor and taste which compromised consumer acceptance. Technological modification and a change in the recipe of the beers were necessary. The use of the India pale ale brew style, with increased hop dosage, resulted in a unique beer with high popularity.
The original contributions presented in the study are included in the article/Supplementary material, further inquiries can be directed to the corresponding author/s.
NB performed the experiments and took care of the chemical analysis of the gained products. MS supervised the experiments. All authors analyzed the data sets, prepared the manuscript, and approved the final version of the manuscript.
We acknowledge support for the Article Processing Charge from the Deutsche Forschungsgemeinschaft (DFG, German Research Foundation, 491232355) and the Open Access Publication Fund of the Hochschule Neubrandenburg (Neubrandenburg University of Applied Sciences).
The authors gratefully acknowledge Mrs. Gabriele Kulik for technical assistance with the brewing plant.
The authors declare that the research was conducted in the absence of any commercial or financial relationships that could be construed as a potential conflict of interest.
All claims expressed in this article are solely those of the authors and do not necessarily represent those of their affiliated organizations, or those of the publisher, the editors and the reviewers. Any product that may be evaluated in this article, or claim that may be made by its manufacturer, is not guaranteed or endorsed by the publisher.
The Supplementary Material for this article can be found online at: https://www.frontiersin.org/articles/10.3389/fsufs.2022.918772/full#supplementary-material
Abedin, R. M. A., and Taha, H. M. (2008). Antibacterial and antifungal activity of cyanobacteria and green microalgae. evaluation of medium components by placket- Burman design for antimicrobial activity of Spirulina platensis. Global J. Biotechnol. Biochem. 7, 22–31.
Araújo, R., Vázquez, C. F., Sánchez, L. J., Azevedo, I. C., Bruhn, A., Fluch, S., et al. (2021). Current status of the algae production industry in Europe: an emerging sector of the blue bioeconomy. Front. Mar. Sci. 7, 626389. doi: 10.3389/fmars.2020.626389
Arun, J., Gopinath, K. P., Rajan, P. S. S., Felix, V., Monica, M. J., and Malolan, R. (2020). A conceptual review on microalgae biorefinery through thermochemical and biological pathways: bio-circular approach on carbon capture and wastewater treatment. Bioresour. Technol. 11, 100477. doi: 10.1016/j.biteb.2020.100477
Back, W., and Narziß, L., (eds.). (2009). “Das Maischen,” in Die Bierbrauerei (Weinheim: Wiley-VCH), 233–396.
Bagchi, D. (2006). Nutraceuticals and functional foods regulations in the United States and around the world. Toxicology 221, 1–3. doi: 10.1016/j.tox.2006.01.001
Barkallah, M., Dammak, M., Louati, I., Hentati, F., Hadrich, B., Mechichi, T., et al. (2017). Effect of Spirulina platensis fortification on physicochemical., textural., antioxidant and sensory properties of yogurt during fermentation and storage. LWT Food Sci. Technol. 84, 23–330. doi: 10.1016/j.lwt.2017.05.071
Batista, A. P., Niccolai, A., Bursic, I., Sousa, I., Raymundo, A., Rodolfi, L., et al. (2019). Microalgae as functional ingredients in savory food products: application to wheat crackers. Foods 8, 1–22. doi: 10.3390/foods8120611
Bierbasis (2013). Braumanufaktur nimmt “Grüne Woche” wörtlich. Available online at: https://bierbasis.de/news/Heut-goenn-ich-mir-ein-Algenbier/89 (accessed March 29, 2022).
Böcker, L., Hostettler, T., Diener, M., Eder, S., Demuth, T., Adamcik, J., et al. (2020). Time-temperature-resolved functional and structural changes of phycocyanin extracted from Arthrospira platensis/Spirulina. Food Chem. 316, 126374. doi: 10.1016/j.foodchem.2020.126374
Böcker, L., Ortmann, S., Surber, J., Leeb, E., Reineke, K., and Mathys, A. (2019). Biphasic short time heat degradation of the blue microalgae protein phycocyanin from Arthrospira platensis. Innov. Food Sci. Emerg. Technol. 52, 116–121. doi: 10.1016/j.ifset.2018.11.007
Busch-Stockfisch, M. (2014). “Einrichten eines Sensoriklabors, Probenvorbereitung und -präsentation,” in Praxishandbuch Sensorik: In der Produktentwicklung und Qualitätssicherung, ed M. Busch-Stockfisch (Hamburg: Behr's Verlag), 1–12.
Chentir, I., Hamdi, M., Li, S., Doumandji, A., Markou, G., and Nasri, M. (2018). Stability, bio-functionality and bio-activity of crude phycocyanin from a two-phase cultured Saharian Arthrospira sp. strain. Algal Res. 35, 395–406. doi: 10.1016/j.algal.2018.09.013
Christaki, E., Florou-Paneri, P., and Bonos, E. (2011). Microalgae: a novel ingredient in nutrition. Int. J. Food Sci. Nutr. 62, 794–799. doi: 10.3109/09637486.2011.582460
Dekoperenkat (2020). Available online at: https://www.dekoperenkat.nl/d-al-g/ (accessed March 29, 2022).
DIN 10962:1997-10. (1997). Deutsches Institut für Normung e. V.: Areas for Sensory Analysis- Specifications of Testrooms. Berlin: Beuth Verlag GmbH.
DIN 10964:2014-11. (2014). Deutsches Institut für Normung e. V.: Sensory Analysis- Simple Descriptive Test. Berlin: Beuth Verlag GmbH.
El-Sheekh, M. M., Daboor, S. M., Swelim, M. A., and Mohamed, S. (2014). Production and characterization of antimicrobial active substance from Spirulina platensis. Iran. J. Microbiol. 6, 112–119.
Enfors, S. O., Jahic, M., Rozkov, A., Xu, B., Hecker, M., Jurgen, B., et al. (2001). Physiological responses to mixing in large scale bioreactors. J. Biotechnol. 85, 175–185. doi: 10.1016/S0168-1656(00)00365-5
Eriksen, N. T. (2008). Production of phycocyanin—a pigment with applications in biology, biotechnology, foods and medicine. Appl. Microbiol. Biotechnol. 80, 1–14. doi: 10.1007/s00253-008-1542-y
FDA (2018). Summary: Substances Generally Regarded As Safe (Final Rule). U.S. Food and Drug Administration (HHS). Available online at: https://www.fda.gov/about-fda/economic-impact-analyses-fda-regulations/summary-substances-generally-recognized-safe-final-rule (accessed March 29, 2022).
Fernández-Rojas, B., Hernández-Juárez, J., and Pedraza-Chaverri, J. (2014). Nutraceutical properties of phycocyanin. J. Funct. Foods. 11, 375–392. doi: 10.1016/j.jff.2014.10.011
Fliedner, I., and Wilhelmi, F. (1993). Grundlagen und Prüfverfahren der Lebensmittelsensorik. Hamburg: Behr's Verlag.
Fradinho, P., Niccolai, A., Soares, R., Rodolfi, L., Biondi, N., Tredici, M. R., et al. (2020). Effect of Arthrospira platensis (spirulina) incorporation on the rheological and bioactive properties of gluten-free fresh pasta. Algal Res. 45, 101743. doi: 10.1016/j.algal.2019.101743
Francezon, N., Tremblay, A., Mouget, J.-L., Pasetto, P., and Beaulieu, L. (2021). Algae as a source of natural flavors in innovative foods. J. Agric. Food Chem. 69, 11753–11772. doi: 10.1021/acs.jafc.1c04409
Hafting, J. T., Critchley, A. T., Cornish, M. L., Hubley, S. A., and Archibald, A. F. (2012). On-land cultivation of functional seaweed products for human usage. J. Appl. Phycol. 24, 385–392. doi: 10.1007/s10811-011-9720-1
Hensel, B., Jakop, U., Scheinpflug, K., Schröter, F., Sandmann, M., Mühldorfer, K., et al. (2021). Low temperature preservation: influence of putative bioactive microalgae and hop extracts on sperm quality and bacterial load in porcine semen. Sustain. Chem. Pharm. 19, 100359. doi: 10.1016/j.scp.2020.100359
Huang, Q., Jiang, F., Wang, L., and Yang, C. (2017). Design of photobioreactors for mass cultivation of photosynthetic organisms. Engineering 3, 318–329. doi: 10.1016/J.ENG.2017.03.020
Kaltner, D. (2000). Untersuchungen zur Ausbildung des Hopfenaromas und technologische Maßnahmen zur Erzeugung Hopfenaromatischer Biere (Ph.D. Thesis). Technische Universität München, München, Germany (In German).
Kerr, E. D., Caboche, C. H., Josh, P., and Schulz, B. L. (2021). Benchtop micro-mashing: high-throughput, robust, experimental beer brewing. Sci. Rep. 11, 1480. doi: 10.1038/s41598-020-80442-7
Khan, M. I., Shin, J. H., and Kim, J. D. (2018). The promising future of microalgae: current status, challenges, and optimization of a sustainable and renewable industry for biofuels, feed, and other products. Microb. Cell Fact. 17, 1–21. doi: 10.1186/s12934-018-0879-x
Kühbeck, F., Dickel, T., Krottenthaler, M., Back, W., Mitzscherling, M., Delgado, A., et al. (2005). Effects of mashing parameters on mash -Glucan, FAN and soluble extract levels. J. Inst. Brew. 111, 316–327. doi: 10.1002/j.2050-0416.2005.tb00690.x
Lafarga, T. (2019). Effect of microalgal biomass incorporation into foods: nutritional and sensorial attributes of the end products. Algal Res. 41, 101566. doi: 10.1016/j.algal.2019.101566
Lee, J.-B., Hou, X., Hayashi, K., and Hayashi, T. (2007). Effect of partial desulfation and oversulfation of sodium spirulan on the potency of anti-herpetic activities. Carbohydr. Polym. 69, 651–658. doi: 10.1016/j.carbpol.2007.01.024
Lee, J. B., Srisomporn, P., Hayashi, K., Tanaka, T., Sankawa, U., and Hayashi, T. (2001). Effects of structural modification of calcium spirulan, a sulfated polysaccharide from Spirulina platensis, on antiviral activity. Chem. Pharm. Bull. 49, 108–110. doi: 10.1248/cpb.49.108
Levasseur, W., Perré, P., and Pozzobon, V. (2020). A review of high value-added molecules production by microalgae in light of the classification. Biotechnol. Adv. 41, 107545. doi: 10.1016/j.biotechadv.2020.107545
Manirafasha, E., Ndikubwimana, T., Zeng, X., Lu, Y., and Jing, K. (2016). Phycobiliprotein: potential microalgae derived pharmaceutical and biological reagent. Biochem. Eng. J. 109:282–296. doi: 10.1016/j.bej.2016.01.025
Martelli, F., Alinovi, M., Bernini, V., Gatti, M., and Bancalari, E. (2020b). Arthrospira platensis as natural fermentation booster for milk and soy fermented beverages. Foods 9, 350. doi: 10.3390/foods9030350
Martelli, F., Cirlini, M., Lazzi, C., Neviani, E., and Bernini, V. (2020a). Edible seaweeds and spirulina extracts for food application: in vitro and in situ evaluation of antimicrobial activity towards foodborne pathogenic bacteria. Foods 9, 1442. doi: 10.3390/foods9101442
Mazinani, S., Fadaei, V., and Khosravi-Darani, K. (2016). Impact of Spirulina platensis on physicochemical properties and viability of Lactobacillus acidophilus of probiotic UF feta cheese. J. Food Proc. Pres. 40, 1318–1324. doi: 10.1111/jfpp.12717
MEBAK (2020a). Methode B-400.11.111. Freier Amino-Stickstoff (FAN) – Ninhydrin-Methode (spektralphotometrisch). Rev. 2020-10. Available online at: https://www.mebak.org/methode/b-400-11-111/freier-amino-stickstoff-fan-ninhydrin-methode-spektralphotometrisch/655 (accessed March 29, 2022).
MEBAK (2020b). Methode B-590.07.902. Scheinbares spezifisches Gewicht – Pyknometer. Available online at: https://www.mebak.org/methode/b-590-07-902/scheinbares-spezifisches-gewicht-pyknometer/769 (accessed March 29, 2022).
MEBAK (2020c). Methode B-590.10.024. Stammwürze, Extrakt und Alkohol – destillativ (Amtliche Methode). Available online at: https://www.mebak.org/methode/b-590-10-024/stammwuerze-extrakt-und-alkohol-destillativ-amtliche-methode/775 (accessed March 29, 2022).
Miller, G. L. (1959). Use of dinitrosalicylic acid reagent for determination of reducing sugar. Anal. Chem. 31, 426. doi: 10.1021/ac60147a030
Niccolai, A., BaŽec, K., Rodolfi, L., Biondi, N., Zlatic, E., Jamnik, P., et al. (2020). Lactic acid fermentation of Arthrospira platensis (Spirulina) in a vegetal soybean drink for developing new functional lactose-free beverages. Front. Microbiol. 11, 560684. doi: 10.3389/fmicb.2020.560684
Niccolai, A., Shannon, E., Abu-Ghannam, N., Biondi, N., Rodolfi, L., and Tredici, M. R. (2019). Lactic acid fermentation of Arthrospira platensis (spirulina) biomass for probiotic-based products. J. Appl. Phycol. 31, 1077–1083. doi: 10.1007/s10811-018-1602-3
Ozdemir, G., Karabay, N. U., Dalay, M. C., and Pazarbasi, B. (2004). Antibacterial activity of volatile component and various extracts of Spirulina platensis. Phytother. Res. 18, 754–757. doi: 10.1002/ptr.1541
Palanisamy, M., Töpfl, S., Berger, R. G., and Hertel, C. (2019). Physico-chemical and nutritional properties of meat analogues based on Spirulina/lupin protein mixtures. Eur. Food Res. Technol. 245, 1889–1898. doi: 10.1007/s00217-019-03298-w
Radiobrocken (2020). Available online at: https://www.radiobrocken.de/nachrichten/sachsen-anhalt-reporter/Blaues-Bier-aus-der-Hochschule-Anhalt-id432065.html (accessed March 29, 2022).
Rauscher, K., Engst, R., and Freimuth, U. (1986). Untersuchung von Lebensmitteln: Einführung und Anleitung zur Untersuchung der Lebensmittel pflanzlicher und tierischer Herkunft einschließlich Fremdstoffe und Trinkwasser. Leipzig: Fachbuchverlag.
Rechter, S., König, T., Auerochs, S., Thulke, S., Walter, H., Dörnenburg, H., et al. (2006). Antiviral activity of Arthrospira-derived spirulan-like substances. Antiviral Res. 72, 197–206. doi: 10.1016/j.antiviral.2006.06.004
Sandmann, M., Münzberg, M., Bressel, L., Reich, O., and Hass, R. (2022). Inline monitoring of high cell density cultivation of Scenedesmus rubescens in a mesh ultra-thin layer photobioreactor by photon density wave spectroscopy. BMC Res. Notes 15, 54. doi: 10.1186/s13104-022-05943-2
Sandmann, M., Schafberg, M., Lippold, M., and Rohn, S. (2018). Analysis of population structures of the microalga Acutodesmus obliquus during lipid production using multi-dimensional single-cell analysis. Sci. Rep. 8, 6242. doi: 10.1038/s41598-018-24638-y
Sandmann, M., Smetana, S., Heinz, V., and Rohn, S. (2021). Comparative life cycle assessment of a mesh ultra-thin layer photobioreactor and a tubular glass photobioreactor for the production of bioactive algae extracts. Bioresour. Technol. 340, 125657. doi: 10.1016/j.biortech.2021.125657
Smetana, S., Sandmann, M., Rohn, S., Pleissner, D., and Heinz, V. (2017). Autotrophic and heterotrophic microalgae and cyanobacteria cultivation for food and feed: Life Cycle Assessment. Bioresour. Technol. 245, 162–170. doi: 10.1016/j.biortech.2017.08.113
Stewart, G. G. (2013). “Biochemistry of brewing,” in Biochemistry of Foods, eds N.A. Eskin, M., and Shahidi, F (London: Academic Press), 291–318.
Stone, H., Bleibaum, R. N., and Thomas, H. A. (2012). Sensory Evaluation Practices. Boston, MA: Elsevier/Academic Press.
Tavakoli, S., Hong, H., Wang, K., Yang, Q., Gahruie, H., Zhuang, H., et al. (2021). Ultrasonic-assisted food-grade solvent extraction of high-value added compounds from microalgae Spirulina platensis and evaluation of their antioxidant and antibacterial properties. Algal Res. 60, 102493. doi: 10.1016/j.algal.2021.102493
Thesseling, F. A., Bircham, P. W., Mertens, S., Voordeckers, K., and Verstrepen, K. J. (2019). A hands-on guide to brewing and analyzing beer in the laboratory. Curr. Protoc. Microbiol. 54, e91. doi: 10.1002/cpmc.91
Tinseth, G. (1995). Glenn's Hop Utilization Numbers. Available online at: http://realbeer.com/hops/research.html (accessed March 29, 2022).
Vaughan, A., O'Sullivan, T., and Van Sinderen, D. (2005). Enhancing the microbiological stability of malt and beer - a review. J. Inst. Brew. 111 355–371. doi: 10.1002/j.2050-0416.2005.tb00221.x
Verdelho, V. V., Cadoret, J.-P., Acien, F. G., and Benemann, J. (2022). Clarification of most relevant concepts related to the microalgae production sector. Processes 10, 175. doi: 10.3390/pr10010175
Wu, H.-L., Wang, G.-H., Xiang, W.-Z., Li, T., and He, H. (2016). Stability and antioxidant activity of food-grade phycocyanin isolated from Spirulina platensis. Int. J. Food Prop. 19, 2349–2362. doi: 10.1080/10942912.2015.1038564
Wurm, H., and Sandmann, M. (2021). Establishment of a simple method to evaluate mixing times in a plastic bag photobioreactor using image processing based on freeware tools. BMC Res. Notes 14, 470. doi: 10.1186/s13104-021-05892-2
Yamaguchi, S. K. F., Moreira, J. B., Costa, J. A. V., de Souza, C. K., Bertoli, S. L., and Carvalho, L. F. D. (2019). Evaluation of adding spirulina to freeze-dried yogurts before fermentation and after freeze-drying. Ind. Biotechnol. 15, 89–94. doi: 10.1089/ind.2018.0030
Keywords: algae, mashing, beer brewing, phycocyanin, Arthrospira platensis, spirulina
Citation: Beisler N and Sandmann M (2022) Integration of Arthrospira platensis (spirulina) into the brewing process to develop new beers with unique sensory properties. Front. Sustain. Food Syst. 6:918772. doi: 10.3389/fsufs.2022.918772
Received: 12 April 2022; Accepted: 09 August 2022;
Published: 29 August 2022.
Edited by:
Myriam Loeffler, KU Leuven - Technologiecampus Gent, BelgiumReviewed by:
Mohan Naik, Karunya Institute of Technology and Sciences, IndiaCopyright © 2022 Beisler and Sandmann. This is an open-access article distributed under the terms of the Creative Commons Attribution License (CC BY). The use, distribution or reproduction in other forums is permitted, provided the original author(s) and the copyright owner(s) are credited and that the original publication in this journal is cited, in accordance with accepted academic practice. No use, distribution or reproduction is permitted which does not comply with these terms.
*Correspondence: Michael Sandmann, c2FuZG1hbm5AaHMtbmIuZGU=
Disclaimer: All claims expressed in this article are solely those of the authors and do not necessarily represent those of their affiliated organizations, or those of the publisher, the editors and the reviewers. Any product that may be evaluated in this article or claim that may be made by its manufacturer is not guaranteed or endorsed by the publisher.
Research integrity at Frontiers
Learn more about the work of our research integrity team to safeguard the quality of each article we publish.