- 1Department of Food Science and Technology, The University of Haripur, Haripur, Pakistan
- 2Department of Biological Sciences, Faculty of Science, King Abdulaziz University, Jeddah, Saudi Arabia
- 3Biology Department, Faculty of Science, University of Tabuk, Tabuk, Saudi Arabia
- 4Department of Biology, College of Science, Imam Abdulrahman Bin Faisal University, Dammam, Saudi Arabia
- 5College of Life Science, Linyi University, Linyi, China
- 6Department of Agronomy, PMAS-Arid Agriculture University, Rawalpindi, Pakistan
- 7Department of Microbiology, PSGVP Mandal's S I Patil Arts, G B Patel Science and STKVS Commerce College, Shahada, India
- 8Hainan Key Laboratory for Sustainable Utilization of Tropical Bioresource, College of Tropical Crops, Hainan University, Haikou, China
- 9Department of Agronomy, The University of Haripur, Haripur, Pakistan
Geranyl butyrate and citronellyl butyrate esters are industrially important fruity flavors that are being used in food and as a fragrance in cosmetics. Previously terpenyl fruity flavors have been successfully synthesized in organic solvents using crude seedlings enzymes. The purpose of the current study was to standardize reaction parameters for the optimal synthesis of geranyl butyrate using the best chosen black cumin seedling lipase in an organic medium through direct esterification reactions. Geranyl butyrate and citronellyl butyrate esters were identified, quantified through gas chromatography, confirmed through GC-MS, and partiallypurified through the distillation process. Effect of organic solvents (acetonitrile, n-hexane, pentane, heptane, and toluene), alcohol and acid concentrations (0.125–0.3 M), temperature (20–50°C), incubation time (1–72 h), and enzyme concentrations (0.05–0.3 g) were studied on the synthesis of geranyl butyrate using black cumin seedling lipase. The highest conversion yields of ester (96%) were obtained when 0.25 M of geraniol and butyric acid were reacted at 37°C for 48 h in the presence of 0.25 g of crude seedling lipase enzyme in n-hexane. It was concluded that the germinated black cumin seedling lipase proved to be the best among the selected biocatalysts for the synthesis of geranyl butyrate in n-hexane.
Introduction
Terpenyl esters are industrially important compounds due to their application as aromatic compounds (Koskinen, 2012) and additives (Câmara et al., 2020). Short chain terpenyl esters are widely used as fragrance and flavoring compounds in foods (Xu et al., 2020), beverages (Bruner and Fox, 2020), cosmetics (Chandra et al., 2020), and pharmaceutical industries (Mehta et al., 2021). Traditionally flavored compounds have been obtained either from natural sources like plants or through chemical reactions. However, several problems are reported after using traditional techniques, such as low extraction efficiency, high production cost, and partial supply to meet global demands (Tian and Hua, 2021). The flavor esters are also synthesized by chemical catalysis, but this process requires a high reaction temperature and long incubation time which have a high economic cost (Wei et al., 2020). In addition, these esters are not considered “natural” products.
Geraniol is a monoterpene compound that is found in many essential oils of fruits, vegetables, and herbs including rose oil, citronella, lemongrass, lavender, and other aromatic plants. It is commonly used in the food, fragrance, and cosmetic industries. Terpene alcohols including geraniol have also been reported to have neutraceutical values including anti-oxidant, anti-inflammatory, and anti-cancer activities. Geraniol has also shown a wide spectrum of pharmacological effects and found effective against cancer of the lungs, breast, prostate, skin, kidney, colon, and liver (Wallington et al., 2016).
The application of enzymes including lipases from various sources to catalyze the synthesis of aromatic esters under mild reaction conditions has been widely described (Wei et al., 2020). Short chain terpenyl esters such as geranyl acetate, propionate, and butyl butyrate have been catalyzed through microbial lipases suspended in a non-aqueous medium (Mehta et al., 2021). Lipases from higher vegetative plants including fenugreek (Niknam et al., 2021), flax (Seralthan and Baskaran, 2020), coriander (Bashir and Safdar, 2020; El-Sayed et al., 2020), peanut (El-Sayed et al., 2020), wheat germ, papaya (Sani et al., 2021), and rapeseed (Liaquat and Apenten, 2000) have also been used for organic phase biocatalyst (OPB). The most important consideration in OPB is the cost of bio-catalyst and the limitation of organic solvents. In our previous study, different plant seedlings were assessed for their ability to catalyze the synthesis of eight unique aromatic esters in organic solvents. Black cumin (Nigella Sativa) seedling lipase proved to be the most promising biocatalyst due to its high catalytic efficiency, high stability, and broad specificity for biotransformation of several substrates (Adebayo-Tayo et al., 2021). The temperature and reaction medium generally influence the enzymatic activity, their stability and also enzymatic selectivity.
Recently our group has synthesized eight unique terpenyl flavor esters using various seedlings lipases in our lab. In the current research, the effect of process variables (enzyme concentration, reaction time, temperature, substrate concentration, and organic solvents) on geranyl butyrate synthesis are described to find out the best reaction conditions for industrially important flavor esters. Geranyl and citronellyl butyrate fruity flavors will be purified. Geranyl butyrate is vital short-chain terpenyl ester that has been authorized by Food and Drug Administration (FDA) as GRAS (Generally Recognized As Safe) flavoring agent for direct addition to food for human consumption (Nur, 2021).
The purpose of this study was to carry out a complete assessment for geranyl and citronellyl butyrate synthesis using crude black cumin seedling lipase through esterification reaction between geraniol, citronellol and butyric acid and to decide the best optimal condition for geranyl butyrate ester synthesis (Scheme 1).
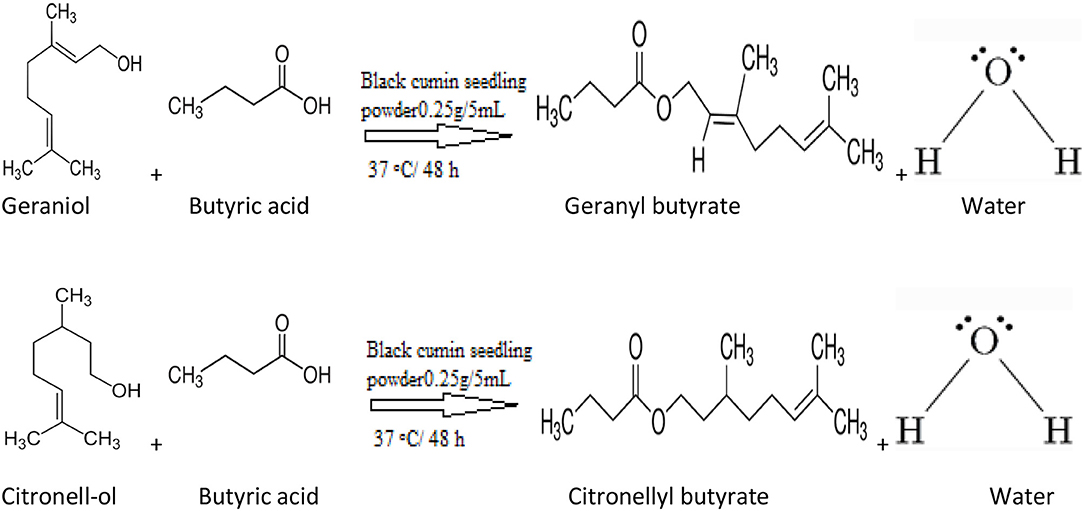
Scheme 1. Synthesis of terpenyl esters catalyzed by black cumin seedlings lipase in organic solvent via esterification.
Materials and Methods
Materials and Chemicals
Reagent grade chemicals, acetic, butyric, propionic, and iso-valaric acids, geraniol and citronellol alcohols, hexane, pentane, acetonitrile, toluene, heptane, sodium hypochlorite solution, and standard ester were obtained from Sigma-Aldrich Pvt Ltd, Fishers, Across, Supleco, and Honey well Pvt Ltd.
Seed Material and Germination
Black cumin seedling was acquired from Standard Seeds Supplier Pvt. Ltd Swabi, Pakistan. The sterilization of the whole seed was performed by dipping the seeds for 10 min in sodium hypochlorite solution (0.1%). First, a whole lot of black cumin seedling was washed with tap water and soaked in a dark incubator for 24 h at a temperature of 35°C. These 24 h were designated as 1st day of germination. Seeds were put on moist filter paper and placed in plastic trays having moist sand to facilitate germination. The average germination temperature was kept at 35°C. For the preparation of acetone powder, seedlings were collected on the 4th day of germination. As previously proved that lipase activity was at its peak during the 4th to 5th days of germination (Liaquat, 2011).
Acetone Powder Preparation From Seedlings
The method explained by Liaquat (2011) was used to prepare acetone powders. The batch of freshly germinated seedlings of black cumin was washed three times with distilled water then cut into small pieces using a pair of scissors and placed for 20 min in a refrigerator at 4°C. The seedlings were ground with the help of a blender and homogenized with five volumes of cold acetone (−4°C or less) for 1 min. The acetone extracts were then removed from the mixture by decanting the whole lot into the Buchner funnel, having Whatman No. 1 filter paper connected with vacuum filtration assembly. Further filtration of the powder was carried out for 4 more times by using five volumes of cold acetone and air dried under a hood for 10 h. The resultant grayish powders were stored in glass vials with screw cork at −20°C for further use as biocatalyst.
Direct Esterification Conditions
The procedure described by Liaquat and Apenten (2000) with minor modification was used to perform esterification. 1.25-Mmoles of acid and 1.25-Mmoles of alcohol were added with 250 mg acetone powder (5% w/v of total reaction volume) as an enzyme source into 10 mL screw capped vials. The final amount of reaction mixture was made to 5 ml with n-hexane. Synthesis was done in a water shaker (Model No: WN814 Germany) by shaking reaction vessels at the temperature of 37°C.
Percent alcohol conversion was calculated by removing 2 ml sample from the reacting mixture after 1, 10, 24, 48, and 72 h. These samples were then centrifuged at 13,000 rpm at 4°C temperature for 20 min in a refrigerated centrifuge machine (Model No: D3024R, Scilogex USA). About 1 ml aliquots were taken from the supernatant and kept at −20°C until used usually within 24 h. The frozen samples were allowed to warm to ambient temperature prior to gas chromatography analysis. All synthesis experiments were performed in triplicate using separate reaction vials.
Esters Analysis
The ester formed and remaining acid and alcohol in the reaction mixture were determined by using a gas chromatography system (Perkin Elmer Shelton, USA, Model Clarus 590) equipped with FID- detector having fused silica capillary column Elite-5MS PerkinElmer (30 m × 0.25 mm ID and film thickness 0.25 μm). Nitrogen was used as carrier gas (2 ml/min, split ratio 2:20). The temperature of the oven was kept at 50°C for 2 min and then increased to 210°C at a constant rate of 15°C per minute and held for 4 min. The oven temperature was further increased up to 280°C at the rate of 15°C/min and held for 5 min. The temperature of detectors and injectors was kept fixed at 260°C. The gas chromatography was connected to the computer system which recorded the peak areas and retention times in a chromatogram.
Ester Identification and Quantification
Alcohol, ester, and acid were identified on the basis of their retention times in GC-chromatograms and by comparing them with the result of standards. A calibration graph of geraniol was constructed. Different alcohol concentrations (0.125 to 0.3 M) were made by diluting in n-hexane and 0.2 μl of each sample was injected into gas chromatography. The injection from each vial was repeated for 3 times. Geraniol percentage conversion yield was calculated on the following formula (Equation 1).
Purification of Esters
This reaction mixture contains ester formed, remaining alcohol, and butyric acid in hexane (Liaquat, 2011; Abol Fotouh et al., 2016). Saturated sodium carbonate solution was prepared and mixed with the reaction mixture in a separatory funnel that formed two immiscible layers. Sodium carbonate solution (Na2CO3) has strong basic property (pH=10) which converted any un-dissociated butyric acid to form a salt (Equation) which settled down in the button layer (Equation 2).
The top layer containing ester, unreacted alcohol, and hexane was poured back into the receiving flask and was further purified by distillation using rotary evaporator at different temperatures (20–210°C) to separate hexane, alcohol, and ester. The separated compounds including the ester were identified through Gas chromatography and conform through GC-MS.
GC-MS Analysis
GC-MS (Agilent 7890A Series GC) has been described as one of the best techniques for the conformation of compounds. The GC-MS was equipped with a multimode injector and 5-MS column (30 m × 250 × 0.25 μm) and electron impact ionization.
About 1 μ of the sample was injected into the column by splitless mode using nitrogen as carrier gas at 1.5 ml/min. The ion source and quadruple temperatures were 230 and 150°C, respectively. Elution was performed as described above. Detection was done using full scan mode between 35 and 6,008 ha m/z and with gain factor 5 and the ester, identification was performed by comparing the fragmentation pattern of the synthesized ester with NIST 2011 MS Library.
Effect of Reaction Temperature
The effect of temperature on the synthesis of geranyl butyrate catalyzed by black cumin seedling lipase was investigated. The reaction mixture contained 250 mg of lipase, 0.25 M geraniol, and 0.25 M of butyric acid in n-hexane. The reaction mixture was incubated at a temperature of 20–50°C with an increasing interval of 5°C for 48 h. The geranyl butyrate ester formed and remaining alcohol in each case was determined using the gas chromatography system.
Effect of Solvents
The reaction of geraniol with butyric acid was examined using five organic solvents (acetonitrile, toluene, heptane, n-hexane, and pentane) of varying hydrophobicity. All other reaction conditions were as described above except for optimized temperature obtained from the previous experiment.
Effect of Reaction Time
The effect of reaction time for synthesis of geranyl butyrate ester was investigated under optimal conditions as described above while keeping the other parameters constant. At equimolar ratio of alcohols: acids at 0.25 M of each were reacted at optimal temperature for a period of 1, 9, 14, 48, and 72 h in a water shaker.
Effect of Substrate Concentration
The effect of increasing the concentration of one of the substrates while keeping the other constant was determined. Different geraniol concentrations (0.05, 0.1, 0.15, 0.2, 0.25, and 0.3 M) were reacted with a fixed 0.25 M concentration of butyric acid. In the opposite study, geraniol concentration was fixed at 0.25 M and butyric acid concentration was varied (0.125, 0.1, 0.75, 0.5, and 0.25 M) and 0.3 M. Best organic solvent was selected and optimized temperature obtained.
Effect of Enzyme Concentrations
The effect of increasing the concentration of enzyme was evaluated for the synthesis of geranyl butyrate ester while keeping the other parameters constant. Enzymes concentration of 0.05, 0.1, 0.15, 0.2, 0.25, and 0.3 g were reacted with optimal concentration of alcohol and acid. The ester formed in each case was determined by Gas chromatography.
Statistical Analysis
A factorial design was used for all the experiments with three replicates. Statistic 8.1 software was used for the statistical analysis of gas chromatography data. The average of the treatments were compared using Tukey's test (p ≤ 0.05).
Results
Synthesis, Identification, and Conformation of Geranyl and Citronellyl Butyrate
Gas chromatograms of geranyl and citronellyl butyrate after 48 h of reaction time are shown in Figure 1. GC-MS results of synthesized and library of geranyl butyrate and citronellyl butyrate are shown in Figures 2, 3, respectively. The identity of esters formed was confirmed from the GC-MS result. There was a close resemblance between fragmentation patterns of geranyl and citronellyl butyrate synthesized and present in the library database.
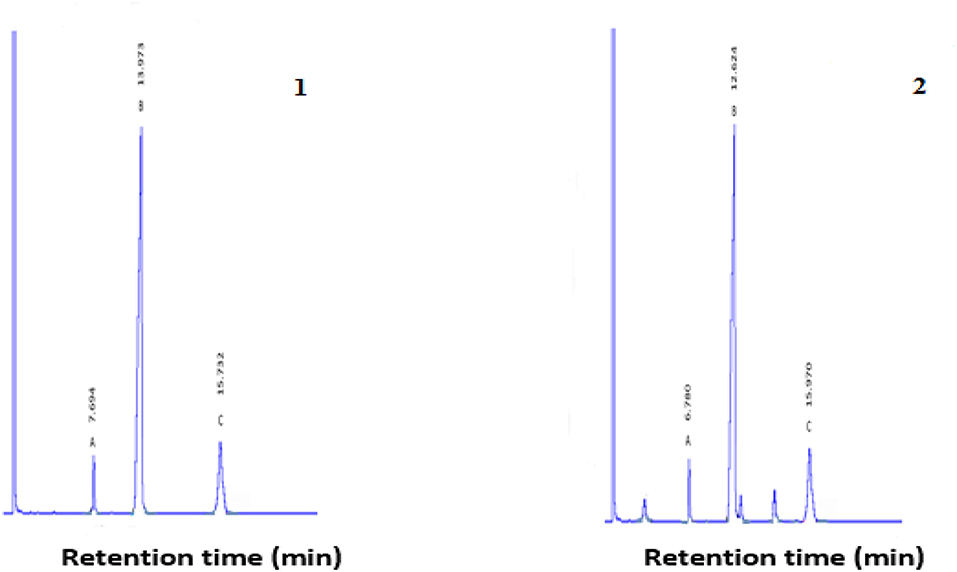
Figure 1. Gas chromatography profile of geraniol butyrate (1) and citronellyl butyrate (2) obtained from the esterification reaction after 48 h at 37°C. 1A: Remaining geraniol, 1B: Geranyl butyrate formed, and 1C: Remaining butyric acid. 2A: Remaining citronellol, 2B: Cotronellyl butyrate formed, and 2C: Remaining butyric acid.
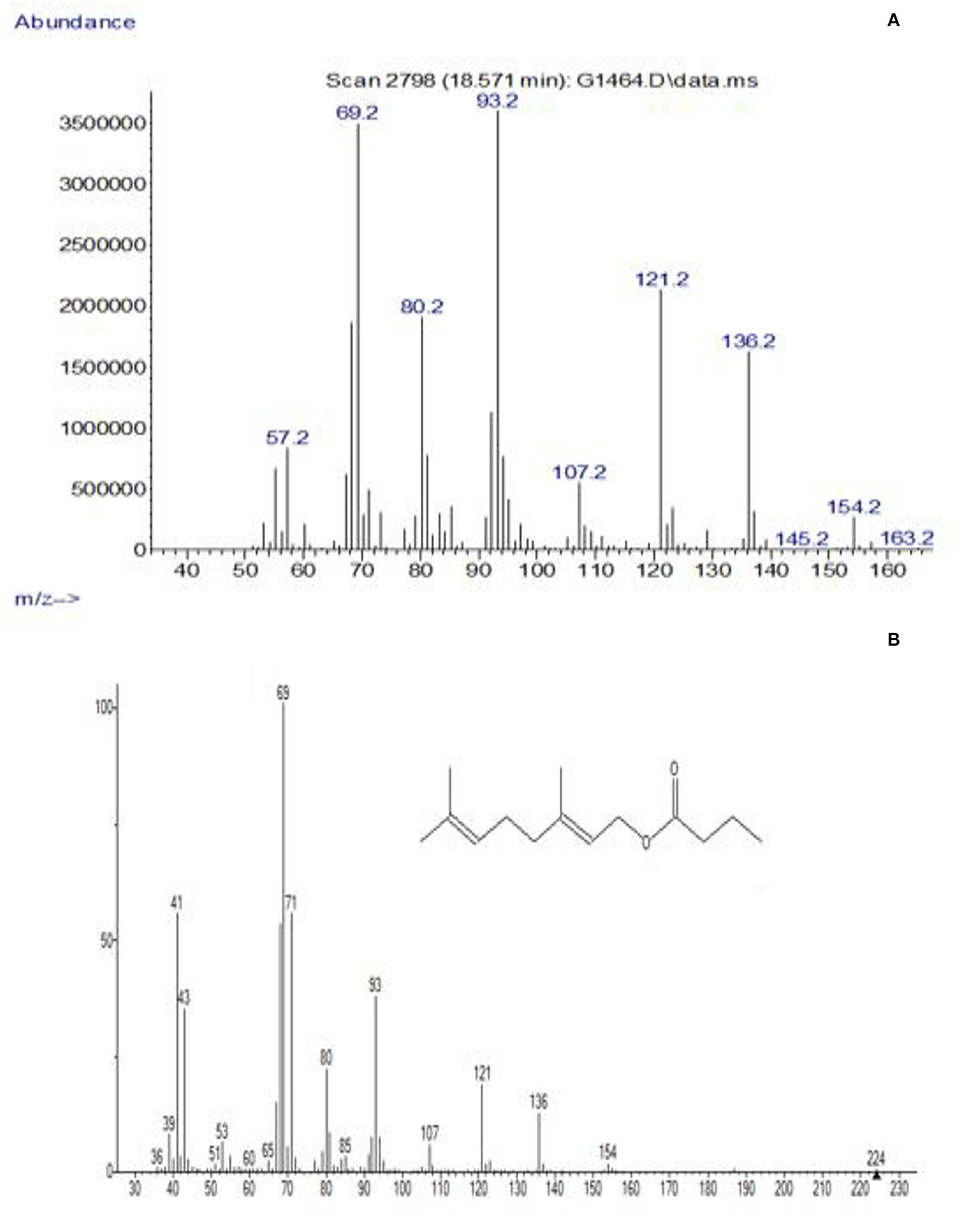
Figure 2. GC-MS spectra of peak (B) identified as geranyl butyrate and its confirmation in this study. (A) Synthesized, (B) MS-library.
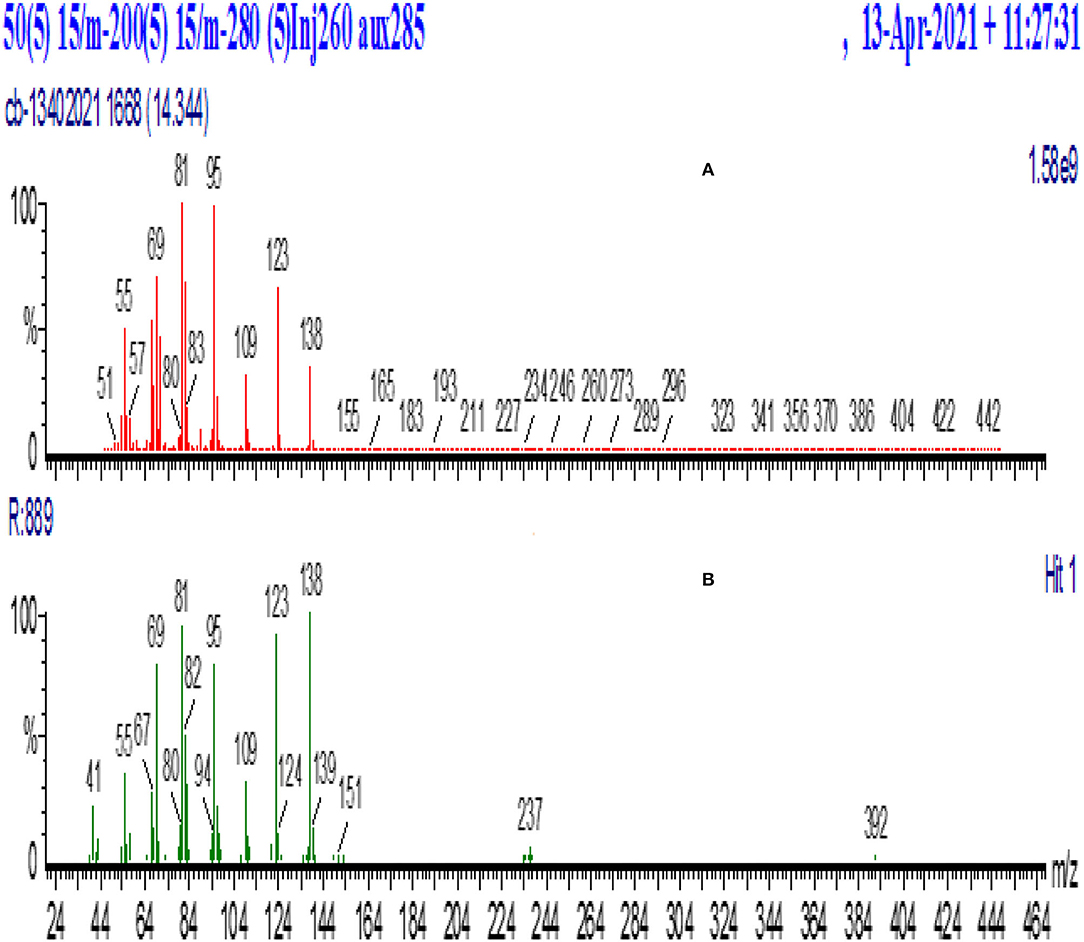
Figure 3. GC-MS spectra of peak (B) identified as citronellyl butyrate and its confirmation in this study. (A) Synthesized, (B) MS-library.
Purification of Synthesized Esters
Synthesized esters were purified from the reaction mixture first by adding saturated sodium carbonate solution (Na2CO3) to the reaction mixture obtained after 72 hr of reaction in a separating funnel. The sample mixture was separated into two immiscible layers. The less dense top layer had the ester while the bottom layer contained sodium carbonate along with water soluble components such as unreacted butyric acid. The experiment was repeated three times with sodium carbonate solution (Na2CO3). The top layer was removed by decanting the bottom waxy soap layer from the funnel.
About 50 ml of the sample of the top layer (containing ester, alcohol, and hexane) was taken in a sample flask of rotatory evaporator for purification through distillation. Silicon oil was used instead of water in the rotary bath. The sample was run at 10 rpm for 2 min at a temperature of 20–170°C with 5°C intervals. Hexane was evaporated at 35°C while the esters (geranyl butyrate and citronellyl butyrate) were evaporated at 55 and 80°C, respectively, and collected in receiving flask. Gas chromatograms of the partially purified geranyl and citronellyl butyrate were obtained at >80% purity as shown in Figure 4. GC-MS results of the synthesized and library of purified geranyl butyrate and citronellyl butyrate are shown in Figures 5, 6, respectively.
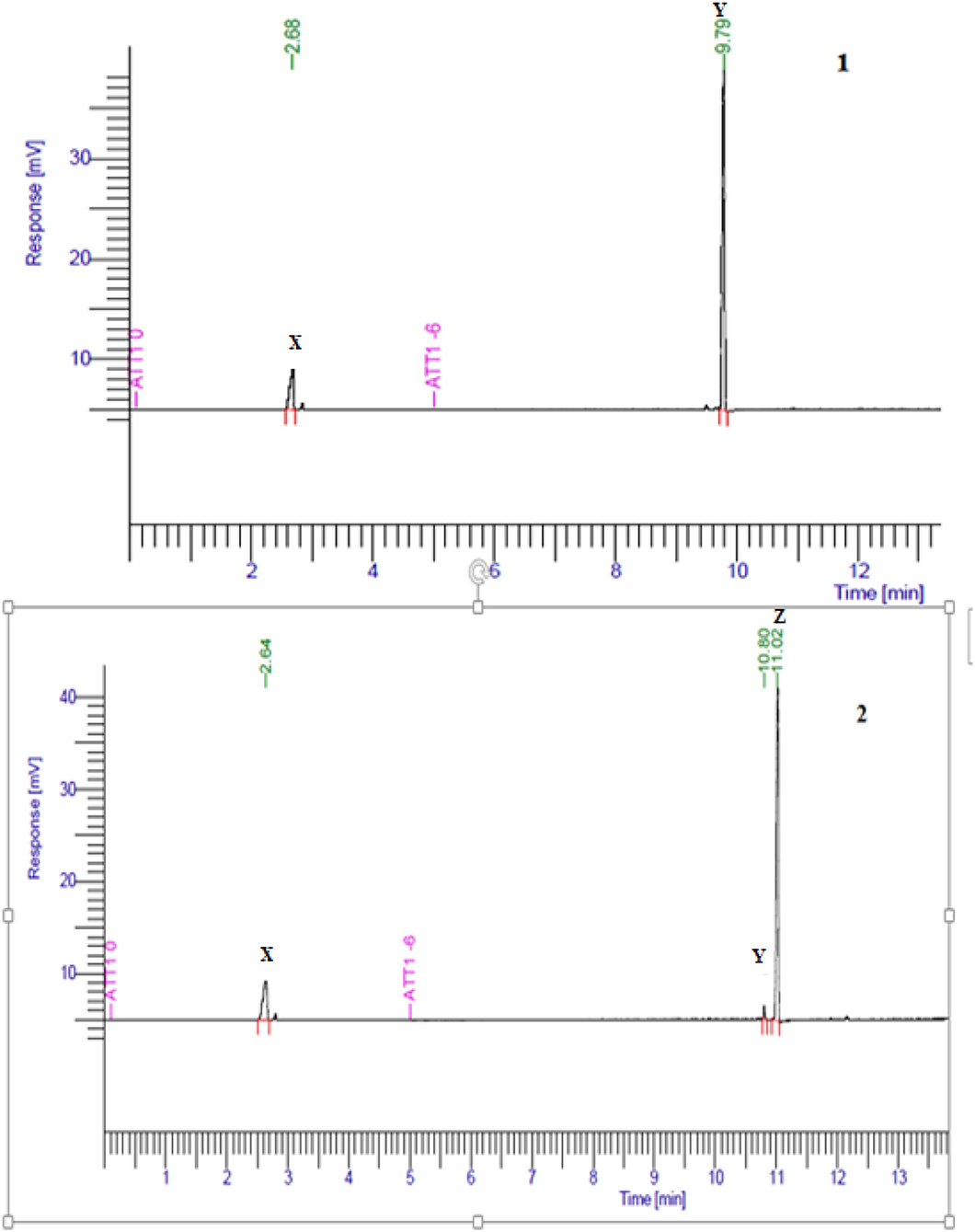
Figure 4. Gas chromatogram profile of purified esters of geranyl butyrate (1Y) and citronellyl butyrate (2Z) obtained after partial purification.
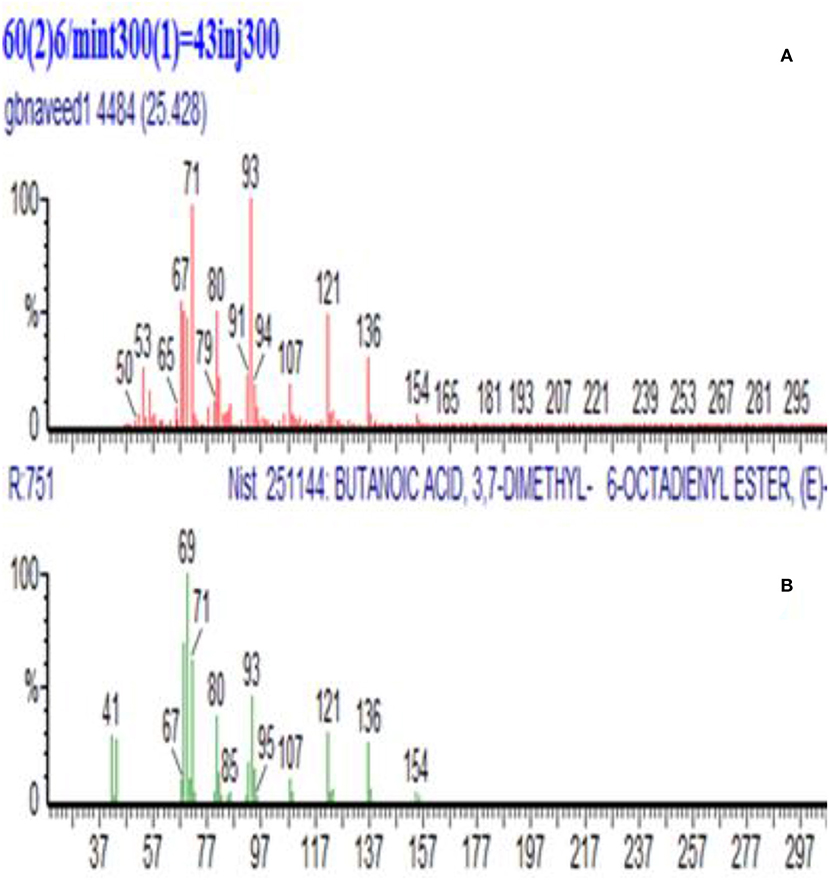
Figure 5. GC-MS spectra of partially purified geranyl butyrate peak (1Y) and its conformation in this study. (A) Synthesized geranyl butyrate. (B) GC-MS Library of geranyl butyrate.
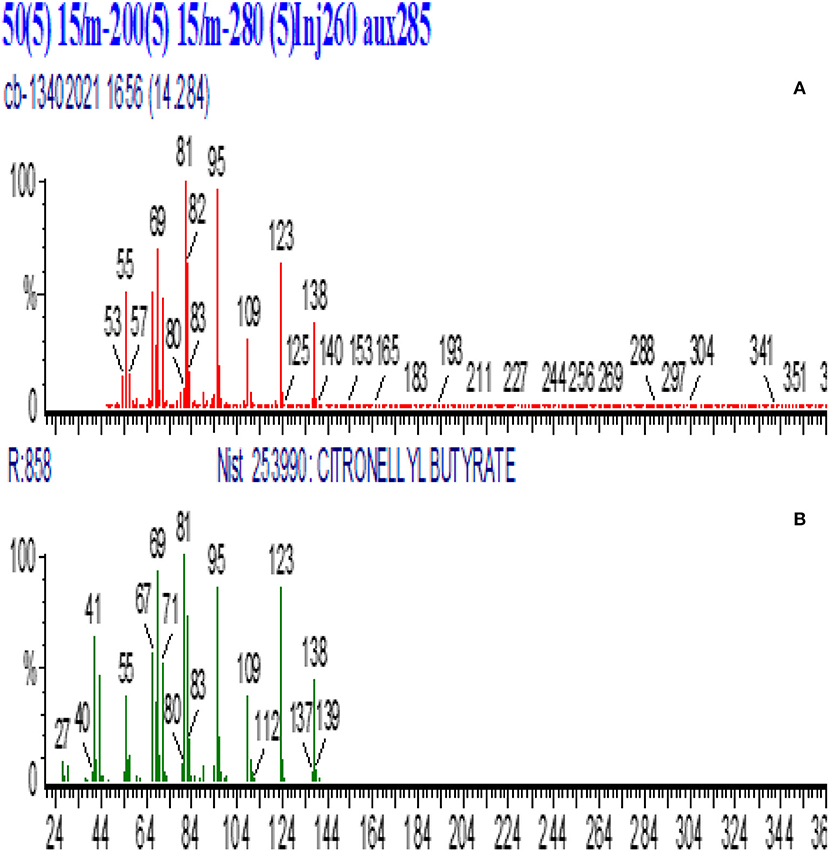
Figure 6. GC-MS spectra of partially purified citronellyl butyrate peak (2Z) and its conformation in this study. (A) Synthesized citronellyl butyrate, (B) GC-MS Library of citronellyl butyrate.
Optimization of Geranyl Butyrate
The design of biocatalysis systems involves several interacting variables; hence, a variable a time search for the optimal reaction conditions in the early stage of the investigation is not practical. The present study deals with the enzymatic synthesis of Geranyl butyrate (as a model compound) catalyzed by Black cumin seedlings (Scheme 1) in the organic solvent. The organic synthesis of geranyl butyrate was also investigated. In view of the results shown in our previous study in our lab, Black cumin was chosen as the best enzyme source. While keeping the total mass of the reaction mixture constant, the impact of several parameters on the bioreaction was carefully investigated and optimized through esterification reactions i.e., effects of different solvents, the amount of enzyme the substrate concentration, temperature, and incubation time. The identification of ester peak was carried out by comparing their retention times by matching their mass spectra with those of the NBS library of flavor and fragrances in the database system.
Effect of Reaction Time and Temperature on Geranyl Butyrate Synthesis
Time course studies were performed to determine the shortest time necessary to obtain high yields and thereby likely make the process cost effective. In addition, a reduction in reaction time decreases the substrate inventories required in the production process and decreases energy consumption and cost. The time scale for esters synthesis is presented in Figure 7, for geranyl butyrate yield synthesis. The reaction was followed by measuring the quantity of alcohol remaining. With increasing reaction time, however, increases in the percent conversions were observed (Figure 7).
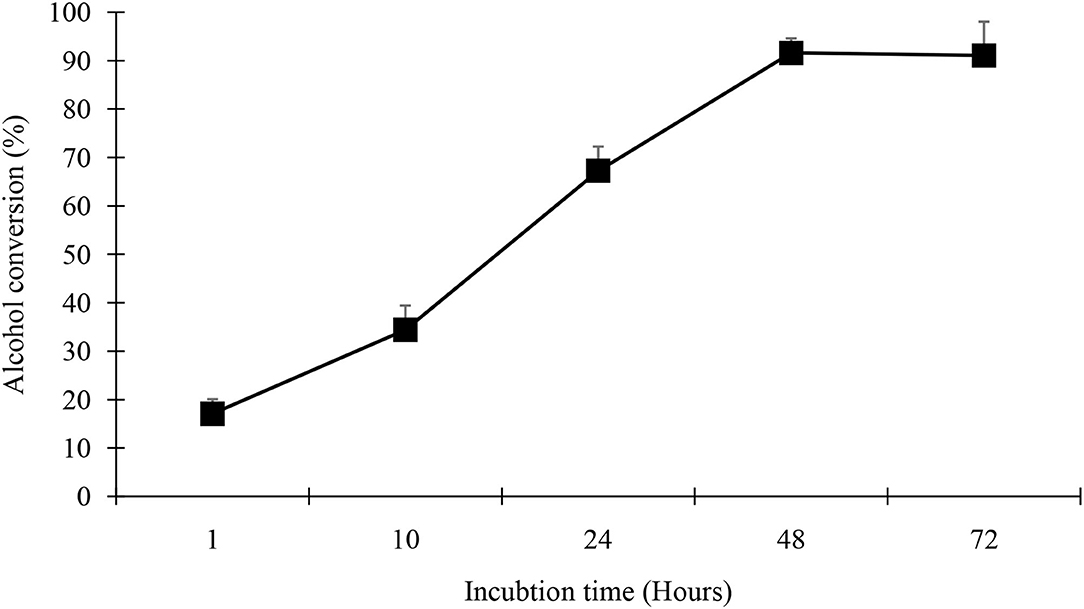
Figure 7. Time course of geranyl butyrate synthesis. The reaction mixture consisted of 0.25 M of butyric acid and 0.25 M of geraniol with 0.25 g of black cumin seedling acetone powder at 37°C. All experiment were done in triplicate and the values reported are means of three determination.
The time scale for geranyl butyrate synthesis is presented in Figure 7. With increasing the reaction time, a rise in the percent yield of ester was observed. Geranyl butyrate synthesis reached completion in 48 h at 37°C. For the baseline study, a product yield of 96% was observed with no further increase beyond 48 h. As 96 % of the geraniol was converted to ester after 48 h at 37°C it means that each of the substrates was esterified to the maximum level to form an ester.
The optimum temperature for a given enzymatic reaction depends on the enzyme source, type of immobilization (if any), and the pH of the reaction mixture. Reaction temperature affects OPB in three main ways; (1) stability of the biocatalyst may be affected (2) catalytic efficiency of the enzyme may be altered and, (3) there may be changes in the solubility of and viscosity of the substrates, as well as the reaction medium. Both (1) and (2) are directly related to the enzymes whereas changes in the physical properties of both the substrates and solvents could have a direct effect on the mass transfer of substrates to the enzyme active site in the reaction system. The effect of temperature on the synthesis of geranyl butyrate catalyzed by black cumin seedling lipase powder was investigated at 20–50°C using hexane as solvent. Figure 8 shows the dependency of temperature on the esterification of geranyl butyrate ester. As the reaction temperature increased, ester yield increased. A maximum yield of 94.5 % was obtained between temperature ranges of 20–50°C. It has been observed that geranyl butyrate ester can be produced in good yield at room temperatures (30–37°C).
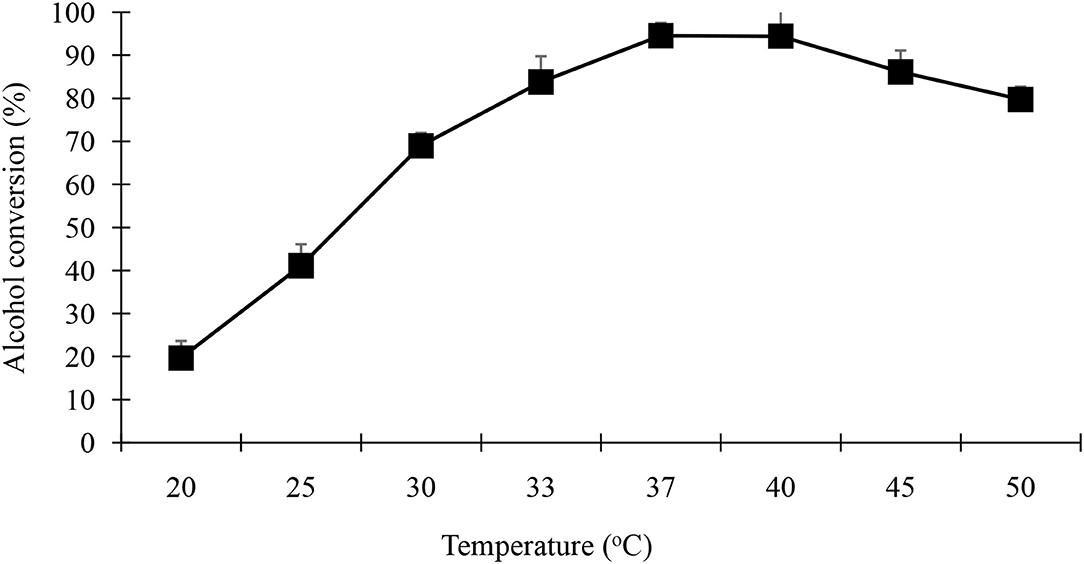
Figure 8. Effect of different temperature on the synthesis of geranyl butyrate ester. The reaction mixture consist of 0.25 M of each of butyric acid and geraniol at 37oC in the presence of 0.25 g of acetone powder from day 4 of germinated black cumin seedling in 5 mL of hexane.
Effect of Organic Medium on Ester Synthesis
Enzyme activity, stability, and selectivity are generally influenced by the reaction media. Because the OPB reaction involves substrate, products, enzyme, and the medium, it is obvious that optimization has to take all participants into account. To change the medium of the reaction, however, is by far the simplest way to change the process.
It is well-known that hydrophobicity of the organic solvent greatly influences the activity of biocatalysts acting in biphasic water/organic solvent systems Polar solvents undoubtedly replace the water on the protein surface and alter the tertiary structure, thereby possibly causing inactivation of the enzyme. Log P (the partition coefficient between water and 1-octanol) is generally used to describe solvent hydrophobicity. Therefore, the esterification yield of geranyl butyrate was tested with 5 solvents of Log P varying from −0.33 to 4.0. Figure 9 depicts the geranyl butyrate yield after 48 h at 37°C, using black cumin acetone powder in different solvents. Clearly, geranyl butyrate percent yield was maximum for non-polar and light polar solvents. A yield of 93.1 % was obtained in n-hexane followed by pentane (78.7%) after 48 h at 37°C.
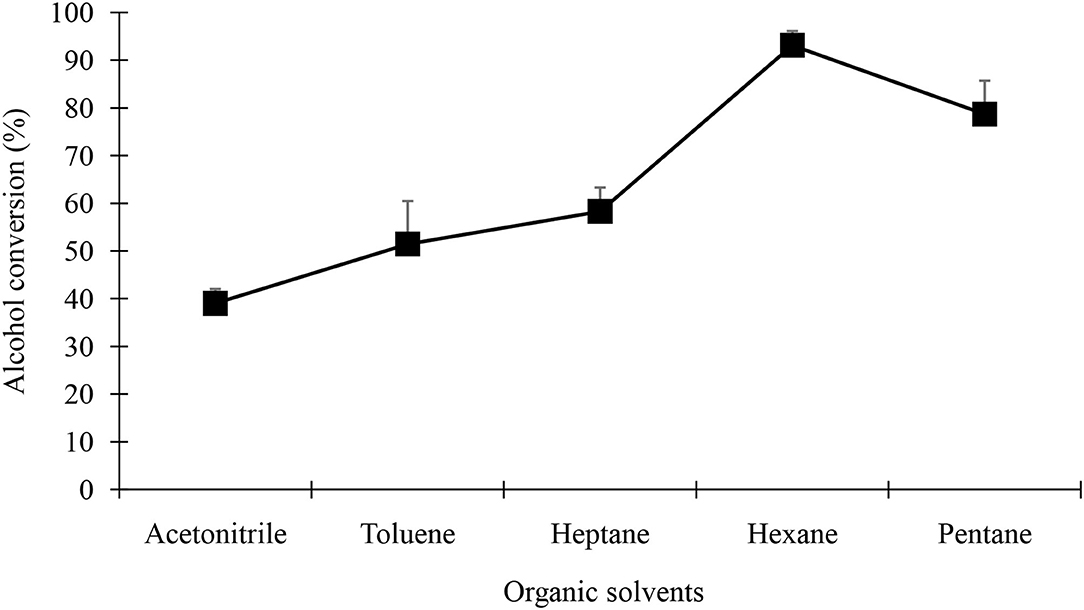
Figure 9. Effect of organic solvents for the synthesis of geranyl butyrate ester. The reaction mixture consist of 0.25 M of each of butyric acid and geraniol at 37oC in the presence of 0.25 g/5 ml of from black cumin seedling acetone powder of day 4th of germination.
Effect of Enzyme Concentration
The concentrations of lipase reported for OPB are often too high to meet industrial requirements. Enzyme concentrations of 83 and even 93% (w/w reactants) have been reported which are unsuitable commercially. Ten percentage (w/w) lipase is used, which is the most commonly used percentage for biochemical reactions. Figure 10 shows the effect of varying enzyme concentration on the yield of geranyl butyrate by direct esterification. As already noted, the rate of esterification was related to the amount of enzyme involved. The ester yield increased when the amount of enzyme was increased. However, the minimum concentration of black cumin seedling powder is necessary to achieve a maximum conversion yield of 94% [5% (w/v of total reaction volume or 250 mg). No significant increase in yield was observed for powder loading of more than 250 mg.
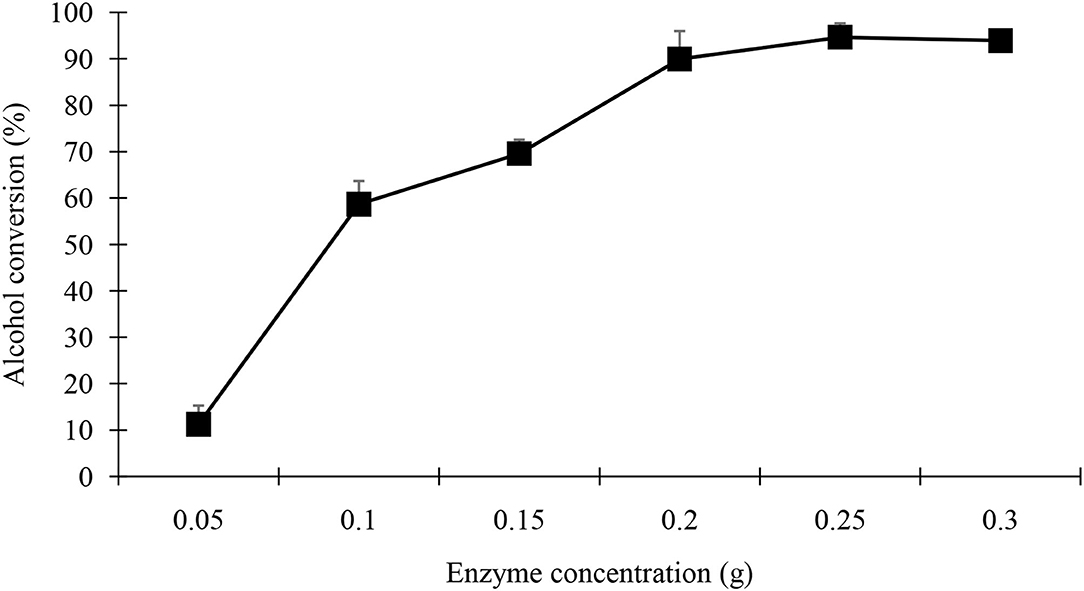
Figure 10. Effect of enzyme amount on the percent yield of geranyl butyrate ester. The reaction mixture consists of 0.25 M of butyric acid and 0.25 M of geraniol at 37oC in 5 ml of hexane containing different amount of black cumin seedling acetone powder for 48 h.
However, there was no significant increase in geranyl butyrate ester with seedling lipase loading more than 0.25 g. With the addition of a small amount of biocatalyst, the ester did not reach equilibrium within 48 h of incubation time.
Effect of Substrate Concentration
The concentration of substrate used in this study influenced the ester yield (Figures 11, 12). When the concentration of one of the substrates increased (0.125–0.3 M) whilst the other co-substrate was fixed at 0.25 M, product yield increased until the butyric acid or geraniol concentrations reached 0.25 M. Further increasing the concentration of acid or alcohol led to a linear or decreased synthesis.
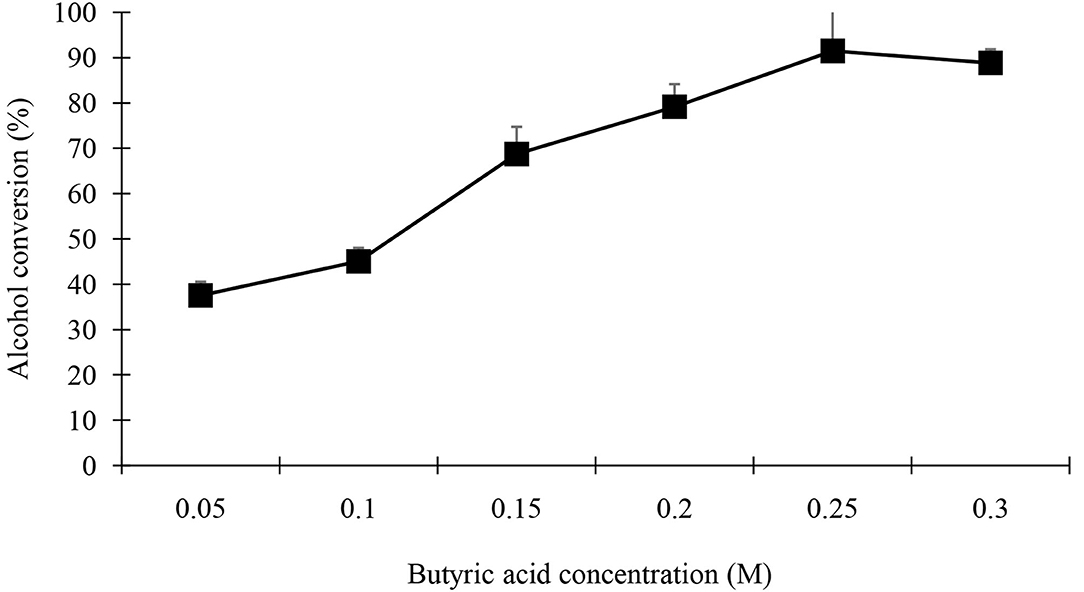
Figure 11. Effect of butyric acid concentration on synthesis of geranyl butyrate ester was investigated. Various concentrations of acid investigated while the concentration of alcohol was kept constant at 0.25 M. Reaction mixture containing 0.25 g of black cumin seedling acetone powder in 5 mL n-hexane. All reactions were carried out over a period of 48 h at 37°C.
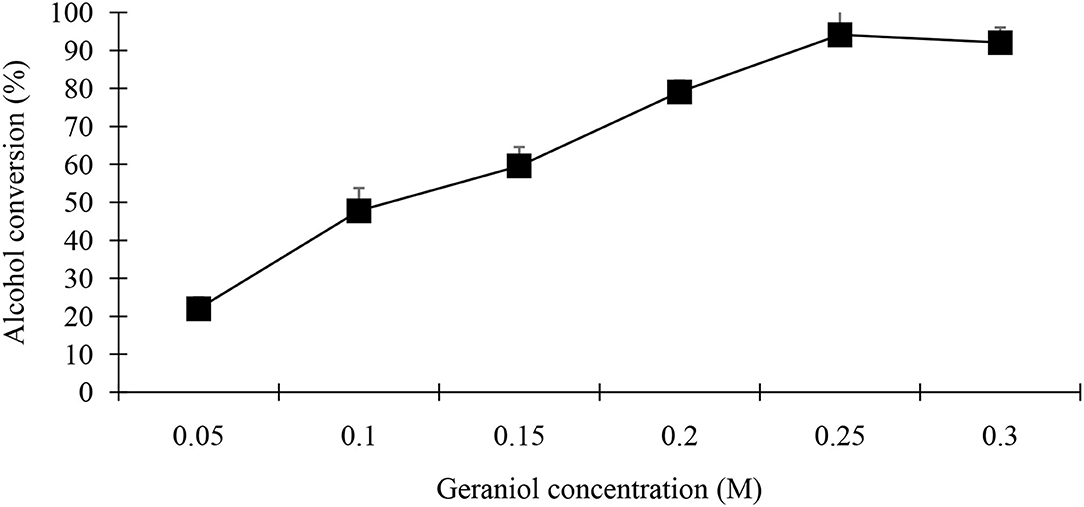
Figure 12. Effect of geraniol alcohol concentration on synthesis of geranyl butyrate ester was investigated. Various concentrations of geraniol were investigated while concentration of butyric acid was kept constant at 0.25 M. Reaction mixture contain 0.25 g of black cumin seedling acetone powder in 5 mL n-hexane. All reactions were carried out over a period of 48 h at 37°C.
Discussion
Synthesis, Identification, and Confirmation of Geranyl, and Citronellyl Butyrate
Gas chromatography is a precise technique for identifying, separating, and quantifying the compound in a complex mixture while GC-MS is the most accurate technique available for the separation and identification of aromatic compounds in complex mixtures. The combination of GC and MS leads to a fingerprint of the compound detected and therefore highly precise identification. The geranyl butyrate and citronellyl butyrate were purified by adding saturated sodium carbonate solution (Na2CO3) with a sample mixture in a separating funnel. The sodium carbonate solution (Na2CO3) has a strongly basic property (pH=10) which converted any un-dissociated butyric acid to its salt (Equation).
As shown in GC-MS results of synthesized and library of purified geranyl butyrate and citronellyl butyrate in Figures 5, 6, there was a close resemblance between fragmentations pattern of esters synthesized with those present in the library data base, which confirms the identity of synthesized esters. Approximately 80% of ester could be purified from the mixture which could be further enhanced by trying other purifying techniques.
Optimization of Geranyl Butyrate
In view of the result obtained in our previous study, the black cumin seedling was chosen as the best crude enzyme source. Geranyl butyrate was selected as a model compound catalyzed by black cumin seedling lipase (Scheme 1) and studied under various conditions.
Effect of Time
The percent yield of geranyl butyrate synthesis ester was increased with time and reached to a maximum of 96% in 48 h at 37°C (Figure 7). The synthesis of geranyl butyrate became quite surprising due to the fact that no attempts have been made to prevent water accumulation in the reaction mixture. This was a considerably quick response compared to the reaction of butyl caprylate ester catalyzed through purified microbial psychrotrophic Pseudomonas fluorescents P38 lipase which reached equilibrium after 96 h at 20°C with a final molar conversion of 75% (Kuddus, 2019). Product yield and reaction time are two critical endpoints in this research. A short incubation time decreases the overall reaction cost, substrate inventory, and also energy requirement. The time of incubation is dependent on factors such as enzyme activity, the quantity of biocatalyst used, co-substrates concentration, temperature, choice of organic solvent, and the degree of shaking, stirring, and sonication that affects mass transfer (Trinh et al., 2018; Esonye et al., 2019; Thawornprasert et al., 2021).
Effect of Temperature
Fruity flavor geranyl butyrate was obtained in a maximum yield of 94.5% between temperature ranges of 20–50°C (Figure 8). Results show that geranyl butyrate ester can be obtained in high conversion at room temperature. This feature can be expected to result in significant savings in energy costs for biotransformation. Compared to this result, the optimal temperature for the synthesis of ester through papaya (Carica papaya) lipase was 63°C (Anwar et al., 2018; Oladipo et al., 2020). While the synthesis of butyl caprylate with Pseudomonas P38 lipase was 20°C (Sose et al., 2017). Temperature above 40°C inactivates the lipase and resulted in decreased ester synthesis (Kutyła et al., 2021). In general, thermo-stability is achieved within an organic solvent if the lipase enzyme is inherently rigid or the environment prevents the enzyme from being flexible. The Enzymes are stable in a hydrophobic solvent having low specific activity at low temperature while high specific activity in the hydrophilic solvent may be associated with a highly flexible and relatively thermo-labile enzymes (Adamczak et al., 2020).
Effect of Organic Medium and Enzyme Concentration
As shown in Figure 9, the ester yield was more in non-polar and light polar solvents such as hexane which gave a percent yield of 93.1 %. It has been previously reported that hydrophobic solvents are associated with higher ester yield (Priyanka et al., 2019; Baek et al., 2020; Zago et al., 2021). Hydrophilic solvents such as acetonitrile, tetra-hydro furan, and dioxane have a layer that adsorbs water from biocatalyst that resulting in the inactivation of biocatalyst (Zongrui et al., 2018; El Rassi et al., 2020). In contrast, solvent like pentane, hexane, and heptane are non-polar solvents that preserve the water layer that surrounds the enzyme particles and therefore helps to maintain enzyme activity resulting in high product yields (Vergara-Fernández et al., 2018; Zongrui et al., 2018; Al-Shawawreh, 2021).
The cost of the biocatalyst is an economical factor for every biotransformation reaction. During the current study, a maximum yield of 94% was obtained with 250 mg of black cumin seedlings. An increase in enzyme concentration beyond that level was not beneficial in terms of conversion. The possible reason for this could be that the extra enzyme molecules (active sites) are not exposed to the substrate and are only present inside the bulk of the powder with zero involvement. For industrial uses, the demand for lipase enzyme is too high for the preparation of pharmaceutical and cosmetic products (Amini et al., 2017; Sarmah et al., 2018). The optimal concentration of biocatalyst (0.2 g) has been reported for P38 lipase (Yue et al., 2016; Sose et al., 2017; Zhang et al., 2021).
Effect of Substrate Concentration
The goal is to increase the percent yield of geranyl butyrate flavor ester. The substrate concentration used in this research influenced the conversion of ester yield. When the concentration of one of the substrates increased (0.125–0.3 M) whilst the other co-substrate was fixed at 0.25 M, product yield increased until the 0.25 M. When the concentration of substrates increase beyond the specific level of 0.25 M, the loss of effectiveness occurred which might be due to the modification in the polarity of the medium (Liaquat et al., 2015). The loss in synthetics activity at high alcohol concentrations has been reported due to the dehydrating effect on the lipase stability of black cumin seedlings (Guimarães et al., 2019). Alcohols have been shown to inhibit lipases from Bacillus licheniformis (Baltaci et al., 2020), M. miehei (Shahedi et al., 2019), Candida Antarctica (Monteiro et al., 2021), Candida cylinracea (Nacer and Chahra, 2021), and goat pregastric lipase (Clark and García, 2017). Short chain alcohols like methanol, ethanol, and butanol have been reported to have inactivating effect compared to long carbon chain alcohols such as geraniol and citronellol likewise the inactivating effects of acid co-substrates are related to their pKa value which increases (acidity decreases) with chain length (Liaquat et al., 2015). A high concentration of butyric acid was shown susceptible during organic phase biocatalyst using various lipases (Suo et al., 2018; Feizi et al., 2021) but not with acetic acid (Liaquat, 2011; Shahid et al., 2018; Reinoso and Boldrini, 2020).
Effect of Enzyme Concentration
The concentrations of lipase reported for OPB are often too high to meet industrial requirements. Enzyme concentrations of 83 and even 93% (w/w reactants) have been reported which are unsuitable commercially. Ten percentage (w/w) lipase is used, which is the most commonly used percentage for biochemical reactions. Figure 10 shows the effect of varying enzyme concentration on the yield of geranyl butyrate by direct esterification. As already noted, the rate of esterification was related to the amount of enzyme involved. Figure shows the ester yield increased when the amount of enzyme was increased. No significant further increase in ester with seedling lipase loading more than 0.25 g (5% w/v) was observed. With the addition of a small amount of biocatalyst, the ester did not reach equilibrium within 48 h of incubation time.
Conclusion
This research shows that geranyl butyrate can be synthesized in quantitative yields (93%) with black cumin seedling lipase (0.25 g/5 ml) and substrate concentration of 0.25 M suspended in n-hexane under normal reaction conditions (37°C for 48 h). Ester synthesis depends on the characteristics of organic solvents, types of biocatalyst, and substrate used. The rate of enzymes remains a chief consideration in organic phase biocatalyst (OPB) because purified enzymes are costly therefore, the crude seedling powder is inexpensive and well-suited for the synthesis of terpenyl esters. In addition, the influence of the quantity of enzyme is crucial and the acetone powders from black cumin seedlings can be used in non-polar solvents over a temperature range of 35–40°C without stringent moisture control. These results suggest the possibility of using 4th-day germinated black cumin seedling acetone powders at ambient temperature for biocatalysis. This is particularly more important as it is commercially economical to use at a temperature of 35–40°C. Ambient temperature OPB in the future might have applications in the preparation of heat-sensitive and high value products. The current data is highly encouraging for pilot scale studies.
Data Availability Statement
The raw data supporting the conclusions of this article will be made available by the authors, without undue reservation.
Author Contributions
NH and ML designed the experiments. NH designed and carried out the experiments and wrote the manuscript. HA, YA, SA, BA, NA, and SS participated and analyzed the data from the experiments. MA, RS, and SF reviewed the manuscript. All authors contributed to the article and approved the submitted version.
Funding
This work was supported by HEC (Pakistan) NRPU Project No: 10378.
Conflict of Interest
The authors declare that the research was conducted in the absence of any commercial or financial relationships that could be construed as a potential conflict of interest.
Publisher's Note
All claims expressed in this article are solely those of the authors and do not necessarily represent those of their affiliated organizations, or those of the publisher, the editors and the reviewers. Any product that may be evaluated in this article, or claim that may be made by its manufacturer, is not guaranteed or endorsed by the publisher.
Acknowledgments
The author is thankful HEC-Pakistan for sponsoring the project.
References
Abol Fotouh, D. M., Bayoumi, R. A., and Hassan, M. A. (2016). Production of thermoalkaliphilic lipase from Geobacillus thermoleovorans DA2 and application in leather industry. Enzyme Res. 2016, 9034364. doi: 10.1155/2016/9034364
Adamczak, A., Ozarowski, M., and Karpiński, T. M. (2020). Curcumin, a natural antimicrobial agent with strain-specific activity. Pharmaceuticals. 13, 153. doi: 10.3390/ph13070153
Adebayo-Tayo, B. C., Briggs-Kamara, A. I., and Moshood, A. (2021). Phytochemical composition, antioxidant, antimicrobial potential and GC-MS analysis of crude and partitioned fractions of nigella sativa seed extract. Acta Microbiol. Bulg. 37, 34–45.
Al-Shawawreh, M. F. M. (2021). Isolation of endogenous fungal isolate producing extracellular lipase with potential in olive oil hydrolysis. Rev. Int. Geogr. Educ. Online 11, 1493–1516. doi: 10.48047/rigeo.11.04.140
Amini, Z., Ong, H. C., Harrison, M. D., Kusumo, F., Mazaheri, H., and Ilham, Z. (2017). Biodiesel production by lipase-catalyzed transesterification of Ocimum basilicum L. (sweet basil) seed oil. Energy Conv. Manage. 132, 82–90. doi: 10.1016/j.enconman.2016.11.017
Anwar, M., Rasul, M. G., and Ashwath, N. (2018). Production optimization and quality assessment of papaya (Carica papaya) biodiesel with response surface methodology. Energy Conv. Manage. 156, 103–112. doi: 10.1016/j.enconman.2017.11.004
Baek, Y., Lee, J., Son, J., Lee, T., Sobhan, A., Lee, J., et al. (2020). Enzymatic synthesis of formate ester through immobilized lipase and its reuse. Polymers 12, 1802. doi: 10.3390/polym12081802
Baltaci, M. O., Orak, T., Taskin, M., Adiguzel, A., and Ozkan, H. (2020). Enhancement of amylase and lipase production from Bacillus licheniformis 016 using waste chicken feathers as peptone source. Waste Biomass Valorizat. 11, 1809–1819. doi: 10.1007/s12649-018-0468-6
Bashir, S., and Safdar, A. (2020). “Coriander seeds: ethno-medicinal, phytochemical and pharmacological profile,” in Science of Spices and Culinary Herbs-Latest Laboratory, Pre-clinical, and Clinical Studies, Vol. 2 (Bentham Science), 39–64. doi: 10.2174/9789811441493120020005
Bruner, J., and Fox, G. (2020). Novel non-Cerevisiae saccharomyces yeast species used in beer and alcoholic beverage fermentations. Fermentation 6, 116. doi: 10.3390/fermentation6040116
Câmara, J. S., Lourenço, S., Silva, C., Lopes, A., Andrade, C., and Perestrelo, R. (2020). Exploring the potential of wine industry by-products as source of additives to improve the quality of aquafeed. Microchem. J. 155, 104758. doi: 10.1016/j.microc.2020.104758
Chandra, P., Singh, R., and Arora, P. K. (2020). Microbial lipases and their industrial applications: a comprehensive review. Microbiol. Cell Fact. 19, 1–42. doi: 10.1186/s12934-020-01428-8
Clark, S., and García, M. B. M. (2017). A 100-year review: Advances in goat milk research. J. Dairy Sci. 100, 10026–10044. doi: 10.3168/jds.2017-13287
El Rassi, Z., Lee, A. L., and Horváth, C. (2020). Reversed-phase and hydrophobic interaction chromatography of peptides and proteins. Separat. Proc. Biotechnol. 9, 447–494. doi: 10.1201/9781003066392-18
El-Sayed, S. M., Salama, H. H., and El-Sayed, M. M. (2020). Function processed cheese sauce fortified with peanut butter. J. Food Proc. Preserv. 44, e14656. doi: 10.1111/jfpp.14656
Esonye, C., Onukwuli, O. D., and Ofoefule, A. U. (2019). Optimization of methyl ester production from prunus amygdalus seed oil using response surface methodology and artificial neural networks. Renew. Energy 130, 61–72. doi: 10.1016/j.renene.2018.06.036
Feizi, H., Moradi, R., Pourghasemian, N., and Sahabi, H. (2021). Assessing saffron response to salinity stress and alleviating potential of gamma amino butyric acid, salicylic acid and vermicompost extract on salt damage. South Afr. J. Bot. 141, 330–343. doi: 10.1016/j.sajb.2021.04.036
Guimarães, A. C., Meireles, L. M., Lemos, M. F., Guimarães, M. C. C., Endringer, D. C., Fronza, M., et al. (2019). Antibacterial activity of terpenes and terpenoids present in essential oils. Molecules 24, 2471. doi: 10.3390/molecules24132471
Koskinen, A. M. (2012). Asymmetric Synthesis of Natural Products. John Wiley & Sons. doi: 10.1002/9781118347300
Kuddus, M. (2019). “Introduction to food enzymes,” in Enzymes in Food Biotechnology (Academic Press), 1–18. doi: 10.1016/B978-0-12-813280-7.00001-3
Kutyła, M., Trytek, M., Buczek, K., Tomaszewska, E., and Muszyński, S. (2021). Biomass of a psychrophilic fungus as a biocatalyst for efficient direct esterification of citronellol. BioEnergy Res. 15, 399–411. doi: 10.1007/s12155-021-10289-x
Liaquat, M. (2011). Optimized synthesis of (Z)-3-hexen-1-yl caproate using germinated rapeseed lipase in organic solvent. J. Mol. Catal. B Enzymat. 68, 59–65. doi: 10.1016/j.molcatb.2010.09.011
Liaquat, M., and Apenten, R. K. O. (2000). Synthesis of low molecular weight flavor esters using plant seedling lipases in organic media. J. Food Sci. 65, 295–299. doi: 10.1111/j.1365-2621.2000.tb15996.x
Liaquat, M., Mehmood, T., Khan, S.-U., Ahmed, Z., Saeed, M., Aslam, S., et al. (2015). Parameters affecting the synthesis of (Z)-3-hexen-1-yl acetate by transesterifacation in organic solvent. J. Chem. Soc. Pakist. 37, 323–334.
Mehta, A., Guleria, S., Sharma, R., and Gupta, R. (2021). “The lipases and their applications with emphasis on food industry,” in Microbial Biotechnology in Food and Health. ed R. Ray (Elsevier), 143–164. doi: 10.1016/B978-0-12-819813-1.00006-2
Monteiro, R. R., Virgen-Ortiz, J. J., Berenguer-Murcia, Á., Da Rocha, T. N., Dos Santos, J. C., Alcántara, A. R., et al. (2021). Biotechnological relevance of the lipase A from Candida antarctica. Catal. Today 362, 141–154. doi: 10.1016/j.cattod.2020.03.026
Nacer, R., and Chahra, B.-H. (2021). Physical and chemical study of coated Candida cylindracea with sorbitan esters. Res. J. Chem. Environ. 25, 10. doi: 10.25303/2510rjce4348
Niknam, R., Kiani, H., Mousavi, Z. E., and Mousavi, M. (2021). “Extraction, detection, and characterization of various chemical components of Trigonella foenum-graecum L. (Fenugreek) known as a valuable seed in agriculture,” in Fenugreek, eds M. Naeem, T. Aftab, M. M. A. Khan (Singapore: Springer), 189–217. doi: 10.1007/978-981-16-1197-1_9
Nur, Ç. (2021). Chemical fingerprinting of the geranium (Pelargonium graveolens) essential oil by using FTIR, raman and GC-MS techniques. Euro. J. Sci. Technol. 25, 810–814. doi: 10.31590/ejosat.969661
Oladipo, B., Ojumu, T. V., Latinwo, L. M., and Betiku, E. (2020). Pawpaw (Carica papaya) peel waste as a novel green heterogeneous catalyst for moringa oil methyl esters synthesis: process optimization and kinetic study. Energies 13, 5834. doi: 10.3390/en13215834
Priyanka, P., Tan, Y., Kinsella, G. K., Henehan, G. T., and Ryan, B. J. (2019). Solvent stable microbial lipases: current understanding and biotechnological applications. Biotechnol. Lett. 41, 203–220. doi: 10.1007/s10529-018-02633-7
Reinoso, D. M., and Boldrini, D. E. (2020). Kinetic study of fuel bio-additive synthesis from glycerol esterification with acetic acid over acid polymeric resin as catalyst. Fuel 264, 116879. doi: 10.1016/j.fuel.2019.116879
Sani, M. S. A., Bakar, J., Rahman, R. A., and Abas, F. (2021). “Effect of temperature on antibacterial activity and fatty acid methyl esters of carica papaya seed extract,” in Multifaceted Protocols in Biotechnology, Vol. 2 ed A. Amid (Cham: Springer), 117–132. doi: 10.1007/978-3-030-75579-9_8
Sarmah, N., Revathi, D., Sheelu, G., Yamuna Rani, K., Sridhar, S., Mehtab, V., et al. (2018). Recent advances on sources and industrial applications of lipases. Biotechnol. Prog. 34, 5–28. doi: 10.1002/btpr.2581
Seralthan, K., and Baskaran, H. (2020). A short voyage towards flaxseed. J. Pharm. Sci. Res. 12, 631–634.
Shahedi, M., Yousefi, M., Habibi, Z., Mohammadi, M., and As' Habi, M. A. (2019). Co-immobilization of Rhizomucor miehei lipase and Candida antarctica lipase B and optimization of biocatalytic biodiesel production from palm oil using response surface methodology. Renew. Energy 141, 847–857. doi: 10.1016/j.renene.2019.04.042
Shahid, A., Jamal, Y., Khan, S. J., Khan, J. A., and Boulanger, B. (2018). Esterification reaction kinetics of acetic and oleic acids with ethanol in the presence of amberlyst 15. Arab. J. Sci. Eng. 43, 5701–5709. doi: 10.1007/s13369-017-2927-y
Sose, M. T., Bansode, S. R., and Rathod, V. K. (2017). Solvent free lipase catalyzed synthesis of butyl caprylate. J. Chem. Sci. 129, 1755–1760. doi: 10.1007/s12039-017-1391-2
Suo, Y., Fu, H., Ren, M., Liao, Z., Ma, Y., and Wang, J. (2018). Enhanced butyric acid production in Clostridium tyrobutyricum by overexpression of rate-limiting enzymes in the embden-meyerhof-parnas pathway. J. Biotechnol. 272, 14–21. doi: 10.1016/j.jbiotec.2018.02.012
Thawornprasert, J., Duangsuwan, W., and Somnuk, K. (2021). “Reduction of free fatty acid in low free fatty acid of mixed crude palm oil (LMCPO): optimization of esterification parameters,” in Materials Science Forum ed R. K. Agarwal (Trans Tech Publication), 111–118. doi: 10.4028/www.scientific.net/MSF.1023.111
Tian, Q., and Hua, Y. (2021). Reaction process and characteristic changes in soybean oil bodies during the formation of volatile flavour compounds in soymilk. Food Sci. Technol. Res. 27, 627–637. doi: 10.3136/fstr.27.627
Trinh, H., Yusup, S., and Uemura, Y. (2018). Optimization and kinetic study of ultrasonic assisted esterification process from rubber seed oil. Bioresour. Technol. 247, 51–57. doi: 10.1016/j.biortech.2017.09.075
Vergara-Fernández, A., Revah, S., Moreno-Casas, P., and Scott, F. (2018). Biofiltration of volatile organic compounds using fungi and its conceptual and mathematical modeling. Biotechnol. Adv. 36, 1079–1093. doi: 10.1016/j.biotechadv.2018.03.008
Wallington, M., Saxon, E. B., Bomb, M., Smittenaar, R., Wickenden, M., Mcphail, S., et al. (2016). 30-day mortality after systemic anticancer treatment for breast and lung cancer in England: a population-based, observational study. Lancet Oncol. 17, 1203–1216. doi: 10.1016/S1470-2045(16)30383-7
Wei, Y., Zou, W., Shen, C. H., and Yang, J. G. (2020). Basic flavor types and component characteristics of Chinese traditional liquors: a review. J. Food Sci. 85, 4096–4107. doi: 10.1111/1750-3841.15536
Xu, Y., Minhazul, K. A., and Li, X. (2020). The occurrence, enzymatic production, and application of ethyl butanoate, an important flavor constituent. Flavour Fragr. J. 35, 601–615. doi: 10.1002/ffj.3613
Yue, X., Wu, M., Jiang, H., Hao, J., Zhao, Q., Zhu, Q., et al. (2016). Endothelial lipase is upregulated by interleukin-6 partly via the p38 MAPK and p65 NF-κB signaling pathways. Mol. Med. Rep. 14, 1979–1985. doi: 10.3892/mmr.2016.5457
Zago, E., Joly, N., Chaveriat, L., Lequart, V., and Martin, P. (2021). Enzymatic synthesis of amphiphilic carbohydrate esters: influence of physicochemical and biochemical parameters. Biotechnol. Rep. 30, e00631. doi: 10.1016/j.btre.2021.e00631
Zhang, J., Mei, F., Zhao, L., Zuo, T., Hong, Y., Li, M., et al. (2021). Inhibition of the p38 MAPK pathway attenuates renal injury in pregnant rats with acute necrotizing pancreatitis. Immunol. Res. 69, 295–306. doi: 10.1007/s12026-021-09195-3
Keywords: flavor esters, esterification, optimization, geranyl butyrate, seedling lipase
Citation: Haq NU, Liaquat M, Alharby HF, Alzahrani YM, Alghamdi SA, Alharbi BM, Alabdallah NM, Saud S, Ahmed M, Sayyed RZ and Fahad S (2022) Optimization and Purification of Terpenyl Flavor Esters Catalyzed by Black Cumin (Nigella sativa) Seedling Lipase in Organic Media. Front. Sustain. Food Syst. 6:915602. doi: 10.3389/fsufs.2022.915602
Received: 08 April 2022; Accepted: 02 May 2022;
Published: 31 May 2022.
Edited by:
Marika Pellegrini, University of L'Aquila, ItalyReviewed by:
Rafiq Ahmad, Northwest A&F University, ChinaMuhammad Nasrullah, Xiangtan University, China
Zheng Mingming, Oil Crops Research Institute (CAAS), China
Copyright © 2022 Haq, Liaquat, Alharby, Alzahrani, Alghamdi, Alharbi, Alabdallah, Saud, Ahmed, Sayyed and Fahad. This is an open-access article distributed under the terms of the Creative Commons Attribution License (CC BY). The use, distribution or reproduction in other forums is permitted, provided the original author(s) and the copyright owner(s) are credited and that the original publication in this journal is cited, in accordance with accepted academic practice. No use, distribution or reproduction is permitted which does not comply with these terms.
*Correspondence: Muhammad Liaquat, bS5saWFxdWF0MTFAbWVtYmVycy5sZWVkcy5hYy51aw==; Shah Fahad, c2hhaF9mYWhhZDgwQHlhaG9vLmNvbQ==; Shah Saud, c2F1ZGhvcnRAZ21haWwuY29t