- 1Department of Biotechnology, Centre of Innovation, Agricultural College and Research Institute, Tamil Nadu Agricultural University, Madurai, India
- 2Department of Plant Breeding and Genetics, Agricultural College and Research Institute, Tamil Nadu Agricultural University, Madurai, India
- 3Department of Plant Breeding and Genetics, Anbil Dharmalingam Agricultural College and Research Institute, Tamil Nadu Agricultural University, Trichy, India
- 4Department of Genetics and Plant Breeding, Centre for Plant Breeding and Genetics, Tamil Nadu Agricultural University, Coimbatore, India
- 5Department of Plant Biotechnology, Centre for Plant Molecular Biology and Biotechnology, Tamil Nadu Agricultural University, Coimbatore, India
- 6Regional Research Station, Tamil Nadu Agricultural University, Vridhachalam, India
- 7Department of Plant Molecular Biology and Bioinformatics, Centre for Plant Molecular Biology and Biotechnology, Tamil Nadu Agricultural University, Coimbatore, India
Black gram (Vigna mungo) is an important pulse crop of India. The leaf crinkle disease caused by the urdbean leaf crinkle virus (ULCV) is a severe threat to black gram production. Black gram plants infected by ULCV show a considerable decline in plant growth and yield. However, detailed information about the interactions between the host, black gram, and ULCV is unclear. This study investigated the responses of two cultivars VBN (Bg) 6 and CO 5 to ULCV infection by physiological, biochemical, and transcriptional analyses. Virus symptoms were mild in VBN (Bg) 6 but were serious in CO 5. Upon the viral infection, VBN (Bg) 6 exhibited a low reduction in chlorophyll content than CO 5. The levels of sugar, protein, phenol, hydrogen peroxide (H2O2), and malondialdehyde (MDA) contents were altered by a viral infection in both cultivars. Although, the activities of antioxidant enzymes [Ascorbate peroxidase (APX), superoxide dismutase (SOD), peroxidase (POD), and catalase (CAT)] were increased by ULCV infection. Following the viral infection, the expression level of the salicylic acid (SA), jasmonic acid (JA), and ethylene (ET) defense pathway-related genes was recorded higher in the VBN (Bg) 6 than that recorded in CO 5, indicating a positive correlation between resistance and these indicative indices. This dynamic physio-biochemical and transcriptional profiles of two black gram cultivars on ULCV infection augment our understanding of the complex interaction response between this crop and its pathogen. Additionally, it offers an inventory of potential indicators for future black gram screening and breeding to enhance resistance.
Introduction
Urdbean (Vigna mungo), popularly known as black gram, is a vital pulse crop widely grown in Asian countries, including Bangladesh, India, Pakistan, and Thailand. It is a short-duration crop and is well adapted to semi-arid conditions. Thus, it is an ideal crop for dryland farming. Black gram is also an excellent nutritional source for vegetarians, and the seeds possess high nutritional value with about 26% protein, which is thrice that of cereals. Additionally, it plays a major role in improving soil fertility by fixing symbiotic nitrogen. India ranks first in the production and consumption of black gram in the world. Black gram occupied an area of 4.53 million ha with 2.08 million tons of production in India (Indiastat, 2020). However, the production is not enough to meet even the domestic consumption demands in the country. The seed yield is reduced by viral and fungal diseases (Gautam et al., 2016). Among the various viral diseases, the black gram is infected by the urdbean leaf crinkle virus (ULCV) that causes leaf crinkle disease and reduces the seed yield between 35 and 81%. The yield reduction depends upon the genotypes and time of virus infection (Bashir et al., 1991).
Black gram is highly susceptible to ULCV than mungbean and other major pulse crops. The virus infection can occur at any stage of crop development, from the seedling stage to harvest. The typical symptoms of the virus include severe crinkling, curling, puckering, and leaves' rugosity, followed by stunting of plants and malformation of floral organs. The virus-infected plants have shown reduced pollen fertility and pod formation. It is transmitted by aphids, whitefly, and leaf-feeding beetles and is also transmitted by seeds and mechanical sap-inoculation (Bashir et al., 2005; Ashfaq et al., 2007; Gautam et al., 2016). Until now, there is no taxonomical classification for ULCV. The diagnosis based on symptoms generally needs to be confirmed only by the virus detection through polymerase chain reaction (PCR) (Bashir et al., 1991; Reddy et al., 2005). Application of fungicides is used to control the disease, but it is expensive and, if used frequently, turns inefficient. To cope with the environmental hazards and combat against pathogens, plants are equipped with enhanced defense mechanisms to defend themselves. Some of these mechanisms are basic, whereas the remainders are triggered by the infection process. Thus, investigating the plant's physiological and biochemical responses after infection improves our understanding of pathogenesis and resistance, or susceptibility of the plants against pathogens.
Plants defense mechanisms against pathogens include (i) the activation of the antioxidant system and pathogenesis-related proteins (Van Loon et al., 2006; Pál et al., 2013); (ii) induction of defense signaling pathways by reactive oxygen species (ROS) production (Torres et al., 2006; Shetty et al., 2008; Derksen et al., 2013; Sewelam et al., 2016); and (iii) strengthening of the cell wall through deposition of defense-related materials, such as lignin, cutin, and suberin at the site of infection (Matern et al., 1995; Hématy et al., 2009; Chang et al., 2016). Plant immune response to the viral infection has been found to increase by enhancing the production of ROS [i.e., hydrogen peroxide (H2O2), superoxide (O), and hydroxyl (OH−) radicals] by higher leakage of electrons to molecular oxygen (Arora et al., 2002). ROS not only cause irreversible DNA damage and cell death, but also function as important signaling molecules that regulate normal plant growth, and responses to stress. However, abundant ROS production leads to oxidative stress that causes damage to the plant's structure. Therefore, to maintain the ROS at the optimal level, plants activate the enzymatic antioxidant system that includes enzymes, viz., ascorbate peroxidase (APX), superoxide dismutase (SOD), peroxidase (POD), and catalase (CAT) (Van Loon et al., 2006). Augmented production of antioxidant enzymes and PR proteins can improve the resistance and adapt plants to systemic viral infection (Hančević et al., 2018). Therefore, the balance in antioxidative enzyme activities and ROS formation governs whether to lead to oxidative signaling or damage to proceed (Møller et al., 2007). Many experimental data from the model plants have also shown that phytohormones [Abscisic acid (ABA), ethylene (ET), gibberellic acid (GA), jasmonic acid (JA), and salicylic acid (SA)] (Vijayan et al., 1998; Navarro et al., 2008; Wang et al., 2013; Lievens et al., 2017), non-enzymatic antioxidants (Glutathione) (Sinha et al., 2015), and defense compounds (flavonoids, polyphenols, and phenolic acids) (Joshi et al., 2016) are induced to respond against pathogens, and thus, activate plant defense.
So far, a detailed study in terms of comparative evaluation of physio-biochemical and transcriptional changes during early infection with ULCV of black gram has not been conducted. Very few preliminary studies were carried out on black gram cultivars and their responses to ULCV (Ashfaq et al., 2010, 2014; Srivastava and Singh, 2010). With this backdrop, this research is aimed to document the physio-biochemical and transcriptional changes of two black gram cultivars after infection with ULCV.
Materials and Methods
Plant Materials
Black gram cultivar VBN (Bg) 6 was obtained from the National Pulses Research Centre, Tamil Nadu Agricultural University, Vamban, Pudhukottai, India. CO 5 was obtained from the Department of Plant Genetic Resources, Centre for Plant Breeding and Genetics, Tamil Nadu Agricultural University, Coimbatore, India. Both cultivars are popular in Tamil Nadu, a South Indian state, which is one of the leading black gram producing states in India.
Virus Source and Maintenance
The leaves of black gram plants with ULCV symptoms were collected from the farmers' fields of Sivagangai and Pudukkottai, two southern districts of Tamil Nadu in India. Furthermore, the leaves were dried in anhydrous calcium chloride desiccators and then moved to vinyl bags, cooled and transported on ice, and stored at −80°C. The virus was cultured separately on grown plants of susceptible black gram cultivar CO 5 by successive inoculations in the insect-free net house at Agriculture College and Research Institute, Tamil Nadu Agricultural University, Madurai, India. Plants of CO 5 were mechanically inoculated with the virus when the unifoliate leaves began to unfold. With the help of the abrasive, namely, 600-mesh carborundum, the leaves were dusted up prior to inoculation and washed in running tap water. Then, the infected leaves were ground in a 0.01 mol/L of phosphate buffer @ 3–5 ml for every black gram of leaf tissue at a pH of 7.2 using a pestle and mortar placed in an icebox for preparing the inoculum. Afterward, the inoculated plants were observed for virus symptoms at 21 days post-inoculation (dpi). When the ULCV symptoms (Severe crinkling, curling, puckering, and rugosity) were visible on the leaves of inoculated plants, symptomatic leaves were collected and used as a source of inoculum for further experiments.
Inoculation of Black Gram Plants and Sample Collection
The seeds for two black gram cultivars [VBN (Bg) 6 and CO 5] were sown in pots (15 L, 30 cm height, 33.0 cm diameter at top, and 25.5 cm bottom diameter) containing a 3:1 ratio of soil and compost in three replications and grown in an insect-free greenhouse at the Agricultural College and Research Institute, Tamil Nadu Agricultural University, Madurai, India. Plants were mechanically inoculated with ULCV and evaluated for virus pathogenicity by visual assessment in 7, 14, 21, 28, and 35 days of post-inoculation (dpi) as per the method described above. The plants were characterized using the disease rating scale (Table 1). The mock-inoculated control plants underwent the same inoculation procedure but, only phosphate buffer was used. The leaves of the cultivars were collected at 0, 1, 3, 7, and 14 dpi from both inoculated and mock-inoculated control plants for biochemical analysis. The collected leaf samples were immediately frozen in liquid nitrogen and stored in a freezer at −80°C up to the time of analysis. Photosynthesis pigments, sugar, proteins, and phenols were estimated from the collected leaves at 0 and 14 dpi. Level of H2O2 and malondialdehyde (MDA) contents, enzyme activities, and gene expression were examined from leaves collected at 0, 1, 3, 7, and 14 dpi.
Determination of Photosynthesis Pigments and Sugar Contents
Total chlorophyll, chlorophyll a, and chlorophyll b contents were determined from the leaves of virus inoculated and control plants of both cultivars following the method described by Lightenthaler (1987). The 0.25 g of leaf samples were added to 10 mL of 80% acetone, grounded, and filtered with muslin cloths. The absorbance readings were recorded at wavelengths of 663 and 645. Total sugar was estimated following the method described by Sadasivam and Manickam (1996). The leaves were treated with 80% ethanol to extract the total sugar in anthrone. The standard curve prepared from standard glucose was used to compare the estimated total sugar as glucose equivalent and were expressed as milligram per gram of fresh weight of tissue at 630 nm.
Estimation of Total Soluble Protein and Phenols
Total soluble protein was estimated using the method described by Bradford (1976). Leaf samples (1 g) were homogenized and then centrifuged at 10,000 rpm at 4°C for 20 min. The readings of absorbance were measured at 595 nm, where bovine serum albumin (BSA) was used as standard. Total phenols were estimated according to the method described by Sadasivam and Manickam (1996). Leaf samples (1 g) were extracted in 80% methanol and then centrifuged at 12,000 rpm for 15 min at 4°C. The absorbance value was recorded at 650 nm. The standard curve was prepared with catechol as standard. The total phenol was estimated and expressed as mg/g fresh weight from the standard curve.
Measurement of Hydrogen Peroxide and Malondialdehyde Concentration
The H2O2 content was measured following the method described by Loreto and Velikova (2001). Leaf samples (1 g) were ground in ice-cold 0.1% TCA and centrifuged at 12,000 rpm for 15 min at 4°C. Afterward, 0.5 ml of 10 mM potassium phosphate buffer and 0.75 ml of 1 M KI were mixed with 0.5 ml of the supernatant. The readings of absorbance were measured at 390 nm against a blank, and a standard calibration curve inferred the H2O2 content and plotted against the curve from known H2O2 concentrations. Results were expressed as μmol/g fresh weight. Lipid peroxidation was measured following the method of Stewart and Bewley (1980) and determined in terms of MDA content. Leaf samples (1 g) were homogenized in 0.1% of trichloroacetic acid (TCA), and the homogenate was centrifuged at 12,000 rpm for 15 min at 4°C. Following this, 0.5 mL of the supernatant was collected and mixed with a 1 mL volume of 20% TCA containing 0.5% thiobarbituric acid (TBA) solution. The reaction mixture was incubated at 95°C for 30 min and then cooled on an ice bath to end the reaction. The mixture was centrifuged at 12,000 rpm for 10 min, the aliquots of supernatant were collected, and the readings of absorbance were measured at 532 nm. The content of MDA was measured by 155 mM−1cm−1 extinction coefficient. The results were expressed as μmol/g fresh weight.
Enzyme Activity Assays
Leaf samples (1 g) were taken and homogenized in 10 mL of ice-cold 50 mM potassium phosphate buffer. The phosphate buffer pH was adjusted to pH 7.0 for APX and CAT and to the pH of 7.8 for SOD and POD assays, containing 0.1-mM Na2 EDTA and 1% (w/v) polyvinylpyrrolidone (PVP). About 1 mM of ascorbate was added with no EDTA in the buffer for APX. With four layers of muslin cloth, the homogenate was filtered, and it was centrifuged at 12,000 rpm at 4°C for 15 min. Enzyme activity assays were performed with the supernatant from centrifugation. The decrease in the absorbance at 290 nm was recorded since ascorbates get oxidized. This results in the estimation of APX activity (Nakano and Asada, 1981). With guaiacol as an electron donor, as per the protocol of Chance and Maehly (1955), the activity of POD was estimated at 470 nm. An absorbance change of 0.01 unit min−1 was referred to as one unit of POD activity. The method used to determine the SOD activity was reported by Madamanchi and Alscher (1991), and the readings of absorbance were measured at 560 nm. SOD activity was examined by measuring its ability to inhibit the photochemical reduction of nitroblue tetrazolium (NBT). One unit of SOD activity is the quantity of the concentration of the enzyme extract that causes a 50% inhibition of the reaction (Beauchamp and Fridovich, 1971). The activity of CAT was determined using the method of Aebi (1984). When the absorbance goes below 240 nm, the consumption of H2O2 occurs at an interval of 2 min, whereas 1 mM of H2O2 was decomposed by one unit of CAT per minute. All the results were expressed as units per mg of protein.
Quantitative Real-Time PCR (qRT- PCR) Analysis
Total RNA was extracted by the RNeasy plant mini kit (Qiagen, USA) following the user guidelines and treated with RNase-free DNAseI (Promega, USA). The quality and quantity of RNA were confirmed by the 1% agarose gel via electrophoresis and the bio spectrometer (Eppendorf, Germany) at the absorbance ratio of 280 nm. First-strand cDNA was synthesized with the transcriptor First Strand cDNA Synthesis Kit (Roche Applied Science, Germany), following the manufacturer's instructions. PCR amplifications were carried out by the Light-Cycler® 480 (Roche Applied Science, USA) containing 1 μl of cDNA, 0.5 μl of each primer (10 μM), and 5 μl (2×) of Fast Start Essential DNA Green Master mix (Roche Applied Science, Germany) and 3 μl of ddH2O in a total volume of 10 μL. The thermal conditions are as follows: 95°C for 5 min, followed by 40 cycles of 95°C for 10 s, 60°C for 30 s, and then 72°C for 30 s. Three technical replications were performed. Primer pairs were designed by Primer 5 from SA, JA, and ET pathway-associated genes of black gram transcript sequences (Supplementary Table 1). The actin gene was used as an internal control for the normalized expression. The relative expression was calculated using the 2–ΔΔCT method.
Statistical Analysis
The three sets of replicated data were recorded in terms of mean ± SE and were processed through a simple variance analysis (ANOVA). All analyses were worked out using the SPSS statistical package (SPSS Inc., Chicago, IL, USA).
Results
Responses of the Black Gram Cultivars to ULCV
The plants of VBN (Bg) 6 and CO 5 were inoculated with ULCV, and the development of symptoms was examined over the course of 5 weeks. Observations taken at 14 and 21 dpi showed a clear difference in symptom development between VBN (Bg) 6 and CO 5. The black gram cultivar CO 5 exhibited symptoms including crinkling, curling, and puckering. In contrast, the development of reduced symptoms in VBN (Bg) 6. No symptom development was observed in the mock-inoculated plants of VBN (Bg) 6 and CO 5. By the completion of the study, it was found that ULCV caused severe symptoms to CO 5, with consistence to the grouping of this cultivar as susceptible, the disease score being (5), whereas VBN (Bg) 6 exhibited less symptoms to ULCV and was therefore consistent with the grouping of this cultivar as moderately resistant, the disease score being (2). With all these data considered together, the results confirm the differing susceptibility nature of VBN (Bg) 6 and CO 5, respectively.
Photosynthesis Pigments and Sugar Contents
The total chlorophyll, chlorophyll a, and chlorophyll b and sugar contents are presented in Table 2. Virus inoculated plants of VBN (Bg) 6 and CO 5 showed a decrease in the total chlorophyll, chlorophyll a, and chlorophyll b contents compared to its control plants. Through comparing the decrease in both the cultivars, we found that the CO 5 showed a significant decrease while a slight decrease was observed in VBN (Bg) 6 compared to their respective control plants. Total sugar was estimated in both the cultivars. The plants of VBN (Bg) 6 and CO 5 recorded an increase in total sugar. CO 5 showed a significant increase, whereas the increase in VBN (Bg) 6 was non-significant compared to its control plants.
Total Soluble Protein and Phenols
The total soluble protein content slightly increased by 7.07% in virus-inoculated plants of VBN (Bg) 6 than its control plants, whereas it decreased by 26.96 % in the inoculated plants than the control plants of CO 5. The level of total phenols increased by 6.67% in the inoculated plants of VBN (Bg) 6, whereas it decreased by 9.35% in inoculated plants of CO 5 when compared to controls. There was a significant decrease in the level of protein content in CO 5. However, the change in the level of phenols in both the cultivars was non-significant. The changes in the level of total soluble protein and phenols are presented in Table 2.
Changes in H2O2 and MDA Contents
The contents of H2O2 in the virus-inoculated plants followed different trends in the two- black gram cultivars. Both cultivars had shown H2O2 burst from 1 dpi, 91.03, and 52.87% was increased in VBN (Bg) 6 and CO 5 plants, respectively at 3 dpi. Until 14 dpi, VBN (Bg) 6 maintained a high level of H2O2, whereas CO 5 plants had decreased contents of H2O2. The level of MDA in both the infected cultivars was found to be higher, implying that CO 5 had higher MDA contents. In fact, after virus inoculation, MDA content increased by 37.47% in the plants of CO 5, but only 16.02% in the plants of the VBN (Bg) 6 at 3 dpi. At 14 dpi, MDA content reached 97.58 and 46.12%, respectively, in the CO 5 and VBN (Bg) 6 plants. The H2O2 and MDA contents in VBN (Bg) 6 and CO 5 are presented in Figure 1.
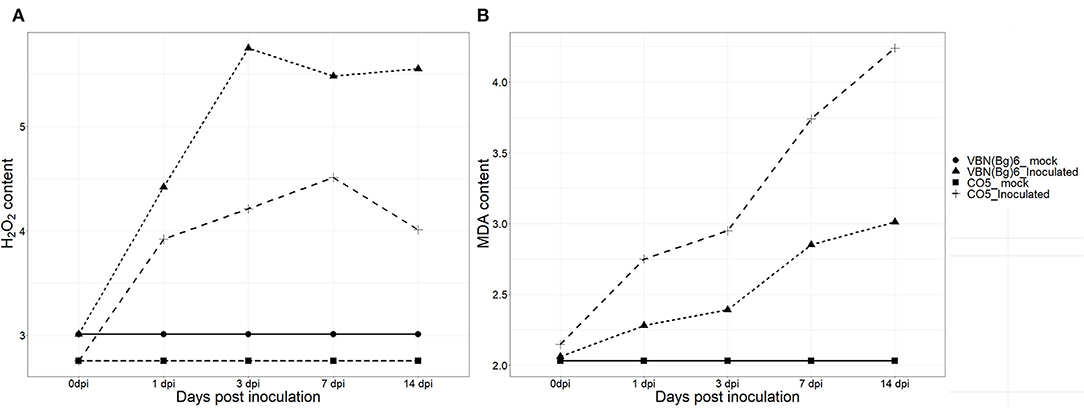
Figure 1. The changes of H2O2 (μmol g−1 FW) (A) and MDA (μmol g−1 FW) (B) concentrations in two blackgram cultivars VBN (Bg) 6 and CO 5 after urdbean leaf crinkle virus infection.
Antioxidant Enzymes Activities
Activities of the antioxidant enzymes (APX, POD, SOD, and CAT) were determined in both the cultivars after virus inoculation (Figure 2). Following the virus inoculation, the activities of antioxidant enzymes were increased in both the cultivars compared to their respective controls. APX, POD, and SOD showed a similar trend in VBN (Bg) 6 and CO 5 plants. The activities of these enzymes were high in VBN (Bg) 6 than that in CO 5 at 1, 3, 7, and 14 dpi. On the other hand, the trend of the CAT activity was opposite to APX, POD, and SOD at 1, 3, and 7 dpi. Interestingly, the CAT activity was higher in CO 5 at 1, 3, and 7 dpi compared to VBN (Bg) 6. However, at 14 dpi, the CAT activity was higher in VBN (Bg) 6 than in CO 5.
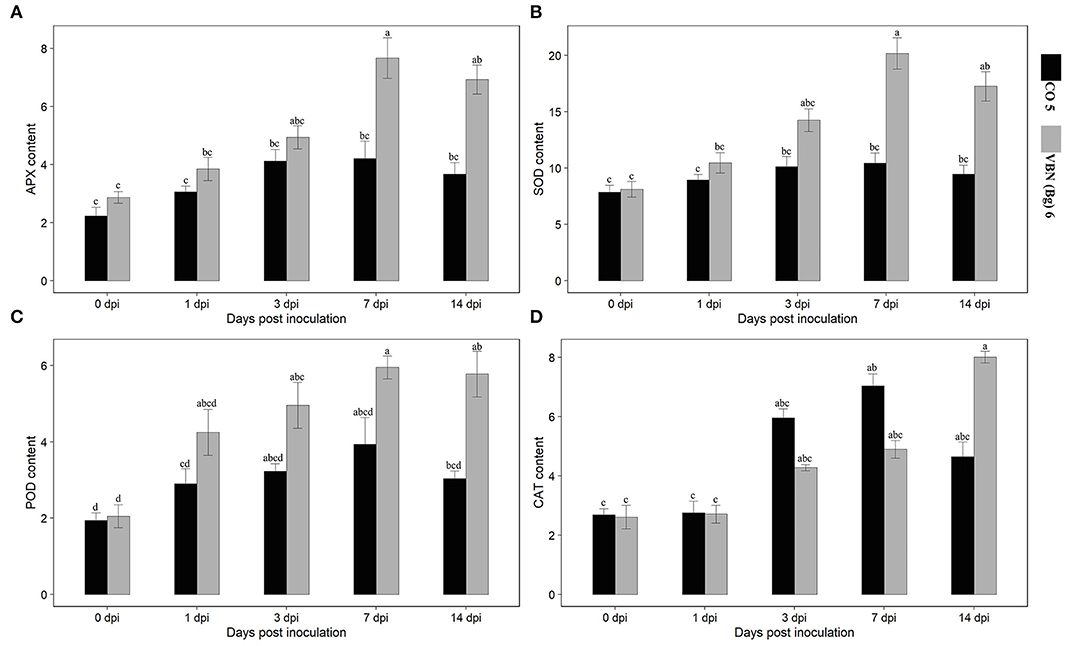
Figure 2. Activities of antioxidants in two blackgram cultivars VBN (Bg) 6 and CO 5 after urdbean leaf crinkle virus infection. (A) APX (Umg−1 Protein), (B) SOD (Umg−1 Protein), (C) POD (Umg−1 Protein), and (D) CAT (Umg−1 Protein). Values followed by the same letter are not significantly different (p ≤ 0.05) according to Duncan's multiple range test. Bars present mean ± SE (n = 3).
Defense Pathway-Related Genes Transcript Changes
After virus inoculation, the levels of expression of the genes corresponding to ET, JA, and SA defense pathways were investigated using the qRT-PCR analysis. It showed that the expressions of all the genes were increased in both the cultivars compared to their respective controls. Although, the genes that were differentially expressed in two cultivars and the expression of ET, JA, and SA-related genes were higher in VBN (Bg) 6 than in CO 5 (Figure 3). First, the transcription factor, VmWRKY, of the SA-related gene was upregulated from 1 dpi in VBN (Bg) 6, but not in CO 5, for which an increase was observed only from 3 dpi. Then, the expression level of VmWRKY increased in both the cultivars at 7 and 14 dpi in VBN (Bg) 6 (5.55- and 5.46-folds) and CO 5 (3.91- and 3.37-folds). After the virus inoculation, the expression of VmNPR1 related to SA exhibited the same trend as that detected with VmWRKY. The expression level of VmNPR1 was increased by 4.51-folds in VBN (Bg) 6 and by 3.08-folds in CO 5 at 3 dpi. Furthermore, the expression level of VmNPR1 increased and reached a peak at 7.35- and 5.29-folds in VBN (Bg) 6 and CO 5 at 7 dpi. The expression level in both the cultivars slightly decreased at 14 dpi.
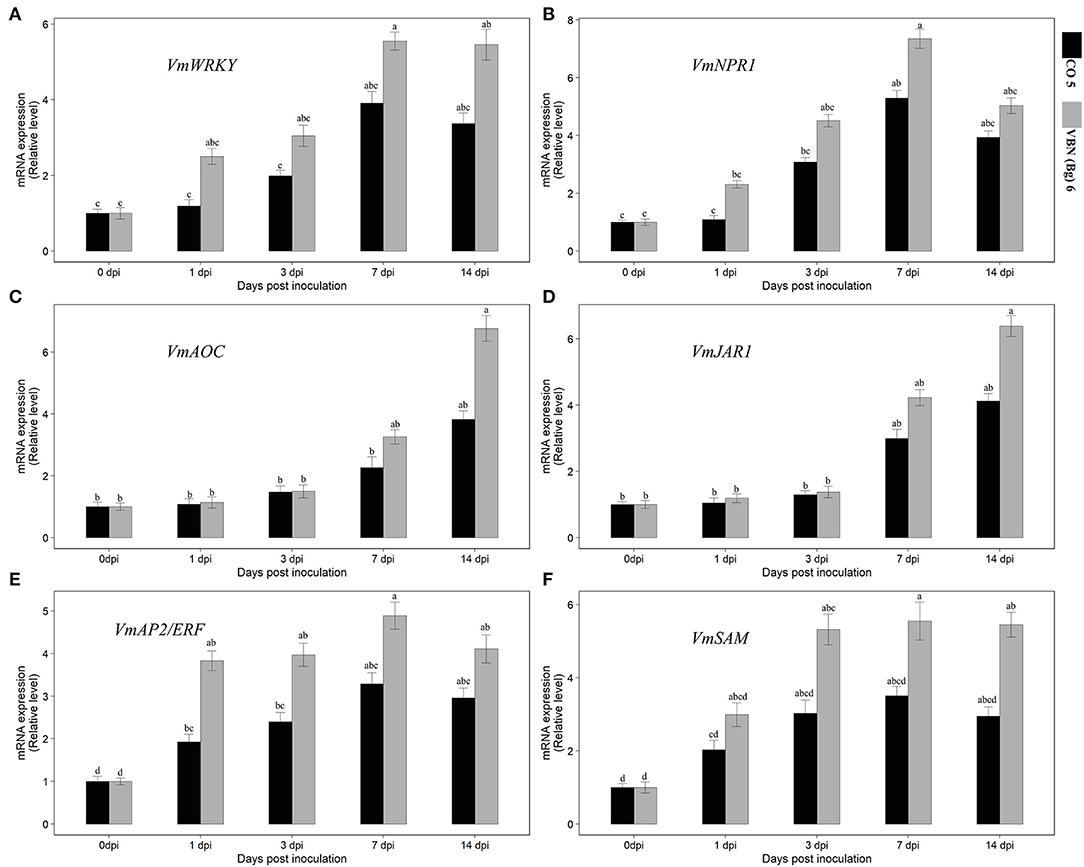
Figure 3. Effect of urdbean leaf crinkle virus infection on the relative expression level of SA [VmWRKY and VmNPR1] (A,B), JA [VmAOC and VmJAR1] (C,D), and ET [VmAP2/ERF and VmSAM] (E,F) pathway-related genes in two blackgram cultivars VBN (Bg) 6 and CO 5. Values followed by the same letter are not significantly different (p ≤ 0.05) according to Duncan's multiple range test. Bars present means ± SE (n = 3).
The JA-associated genes also showed that the differential expression pattern similar to the SA-related genes. However, the higher expression was observed in later days, unlike SA, which showed upregulation in the early days. VmJAR1 did not show higher expression at 1 and 3 dpi in both the cultivars. But, both cultivars increased at 7 and 14 dpi, which was 4.23- and 6.39-folds and 3- and 4.13-folds, respectively, in VBN (Bg) 6 and CO 5. The expression of VmAOC associated with JA followed the same trend in the pattern of expression in VBN (Bg) 6 and CO 5, as that detected with VmJAR1. In particular, the activity of VmAOC was lower in CO 5. After virus inoculation, the VmAP2/ERF and VmSAM genes related to ET showed differential upregulation in the two cultivars. In the genes, VmAP2/ERF and VmSAM recorded a considerable increase in the level of expression in the two cultivars by 14th dpi, with a higher upregulation up to 4.11- and 2.96-folds and 5.45- and 2.95-folds, respectively, in VBN (Bg) 6 and CO 5. The expression pattern was found to be the same in both genes.
Discussion
Despite the relevance of studying host–pathogen interactions in both resistant and susceptible backgrounds, research in this area is limited. Differential studies in the immune responses of resistance against a susceptible variety over an infection by a pathogen are indispensable since it explains the basis of plant immunity mechanism and also help in the complete understanding of the resistance mechanism. So, elite disease-resistant crop lines could be developed successfully. Hence, this research describes the physio-biochemical and transcriptional changes of black gram cultivars differing in their response to ULCV. Many researchers stated and discussed the chlorophyll and sugar alterations due to viral infections. In many cases, as a result of the virus and host interactions, as the infection progresses, the photosynthetic activity declines (Sampol et al., 2003; Zhou et al., 2004). Several studies on the photosynthetic activity in the virus-infected leaves found that the growth of the plants is directly affected by faded photosynthesis. Funayama-Noguchi and Terashima (2006) showed that a decrease in photosynthesis was associated with chlorophyll content in virus-infected leaves. Viruses are known to produce toxic metabolites that may affect the chloroplast resulting in the decrease of chlorophyll pigments. In our study, there was higher chlorophyll content in the VBN (Bg) 6 than CO 5, and it was decreased following the viral infection. But a slight decrease was observed in VBN (Bg) 6, whereas CO 5 showed a considerable decrease after the viral infection. Similar results were obtained in the contents of chlorophyll “a” and “b”. It was also observed that after the viral infection, total sugar was increased in VBN (Bg) 6 and CO 5. Notably, CO 5 showed a considerable increase, whereas a slight increase was observed in VBN (Bg) 6 compared to its control plants. The accumulation of sugar induced the production of cell wall invertase, which inhibits phloem loading through the breakdown of sucrose into glucose and fructose. This, in turn, may lead to a possible transcriptional downregulation of photosynthesis. From this, we conclude that the viral infection increased the sugar accumulation and decreased the photosynthetic properties (chlorophyll “a” and “b”). The increase or decrease in VBN (Bg) 6 is not very high and it indicates that the nature of moderate resistance in VBN (Bg) 6 is due to its better photosynthetic properties and less accumulation of sugar, and our findings agree with the statements of Singh et al. (2015), Souza et al. (2017), and Patwa et al. (2020).
The plants that are infected by the virus have recorded high protein contents, and this could be attributed to the activation of the host defense and the attack mechanisms of the virus. Following viral infection, protein content was marginally increased in VBN (Bg) 6, whereas it was found to considerably decline in the plants of CO 5 compared to their respective control plants. A probable reason behind the substantial decline in the levels of total soluble protein content in the susceptible cultivar CO 5 after the viral infection is because of the higher levels of susceptibility that are imminent in this cultivar. The findings are also according to the reports on virus-infected with cotton and mungbean genotypes (Siddique et al., 2014; Madhumitha et al., 2020). Lignin and suberin are the key molecules that physiologically hinder physical blockades and thus prevent the entry and spread of pathogens. These two compounds are principally synthesized by phenolic compounds that augment the strength of the cell walls inside the host plants (Ngadze et al., 2012; Singh et al., 2014). Total phenols are always found to be in higher levels in the resistant genotypes than in susceptible ones. In this study, the total phenols were found to be high in the VBN (Bg) 6. After the viral infection, total phenols were found to increase in VBN (Bg) 6, while they were found to be low in CO 5 compared with the controls. A slight decrease or increase in the levels of total phenols was found in both the cultivars. The alteration in the levels of phenolic compounds after viral infection in the CO 5 cultivar was because of the suppression of the gene expression in the plant defense reactions by the virus on the infection (Markakis et al., 2010). Some phenols and phenolic derivatives are found to be toxic to plant pathogens, as reported by previous studies on pathogenic fungal, bacterial, and viral interactions in plants. Hence, they are classified as very noteworthy compounds related to the plant defense mechanism and are accumulated in higher quantities in many resistant germplasm and varieties (Gogoi et al., 2001). In such wild resistant varieties, they are found to be accumulated after pathogenic infection (Agrios, 1997). When a moderate resistant cultivar is infected in an early stage, H2O2 is produced more in abundance than in a susceptible cultivar. So, it is understood here in the mechanism of defense of VBN (Bg) 6 plants against ULCV that there is an early ROS burst and its prominent play in defense is inevitable. To avoid the intracellular attack from a pathogen, H2O2 is produced abundantly on the infection site. This is the first step of defense in prevention, and it is achieved by the microbial activity at the site of invasion, or by phytoalexins synthesis, or by the oxidative crosslinking of hydroxyproline-rich protein or other cell-wall reinforcing processes (Shetty et al., 2007). Notably, substantial induced ROS bursts were already reported in other pathosystems, for example mungbean yellow mosaic India virus (MYMIV) and tobacco mosaic virus (TMV) in mungbean and tomato, respectively (Chakraborty and Basak, 2018; Zhang et al., 2020). Our results are in accordance with the earlier studies by Díaz-Vivancos et al. (2008), wherein the production of H2O2 in young host plants was noted as the primary defense response against viruses.
To protect the host plant cells from the toxicity caused by ROS, the cells direct the synthesis of enzymes like APX, POD, CAT, and SOD. An increased accumulation of H2O2 at the site of invasion in the young resistant plants was noticed on infection in this study. The increase was attributed to the corresponding upsurge in SOD activity from 3 dpi and suppression in CAT activity until 7 dpi in the inoculated resistant plants. On the contrary, an instantaneous upregulation of CAT and APX activities from 3 dpi was noticed in the inoculated susceptible plants. This is why there was no burst of ROS. The upsurge in H2O2 production in the VBN (Bg) 6 on viral infection is the reason for the decreased scavenging activity of the host system. In VBN (Bg) 6 (A moderately resistant cultivar), the upregulation of the genes such as SOD, involved in H2O2 synthesis, enhances the production of H2O2. The CO 5 plants recorded lower levels of H2O2, and that was correlated to the production of the enzymes with scavenging activities, namely, APX and CAT. There may or may not be a decrease in the production of H2O2 accumulation or its upregulation except for the transcriptional upregulation of SOD. Higher and considerate levels of H2O2 are directly associated with the increased resistance in transgenic tobacco plants with lower contents of antioxidative enzymes (Mittler et al., 1999). When the VBN (Bg) 6 reaches the later stages of viral infection, the APX and CAT levels are increased substantially, promoting the host plant's antioxidation system. At the same time, the H2O2 levels are not changed in the host cells to avoid irreparable damage and death of the cells.
Through the activation of defense response pathways, plants turn resistant to various pathogens or insects. The primary participants of the defense mechanism of plants are phytohormones, namely, SA, JA, and ET (Van Wees et al., 2000). A very complex association of both synergistic and antagonistic interactions make up the signaling pathways in plants, and they altogether influence each other. It is found in many studies involving plant defense that ET is a modulator of either SA or JA (Adie et al., 2007). Although SA, JA, and ET pathways are involved in plant defense against viral pathogen infection, a convergence point at which these pathways interlink is through many defense-related genes. Following ULCV infection, SA, JA, and ET pathways linked genes (VmJAR1, VmAOC, VmWRKY, VmNPR1VmAP2/ERF, and VmSAM) expression was higher in the moderately resistant cultivar but not in the susceptible one. This shows that the effectual alteration in SA, JA, and ET pathways in the moderately resistant cultivar may play a vital role in decreasing the viral symptoms and enhancing ULCV resistance.
Many studies have shown that the early activation of the SA pathway is necessary for plants to defend against viral pathogens, particularly in the initiation of the SAR response (Alazem and Lin, 2015). It is possible that higher expression obtained in the SA-related genes may create a SAR response in VBN (Bg) 6 earlier than CO 5. Also, the higher expression of VmWRKY and VmNPR1 was concurrently accompanied by the event of H2O2 burst in the resistant cultivar. This implies that higher amounts of SA and H2O2 were produced in this cultivar and has been in agreement with earlier studies, with similar rises in the level of SA leading to H2O2 bursts (Shirasu et al., 1997). Moreover, SA controls the antioxidant enzyme activities, which play a crucial role in plants to defend against pathogens. Thus, we compared the level of gene expression in SA-related genes and the activities of antioxidant enzymes. In our study, the higher activities of antioxidant enzymes (APX, SOD, and POD) are accompanied by SA induction, particularly in the early stage of ULCV infection. This finding is consistent with other studies that have found a significant role for the SA-associated pathway in stimulating defense-related genes to viral pathogens (Love et al., 2007).
JA pathway is well known as a key regulator of plant defenses against viruses. In the present study, JA pathway-related genes (VmJAR1 and VmAOC) and their level of expression were high in moderately resistant cultivars but not in the susceptible ones. The late induction of VmJAR1 and VmAOC were observed in the expression patterns of the two genes. So, it could be concluded as the responsible factor for the susceptibility reaction for ULCV in black gram. Hence, the JA pathway activates the SAR pathway upon the viral infection. While comparing the SA and JA pathways-related genes and their level of expression, we found that the expression of both the genes and the associated pathways of the genes were increased following the viral infection, but their patterns at the time course were different. JA pathway-related genes showed upregulation at a later stage of infection, whereas SA pathway-related genes exhibited up regulation at an earlier stage and then were downregulated at a later stage. It suggests that the antagonism between SA and JA signaling pathways concurs with previous reports (Caarls et al., 2015). Comparable results have been stated in tobacco plants infected with the TMV (Zhu et al., 2014). ET pathway-related genes (VmAP2/ERF and VmSAM) also showed higher expression in moderately resistant cultivars than in susceptible one in later and earlier stages of infection. Higher and lower expression of VmAP2/ERF and VmSAM is accompanied by a higher and lesser accumulation of H2O2 in moderately resistant and susceptible cultivars, respectively. This reveals that ET has a predominant role in improving the resistance to ULCV in black gram. Similar results were obtained in Knoester et al. (2001) reports, who described the importance of ET in tobacco plants infected with the TMV. Collectively, the findings showed that JA, SA, and ET-linked genes used in this study could be used as molecular markers for examining the resistance of black gram genotypes to ULCV.
In conclusion, the resistance mechanism of the black gram cultivar to ULCV is roughly correlated to the ROS burst in VBN (Bg) 6 at the initial stage of infection. Moreover, the increased induction of SA, JA, and ET biosynthetic pathways is related to the increased resistance in cultivars like VBN (Bg) 6. In the moderately resistant cultivar, the reduction of ROS by the enzymatic antioxidant system is better than in the susceptible cultivar, and the MDA levels are estimated to quantify the oxidative damage, which was found to be less in VBN (Bg) 6. The reasons discussed above clearly elucidate the causes of VBN (Bg) 6, a moderate resistant cultivar, and CO 5, the susceptible one against ULCV. These reports complement our understanding of the resistance mechanism involved in ULCV and pave the way for the most conceivable research approaches and strategies for breeding increased resistance against ULCV.
Data Availability Statement
The raw data supporting the conclusions of this article will be made available by the authors, without undue reservation.
Author Contributions
Conceptualization and methodology: AK and NS. Experiments, data curation, and visualization: AK, MA, SS, GA, KV, MT, KK, and MS. Supervision: MP and NS. Writing—review and editing: AK, VS, and MA. All authors contributed to the article and approved the submitted version.
Funding
This article was supported by Science and Engineering Research Board (SERB), Department of Science and Technology (DST), Government of India (GOI) for the DST-SERB NPDF fellowship program (PDF/2016/003676).
Conflict of Interest
The authors declare that the research was conducted in the absence of any commercial or financial relationships that could be construed as a potential conflict of interest.
Publisher's Note
All claims expressed in this article are solely those of the authors and do not necessarily represent those of their affiliated organizations, or those of the publisher, the editors and the reviewers. Any product that may be evaluated in this article, or claim that may be made by its manufacturer, is not guaranteed or endorsed by the publisher.
Supplementary Material
The Supplementary Material for this article can be found online at: https://www.frontiersin.org/articles/10.3389/fsufs.2022.916795/full#supplementary-material
References
Adie, B. A., PaRez-PaRez, J. N., PaRez-PaRez, M. M., Godoy, M., Sanchez-Serrano, J.-J., Schmelz, E. A., et al. (2007). ABA is an essential signal for plant resistance to pathogens affecting JA biosynthesis and the activation of defenses in Arabidopsis. The Plant Cell 19, 1665–1681. doi: 10.1105/tpc.106.048041
Aebi, H.. (1984). “Catalase in vitro,” in Methods in Enzymology, ed. L. Packer. Academic Press Inc. p. 121–126. doi: 10.1016/S0076-6879(84)05016-3
Alazem, M., and Lin, N. S. (2015). Roles of plant hormones in the regulation of host–virus interactions. Molec. Plant Pathol. 16, 529–540. doi: 10.1111/mpp.12204
Arora, A., Sairam, R., and Srivastava, G. (2002). Oxidative stress and antioxidative system in plants. Curr. Sci. 82, 1227–1238.
Ashfaq, M., Khan, M. A., Javed, N., Mughal, S., Shahid, M., and Sahi, S. (2010). Effect of urdbean leaf crinkle virus infection on total soluble protein and antioxidant enzymes in blackgram plants. Pakistan J. Botany 42, 447–454.
Ashfaq, M., Khan, M. A., Mughal, S., Javed, N., Mukhtar, T., and Bashir, M. (2007). Evaluation of urdbean germplasm for resistance against urdbean leaf crinkle virus. Pakistan J. Botany 39, 2103–2111.
Ashfaq, M., Khan, M. A., Mukhtar, T., and Sahi, S. T. (2014). Role of mineral metabolism and some physiological factors in resistance against urdbean leaf crinkle virus in blackgram genotypes. Int. J. Agric. Biol. 16, 189–194.
Bashir, M., Ahmad, Z., and Ghafoor, A. (2005). Sources of genetic resistance in mungbean and blackgram against Urdbean leaf crinkle virus (ULCV). Pakistan J. Botany 37, 47–51.
Bashir, M., Mughal, S., and Malik, B. (1991). Assessment of yield losses due to leaf crinkle virus in urdbean, Vigna mungo (L) Hepper. Pakistan J. Botany 23, 140–142.
Beauchamp, C., and Fridovich, I. (1971). Superoxide dismutase: improved assays and an assay applicable to acrylamide gels. Analyt. Biochem. 44, 276–287. doi: 10.1016/0003-2697(71)90370-8
Bradford, M. M.. (1976). A rapid and sensitive method for the quantitation of microgram quantities of protein utilizing the principle of protein-dye binding. Analyt. Biochem. 72, 248–254. doi: 10.1016/0003-2697(76)90527-3
Caarls, L., Pieterse, C. M., and Van Wees, S. (2015). How salicylic acid takes transcriptional control over jasmonic acid signaling. Front. Plant Sci. 6, 1–11. doi: 10.3389/fpls.2015.00170
Chakraborty, N., and Basak, J. (2018). Molecular and biochemical characterization of mungbean yellow mosaic India virus resistance in leguminous host Vigna mungo. J. Plant Biochem. Biotechnol. 27, 318–330. doi: 10.1007/s13562-018-0441-2
Chance, B., and Maehly, A. (1955). “Assay of catalases and peroxidases,” in Methods in Enzymology. (New York, NY: Academic Press Inc), 764–775. doi: 10.1016/S0076-6879(55)02300-8
Chang, S. P., Jeon, Y. H., and Kim, Y. H. (2016). Defense-related responses in fruit of the nonhost chili pepper against Xanthomonas axonopodis pv. glycines Infection. Plant Pathol. J. 32, 311–320. doi: 10.5423/PPJ.OA.12.2015.0256
Derksen, H., Rampitsch, C., and Daayf, F. (2013). Signaling cross-talk in plant disease resistance. Plant Sci. 207, 79–87. doi: 10.1016/j.plantsci.2013.03.004
Díaz-Vivancos, P., Clemente-Moreno, M. J., Rubio, M., Olmos, E., García, J. A., Martínez-Gómez, P., et al. (2008). Alteration in the chloroplastic metabolism leads to ROS accumulation in pea plants in response to plum pox virus. J. Exper. Botany 59, 2147–2160. doi: 10.1093/jxb/ern082
Funayama-Noguchi, S., and Terashima, I. (2006). Effects of Eupatorium yellow vein virus infection on photosynthetic rate, chlorophyll content and chloroplast structure in leaves of Eupatorium makinoi during leaf development. Funct. Plant Biol. 33, 165–175. doi: 10.1071/FP05172
Gautam, N. K., Kumar, K., and Prasad, M. (2016). Leaf crinkle disease in urdbean (Vigna mungo L. Hepper): an overview on causal agent, vector and host. Protoplasma 253, 729–746. doi: 10.1007/s00709-015-0933-z
Gogoi, R., Singh, D., and Srivastava, K. (2001). Phenols as a biochemical basis of resistance in wheat against Karnal bunt. Plant Pathol. 50, 470–476. doi: 10.1046/j.1365-3059.2001.00583.x
Hančević, K., Radić, T., Pasković, I., and Urlić, B. (2018). Biochemical and physiological responses to long-term Citrus tristeza virus infection in Mexican lime plants. Plant Pathol. 67, 987–994. doi: 10.1111/ppa.12799
Hématy, K., Cherk, C., and Somerville, S. (2009). Host–pathogen warfare at the plant cell wall. Curr. Opin. Plant Biol. 12, 406–413. doi: 10.1016/j.pbi.2009.06.007
Indiastat (2020). Season-wise Area, Production and Productivity of Urad in India. Ministry of Agriculture and Farmers welfare, Govt. of India. Available online at: https://www.indiastat.com/ (accessed April 7, 2022).
Joshi, J. R., Burdman, S., Lipsky, A., Yariv, S., and Yedidia, I. (2016). Plant phenolic acids affect the virulence of Pectobacterium aroidearum and P. carotovorum ssp. brasiliense via quorum sensing regulation. Molec. Plant Pathol. 17, 487–500. doi: 10.1111/mpp.12295
Knoester, M., Linthorst, H., Bol, J., and Van Loon, L. (2001). Involvement of ethylene in lesion development and systemic acquired resistance in tobacco during the hypersensitive reaction to tobacco mosaic virus. Physiol. Molec. Plant Pathol. 59, 45–57. doi: 10.1006/pmpp.2001.0346
Lievens, L., Pollier, J., Goossens, A., Beyaert, R., and Staal, J. (2017). Abscisic acid as pathogen effector and immune regulator. Front. Plant Sci. 8, 1–15. doi: 10.3389/fpls.2017.00587
Lightenthaler, H.. (1987). “Chlorophylls and carotenoids: pigments of photosynthetic biomembranes,” in Methods in Enzymology, eds. L. Packer and R. Douce. (New York, NY: Academic Press Inc), 350–382. doi: 10.1016/0076-6879(87)48036-1
Loreto, F., and Velikova, V. (2001). Isoprene produced by leaves protects the photosynthetic apparatus against ozone damage, quenches ozone products, and reduces lipid peroxidation of cellular membranes. Plant Physiol. 127, 1781–1787. doi: 10.1104/pp.010497
Love, A. J., Laval, V., Geri, C., Laird, J., Tomos, A. D., Hooks, M. A., et al. (2007). Components of Arabidopsis defense-and ethylene-signaling pathways regulate susceptibility to Cauliflower mosaic virus by restricting long-distance movement. Molec. Plant-Microbe Interact. 20, 659–670. doi: 10.1094/MPMI-20-6-0659
Madamanchi, N. R., and Alscher, R. G. (1991). Metabolic bases for differences in sensitivity of two pea cultivars to sulfur dioxide. Plant Physiol. 97, 88–93. doi: 10.1104/pp.97.1.88
Madhumitha, B., Karthikeyan, A., Devi, G. P., Aiyanathan, K. E. A., and Sudha, M. (2020). Comparative evaluation of biochemical changes in the leaves of resistant and susceptible mungbean plants infected by Mungbean Yellow Mosaic Virus. Res. J. Biotechnol. 15, 47–54.
Markakis, E. A., Tjamos, S. E., Antoniou, P. P., Roussos, P. A., Paplomatas, E. J., and Tjamos, E. C. (2010). Phenolic responses of resistant and susceptible olive cultivars induced by defoliating and nondefoliating Verticillium dahliae pathotypes. Plant Dis. 94, 1156–1162. doi: 10.1094/PDIS-94-9-1156
Matern, U., Grimmig, B., and Kneusel, R. E. (1995). Plant cell wall reinforcement in the disease-resistance response: molecular composition and regulation. Canad. J. Botany 73, 511–517. doi: 10.1139/b95-290
Mittler, R., Herr, E. H., Orvar, B. L., Van Camp, W., Willekens, H., Inzé, D., et al. (1999). Transgenic tobacco plants with reduced capability to detoxify reactive oxygen intermediates are hyperresponsive to pathogen infection. Proc. Nat. Acad. Sci. USA 96, 14165–14170. doi: 10.1073/pnas.96.24.14165
Møller, I. M., Jensen, P. E., and Hansson, A. (2007). Oxidative modifications to cellular components in plants. Ann. Rev. Plant Biol. 58, 459–481. doi: 10.1146/annurev.arplant.58.032806.103946
Nakano, Y., and Asada, K. (1981). Hydrogen peroxide is scavenged by ascorbate-specific peroxidase in spinach chloroplasts. Plant Cell Physiol. 22, 867–880.
Navarro, L., Bari, R., Achard, P., Lisón, P., Nemri, A., Harberd, N. P., et al. (2008). DELLAs control plant immune responses by modulating the balance of jasmonic acid and salicylic acid signaling. Curr. Biol. 18, 650–655. doi: 10.1016/j.cub.2008.03.060
Ngadze, E., Icishahayo, D., Coutinho, T. A., and Van Der Waals, J. E. (2012). Role of polyphenol oxidase, peroxidase, phenylalanine ammonia lyase, chlorogenic acid, and total soluble phenols in resistance of potatoes to soft rot. Plant Dis. 96, 186–192. doi: 10.1094/PDIS-02-11-0149
Pál, M., Kovács, V., Vida, G., Szalai, G., and Janda, T. (2013). Changes induced by powdery mildew in the salicylic acid and polyamine contents and the antioxidant enzyme activities of wheat lines. Eur. J. Plant Pathol. 135, 35–47. doi: 10.1007/s10658-012-0063-9
Patwa, N., Chatterjee, C., and Basak, J. (2020). Differential responses of Phaseolus vulgaris cultivars following mungbean yellow mosaic India virus infection. Physiol. Molec. Biol. Plant. 26, 817–828. doi: 10.1007/s12298-019-00741-w
Reddy, C. R., Tonapi, V. A., Varanavasiappan, S., Navi, S., and Jayarajan, R. (2005). Influence of plant age on infection and symptomatological studies on urd bean leaf crinkle virus in urd bean (Vigna mungo). Int. J. Agric. Sci. 1, 1–6.
Sadasivam, S., and Manickam, A. (1996). Biochemical methods for agricultural sciences, eds. S. Sadasivam and A. Manickam. New Delhi: Wiley eastern Ltd. p. 184–185.
Sampol, B., Bota, J., Riera, D., Medrano, H., and Flexas, J. (2003). Analysis of the virus-induced inhibition of photosynthesis in malmsey grapevines. New Phytol. 160, 403–412. doi: 10.1046/j.1469-8137.2003.00882.x
Sewelam, N., Kazan, K., and Schenk, P. M. (2016). Global plant stress signaling: reactive oxygen species at the cross-road. Front. Plant Sci. 7, 1–21. doi: 10.3389/fpls.2016.00187
Shetty, N. P., Jørgensen, H. J. L., Jensen, J. D., Collinge, D. B., and Shetty, H. S. (2008). Roles of reactive oxygen species in interactions between plants and pathogens. Eur. J. Plant Pathol. 121, 267–280. doi: 10.1007/s10658-008-9302-5
Shetty, N. P., Mehrabi, R., Lütken, H., Haldrup, A., Kema, G. H., Collinge, D. B., et al. (2007). Role of hydrogen peroxide during the interaction between the hemibiotrophic fungal pathogen Septoria tritici and wheat. New Phytol. 174, 637–647. doi: 10.1111/j.1469-8137.2007.02026.x
Shirasu, K., Nakajima, H., Rajasekhar, V. K., Dixon, R. A., and Lamb, C. (1997). Salicylic acid potentiates an agonist-dependent gain control that amplifies pathogen signals in the activation of defense mechanisms. Plant Cell 9, 261–270. doi: 10.1105/tpc.9.2.261
Siddique, Z., Akhtar, K. P., Hameed, A., Sarwar, N., and Imran-Ul-Haq, Khan, S. A. (2014). Biochemical alterations in leaves of resistant and susceptible cotton genotypes infected systemically by cotton leaf curl Burewala virus. J. Plant Interact. 9, 702–711. doi: 10.1080/17429145.2014.905800
Singh, H. P., Kaur, S., Batish, D. R., and Kohli, R. K. (2014). Ferulic acid impairs rhizogenesis and root growth, and alters associated biochemical changes in mung bean (Vigna radiata) hypocotyls. J. Plant Interact. 9, 267–274. doi: 10.1080/17429145.2013.820360
Singh, R., Rai, N., Singh, M., Singh, S., and Srivastava, K. (2015). Selection of tomato genotypes resistant to tomato leaf curl virus disease using biochemical and physiological markers. J. Agric. Sci. 153, 646–655. doi: 10.1017/S0021859614000616
Sinha, R., Kumar, D., Datta, R., Hazra, S., Bhattacharyya, D., Mazumdar, A. B., et al. (2015). Integrated transcriptomic and proteomic analysis of Arabidopsis thaliana exposed to glutathione unravels its role in plant defense. Plant Cell, Tissue Organ Cult. 120, 975–988. doi: 10.1007/s11240-014-0651-9
Souza, P. F., Silva, F. D., Carvalho, F. E., Silveira, J. A., Vasconcelos, I. M., and Oliveira, J. T. (2017). Photosynthetic and biochemical mechanisms of an EMS-mutagenized cowpea associated with its resistance to cowpea severe mosaic virus. Plant Cell Rep. 36, 219–234. doi: 10.1007/s00299-016-2074-z
Srivastava, S., and Singh, A. K. (2010). Changes in catalase activity and total protein content in Urdbean [Vigna mungo (L.) Hepper] plants as a result of ULCV Infection. Indian J. Sci. Res. 1, 67–69.
Stewart, R. R., and Bewley, J. D. (1980). Lipid peroxidation associated with accelerated aging of soybean axes. Plant Physiol. 65, 245–248. doi: 10.1104/pp.65.2.245
Torres, M. A., Jones, J. D., and Dangl, J. L. (2006). Reactive oxygen species signaling in response to pathogens. Plant Physiol. 141, 373–378. doi: 10.1104/pp.106.079467
Van Loon, L. C., Rep, M., and Pieterse, C. M. (2006). Significance of inducible defense-related proteins in infected plants. Ann. Rev. Phytopathol. 44, 135–162. doi: 10.1146/annurev.phyto.44.070505.143425
Van Wees, S. C., De Swart, E. A., Van Pelt, J. A., Van Loon, L. C., and Pieterse, C. M. (2000). Enhancement of induced disease resistance by simultaneous activation of salicylate-and jasmonate-dependent defense pathways in Arabidopsis thaliana. Proc. Nat. Acad. Sci. USA. 97, 8711–8716. doi: 10.1073/pnas.130425197
Vijayan, P., Shockey, J., Lévesque, C. A., and Cook, R. J. (1998). A role for jasmonate in pathogen defense of Arabidopsis. Proc. Nat. Acad. Sci. USA. 95, 7209–7214. doi: 10.1073/pnas.95.12.7209
Wang, X., Han, F., Yang, M., Yang, P., and Shen, S. (2013). Exploring the response of rice (Oryza sativa) leaf to gibberellins: a proteomic strategy. Rice. 6, 1–12. doi: 10.1186/1939-8433-6-17
Zhang, X.-N., Wang, X.-R., Zhang, L., Ahammed, G. J., Li, Q.-Y., and Li, X. (2020). Epigallocatechin-3-gallate enhances tomato resistance to tobacco mosaic virus by modulating RBOH1-dependent H2O2 signaling. Plant Physiol. Biochem. 150, 263–269. doi: 10.1016/j.plaphy.2020.03.008
Zhou, Y., Peng, Y., Lei, J., Zou, L., Zheng, J., and Yu, J. (2004). Effects of potato virus YNTN infection on gas exchange and photosystem 2 function in leaves of Solanum tuberosum L. Photosynthetica 42, 417–423. doi: 10.1023/B:PHOT.0000046161.16215.dd
Keywords: biotic stress, black gram (vigna mungo L. hepper), pulses, urdbean leaf crinkle virus (UCLV), Legumes (Fabaceae)
Citation: Karthikeyan A, Akilan M, Samyuktha SM, Ariharasutharsan G, Shobhana VG, Veni K, Tamilzharasi M, Keerthivarman K, Sudha M, Pandiyan M and Senthil N (2022) Untangling the Physio-Chemical and Transcriptional Changes of Black Gram Cultivars After Infection With Urdbean Leaf Crinkle Virus. Front. Sustain. Food Syst. 6:916795. doi: 10.3389/fsufs.2022.916795
Received: 10 April 2022; Accepted: 09 June 2022;
Published: 14 July 2022.
Edited by:
Praveen Guleria, DAV University, IndiaReviewed by:
Phetole Mangena, University of Limpopo, South AfricaGayacharan, National Bureau of Plant Genetic Resources (ICAR), India
Copyright © 2022 Karthikeyan, Akilan, Samyuktha, Ariharasutharsan, Shobhana, Veni, Tamilzharasi, Keerthivarman, Sudha, Pandiyan and Senthil. This is an open-access article distributed under the terms of the Creative Commons Attribution License (CC BY). The use, distribution or reproduction in other forums is permitted, provided the original author(s) and the copyright owner(s) are credited and that the original publication in this journal is cited, in accordance with accepted academic practice. No use, distribution or reproduction is permitted which does not comply with these terms.
*Correspondence: Muthaiyan Pandiyan, bXBhbmRpeWFuOCYjeDAwMDQwO3lhaG9vLmNvLmlu; Natesan Senthil, c2VudGhpbF9uYXRlc2FuJiN4MDAwNDA7dG5hdS5hYy5pbg==