- 1Department of Food Material Science, Institute of Food Science and Biotechnology, University of Hohenheim, Stuttgart, Germany
- 2Department of Food Science, University of Massachusetts Amherst, Amherst, MA, United States
- 3Department of Microbial and Molecular Systems, Faculty of Engineering Technology, KU (Katholieke Universiteit) Leuven, Ghent, Belgium
- 4GNT Europa GmbH, Aachen, Germany
Phycocyanin is a protein-chromophore structure present in Arthrospira platensis commonly used as a blue-colorant in food. Color losses of phycocyanin can be reduced by electrostatic complexation with λ-carrageenan. The aim of this study was to investigate the effect of molecular weight (MW) of λ-carrageenan on the color stabilization of electrostatic complexes formed with phycocyanin and λ-carrageenan. Samples were heated to 70 or 90°C at pH 3.0 and stored at 25°C for 14 days. The MW of λ-carrageenan was reduced by ultrasound treatments for 15, 30, 60, and 90 min. Prolonged ultrasonication had a pronounced effect on the Mw, which decreased from 2,341 to 228 kDa (0–90 min). Complexes prepared with low MW λ-carrageenan showed greater color changes compared to complexes prepared with high MW λ-carrageenan. The MW had no visible effect on color stability on day 0, but green/yellow shifts were observed during storage and after heating to 70°C. Medium MW showed less color stabilization effects compared to low MW when heated to 70°C. Moreover, for solutions prepared with ultrasonicated λ-carrageenan, significant hue shifts toward green/yellow, and precipitation were observed after a heat treatment at 90°C. In addition, the sizes of the complexes were significantly reduced (646–102 nm) by using ultrasonicated λ-carrageenan, except for the lowest MW λ-carrageenan when heated to 90°C. Overall, these findings demonstrated that decreasing the MW of λC had adverse effects on the color stability of PC:λC complexes heated to 70 and 90°C.
Introduction
Phycocyanin (PC) is a blue food-colorant with a low stability against pH-shifts and heat treatments (Berns and MacColl, 1989; Chaiklahan et al., 2012). Concentrations of more than 20% PC are found in the dry mass of Arthrospira platensis, therefore the cyanobacterium can be utilized for the industrial production of PC (Vernès et al., 2016; Martínez et al., 2017; Kilimtzidi et al., 2019). Arthrospira platensis accumulates allophycocyanin (aPC) and C-phycocyanin (CPC), both of which stabilize at least 1 chromophore per subunit. APC and CPC are structured by α- and β-subunits with globular folding that build the PC monomers, which assemble into trimers and hexamers (Soulier and Bryant, 2021). Both protein-chromophore complexes are part of the light-harvesting units located on the thylakoid membrane of eukaryote algae and cyanobacteria. While having a similar function, CPC differs from aPC by having an additional chromophore connected to its β-subunit (Böcker et al., 2020; Soulier and Bryant, 2021).
Not only the unique color arising from PC but its high nutritional value have been exploited for a few decades (Amara and Steinbüchel, 2013; Lupatini et al., 2017; Costa et al., 2019). In particular, the phycocyanobilins from CPC were shown to have antioxidant activities and radical scavenging properties. Additionally, the apoprotein from aPC is believed to possess an antioxidative character (Eriksen, 2008). Moreover, PC is the only approved blue coloring food within the European Union with some others currently pending approval (Abiusi et al., 2022). Coloring foods provide an excellent solution for appealing colors while meeting customer demands for natural products and they are generally defined as purely physically and non-selctively extracted coloring additives from foodstuff (Carle and Schweiggert, 2016).
In the near future, the color stability of PC-containing extracts will be of tremendous importance (García et al., 2021). Amino acid residues of the protein interact with the phycocyanobilin chromophore via non-covalent interactions, mainly hydrogen bonds and some hydrophobic interactions (Tong et al., 2020). Thereby, the chromophore keeps extended and appears blue (Kupka and Scheer, 2008). These interactions are weakened by structural changes of the protein arising from altering environmental conditions such as changes in pH, temperature, or ionic strength (MacColl and Guard-Friar, 1987; Berns and MacColl, 1989; Chaiklahan et al., 2012).
Typically, the denaturation of PC causes a shift of absorption from 620–650 nm to 346–360 nm (Scheer and Kufer, 1977; Berns and MacColl, 1989) and structural changes of the chromophore can cause bathochromic shifts (Scheer and Kufer, 1977; Soulier and Bryant, 2021). However, the susceptibility of the protein to different treatments is a major drawback because foods are commonly preserved by temperatures ranging from 72 to 121°C and often undergo a change in pH (Heiss and Eichner, 1995; Böcker et al., 2019).
Recently, electrostatic complexation with lambda carrageenan (λC) was suggested as a promising tool for the stabilization of PC in acidic environments and during heat treatments (Li et al., 2021; Buecker et al., 2022a). Carrageenan is commonly found among various red algae (Rhodophyta) families where it is located in between the cellulose structure. It is assembled of repeating 2-sulfated 1,3 linked α-D-galactose and 2,6-disulfated 1,4-linked β-D-galactose, having a MW of 200–800 kDa. λC carries three sulfate groups which result in a highly negative charge (Phillips and Williams, 2009; Wüstenberg, 2015; Zia et al., 2017; McKim et al., 2019). The electrostatic interactions with proteins at pH-values below the isoelectric point (pI) showed to be essential for the complex formation of PC and λC (Buecker et al., 2022a). Further, hydrophobic interaction and hydrogen bonding between the sulfate groups and the α-, β-subunits have been proposed to contribute to the color stability of PC:λC complexes (Buecker et al., 2022a).
In general, molecules of high MW showed to have beneficial effects on complexation by reduced solvent-solute (i.e., the complex is favored) interactions due to three reasons (Pathak et al., 2017; Weiss et al., 2019). First, a higher Mw results in higher viscosity, slowing down diffusion and thus protein aggregation (La Fuente et al., 2004). Second, increasing the MW decreases the entropy of mixing. Thus, large molecules tend to form soluble complexes. Third, λC of high MW has a greater spatial volume which increases the number of anchor points for the protein (Schmitt et al., 1998; Pathak et al., 2017).
The cavitation forces of ultrasound can be used to break down polysaccharides. In particular, high-intensity ultrasound with high power (10–1,000 Wcm−2) and low frequencies (10–100 kHz) is suitable for MW reduction of carbohydrates (Feng et al., 2011; Zabot et al., 2021). In this process, cavitation bubbles expand to a size where their implosion generates strong shear forces. High molecular weight molecules tend to break in the center, while smaller molecules break at random locations along the chain. Low-intensity ultrasound, which applies high frequencies above 1 MHz and power intensities below 1 Wcm−2, is more commonly used to promote sonochemical reactions or to obtain information about media. Shear forces with low-intensity ultrasound are much lower because the gas bubbles generated do not grow as large (Feng et al., 2011).
Yet, there has been no detailed investigation of how PC stability and thus color stability is affected by complexation of λC with different MW's. In the present study, an ultrasonication treatment was performed to obtain λC of different MW, which has been established in previous studies as a technique to reduce the Mw of carrageenan (Hosseini et al., 2013). The aim of the study was to gain insight into the complexation behavior of PC:λC in order to tailor protein-polysaccharide complexes in food applications with respect to their colloidal and color stability. Our general hypothesis was that a higher MW would favor color stability.
Materials and Methods
Materials
Powders of λC and an Arthrospira platensis extract rich in phycocyanin were contributed by GNT International B.V. (Mierlo, Netherlands) and TIC Gums Inc. (White Marsh, USA), respectively. The Arthrospira platensis extract will be referred to as PC powder in this study. All chemicals, namely sodium hydroxide (NaOH), hydrochloric acid (HCL), sodium azide (NaN3), sodium nitrate (NaNO3) were at least of analytical grade and utilized from Carl Roth GmbH & Co. KG (Karlsruhe, Germany).
Methods
Complex Formation and Treatments
Solution Preparation
Stock solutions of λC (c = 1.0% w/w) and PC (c = 0.1% w/w) were prepared with double-distilled water or in 0.1 M NaNO3 for high pressure size exclusion chromatography (HPSEC) analyses. The solutions were treated with NaN3 (c = 0.05% w/w) to prevent microbial growth and were stirred for 8 h to ensure complete hydration (Buecker et al., 2022a).
Ultrasonication
To lower the Mw, stock solutions of λC were treated for 0, 15, 30, 60, and 90 min using a volume of 100 mL. The ultrasound treatment times were chosen based on previous studies (Meunier et al., 2001; Wu et al., 2008). A Bandelin Sonopuls HD2200 with a TT 13 probe, having a diameter of 13 mm, (BANDELIN electronic GmbH & Co. KG, Berlin, Germany) was used for the ultrasound treatment. The system was operated at an amplitude of 100% (149 μmss) with a frequency of 20 kHz and a constant power of 200 W. The highest possible energy inputs were chosen to be within the range of high-intensity ultrasound treatment (>10 W·cm−2, 10–100 kHz) and to efficiently generate λC with different MW (Feng et al., 2011). Evaporation during the ultrasonic treatment was prevented by the covering the sample with a lid and simultaneously cooling of the samples in ice water. Thereby it was possible to maintain the temperature at 12 ± 2°C for the entire ultrasonic treatment time.
Formation of Electrostatic Complexes
Complex formation was induced by lowering the pH from ~6.7 to 3.0 using a concentration of 0.15% w/w λC and 0.1% w/w PC powder at a ratio of 1:4 (PC:λC), which was based on a previous study (Buecker et al., 2022a). The biopolymer solutions were set to pH 3.0 and 6.0 ± 0.02 by HCL or NaOH while being stirred steadily. After initial pH-equilibration, the samples were stored overnight at 4°C. A pH readjustment was performed the next morning prior to the heat treatment.
Heat Treatment
Heat treatment was carried out in polycarbonate centrifuge tubes containing 45 g of the solution. The solutions were heated to 70 and 90°C in a forced-convection water bath (MGW Lauda M20 MS Circulating Bath, Lauda Dr. R. Wobser GmbH & Co. KG, Germany) and the temperature was held constant for 1 min. The samples were subsequently cooled down in a water bath to 25°C. A thermal treatment was carried out to demonstrate the influence of the MW on the color stabilization during temperatures typically used in thermal food processing. The temperatures were chosen because they are in the upper and lower range of common temperatures utilized for pasteurization of most fruit juices (Ramesh, 2007).
Storage Tests
Zeta potential, size by dynamic light scattering (DLS), absorption, and transmittance (see below) values were analyzed at day 0, 3, 6, and 14. In between measurements all samples were stored at 25°C in a climate cabinet (HCP50, Memmert GmbH & Co. KG, Schwabach, Germany).
Analyses
PC Concentration
The individual concentrations of C-phycocyanin (CPC) and allophycocyanin (aPC) in the Arthrospira platensis powder were determined using their absorption maxima of 620 and 650 nm, respectively. According to the two-wavelength method of Yoshikawa and Belay (2008) the total concentration of PC was calculated by summing up the amount of aPC and CPC (Equations 1, 2) (Yoshikawa and Belay, 2008). To obtain the concentration in g/100 g the results were multiplied by 0.1.
here, A620 and A650 refer to the absorption at 620 and 650 nm.
HPSEC Measurement
For the HPSEC measurements, the 0, 15, 30. 60, 90 min ultrasonicated λC solutions were dissolved in the mobile phase (0.1 M NaNO3) (Gómez-Ordóñez et al., 2012). Prior to the measurement, the samples were filtered by Chromafil regenerated cellulose filters RC-20/25 with a pore size of 0.45 μm from Chromafil (Machery-Nagel GmbH & Co. KG, Düren, Germany). The samples were injected into an Agilent HP Series 1100 (Agilent Technologies GmbH & Co. KG, Waldbronn, Germany) with a phase hydroxylated methacrylate packed column TSKgel G5000PWXL (TOSOH Bioscience, Tokyo, Japan) that was equipped with an Agilent 1100 refractive index detector (Agilent Technologies GmbH & Co. KG, Waldbronn, Germany). The injection volume was set to 20 μL and the flow rate to 0.6 mL/min. A six-point calibration curve with dextran standards ranging from 12 to 270 kDa (Fluka Analytical, Buchs, Switzerland) was used to calculate the number average molecular weight (Mn) distribution from the peak of the RID signal according to Equation (3). Herein ci corresponds to the concentration of component i and Mi being the molecular weight of fraction i (Balke et al., 1994; Spichtig and Austin, 2008).
Zeta Potential
For the zeta potential measurement, the samples were filled in folded capillary cells. The zeta potential was analyzed by a Zetasizer Nano Series particle size analyzer (Nano-Zs ZEN 3600, Dispersion Technology Software DTS v 5.1, Malvern Instruments Ltd., Worcestershire, UK). Throughout the measurement, the sample temperature was kept constant at 25°C. The instrument utilizes a laser with a 90° scattering angle and an operating wavelength of 633 nm. The zeta potential is calculated from the electrophoretic mobility of the particles in solution. By utilizing the Smoluchowski approximation the random motion of the particles in solution can be estimated. Herein f(κa) equals 1.5 with κa referring to the ratio of the particle radius to its electrostatic double-layer thickness in polar media (>10−3 molar salt) (Jiang et al., 2009). The measurement was repeated three times.
Size Measurement
The sample size was determined in folded capillary cells by dynamic light scattering (DLS) at a constant temperature of 25°C. The laser measurement was carried out by a Zetasizer Nano Series particle size analyzer (Nano-Zs ZEN 3600, Dispersion Technology Software DTS v 5.1, Malvern Instruments Ltd., Worcestershire, UK) (Jiang et al., 2009). The laser operates at a wavelength of 633 nm and a measurement angle of 173°. Results are reported as intensity mean.
Transmittance and Absorption Measurement
Absorption analysis of the samples was carried out by an Ultrospec 2100 pro (Biochrom GmbH, Berlin, Germany) spectrophotometer in semi-micro UV cuvettes. The instrument was blanked with double-distilled water. The absorption spectrum was determined from 240 to 750 nm and the transmittance was detected at 780 nm. A turbidity baseline correction was performed using the absorption values at 750 nm. All absorption and transmittance data are shown as relative values to unheated and pure PC powder solutions (c = 0.1% w/w) having a pH of 6.0 (Buecker et al., 2022a).
Color Analysis
The colorimetric values lightness (L*), a* (– green/+ red value), b* (– blue/+ yellow value), chroma (C*) and huea, b (h°) in the CIELAB color space 1931 were determined using the absorption spectra. An absorber angle of 2° and illuminant C were employed for the calculation (Schanda, 2007; Wrolstad and Smith, 2017).
Statistics
Statistical analysis was carried out by SPSS Statistics 27 (IBM Inc., Armonk, USA) and Excel (Microsoft Inc., Redmond, USA). Excel was used for the calculation of means and standard deviations. SPSS was used to analyze for statistically significant differences between means. An ANOVA with a subsequent Tukey post-hoc test or double-sided t-Tests was performed. The confidence interval was set to 95%. All samples were prepared in duplicate and at least one repetition of the measurements was conducted.
Results and Discussion
First, the PC content was determined at pH 6.0 (Equations 1, 2). Herein, an aPC content of 5.6% (w/w) and a CPC content of 31.3% (w/w) were determined, resulting in a total PC content of 36.9% (w/w) in the powder. This amount is similar to the values that have been reported previously (Buecker et al., 2022a).
SEC of Ultrasonicated λC
The overall aim of this study was to analyze the effect of molecular weight on the color stabilization properties of λC. Changes in MW by ultrasonication treatment were analyzed by SEC (Figure 1).
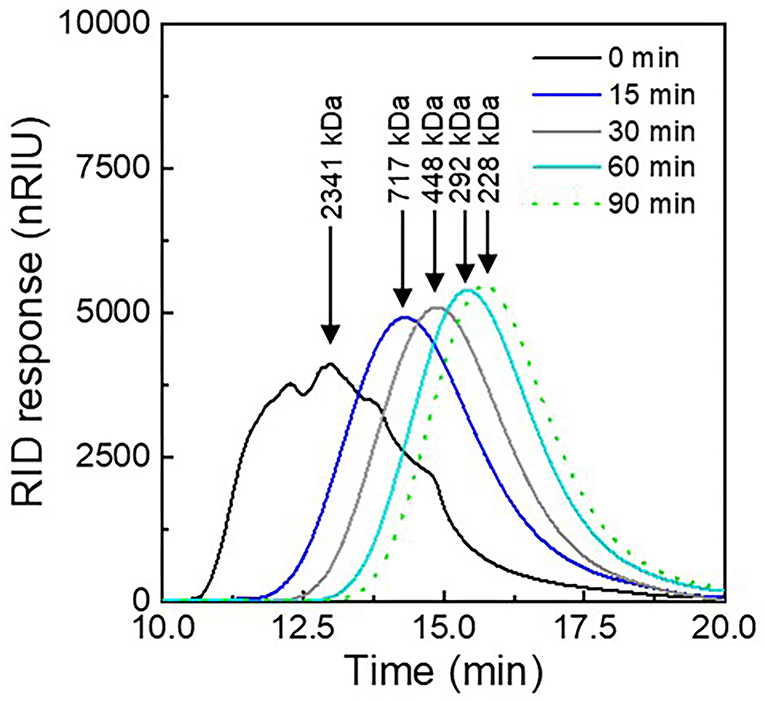
Figure 1. Molecular weight elution profiles of λC solutions prepared in NaNO3 after different ultrasonication treatment times (0–90 min).
During SEC, molecules with a lower MW can diffuse into a larger number of pores of the column material, which leads to a prolonged elution time through the column (Catherine, 2000). As expected, ultrasonicated λC exhibited a shift to longer elution times with increasing treatment times, indicating a decrease in MW caused by the ultrasonication treatment. Untreated λC eluted after about 13 min, while λC treated for 90 min eluted after 16 min. Also, the peak of the signal appeared sharper after the ultrasonication treatment indicating a more homogenous size distribution of λC with increasing treatment time.
In addition, with prolonged ultrasonic treatment, the rate of λC hydrolysis decreased, causing the change in MW to be smaller with increasing treatment time. This is due to the fact that a higher amount of energy is required to break stiffer λC with low MW, causing the molecular degradation rate constant to decrease (Weiss et al., 2011; Ogutu, 2015; Tecson et al., 2021). Thus, the strongest reduction in size was obtained for the 15 min treatment. These findings were in agreement with the observations made for κ-carrageenan in an earlier study (Meunier et al., 2001). Polymers with lower MW exhibit increased ultrasonic damping in solution. This increased relaxation behavior leads to sound waves with a lower amplitude and higher frequency which decreases the power of cavitation (Cochran et al., 1974; Lii et al., 1999). Accordingly, only minor Mw differences were observed for λC ultrasonicated for 60 and 90 min. The MW in turn determines the intrinsic viscosity, which is also used to measure polymer degradation. Thus, it can be assumed that the viscosity of the samples decreased with increasing duration of ultrasonic treatment (Mohod and Gogate, 2011; Azizi and Farahnaky, 2016).
The number average MW of λC was calculated from the chromatogram using Equation (3). The native λC had a MW of ~2,340 kDa which is about 100 times higher than the MW of poligeenan, which is known to have adverse health effects when consumed (Younes et al., 2018). The MW reached around 228 kDa after 90 min treatment time, and thus the ultrasonication treatment resulted in about a 10-fold decrease in MW. However, as the MW decrease between 60 and 90 min was only minor, the following experiments were carried out with λC ultrasonicated for 15, 30, and 90 min.
Visual Appearance
After the decrease in MW was established, the color stability of PC:λC complexes was analyzed at pH 3.0 (strong complexation) and pH 6.0 (no/low complexation) after heat treatment at 70 or 90°C. Initially, the solutions were visually evaluated (Figure 2).
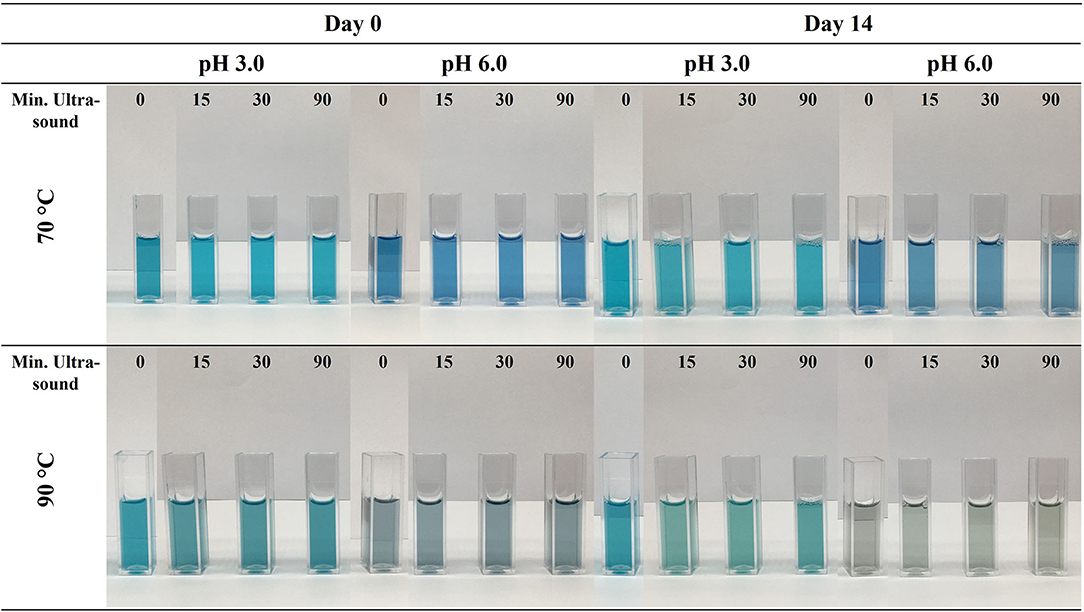
Figure 2. Visual appearance PC:λC (1:4) complexes prepared with λC solutions treated with ultrasonication for 0, 15, 30, and 90 min. Samples were heated to 70 and 90 C at pH 3.0 and 6.0, the solutions were stored for 14 days at 25°C.
The aim of these experiments was to elucidate the effect of different MW of λC on the stabilization of PC:λC complexes during a thermal treatment. Unheated samples were not considered in this study since this would possess a potential food safety risk and more studies are needed to investigate the long term safety aspect of such solutions. At first glance, the decrease in λC MW did not influence the color stability at day 0. All PC:λC complexes occurred turquoise at pH of 3.0 and royal blue at a pH of 6.0. Moreover, the color fading became evident for samples heated at 70°Cand was even more pronounced when heated to 90°C, as expected. The change to a turquoise color at pH 3.0 is related to a change in concentration of the individual PC monomers, trimers, and hexamers, the more pronounced stability of the aPC trimer, and possibly the dissociation of the α- and β-subunit causing the chromophore to change its planarity (Hefferle et al., 1984; Berns and MacColl, 1989; Marx and Adir, 2014; Soulier and Bryant, 2021; Buecker et al., 2022a).
With increasing treatment temperature, the color of solutions at pH 6.0 was less stable than at pH 3.0. This was in agreement with prior experiments and can be related to a stabilizing effect of electrostatic complexation of PC and λC that is not occurring at pH 6.0 (Buecker et al., 2022a). When the samples were stored for the 14 days period, a strong color degradation and green/yellow shift were observed in ultrasonication treated solutions, especially for samples heated to 90°C and to a lesser extent for samples heated to 70°C. At storage conditions of 25°C, the half-life of PC color in solutions without added preservatives is about 18 days, however much shorter at lower pH values (Kannaujiya and Sinha, 2016; Buecker et al., 2022b). The strong time-dependent color degradation was intended to highlight the influence of MW and could have been reduced by lowering the storage temperature. Moreover, color degradation might be slowed down by inducing complexation with polymers that exhibit a strong electrostatic interaction with the protein. Additionally, samples prepared at pH 3.0 with 90 min ultrasonicated λC and subsequent heating to 90°C exhibited precipitation (Figure 4). The aggregation of PC:λC complexes and subsequent precipitation might be associated to a decreased electrostatic repulsion among complexes (Wagoner et al., 2016). Thus, the density of the λC:PC complexes increased. The zeta potential measurements showed that the charge magnitude of the solutions decreased with extended ultrasonication treatment (Supplementary Figure 1). This could be related to hydrolysis of λC (~pKa 2.0) or sonochemical reactions reducing the reactive sites of the backbone (Kardos and Luche, 2001; Al-Zebari et al., 2019). Especially at high temperatures, hydrophobic interactions among PC increase, which might overcome the electrostatic repulsion (Tanford, 1980). Further, an increased charge neutralization upon complexation could lead to a decreased zeta potential (Pillai et al., 2021). Last, according to Stoke's law of sedimentation, the decreased viscosity might additionally favor complex precipitation (Phillips and Williams, 2009).
Expressed in the CIE 1931 L*C*h° chromaticity diagram, the green/yellow shift as a function of storage time and MW becomes very clear for samples heated to 90°C (Figure 3).
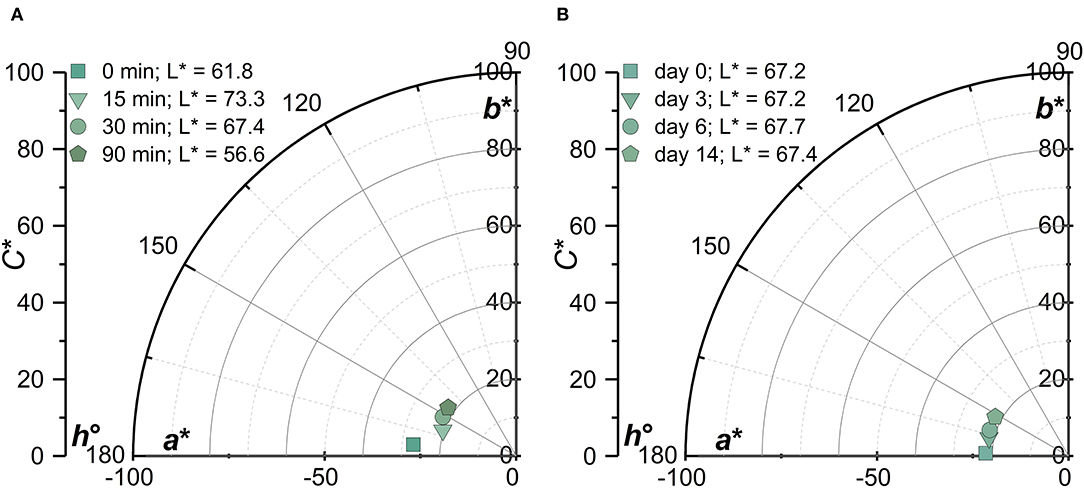
Figure 3. CIE 1931 L*C*h° chromaticity diagram of PC:λC (1:4) complexes at a pH of 3 and heated to 90 C as a function of (A): λC ultrasonication treatment time 0–90 min (2,341–228 kDa) at day 14 and (B): storage time (days 0–14).
The differences upon change in MW became more evident after time and with increasing treatment temperature. Samples prepared with 0 min ultrasonicated λC showed to stabilize the turquoise color at a pH of 3. This was in alignment with prior studies (Li et al., 2021; Buecker et al., 2022a), but ultrasonicated samples showed a destabilization in color over time, especially when heated at 90°C. On the one hand it was suggested that the decreasing MW lead to the formation of denser complexes and on the other hand the increasing stiffness of the low MW λC lead to decreased complex stability and thus to a pronounced fading of the color (Ogutu, 2015; Pathak et al., 2017; Weiss et al., 2019).
Effect on Transmittance and Absorption
Color is the first sensory characteristic that consumers perceive in foods, which impacts their purchasing behavior (Carle and Schweiggert, 2016). To quantify the color degradation, transmittance and absorption measurements were carried out (Figure 4). In addition, the color differences (ΔE) and difference vectors of the colors in the CIE 1931 L*C*h° color space were calculated (Table 1).
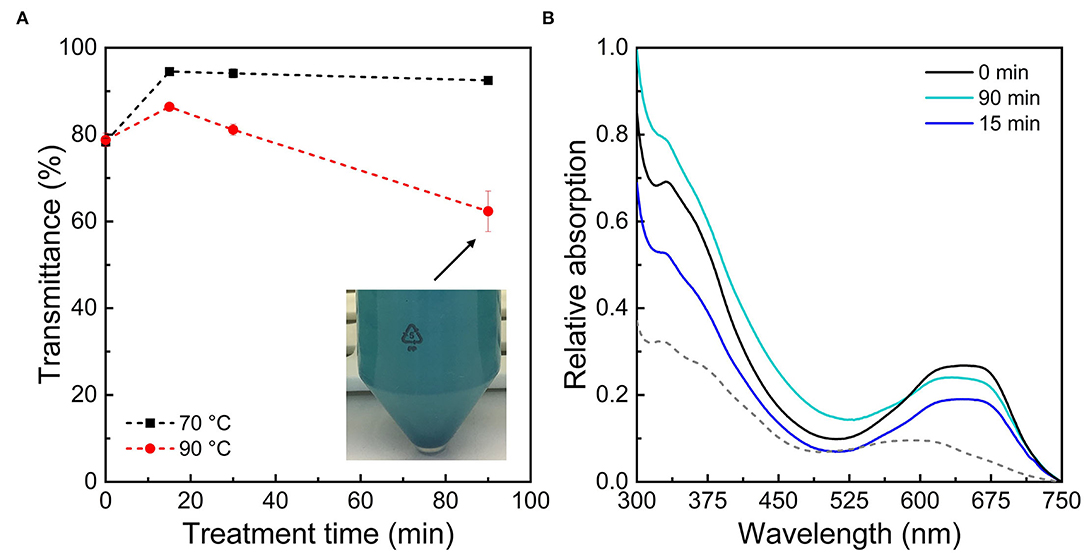
Figure 4. (A) Relative transmittance of PC:λC (1:4) complexes prepared at pH 3.0 stored for 14 days at 25°C after heat treatments to 70 and 90°C plotted over ultrasonication treatment time. (B) Relative absorption of PC:λC complexes heated to 90°C at pH 3.0 with a subsequent storage of 14 days at 25°C after different ultrasonication treatment times. The absorption of PC:λC complexes prepared with untreated λC at pH 6.0, heated to 90°C and stored for 14 days (25°C) is plotted as dotted line.
The color was not affected by the λC ultrasonic treatment until the samples were heated and stored (data not shown). As only minor changes have been observed at day 0, values are only shown for day 14 at 90°C.
The transmittance measurements showed that the effect of MW differed between solutions heated to 70 and 90°C (Figure 4A). Transmittance decreases with the formation of larger complex structures because more light is scattered (Kelley and McClements, 2003). The transmittance decreased for PC:λC complexes heated to 70°C only when solutions were prepared with λC that was not ultrasonicated. As samples were prepared with ultrasonicated λC, the transmittance increased. Possibly, smaller and more soluble complexes were formed by the smaller size of carrageenan, which results in less light scattering.
Solutions heated to 90°C prepared with ultrasonicated λC exhibited a decrease in transmittance with increasing treatment time. Complex solutions containing untreated λC as well as λC which was ultrasonicated for 90 min had the lowest transmittance, whereas solutions containing λC which was ultrasonicated for 15 min had higher transmittance values. The complexes formed from 15 min ultrasonicated λC and PC might be smaller and consequently more soluble. It is suggested that λC which was not ultrasonicated forms large complexes with λC surrounding multiple PC molecules. Wang et al. (2000) showed that increasing the MW of a polymer resulted in larger biopolymer complexes. A critical MW needs to be exceeded in terms of complex formation (Schmitt et al., 1998). However, as the low MW λC (~228 kDa) also stabilized the color of the PC:λC complexes, it can be assumed that the critical MW was reached (Figure 4B). This might be related to the flexibility of λC and a low intrinsic persistence length of 2.7 nm (Berth et al., 2008; Pathak et al., 2017). Our findings are in agreement with Hosseini et al. (2013) who reported decreased affinity of ultrasonicated κ-carrageenan to β-lactoglobulin.
With extended ultrasonication and thereby MW reduction of λC the transmittance decreased for samples heated to 90°C, which was related to increased light scattering because of an increase in complex size (Table 2). This could be related to multiple effects. It was reported that polymers of low MW form denser complexes, possibly because of less steric hindrance (Liu, 2007; Pathak et al., 2017). Larger λC molecules create a stronger steric hindrance that increases the repulsive interactions between the particles, which keeps them in a distance that is not relevant for attractive interactions (Moore et al., 2015). The radius of gyration is roughly 93 nm for λC not treated with ultrasound (Berth et al., 2008). This can slow down the aggregation and denaturation of PC in the samples (Stone et al., 2013; Zhang et al., 2021). Also, a decreased binding affinity of λC to PC could result in accelerated protein unfolding during heating and thereby exposure of hydrophobic groups (Pathak et al., 2017). Hydrophobic interactions among PC could be beneficial during complex development over time as PC association (i.e., intramolecular interactions) within the complexes might be improved and thereby decrease the hydrophobic surface of PC aggregates (Tanford, 1980). This might enlarge the binding sites for λC resulting in denser complexes. In emulsions, protein-polysaccharide complexes with high MW polysaccharides have been shown to prevent droplet aggregation. It was suggested that steric hindrance prevents aggregation processes (Ibanoglu, 2005; Zhao et al., 2018). Our results show that larger polysaacharides are similarly more effective at preventing protein aggregation. Thus, a complex growth over time could be a result of decreased electrostatic and steric stabilization with decreasing λC MW (Wagoner et al., 2016). As mentioned previously, the magnitude of the zeta potential was decreased which could be associated with either enhanced charge neutralization or dissociation of λC (Supplementary Figure 1). Further, the decreased MW might alter the ideal mixing ratio of the biopolymers and thereby change the surface charge of the complex due to increased charge neutralization (Hosseini et al., 2013). However, clearly more research is necessary to find the exact mechanism of increasing optical density over ultrasonication treatment time using these parameters.
The absorption spectrum of PC:λC solutions which were stored for 14 days at 25°C after a heat treatment to 90°C is shown in Figure 4B. To assess the color stabilization a reference line of PC:λC samples prepared at pH 6.0 is shown as well. At pH 3.0, complexation with λC stabilized all solutions against color fading, which is in accordance with the visual observation presented in Figure 2. All samples prepared at pH 3.0 exhibited a pronounced shoulder at ~670 nm, which was expected for a successful complexation with λC and which was responsible for the turquoise color appearance. The bathochromic shift was associated with a planarity changes of the phycocyanobilin (Buecker et al., 2022a). The best color preservation was obtained with high MW λC (2,341 kDa), which was related to a better binding affinity (Pathak et al., 2017). However, with prolonged ultrasonication up to 90 min color perception was increasingly influenced by the enhanced scattering of denatured proteins (Figure 4A) such as PC. Thus, the absorption peak at 347 nm increased (Figure 4B) (Scheer and Kufer, 1977; Wang et al., 2000; Hosseini et al., 2013). Overall, the color perception changed to slightly intenser color values which was reflected by an increased absorption (Figures 2, 4B).
Table 1 shows the color differences between unheated and heated (70°C) PC:λC complexes having a pH of 3.0 at day 0. When heated to 70°C, a ΔE below 10 was observed only for complexes prepared with 0 min ultrasonicated λC. Thus, the color difference was perceptible but can still be considered the same color (Karma, 2020). Samples prepared with ultrasonicated λC must be considered to have a different color (ΔE > 10) after the thermal treatment to 70°C. Prolonged ultrasonication time and storage of the samples increased the color difference, especially for samples heated to 90°C. Samples with high MW λC were chosen as reference. The L*a*b*-values of the references were L* = 62.92, a* = −35.91, b* = −13.92 for unheated samples and L* = 63.10, a* = −32.80, b* = −4.54 for samples heated to 70°C (Buecker et al., 2022a). The L* values increased with storage time and decreased with longer ultrasonic treatment. While the L* values of the samples prepared with 0 min ultrasonicated λC were hardly affected by heat, heating had a strong influence on the samples prepared with ultrasonicated λC. The a* and b* values decreased with increasing storage time and when heated to 90°C. For samples prepared with ultrasound treated λC, the b* values increased when heated to 70°C and decreased when heated to 90°C. The color differences increased considerably due to the ultrasonic treatment of λC, which was especially evident after heating to 90°C. It is supposed that the MW reduction had a negative effect on complex formation and thus negatively impacted color stabilization, as explained before. This is consistent with our previous observations and in line with the literature (Hosseini et al., 2013; Pathak et al., 2017).
Effect on Complex Size
The last experiments were carried out to measure the size of the formed complexes to shed light on the growth behavior. The size was determined by DLS for PC:λC complexes formed and stored at pH 3.0 heated to 70 and 90°C (Table 2).
The size of the complexes formed after heating to 70°C decreased due to the ultrasound treatment. While complexes formed with untreated λC were about 650 nm, complexes formed by λC which was ultrasonicated for 90 min were about 100 nm. The decrease of complex size by lowering the biopolymers MW is in agreement with previous findings and the transmittance measurements presented earlier (Wang et al., 2000; Hosseini et al., 2013). The complex size of the samples prepared with untreated λC decreased over time which could be referred to structural rearrangements driven by an entropy gain as described by the Veis and Aranyi (1960) theory (Veis and Aranyi, 1960; Phillips and Williams, 2009). Intramolecular hydrophobic interactions among proteins change up over time because of internal restructuring processes to obtain the lowest possible free energy and is in agreement with previous findings for PC:λC complexes (Li et al., 2020; Buecker et al., 2022b).
After heating the samples to 90°C the size of the samples prepared with untreated λC and λC treated for 30 and 90 min was similar at day 0. Heated samples with untreated λC had a constant size of 202 nm over the entire storage time whereas solutions with ultrasonicated λC increased in size. The increase in size could be related to multiple effects as mentioned in the previous chapter (Effect on Transmittance and Absorption). We suggest a complex association due to decreased repulsion among complexes. Zeeb et al. (2018) showed that an Ostwald ripening type growth type behavior occurred for biopolymer complexes of whey protein isolate and pectin after heating in the associative regime due to increasing hydrophobic and decreasing electrostatic interactions (Zeeb et al., 2018). This is in accordance with the considerable increase in size of the samples prepared with λC ultrasonicated for 15 min. The increase in size was not significant for the other solutions. However, after 3 days of storage, the samples ultrasonicated for 90 min increased in size. Thus, they were significantly bigger than all the other samples. The increasing size of complexes prepared with ultrasonicated λC is proposed to cause complex sedimentation and storage instability.
Conclusion
This study demonstrated that the decreasing MW of λC had adverse effects on the color stability of PC:λC complexes heated to 70 and 90°C. Herein samples heated to 90°C showed best performances with high MW (2,341 kDa) λC, which was related to an increased binding affinity. The green/yellow shift of the complexes' during storage was promoted by lower MW λC. However, low MW polymers are also suitable for complexation of PC, but to a slightly lesser extent. The size of the complexes was decreased which could be beneficial in terms of storage stabilization and transmittance of food products. Large and dense complexes formed upon heating to low MW λC:PC-complexes to 90°C which could be more suitable for cloudy foods. Moreover, a secondary aggregation by decreased electrostatic repulsion was proposed to result in complex growth of samples prepared with ultrasonicated λC. Hence, precipitation needs to be prevented in products with a high thermal load and λC of lower MW (below 717 kDa) (Figure 5). Yet, prolonged ultrasonication enhanced color intensity.
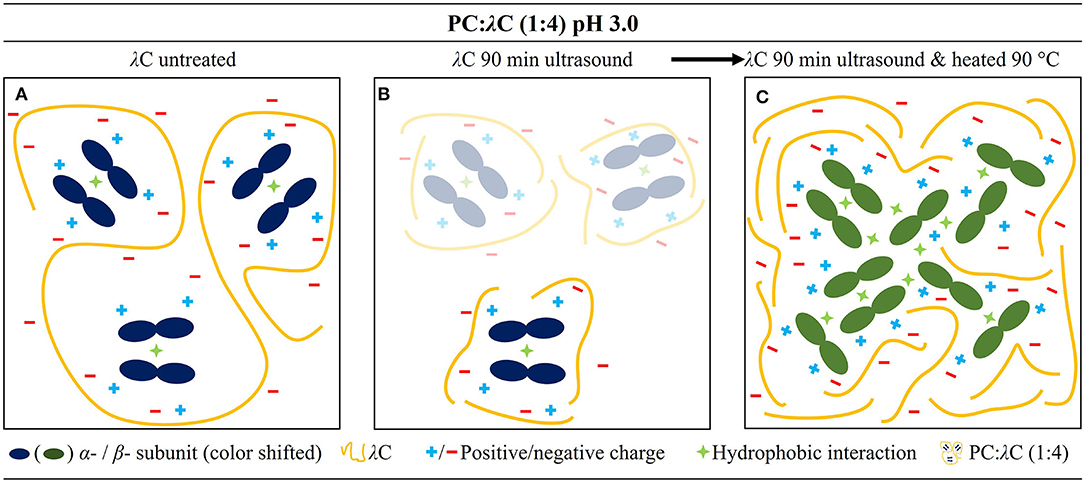
Figure 5. Mechanistic drawing of the PC:λC complex at a pH of 3.0. (A) Complex prepared with 0 min ultrasonicated λC, (B) prepared with 90 min ultrasonicated λC, and (C) complex prepared with 90 min ultrasonicated λC which was heated to 90 C.
The results of the study are important for, among others, carrageenan manufacturers with regard to tailor-made product solutions and food companies stabilizing proteins by complexation with polysaccharides.
Data Availability Statement
The raw data supporting the conclusions of this article will be made available by the authors, without undue reservation.
Author Contributions
SB: writing—original draft, software, investigation, formal analysis, validation, data curation, and visualization. LG: supervision, conceptualization, writing—original draft, and writing—review and editing. ML: methodology and writing—review and editing. EL: supervision and writing—review and editing. JW: conceptualization, project administration, supervision, writing—review and editing, resources, and funding acquisition. All authors contributed to the article and approved the submitted version.
Funding
This project was partly funded by GNT Europa GmbH.
Conflict of Interest
EL is employed by GNT Europa Gmbh.
The remaining authors declare that the research was conducted in the absence of any commercial or financial relationships that could be construed as a potential conflict of interest.
Publisher's Note
All claims expressed in this article are solely those of the authors and do not necessarily represent those of their affiliated organizations, or those of the publisher, the editors and the reviewers. Any product that may be evaluated in this article, or claim that may be made by its manufacturer, is not guaranteed or endorsed by the publisher.
Supplementary Material
The Supplementary Material for this article can be found online at: https://www.frontiersin.org/articles/10.3389/fsufs.2022.915194/full#supplementary-material
Supplementary Figure 1. Zeta potential (mV) of PC:λC (1:4) solutions heated to 70 or 90°C at pH 3.0 or 6.0 and unheated λC at pH 3.0 plotted over ultrasonication treatment time.
References
Abiusi, F., Trompetter, E., Pollio, A., Wijffels, R. H., and Janssen, M. (2022). Acid tolerant and acidophilic microalgae: an underexplored world of biotechnological opportunities. Front. Microbiol. 13, 820907. doi: 10.3389/fmicb.2022.820907
Al-Zebari, N., Best, S. M., and Cameron, R. E. (2019). Effects of reaction pH on self-crosslinked chitosan-carrageenan polyelectrolyte complex gels and sponges. J. Phys. Mater. 2, 15003. doi: 10.1088/2515-7639/aae9ab
Amara, A. A., and Steinbüchel, A. (2013). New medium for pharmaceutical grade arthrospira. Int. J. Bacteriol. 2013, 203432. doi: 10.1155/2013/203432
Azizi, R., and Farahnaky, A. (2016). Ultrasound assisted-viscosifying of kappa carrageenan without heating. Food Hydrocoll. 61, 85–91. doi: 10.1016/j.foodhyd.2016.05.006
Balke, S. T., Mourey, T. H., and Harrison, C. A. (1994). Number-average molecular weight by size exclusion chromatography. J. Appl. Polym. Sci. 51, 2087–2102. doi: 10.1002/app.1994.070511214
Berns, D. S., and MacColl, R. (1989). Phycocyanin in physical chemical studies. Chem. Rev. 89, 807–825. doi: 10.1021/cr00094a005
Berth, G., Vukovic, J., and Lechner, M. D. (2008). Physicochemical characterization of carrageenans-a critical reinvestigation. J. Appl. Polym. Sci. 110, 3508–3524. doi: 10.1002/app.28937
Böcker, L., Hostettler, T., Diener, M., Eder, S., Demuth, T., Adamcik, J., et al. (2020). Time-temperature-resolved functional and structural changes of phycocyanin extracted from Arthrospira platensis/Spirulina. Food Chem. 316, 126374. doi: 10.1016/j.foodchem.2020.126374
Böcker, L., Ortmann, S., Surber, J., Leeb, E., Reineke, K., and Mathys, A. (2019). Biphasic short time heat degradation of the blue microalgae protein phycocyanin from Arthrospira platensis. Innov. Food Sci. Emerg. Technol. 52, 116–121. doi: 10.1016/j.ifset.2018.11.007
Buecker, S., Grossmann, L., Loeffler, M., Leeb, E., and Weiss, J. (2022a). Thermal and acidic denaturation of phycocyanin from Arthrospira platensis: effects of complexation with λ-carrageenan on blue color stability. Food Chem. 380, 132157. doi: 10.1016/j.foodchem.2022.132157
Buecker, S., Grossmann, L., Loeffler, M., Leeb, E., and Weiss, J. (2022b). Influence of storage temperature on the stability of heat treated phycocyanin-λ-carrageenan complexes in liquid formulations. Green Chem. 24, 4174–4185. doi: 10.1039/D2GC00809B
Carle, R., and Schweiggert, R. M., (eds.). (2016). Handbook on Natural Pigments in Food and Beverages: Industrial Applications for Improving Food Color. Duxford, UK: Woodhead Publishing.
Catherine, M. M. (2000). Analysis of Carrageenans Using Capillary Electrophoresis (doctor thesis). England, UK: University of York.
Chaiklahan, R., Chirasuwan, N., and Bunnag, B. (2012). Stability of phycocyanin extracted from Spirulina sp: influence of temperature, pH and preservatives. Process. Biochem. 47, 659–664. doi: 10.1016/j.procbio.2012.01.010
Cochran, M. A., Dunbar, J. H., North, A. M., and Pethrick, R. A. (1974). Acoustic studies of solutions of narrow molecular weight polystyrene in toluene. J. Chem. Soc. Faraday Trans. 2, 70215. doi: 10.1039/f29747000215
Costa, J. A. V., Freitas, B. C. B., Rosa, G. M., Moraes, L., Morais, M. G., and Mitchell, B. G. (2019). Operational and economic aspects of Spirulina-based biorefinery. Bioresour. Technol. 292, 121946. doi: 10.1016/j.biortech.2019.121946
Eriksen, N. T. (2008). Production of phycocyanin–a pigment with applications in biology, biotechnology, foods and medicine. Appl. Microbiol. Biotechnol. 80, 1–14. doi: 10.1007/s00253-008-1542-y
Feng, H., Barbosa-Canovas, G., and Weiss, J., (eds.). (2011). Ultrasound Technologies for Food and Bioprocessing. New York, NY: Springer New York.
García, A. B., Longo, E., and Bermejo, R. (2021). The application of a phycocyanin extract obtained from Arthrospira platensis as a blue natural colorant in beverages. J. Appl. Phycol. 33, 3059–3070. doi: 10.1007/s10811-021-02522-z
Gómez-Ordóñez, E., Jiménez-Escrig, A., and Rupérez, P. (2012). Molecular weight distribution of polysaccharides from edible seaweeds by high-performance size-exclusion chromatography (HPSEC). Talanta 93, 153–159. doi: 10.1016/j.talanta.2012.01.067
Hefferle, P., John, W., Scheer, H., and Schneider, S. (1984). Thermal denaturation of monomeric and trimeric phycocyanins studied by static and polarized time-resolved fluorescence spectroscopy. Photochem. Photobiol. 39, 221–232. doi: 10.1111/j.1751-1097.1984.tb03431.x
Heiss, R., and Eichner, K. (1995). Haltbarmachen von Lebensmitteln: Chemische, Physikalische und Mikrobiologische Grundlagen der Verfahren. Berlin: Heidelberg, s.l. Springer Berlin Heidelberg.
Hosseini, S. M. H., Emam-Djomeh, Z., Razavi, S. H., Moosavi-Movahedi, A. A., Saboury, A. A., Mohammadifar, M. A., et al. (2013). Complex coacervation of β-lactoglobulin - κ-carrageenan aqueous mixtures as affected by polysaccharide sonication. Food Chem. 141, 215–222. doi: 10.1016/j.foodchem.2013.02.090
Ibanoglu, E. (2005). Effect of hydrocolloids on the thermal denaturation of proteins. Food Chem. 90, 621–626. doi: 10.1016/j.foodchem.2004.04.022
Jiang, J., Oberdörster, G., and Biswas, P. (2009). Characterization of size, surface charge, and agglomeration state of nanoparticle dispersions for toxicological studies. J. Nanopart. Res. 11, 77–89. doi: 10.1007/s11051-008-9446-4
Kannaujiya, V. K., and Sinha, R. P. (2016). Thermokinetic stability of phycocyanin and phycoerythrin in food-grade preservatives. J. Appl. Phycol. 28, 1063–1070. doi: 10.1007/s10811-015-0638-x
Kardos, N., and Luche, J.-L. (2001). Sonochemistry of carbohydrate compounds. Carbohydr. Res. 332, 115–131. doi: 10.1016/S0008-6215(01)00081-7
Karma, I. G. M. (2020). Determination and measurement of color dissimilarity. IJEET 5, 67. doi: 10.24843/IJEET.2020.v05.i01.p13
Kelley, D., and McClements, D. (2003). Interactions of bovine serum albumin with ionic surfactants in aqueous solutions. Food Hydrocoll. 17, 73–85. doi: 10.1016/S0268-005X(02)00040-1
Kilimtzidi, E., Cuellar Bermudez, S., Markou, G., Goiris, K., Vandamme, D., and Muylaert, K. (2019). Enhanced phycocyanin and protein content of Arthrospira by applying neutral density and red light shading filters: a small-scale pilot experiment. J. Chem. Technol. Biotechnol. 94, 2047–2054. doi: 10.1002/jctb.5991
Kupka, M., and Scheer, H. (2008). Unfolding of C-phycocyanin followed by loss of non-covalent chromophore-protein interactions 1. Equilibrium experiments. Biochim. Biophys. Acta 1777, 94–103. doi: 10.1016/j.bbabio.2007.10.009
La Fuente, M. A., de Hemar, Y., and Singh, H. (2004). Influence of κ-carrageenan on the aggregation behaviour of proteins in heated whey protein isolate solutions. Food Chem. 86, 1–9. doi: 10.1016/j.foodchem.2003.08.008
Li, X., Li, L., Ma, Y., Wang, R., Gu, Y., and Day, L. (2020). Changes in protein interactions in pasteurized milk during cold storage. Food Biosci. 34, 100530. doi: 10.1016/j.fbio.2020.100530
Li, Y., Zhang, Z., and Abbaspourrad, A. (2021). Improved thermal stability of phycocyanin under acidic conditions by forming soluble complexes with polysaccharides. Food Hydrocoll. 119, 106852. doi: 10.1016/j.foodhyd.2021.106852
Lii, C., Chen, C.-H., Yeh, A.-I., and Lai, V. M.-F. (1999). Preliminary study on the degradation kinetics of agarose and carrageenans by ultrasound. Food Hydrocoll. 13, 477–481. doi: 10.1016/S0268-005X(99)00031-4
Liu, S. (2007). Steric effect: a quantitative description from density functional theory. J. Chem. Phys. 126, 244103. doi: 10.1063/1.2747247
Lupatini, A. L., Colla, L. M., Canan, C., and Colla, E. (2017). Potential application of microalga Spirulina platensis as a protein source. J. Sci. Food Agric. 97, 724–732. doi: 10.1002/jsfa.7987
Martínez, J. M., Luengo, E., Saldaña, G., Álvarez, I., and Raso, J. (2017). C-phycocyanin extraction assisted by pulsed electric field from Artrosphira platensis. Food Res. Int. 99, 1042–1047. doi: 10.1016/j.foodres.2016.09.029
Marx, A., and Adir, N. (2014). Structural characteristics that stabilize or destabilize different assembly levels of phycocyanin by urea. Photosyn. Res. 121, 87–93. doi: 10.1007/s11120-014-9996-5
McKim, J. M., Willoughby, J. A., Blakemore, W. R., and Weiner, M. L. (2019). Clarifying the confusion between poligeenan, degraded carrageenan, and carrageenan: a review of the chemistry, nomenclature, and in vivo toxicology by the oral route. Crit. Rev. Food Sci. Nutr. 59, 3054–3073. doi: 10.1080/10408398.2018.1481822
Meunier, V., Nicolai, T., and Durand, D. (2001). Structure of aggregating κ-carrageenan fractions studied by light scattering. Int. J. Biol. Macromol. 28, 157–165. doi: 10.1016/S0141-8130(00)00166-5
Mohod, A. V., and Gogate, P. R. (2011). Ultrasonic degradation of polymers: effect of operating parameters and intensification using additives for carboxymethyl cellulose (CMC) and polyvinyl alcohol (PVA). Ultrason. Sonochem. 18, 727–734. doi: 10.1016/j.ultsonch.2010.11.002
Moore, T. L., Rodriguez-Lorenzo, L., Hirsch, V., Balog, S., Urban, D., Jud, C., et al. (2015). Nanoparticle colloidal stability in cell culture media and impact on cellular interactions. Chem. Soc. Rev. 44, 6287–6305. doi: 10.1039/C4CS00487F
Ogutu, F. O. (2015). Ultrasonic modification of selected polysaccharides-review. J. Food Process. Technol. 6, 1–8. doi: 10.4172/2157-7110.1000446
Pathak, J., Priyadarshini, E., Rawat, K., and Bohidar, H. B. (2017). Complex coacervation in charge complementary biopolymers: Electrostatic versus surface patch binding. Adv. Colloid Interface Sci. 250, 40–53. doi: 10.1016/j.cis.2017.10.006
Pillai, P. K., Guldiken, B., and Nickerson, M. T. (2021). Complex coacervation of pea albumin-pectin and ovalbumin-pectin assessed by isothermal titration calorimeter and turbidimetry. J. Sci. Food Agric. 101, 1209–1217. doi: 10.1002/jsfa.10733
Ramesh, M.S. (2007). “Pasteurization and food preservation,” in Handbook of Food Preservation, ed M. S. Rahman (Boca Raton, FL: CRC Press).
Schanda, J. (2007). Colorimetry: Understanding the CIE system. Vienna; Hoboken, NJ: CIE/Commission internationale de l'eclairage; Wiley-Interscience.
Scheer, H., and Kufer, W. (1977). Conformational studies of phycocyanin from Spirulina platensis. Z. Naturforsch. 32, 513–519. doi: 10.1515/znc-1977-7-806
Schmitt, C., Sanchez, C., Desobry-Banon, S., and Hardy, J. (1998). Structure and technofunctional properties of protein-polysaccharide complexes: a review. Crit. Rev. Food Sci. Nutr. 38, 689–753. doi: 10.1080/10408699891274354
Soulier, N., and Bryant, D. A. (2021). The structural basis of far-red light absorbance by allophycocyanins. Photosyn. Res. 147, 11–26. doi: 10.1007/s11120-020-00787-y
Spichtig, V., and Austin, S. (2008). Determination of the low molecular weight fraction of food-grade carrageenans. J. Chromatogr. B Analyt. Technol. Biomed. Life Sci. 861, 81–87. doi: 10.1016/j.jchromb.2007.11.012
Stone, A. K., Cheung, L., Chang, C., and Nickerson, M. T. (2013). Formation and functionality of soluble and insoluble electrostatic complexes within mixtures of canola protein isolate and (κ-, ι- and λ-type) carrageenan. Int. Food Res. J. 54, 195–202. doi: 10.1016/j.foodres.2013.06.009
Tanford, C. (1980). The Hydrophobic Effect: Formation of Micelles and Biological Membranes. New York, NY: Wiley.
Tecson, M. G., Abad, L. V., Ebajo, V. D., and Camacho, D. H. (2021). Ultrasound-assisted depolymerization of kappa-carrageenan and characterization of degradation product. Ultrason. Sonochem. 73, 105540. doi: 10.1016/j.ultsonch.2021.105540
Tong, X., Prasanna, G., Zhang, N., and Jing, P. (2020). Spectroscopic and molecular docking studies on the interaction of phycocyanobilin with peptide moieties of C-phycocyanin. Spectrochim. Acta A Mol. Biomol. Spectrosc. 236, 118316. doi: 10.1016/j.saa.2020.118316
Veis, A., and Aranyi, C. (1960). Phae separation in polyelectrolyte systems: I. Complex coacervates of gelatin. J. Phys. Chem. 64, 1203–1210. doi: 10.1021/j100838a022
Vernès, L., Granvillain, P., Chemat, F., and Vian, M. (2016). Phycocyanin from Arthrospira platensis. Production, extraction and analysis. Curr. Biotechnol. 4, 481–491. doi: 10.2174/2211550104666151006002418
Wagoner, T., Vardhanabhuti, B., and Foegeding, E. A. (2016). Designing whey protein-polysaccharide particles for colloidal stability. Annu. Rev. Food Sci. Technol. 7, 93–116. doi: 10.1146/annurev-food-041715-033315
Wang, Y., Kimura, K., Dubin, P. L., and Jaeger, W. (2000). Polyelectrolyte–micelle coacervation: effects of micelle surface charge density, polymer molecular weight, and polymer/surfactant ratio. Macromolecules 33, 3324–3331. doi: 10.1021/ma991886y
Weiss, J., Kristbergsson, K., and Kjartansson, G. T. (2011). “Engineering food ingredients with high-intensity ultrasound,” in Ultrasound Technologies for Food and Bioprocessing, eds H. Feng, G. Barbosa-Canovas, and J. Weiss (New York, NY: Springer New York), 239–285.
Weiss, J., Salminen, H., Moll, P., and Schmitt, C. (2019). Use of molecular interactions and mesoscopic scale transitions to modulate protein-polysaccharide structures. Adv. Colloid Interface Sci. 271, 101987. doi: 10.1016/j.cis.2019.07.008
Wrolstad, R. E., and Smith, D. E., (eds.). (2017). Color Analysis. Cham: Springer International Publishing.
Wu, T., Zivanovic, S., Hayes, D. G., and Weiss, J. (2008). Efficient reduction of chitosan molecular weight by high-intensity ultrasound: underlying mechanism and effect of process parameters. J. Agric. Food Chem. 56, 5112–5119. doi: 10.1021/jf073136q
Wüstenberg, T. (2015). Cellulose and Cellulose Derivatives in the Food Industry: Fundamentals and Applications. Weinheim: Wiley-VCH.
Yoshikawa, N., and Belay, A. (2008). Single-laboratory validation of a method for the determination of c-phycocyanin and allophycocyanin in Spirulina (Arthrospira) supplements and raw materials by spectrophotometry. J. AOAC Int. 91, 524–529. doi: 10.1093/jaoac/91.3.524
Younes, M., Aggett, P., Aguilar, F., Crebelli, R., Filipič, M., Frutos, M. J., et al. (2018). Re-evaluation of carrageenan (E 407) and processed Eucheuma seaweed (E 407a) as food additives. EFSA J. 16, e05238. doi: 10.2903/j.efsa.2018.5238
Zabot, G. L., Viganó, J., and Silva, E. K. (2021). Low-frequency ultrasound coupled with high-pressure technologies: impact of hybridized techniques on the recovery of phytochemical compounds. Molecules 26:1–20. doi: 10.3390/molecules26175117
Zeeb, B., Mi-Yeon, L., Gibis, M., and Weiss, J. (2018). Growth phenomena in biopolymer complexes composed of heated WPI and pectin. Food Hydrocoll. 74, 53–61. doi: 10.1016/j.foodhyd.2017.07.026
Zhang, Z., Cho, S., Dadmohammadi, Y., Li, Y., and Abbaspourrad, A. (2021). Improvement of the storage stability of C-phycocyanin in beverages by high-pressure processing. Food Hydrocoll. 110, 106055. doi: 10.1016/j.foodhyd.2020.106055
Zhao, C., Zhang, H., Yan, J., Xu, X., Liu, M., Cao, Y., et al. (2018). Effect of dextran molecular weight on structure and emulsifying property of zein conjugates. Nongye Gongcheng Xuebao/Trans. Chin. Soc. Agric. Eng. 34, 288–295. doi: 10.11975/j.issn.1002-6819.2018.14.037
Keywords: complexation, coloring food, binding affinity, size exclusion chromatography, carbohydrates, sonochemistry, interaction, microalgae
Citation: Buecker S, Grossmann L, Loeffler M, Leeb E and Weiss J (2022) High Molecular Weight λ-Carrageenan Improves the Color Stability of Phycocyanin by Associative Interactions. Front. Sustain. Food Syst. 6:915194. doi: 10.3389/fsufs.2022.915194
Received: 07 April 2022; Accepted: 09 May 2022;
Published: 10 June 2022.
Edited by:
Ashish Rawson, Indian Institute of Food Processing Technology, IndiaReviewed by:
Chetan A. Nayak, BMS College of Engineering, IndiaPintu Choudhary, Lovely Professional University, India
Mohan Naik, Karunya Institute of Technology and Sciences, India
Copyright © 2022 Buecker, Grossmann, Loeffler, Leeb and Weiss. This is an open-access article distributed under the terms of the Creative Commons Attribution License (CC BY). The use, distribution or reproduction in other forums is permitted, provided the original author(s) and the copyright owner(s) are credited and that the original publication in this journal is cited, in accordance with accepted academic practice. No use, distribution or reproduction is permitted which does not comply with these terms.
*Correspondence: Jochen Weiss, ai53ZWlzcyYjeDAwMDQwO3VuaS1ob2hlbmhlaW0uZGU=