- 1ICAR-Indian Agricultural Research Institute, New Delhi, India
- 2Indian Council of Agricultural Research (ICAR)-National Bureau of Plant Genetic Resources, New Delhi, India
- 3ICAR-Indian Agricultural Statistics Research Institute, New Delhi, India
A total of 120 highly diverse cowpea [Vigna unguiculata (L.) Walp] genotypes, including indigenous and exotic lines, were evaluated for different biochemical traits using AOAC official methods of analysis and other standard methods. The results exhibited wide variability in the content of proteins (ranging from 19.4 to 27.9%), starch (from 27.5 to 42.7 g 100 g−1), amylose (from 9.65 to 21.7 g 100 g−1), TDF (from 13.7 to 21.1 g 100 g−1), and TSS (from 1.30 to 8.73 g 100 g−1). The concentration of anti-nutritional compounds like phenols and phytic acid ranged from 0.026 to 0.832 g 100 g−1 and 0.690 to 1.88 g 100 g−1, respectively. The correlation coefficient between the traits was calculated to understand the inter-trait relationship. Multivariate analysis (PCA and HCA) was performed to identify the major traits contributing to variability and group accessions with a similar profile. The first three principal components, i.e., PC1, PC2, and PC3, contributed to 62.7% of the variation, where maximum loadings were from starch, followed by protein, phytic acid, and dietary fiber. HCA formed six distinct clusters at a squared Euclidean distance of 5. Accessions in cluster I had high TDF and low TSS content, while cluster II was characterized by low amylose content. Accessions in cluster III had high starch, low protein, and phytic acid, whereas accessions in cluster IV contained high TSS, phenol, and low phytic acid. Cluster V was characterized by high protein, phytic acid, TSS, and phenol content and low starch content, and cluster VI had a high amount of amylose and low phenol content. Some nutri-dense accessions were identified from the above-mentioned clusters, such as EC169879 and IC201086 with high protein (>27%), TSS, amylose, and TDF content. These compositions are promising to provide practical support for developing high-value food and feed varieties using effective breeding strategies with a higher economic value.
Introduction
By 2050, the world's population can be expected to grow by 70% of the current population, bringing immense challenges to the global food community for providing safe and secure food supplies (Omomowo and Babalola, 2021). Legumes are one of the food sources to achieve the sustainable objective of providing a good amount of essential nutrients, particularly low-cost protein. Cowpea is one such versatile and hardy legume crop that is adaptable in areas with water scarcity and low-fertile soils. Its high protein, low carbon footprint, less growth period, and high productivity in marginal areas will allow the fulfillment of three sustainable development goals, i.e., SDG 2, 3, and 13.
Cowpea (Vigna unguiculata), a warm-weather legume of the Fabaceae family, is a native of Central Africa (Harlan, 1971). Globally, cowpea is cultivated in more than 12.5 million hectares (ha) of land, of which Africa accounts for 98% of the total cropped area. Nigeria is the largest producer of cowpea, accounting for 48% of the total production in Africa (FAO, 2019),1 and other major producing regions are Central and South America. In India, it is a minor pulse cultivated in an area of 3.9 million ha grossing an annual production of 2.21 million tons (Giridhar et al., 2020), mainly in arid and semi-arid tracts of Punjab, Haryana, Delhi, and western Uttar Pradesh.
Malnutrition, particularly related to low intake of protein, iron, and zinc, is the primary health concern for children of poor and developing nations, where a cereal-based diet constitutes the bulk of food intake (Gonçalves et al., 2016). Cowpea is one such legume with a high protein (20.7–27.3%) and low fat (1–1.2 g 100 g−1) content and significant amounts of carbohydrates, minerals, and other nutrients. High adaptability for different environments, low input costs, and high yield make it highly suitable for cultivation in countries with protein deficiency. Considerable genetic variation in the protein content of cowpea (18.3–30.3%) was reported (Elharadallou et al., 2015). A high-protein cultivar was developed with a protein content of 30%, which is a value close to that observed in some soybean cultivars (Glycine max). Still, in currently grown cowpea cultivars, the protein content in seeds is within the range of 22–25% (Boukar et al., 2011). Biochemically, cowpea protein has a lower methionine and higher lysine, leucine, and isoleucine content (Nikmaram et al., 2017). Besides protein, the seeds of leguminous crops are a good source of complex carbohydrates, dietary fiber, and resistant starch. Typically, the total carbohydrate content was found to be in the range of 56–68 g 100 g−1, of which starch was the major constituent (about 52 g 100 g−1) (Oghbaei and Prakash, 2016). Cowpea grains show wide variability (22.3–66.5 g 100 g−1) and the highest starch content among legumes along with a good amount of dietary fiber and resistant starch (Ali et al., 2013; Gonçalves et al., 2016). The high starch content in cowpea makes it suitable for the preparation of many processed products, such as moin moin and akara (Mamiro et al., 2011). Moreover, being rich in dietary fiber (16–20.9 g 100 g−1), the consumption of cowpea helps to keep the digestive system healthy and reduces the risk of diabetes and cardiovascular diseases (Gonçalves et al., 2016). Total soluble sugar usually plays a vital role in the metabolism of carbohydrates, imparting abiotic stress tolerance and improving the palatability of the accessions in different food items. A wide variation in the sugar content (ranging from 3.26–8.61 g 100 g−1) was reported in cowpea (Weng et al., 2018).
Cowpea grains are rich in phenolic compounds, tannins, and flavonoids and show considerable antioxidant and radical scavenging activity. These compounds are found more in colored seeds than in white ones, with an optimum range of 0.544–0.844 g 100 g−1, and protocatechuic acid is the primary polyphenol present in cowpea, followed by ferulic acid and p-coumaric acid (Awika and Duodu, 2017). In dry beans like cowpea, phytic acid is the primary storage form of phosphorous. The phytic acid content of cowpea generally ranges from 660 to 836 mg 100 g−1 (Jayathilake et al., 2018; Feizollahi et al., 2021), which is higher than that present in cereals. However, polyphenols and phytates also limit nutrient bioavailability through chelation of divalent cations (Madodé et al., 2011).
Multivariate analysis like principal component analysis (PCA) and hierarchical cluster analysis (HCA) has been used for enhancing our comprehension of the data structure and for determining how nutritional qualities are distributed and linked (Tomar et al., 2021). These techniques can also characterize genetic divergence and the possible coinheritance of traits. In the past, crop improvement programs majorly focused on the production and productivity of grain legumes; however, with the realization of hidden hunger, emphasis has now shifted toward breeding for nutritional traits. Hence, it is required to evaluate the germplasm collections in different crops for important nutritional traits and identify trait-specific nutri-dense accessions.
The present study was carried out on 120 diverse accessions, including indigenous and exotic collections, of cowpea for selected biochemical compositions, i.e., protein, starch, amylose, total dietary fiber (TDF), total soluble sugar (TSS), phenols, and phytic acid, and to identify nutri-dense or nutritionally superior germplasm from the whole set based on the biochemical traits.
From the available germplasm collection, it is imperative to select appropriate diverse genotypes with the desired trait that can be used in crop improvement programs to develop genetic stocks or to develop improved varieties.
Materials and Methods
Under natural conditions, cowpea accessions were sown for Kharif Vigna species at the research farm of ICAR-NBPGR, New Delhi, India, located at a latitude of 28°40′N, a longitude of 77°12′E, and an altitude of 228 m AMSL. Recommended package of practices was followed, and 100 kg ha−1 of diammonium phosphate (DAP) was used as a basal dose before sowing. To ensure proper germination, one irrigation was given before sowing and one light irrigation at the flowering stage, ensuring the availability of sufficient moisture during the entire cropping season. Every cowpea accession was grown in two rows with a spacing of 60 cm between the rows and each 4 cm in length. No insecticide was sprayed, and after 25–30 days of sowing, manual weeding was done. The experimental location falls under the tropical zone in the trans-Gangetic plain with an annual rainfall of 750 mm. The site was well-drained, and the soil was slightly alkaline (pH 7.8) and sandy loam. The climate had maximum and minimum temperatures of 31 and 17.3°C, respectively.
Sample Collection and Processing
Pods were collected from all the accessions grown at the physiological maturity stage and sun-dried. A seed set of 475 cowpea accessions with high variability in seed morphology were collected from mature and dried pods and cleaned to remove extraneous material.
Sample Selection Using Near-Infrared Reflectance Spectroscopy (NIRS)
Seeds of all the 475 accessions were scanned using FOSS NIRS 6500, and the reflectance spectrum (ratio of reflected vs. incident light) was recorded from 400 to 2,490 nm (Supplementary Figure 1). Normalized spectral data of 475 samples were subjected to hierarchical clustering by Ward's method using squared Euclidean distance. Major clusters were separated and sub-clustered in the same manner. A representative set of samples was obtained by selecting samples from cluster/sub-cluster centers and extreme boundaries. All accessions where clusters/sub-clusters had up to four members were selected, resulting in a set of 120 accessions. The selected samples were ground using Foss cyclotech mill equipped with a 1 mm sieve to get homogenized flour for analysis.
Biochemical Analysis
Protein
Total nitrogen content was estimated using the Kjeldahl method (AOAC 984.13), with some modifications in the digestion method. The organic compounds present in the sample were digested overnight at room temperature by adding the digestion mixture (mixture of sulfuric acid, selenium catalyst, lithium sulfate, and hydrogen peroxide) (Michałowski et al., 2013). Partially digested samples were heated at 400°C for 20 min to complete the digestion and get a clear and transparent solution. A Foss Kjeltec Nitrogen Autoanalyzer (Foss Tecator 2300 Kjeltec Analyzer Distiller Unit) estimated the nitrogen content in the samples and converted it to protein percentage using Jone's factor 6.25.
Total Starch Content
About 100 mg of homogenized sample was extracted twice using 80% ethanol at 80°C for 30 min. After each extraction cycle, the samples were centrifuged at 10,000 rpm for 10 min, supernatants were pooled, and the residue thus obtained was used to estimate the total starch content. Total starch content was estimated by Megazyme total starch assay kit as per AOAC 996.11. It initially uses α-amylase to hydrolyze starch into maltodextrin, which is followed by the action of amyloglucosidase to hydrolyze maltodextrin into D-glucose. Glucose was oxidized causing the liberation of hydrogen peroxide, which was measured using a GOPOD reagent that gave pink color. The absorbance was recorded at 510 nm using a UV–Vis spectrophotometer. The results were expressed as g100 g−1.
Amylose
Amylose was determined by the modified KI–I2 iodometric assay (Perez and Julian, 1978) based on the binding capacity of the amylose–iodine complex. The absorbance was recorded at 620 nm using a UV–Vis spectrophotometer, and the results were expressed as g100 g−1. A standard calibration curve was also developed using potato amylose obtained from Sigma Aldrich.
Total Dietary Fiber (TDF)
The TDF content was estimated in duplicates using a commercial assay kit purchased from Megazyme International, Wicklow, Ireland (AOAC 985.29). The method included the use of α-amylase, protease, and amyloglucosidase, followed by precipitation with 95% ethanol. After vacuum filtration, one of the duplicates was estimated for total ash content using a muffle furnace. In contrast, the other one was used for the estimation of total protein content using the Kjeldahl method, and the result was expressed as g100 g−1.
Total Soluble Sugar Content
The extract obtained by the procedure mentioned in section starch was used to estimate the TSS content using the anthrone reagent method (Dubois et al., 1956), which involves the dehydration of sugars by sulfuric acid to form furfural. The furfural–anthrone complex (blue-green) was read for absorbance at 630 nm using a UV–Vis spectrophotometer, while D-glucose was used as a standard to develop a calibration curve. This method measures the content of total soluble sugars, including monosaccharides, disaccharides, and oligosaccharides. The results were expressed as glucose equivalent in g 100 g−1.
Total Phenols
The extract obtained by the procedure mentioned in section starch was used to estimate the total phenolic content using an FCR assay (Singleton et al., 1999), which includes oxidation/reduction reaction. FCR is a mixture of molybdates and tungstates that get reduced by phenolic compounds, which is marked by the development of a blue complex. The absorbance was read at 650 nm using a UV–Vis spectrophotometer, and the results were expressed as GAE in g 100 g−1.
Phytic Acid
Phytic acid content was estimated by enzymatic method using a Phytate assay kit (K-PHYT) from Megazyme International, Wicklow, Ireland (AOAC 986.11). Total and free phosphorous content was estimated separately using an ascorbic acid-molybdate color reagent. The absorbance was recorded after incubation at 655 nm using a UV–Vis spectrophotometer, and the result was expressed as g 100 g−1.
Statistical Analysis
All the measurements were performed in duplicates. Descriptive statistics, including mean ± standard deviations (SD) values, were calculated for the data. Principal component analysis (PCA) was performed to analyze the differences and identify the accessions with characteristics comparable to their nutritional composition. Descriptive statistics, PCA, and Pearson's correlation test were analyzed in The Jamovi Project (2020) (version 1.6). Using a trial version of IBM SPSS, hierarchical cluster analysis (HCA) was performed using Ward's clustering method and squared Euclidean distance.
Results and Discussion
Nutritional Analysis
The results of the estimation of biochemical traits by different methodologies are given in Supplementary Table 1. Descriptive statistics of data are calculated to assess the variability in each trait (Table 1). Results show high variability for each trait with the following range of values: 19.4–27.9% (proteins), 27.5–42.7 g 100 g−1 (starch), 9.65–21.7 g 100 g−1 (amylose), 13.7–21.1 g 100 g−1 (TDF), 1.30–8.73 g100 g−1 (TSS), 0.026–0.832 g 100 g−1 (phenols), and 0.690–1.88 g100 g−1 (phytate). The high variability in the nutritional profile among the accessions can be due to the differences in the genetic composition of the cowpea genotypes (Mbuma et al., 2021). Each parameter was visualized using a box and whiskers plot, as shown in Supplementary Figures 2A–G, which is discussed in the subsequent sections.
Total Protein Content
There was a significant difference between the accessions where the protein content ranged from 19.3% (EC724681) to 27.9% (EC390248) with a mean value of 24% (Table 1), which is considerably higher than that found in white and brown cowpea seeds (20.4 and 21.3%, respectively; Longvah et al., 2017). Our values agree with the previously reported values in cowpea which ranged from 17.4 to 31.7% (Antova et al., 2014). Cowpea is known for its high protein content in seeds, but it can vary depending on the cultivar or edaphic factors. The protein content values higher than those obtained in our study (26.5–29%) have been reported in three cowpea varieties from Ghana (Appiah et al., 2011). Owing to the high protein present in cowpea, it will fulfill SDG 2, i.e., achieving zero hunger by tackling malnutrition diseases like kwashiorkor in poor children. It can be used to make protein-rich food and food additives that could be beneficial for infants in particular and as high protein feed for animals. Moreover, a good crop improvement program could be conducted by selecting promising accessions, including EC390248 (27.9%), EC169879 (27.7%), and EC240667 (26.6%), from our study.
Total Starch Content
Significant variation was observed in the starch content between accessions and ranged from 27.5 g 100 g−1 (IC214752) to 42.7 g 100 g−1 (EC724681) with a mean value of 32.6 g 100 g−1 (Table 1), which is considerably lower than the starch values reported for brown (47.1 g100 g−1) and white (47.5 g 100 g−1) cowpea grains (Longvah et al., 2017). The difference could be due to the presence of highly hydrated fine fiber fraction derived from the cell wall enclosing starch granules or high content of insoluble and closely associated starch granules (Hall et al., 2017). The values obtained in our study were in the range of 28.3–36.2 g 100 g−1 (Antova et al., 2014), which are lesser than the values reported by the studies done on 15 Nigerian cowpea cultivars (60 g 100 g−1; Gonçalves et al., 2016). Moreover, compared to other legumes, cowpea has a higher starch content and in vitro starch hydrolysis rate (Nkhata et al., 2018). The starch yield of isolated cowpeas was low when compared to the yield of other legumes like black gram (45 g 100 g−1) and red bean (46 g100 g−1; Hoover et al., 2010); however, the starch yield was found to be high when compared to beach pea (12.3 g100 g−1), grass pea (26 g100 g−1), green pea (30 g100 g−1), and adzuki bean (21.5 g100 g−1; Ren et al., 2021). These results confirmed that cowpea starch could be a valuable source of legume starch. It is considered to be beneficial for human health, as the legume starch is digested more slowly than the starch obtained from cereals. High starch-containing accessions can also be used to make processed products like moin moin and akara (Phillips et al., 2022) because of their superior processing properties, which will significantly contribute to SDG 2 and 3.
Amylose
Amylose content of cowpea differed significantly between accessions and ranged from 9.7 g 100 g−1 (IC249141) to 21.7 g100 g−1 (EC472284) with a mean value of 15.8 g 100 g−1 (Table 1). Higher amylose content has been reported in the starch of cowpea and mungbean in the range of 36–39 g100 g−1 (Kim et al., 2018). The amylose content affects the physicochemical properties of starch, such as viscosity, swelling power, gelatinization capacity, retrogradation, and starch crystallinity (Petroni et al., 2017). High-amylose starch is prone to retrogradation by nature and is resistant to digestion. Although legume starches have high amylose content (Zia-ud-Din et al., 2017), the starch and protein contents are inversely related, and the high protein content in our samples explains the low amylose content. The reported range of amylose in cowpea cultivars is 13.9–15.5 g 100 g−1 and 17.0–18.7 g 100 g−1 for whole-grain and decorticated flour respectively (Adebooye and Singh, 2008) and 16.7–19.75 g 100 g−1 (Naiker et al., 2019) are similar to our values. Accessions having high amylose content, i.e., EC472284 (21.7 g 100 g−1), IC128727 (21.4 g 100 g−1), and EC244077 (20.5 g100 g−1), would have a low GI, which slows down the process of digestion, thus preventing the increase of blood sugar levels right after a meal and thereby helping diabetic patients. Low-amylose accessions, such as IC249141 (9.7 g 100 g−1), EC240900A (10 g100 g−1), and IC214752 (10.8 g 100 g−1) have high swelling power, which gradually softens the grains and facilitates easy cooking of the grains (Wani et al., 2012). Hence, accessions containing low and high amylose content have their significance depending on the user's interests and requirements.
Total Dietary Fiber
The content of total dietary fiber ranged from 13.7 g 100 g−1 (EC724690) to 21.1 g 100 g−1 (EC244138) with an average value of 17.3 g 100 g−1 (Table 1), which is greater than the values of brown and white cowpea grains (11.5 g 100 g−1 and 11.7 g 100 g−1, respectively; Longvah et al., 2017), but close to the previously reported value of 15 g 100 g−1 (Akissoé et al., 2021) and less than the reported values of 27.4 g 100 g−1 (Ghavidel and Prakash, 2007) and 27 g 100 g−1 (Kalogeropoulos et al., 2010) for raw cowpea. Genetic and environmental factors might attribute to the variation of TDF content. Accessions with high TDF (EC724033 and EC244138) could be a preferred dietary fiber source for people suffering from constipation, cardiovascular diseases, and obesity, which will facilitate achieving SDG 3, i.e., good health and wellbeing of individuals. WHO recommends an intake of >25 g of fiber, which could be contributed by cowpea along with cereals, fruits, and vegetables (Joint and Consultation, 2018). Foods derived from cowpea accessions having high dietary fiber could prove to be beneficial for maintaining a regular bowel function, assisting in weight loss, and lowering the risk of heart diseases and type-II diabetes.
Total Soluble Sugar
The TSS content varied from 1.30 g 100 g−1 (EC724385) to 8.73 g 100 g−1 (EC149303) with a mean value of 5.58 g 100 g−1 (Table 1), which is comparatively higher than the reported range of 1.11–4.07 g 100 g−1 with a mean value of 2.23 g 100 g−1 (Nassourou et al., 2017). The experimental values are similar to the reported range of 3.26–8.60 g 100 g−1 with a mean value of 5.45 g 100 g−1 (Weng et al., 2018). High TSS content imparts tolerance to abiotic stress factors and improves the storability of seeds by increasing desiccation tolerance. TSS contributes to the desirable taste and availability of fermentable sugars. Fermented pulse-based food products in different forms (masyaura, papad, sepubari, daal vada, kanji vada, idly, dosa, and uttapam) are commonly consumed in India and other developing countries (Tamang et al., 2016). Pulses are a rich source of vitamins and minerals compared to cereals, but they also contain a high level of phytates. The fermentation process increases the content and bioavailability of thiamine, niacin, phosphorus, energy, protein, and minerals (Kapravelou et al., 2020). Thus, accessions with high fermentability are desired not only for making local dishes but also to meet the nutritional needs in a better manner. In our study, we have identified high TSS content with a possibility of finding oligosaccharide-rich accessions. Oligosaccharides have a probiotic activity, help regulate gut microflora, and aid in synthesizing short-chain fatty acids and vitamins of the B group. Not much work has been done so far to study the TSS of cowpea, and this aspect needs to be explored in breeding programs and genetic improvement of the cowpea accessions, which will generally meet the needs of the market and consumer.
Phenols
The total phenolic content ranged from 0.026 g100 g−1 (EC724674) to 0.883 g 100 g−1 (EC109112) with a mean value of 0.272 g 100 g−1 (Table 1). The amount of polyphenol tends to be higher in brown and cream-colored (0.192–0.196 g 100 g−1) rather than white-colored seeds (0.099 g 100 g−1; Gonçalves et al., 2016) and is present mostly in seed coats. Polyphenolics determine the antioxidant capacity of legumes (Zhang et al., 2015) and differ across the cultivars in legumes, which might be due to genotypic variations and geographical conditions. Higher values observed in our samples are similar to the reported values of 0.544–0.844 g 100 g−1, while the lower values agree with 0.035–0.377 g 100 g−1 (Cai et al., 2003). Polyphenols cause a reduction in protein and starch digestibility and also act as a metal chelator (Hall et al., 2017). However, cowpea polyphenols have the most active DPPH radical scavenging activity, thus acting as hydrogen peroxide scavengers and strong antioxidants. Therefore, low and high phenol content has unique relevance from a nutritional perspective.
Phytic Acid Content
The content of phytic acid varied significantly across the different accessions and ranged from 0.690 g 100 g−1 (EC723824) to 1.88 g 100 g−1 (EC101970) with a mean value of 1.10 g 100 g−1 (Table 1), which is considerably higher than the phytic acid value of brown and white cowpea grains (0.550 g 100 g−1 and 0.573 g 100 g−1, respectively; Longvah et al., 2017). The values are quite similar to the results reported for Brazilian cowpea (0.870–1.26 g 100 g−1; Almeida et al., 2008) but considerably lower than the values reported in soy (3.17–3.90 g 100 g−1; Kumar et al., 2006). Phosphorus is mainly stored as phytic acid in the seeds, which is widely distributed in the grains of cereals and legumes. Humans lack digestive enzymes to hydrolyze phytate, as phytate binds to the minerals, thus limiting their bioavailability. Phytate is also known to inhibit the activities of food digestive enzymes, such as proteases and amylases (Pramitha et al., 2021); hence, low phytate-containing germplasm is much in demand among the crop breeders. The results show that cowpea has high phytate content, which can be reduced by cooking and long germination time (Jayathilake et al., 2018). In contrast, low phytate-containing accessions, such as EC723824 (0.690 g 100 g−1), EC240697 (0.797 g 100 g−1), and EC004218 (0.810 g 100 g−1), could be consumed regularly along with the staple food.
Statistical Analysis
Correlation Analysis
Correlation studies are extremely important for finding positive or negative values which indicate significant or non-significant relationships between the parameters. The substantial relationships between the nutritional indicators were determined using Pearson's correlation test. Pearson's r > 0 indicates a positive correlation, whereas r < 0 indicates a negative correlation with p < 0.05. Boost in a particular parameter leads to changes in the characteristics of other related parameters. The content of phytate, protein, TDF, phenols, TSS, starch, and amylose was evaluated to determine their correlation coefficients.
Table 2 shows the correspondence between seven biochemical parameters of 120 cowpea accessions, along with the exact values. It can be inferred that a highly significant and negative correlation was found between starch and protein content (r = −0.63, p < 0.001), where protein increases due to the inhibition of starch synthesis (Yu et al., 2017). The catalytic activity of starch synthases varies depending on the protein levels, thus resulting in changes in starch content. Such correlations could occur due to the nature of the starch–protein matrix.
A significant negative correlation was found between phenol and starch content (r = −0.326, p < 0.001), since phenolics interact with enzymes such as amylases, which is followed by a decrease in starch digestion in several legumes (Zhu, 2015). At the same time, phytic acid has a positive correlation with protein (r = 0.22, p < 0.05) and TDF (r = 0.238, p < 0.01). The positive correlation with protein could be attributed to the anionic phytic acid groups, which attach tightly to the cationic groups of protein, i.e., the ε-NH2 of lysine, the α-NH2 terminal group of the protein chain, the guanidyl group of arginine, and the imidazole group of histidine (Nkhata et al., 2018). TDF also tends to bind mineral ions, which might decrease the bioavailability of some mineral elements, similar to phytic acid, which explains the positive correlation between them (Nkhata et al., 2018). Phytic acid showed a negative and significant correlation with amylose (r = −0.226, p < 0.05), which is similar to the results reported by Sivakumaran et al. (2018) in legumes. The relationship between the traits indicates that it is possible to find accessions with low starch content and higher dietary fiber, protein, phytate, and phenol levels. High phenolic, tannin, and phytate levels are associated with enzyme inhibition and prevent the interaction of amylases with the starch molecule. Accessions with low starch and high dietary fiber, protein, phytate, and phenol content are likely to have low GI and impair the release of glucose from other food components consumed with it.
Principal Component Analysis
The PCA was used to correlate the findings obtained for different parameters, such as protein content, starch content, amylose content, TDF, TSS, phenolic profile, and phytic acid. Loadings were studied to identify the underlying link between the data structure after representative PCs were established based on sample grouping/differentiation and variance explained. An Eigenvalue is assigned to each PC that accounts for a fraction of the variation in the dataset. Each Eigenvalue has an eigenvector linked to it, which defines the variation within the main components. A Scree plot was used to plot the eigenvalues of factors or principal components. The scree plot containing the principal components based on eigenvalues (>1) is shown in Figure 1A. Three components have been identified (Table 3), where phytic acid (FL2: 0.708), TSS (FL3: 0.712), phenol (FL3: 0.677), protein (FL1: 0.878), and TDF (FL2: 0.636) have large positive factor loading values, which indicate that the specific values will be higher on the positive axis (Figure 1B). Correspondingly, starch (FL1: −0.886) and amylose (FL2: −0.662; FL3: −0.454) have negative factor loading values, indicating that the values are higher on the negative axis (Figure 1B).
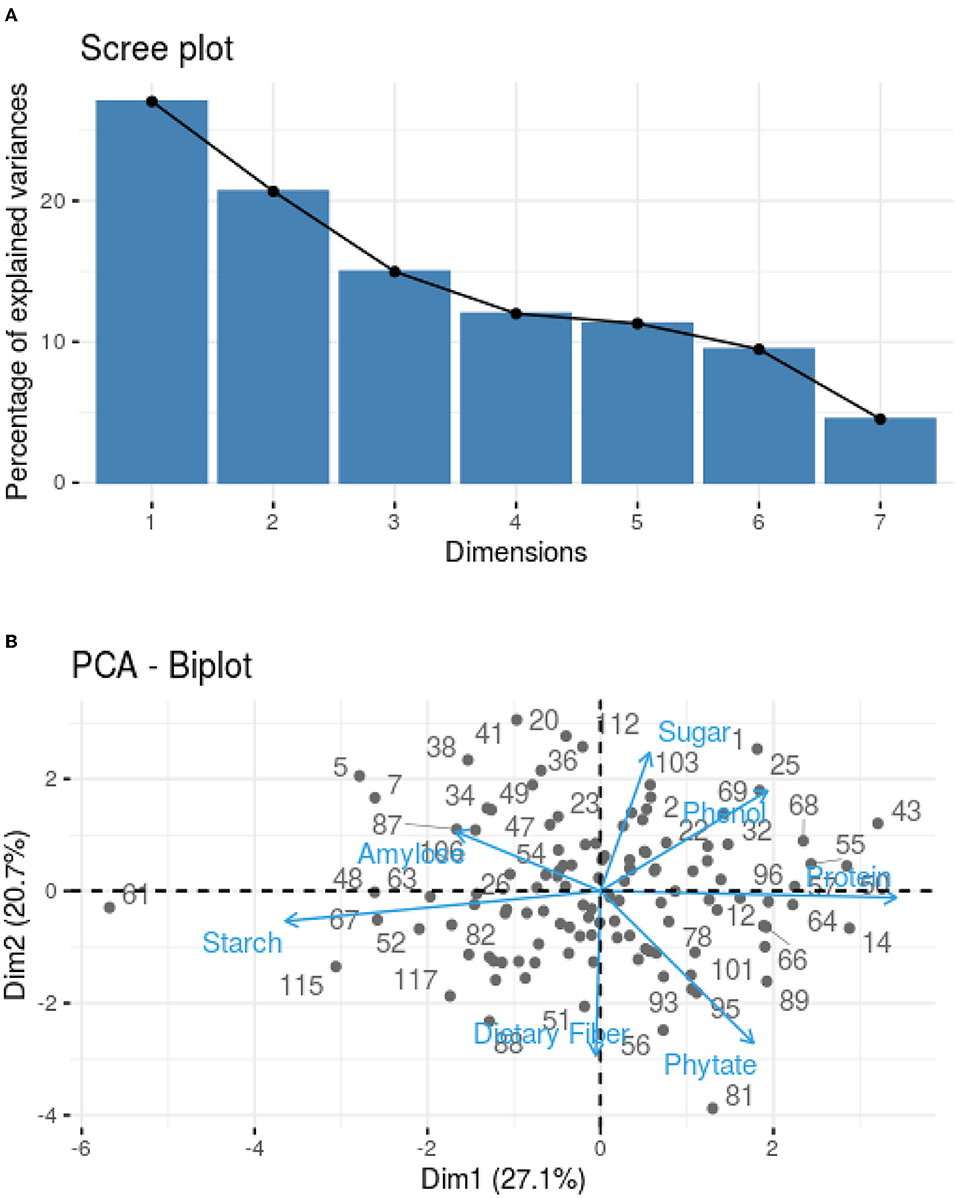
Figure 1. (A) Scree plot explaining principal component variances in terms of components. (B) PCA biplot indicating the distribution of nutritional traits in 120 samples based on their calculated component loading values for phytic acid, starch, phenols, TSS, protein, TDF, and amylose.
Nearly, all the useful data are present in the first few PCs, while noise is mostly present in the remaining PCs (Chaudhry et al., 2018). As in our study, 62.7% of the overall variation was accounted for by the first three main components (PC1, PC2, and PC3; eigenvalues >1; Figure 1A), which is in agreement with the studies conducted in maize hybrids where the remaining principal components accounted for a minimal variability (Ali et al., 2015). Protein (0.878) is the major contributor to the variability, and on the negative axis, starch (−0.886) contributes to the maximum variation in PC1, accounting for 27.05% of the total variation (Table 3). Phytic acid (0.708) followed by TDF (0.636) contributed to the maximum variation, whereas amylose (−0.662) contributed negatively in PC2 (Table 3), accounting for 20.68% of the total variation. The third principal component (PC3) accounted for 14.98% of the total variation, where maximum variability was contributed by TSS (0.712), followed by phenols (0.677), and negatively contributed by amylose (−0.454) (Table 3). The first six principal components explained 95.5% of the variability within the observations, where PC1, PC2, PC3, PC4, PC5, and PC6 showed 27.05, 20.68, 14.98, 12.00, 11.30, 9.47, and 4.52% of the total variation, respectively, as given in Supplementary Table 2.
The dominant factors showed high component loadings along with low uniqueness values. Uniqueness defines the fraction of variation that is unique to a single variable and cannot be explained by PC, while loading establishes the contribution of each variable to PC. Thus, the larger the contribution of a given variable to PCA, the lower the uniqueness value. According to Figure 1B, the lower angle between the two vectors represents the positive correlation between the traits. When the vectors make 90°, they are less likely to be correlated, and when the vectors make 180°, the traits are negatively correlated. Thus, accessions could be grouped based on the relationship between variables and the uniqueness of dominant factors.
Hierarchical Clustering Analysis
Hierarchical clustering analysis was employed to evaluate the multivariate association between biochemical characteristics of accessions being assessed. The accessions were divided into six clusters using Ward's method at a square Euclidian distance of 5 and are shown as a dendrogram in Supplementary Figure 3. Table 4 depicts the accessions that are divided into six clusters, along with the mean values of their nutritional properties.
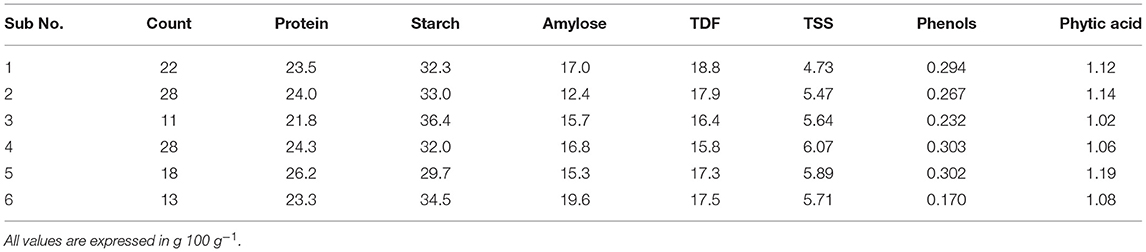
Table 4. Number of accessions and mean values of six clusters for seven different biochemical traits.
Figure 2 illustrates the grouping of accessions into six clusters, where cluster I contains 22 accessions characterized by a low amount of TSS (4.73 g 100 g−1), a high amount of TDF (18.8 g 100 g−1), and a moderate level of other constituents. Cluster II contains 28 accessions characterized by a low amount of amylose (12.4 g 100 g−1) and a moderate level of other constituents. Cluster III contains 11 accessions characterized by a low amount of phytate (1.02 g 100 g−1) and protein (21.8%), a higher amount of starch (36.4 g 100 g−1), and a moderate level of other constituents. Cluster IV contains 28 accessions characterized by a low amount of phytic acid (1.06 g 100 g−1), a higher amount of TSS (6.07 g 100 g−1) and phenol (0.303 GAE g 100 g−1), and a moderate level of other constituents. Cluster V contains 18 accessions characterized by a higher amount of phytic acid (1.19 g 100 g−1), protein (26.2%), TSS (5.89 g 100 g−1), and phenol (0.302 GAE g 100 g−1) and a lower amount of starch (29.7 g 100 g−1). Cluster VI contains 13 accessions characterized by a higher amount of amylose (19.5 g 100 g−1), a lower amount of phenol (0.170 GAE g 100 g−1), and a moderate level of other constituents.
Germplasm of clusters IV and V had higher TSS content, and cluster V contained a high amount of protein; the accessions of these clusters could be used as a source for designing high protein and palatable food, suitable for use as a protein supplement, for making protein isolates, and useful for combating protein-energy malnutrition in the developing countries. Accessions of cluster III contain high starch content, and cluster VI contains higher amylose content, and these characteristics contribute to the best physico-functional features. Therefore, these clusters might be useful in making processed products, confectionery items, and legume starch in the food processing industry. In comparison, owing to the high TDF and amylose content in clusters I and VI, respectively, they could be helpful in making low GI foods. Accessions from both of these clusters are suitable for persons with cardiovascular ailments and diabetes. Accessions of cluster II have low amylose content and high swelling power, and hence are preferred for ease in cooking. Clusters III and VI contain a lower amount of phytic acid and phenol, respectively, which can be used as functional ingredients and for food diversification. This information could help in the identification of eligible parents in the cowpea improvement programs for improving the nutritional properties.
The studied cowpea accessions have good nutritional properties which could be exploited for nutrition and food formulations. Some nutri-dense accessions have been found that can be used in different areas of the food industry and crop improvement programs. EC169879 and IC201086 (cluster V) have high protein, TSS, amylose, and TDF with low starch content. These accessions can be used to make high-protein foods and feeds and can be used for people with obesity, cardiovascular diseases, and type-II diabetes, because of their high amylose and TDF content. Several authors have discussed the use of legume flours as components in diverse culinary products, such as bread, biscuits, pasta, tortillas, and doughnuts (Naiker et al., 2019). Keeping this information in mind, EC724681, EC240663, and EC472284 (cluster III) can be used for this purpose owing to their high starch content. Except for EC240663, the other two accessions also have high amylose and high TDF content. The food products prepared from these accessions will have a low glycemic index, ultimately helping diabetic and obese patients. Accessions like IC214752, IC249141, and EC 367678 (cluster II) have low amylose and high TDF and protein content, which will contribute to higher swelling capacity and solubility index (Ashogbon and Akintayo, 2012), and lower syneresis rate (Naiker et al., 2019). These properties can further help to increase the shelf life, texture, and frozen quality of processed food products.
Conclusion
The results of the present study in cowpea have revealed good nutritional properties, with high variability in the given accessions with respect to seed morphology and nutritional traits studied. Starch, followed by protein, TSS, and phytic acid, are the major contributors to the variability observed in the accessions, as revealed by three components of PCA. Many nutrient-dense accessions were found to have more than one trait with high nutritional values, which are present in six different clusters as revealed by HCA. Based on MVA, unique and trait-specific accessions having different combinations with respect to nutritional traits are identified. These accessions can be directly selected as parents in crop improvement programs to produce cowpea varieties with specific applications for targeted nutrition and food processing requirements. The identified germplasm will add to widening the genetic foundation in the cowpea gene pool and breeding programs.
Data Availability Statement
The original contributions presented in the study are included in the article/Supplementary Material, further inquiries can be directed to the corresponding author.
Author Contributions
SP, AB, and RJ contributed to biochemical profiling and preparing of the draft. KT provided agronomically diverse cowpea accessions. KG, SA, DW, and GM contributed to the review and critical evaluation of the manuscript. SK contributed to statistical analysis and interpretation. RB contributed to conceptualization, planning, and supervision of the study. All authors contributed to the article and approved the submitted version.
Funding
This study was supported by funding from the Department of Biotechnology, Government of India, under the project on minor pulses (No. BT/Ag/Network/Pulse-I /2017-18, date:187 24th October 2018) and in-house project on Biochemical evaluation of Field and vegetable crops, and Junior Research Fellowship granted to the first author by ICAR Research [Grant No. 2(9)/2018-HRD, dated 30th October 2018].
Conflict of Interest
The authors declare that the research was conducted in the absence of any commercial or financial relationships that could be construed as a potential conflict of interest.
Publisher's Note
All claims expressed in this article are solely those of the authors and do not necessarily represent those of their affiliated organizations, or those of the publisher, the editors and the reviewers. Any product that may be evaluated in this article, or claim that may be made by its manufacturer, is not guaranteed or endorsed by the publisher.
Acknowledgments
Authors acknowledge the support received from HOD DGE, HOD DGC, and Dr. Neeta Singh DGC, Professor in PGR, PG School, ICAR-IARI, in carrying out these studies.
Supplementary Material
The Supplementary Material for this article can be found online at: https://www.frontiersin.org/articles/10.3389/fsufs.2022.888041/full#supplementary-material
Supplementary Figure 1. NIRS spectra of 475 cowpea samples.
Supplementary Figure 2. Range of variability for protein, starch, amylose, TDF, TSS, phenols, and phytic acid of 120 cowpea germplasm lines are represented by box-and-whisker plots.
Supplementary Figure 3. Cluster dendrogram of 120 cowpea accessions for seven different biochemical traits.
Supplementary Table 1. Nutritional composition of 120 cowpea germplasm lines.
Supplementary Table 2. Initial eigenvalues of PCA.
Abbreviations
C, Celsius; EC, Exotic collection; FCR, Folin-Ciocalteu reagent; g, grams; GAE, Gallic acid equivalent; GOPOD, Glucose oxidase/peroxidase; ha, Hectare; IC, Indigenous collection; mg, milligrams; ml, milliliters; mm, millimeters; NIRS, Near-infrared spectroscopy; nm, nanometer; PCA, Principal component analysis; rpm, Revolutions per minute; TSS, Total soluble sugars; TDF, Total dietary fiber; HCA, Hierarchical clustering analysis; PCA, Principal component analysis; AOAC, Association of official analytical chemists; UV-Vis, Ultra violet–Visible; KI, Potassium iodide; I2, Iodine; SD, Standard deviation; DPPH, 2, 2-diphenyl-1-picrylhydrazyl; WHO, World Health Organization; PC, Principal component; FL, Factor loading.
Footnotes
1. ^www.fao.org/faostat/en/#data/QC
References
Adebooye, O. C., and Singh, V. (2008). Physico-chemical properties of the flours and starches of two cowpea varieties [Vigna unguiculata (L.) Walp]. Innov. Food Sci. Emerg. Technol. 9, 92–100. doi: 10.1016/j.ifset.2007.06.003
Akissoé, L., Madodé, Y. E., Hemery, Y. M., Donadjè, B. V., Icard-Vernière, C., Hounhouigan, D. J., et al. (2021). Impact of traditional processing on proximate composition, folate, mineral, phytate, and alpha-galacto-oligosaccharide contents of two West African cowpea (Vigna unguiculata L. Walp) based doughnuts. J. Food Compos. Anal. 96:103753. doi: 10.1016/j.jfca.2020.103753
Ali, A., Al-Saady, N. A., Waly, M. I., Bhatt, N., Al-Subhi, A. M., and Khan, A. J. (2013). Evaluation of indigenous Omani legumes for their nutritional quality, phytochemical composition and antioxidant properties. Int. J. Postharvest Technol. Innov. 3, 333–346. doi: 10.1504/IJPTI.2013.060267
Ali, F., Kanwal, N., Ahsan, M., Ali, Q., Bibi, I., and Niazi, N. K. (2015). Multivariate analysis of grain yield and its attributing traits in different maize hybrids grown under heat and drought stress. Scientifica 2015:563869. doi: 10.1155/2015/563869
Almeida, D. T., Greiner, R., Furtunado, D. M., Trigueiro, I. N., and Araújo, M. D. P. N. (2008). Content of some antinutritional factors in bean cultivars frequently consumed in Brazil. Int. J. Food Sci. Technol. 43, 243–249. doi: 10.1111/j.1365-2621.2006.01426.x
Antova, G. A., Stoilova, T. D., and Ivanova, M. M. (2014). Proximate and lipid composition of cowpea (Vigna unguiculata L.) cultivated in Bulgaria. J. Food Compos. Anal. 33, 146–152. doi: 10.1016/j.jfca.2013.12.005
Appiah, F., Asibuo, J. Y., and Kumah, P. (2011). Physicochemical and functional properties of bean flours of three cowpea (Vigna unguiculata L. Walp) varieties in Ghana. Afr. J. Food Sci. 5, 100–104. doi: 10.17660/ActaHortic.2011.911.51
Ashogbon, A. O., and Akintayo, T. E. (2012). Isolation, composition, morphological and pasting properties of starches from rice cultivars grown in Nigeria. Starch-Stärke 64, 181–187. doi: 10.1002/star.201100044
Awika, J. M., and Duodu, K. G. (2017). Bioactive polyphenols and peptides in cowpea (Vigna unguiculata) and their health promoting properties: a review. J. Funct. Foods. 38, 686–697. doi: 10.1016/j.jff.2016.12.002
Boukar, O., Massawe, F., Muranaka, S., Franco, J., Maziya-Dixon, B., Singh, B., et al. (2011). Evaluation of cowpea germplasm lines for protein and mineral concentrations in grains. Plant Genet. Resour. 9, 515–522. doi: 10.1017/S1479262111000815
Cai, R., Hettiarachchy, N. S., and Jalaluddin, M. (2003). High-performance liquid chromatography determination of phenolic constituents in 17 varieties of cowpeas. J. Agric. Food Chem. 51, 1623–1627. doi: 10.1021/jf020867b
Chaudhry, M. M., Amodio, M. L., Babellahi, F., de Chiara, M. L., Rubio, J. M. A., and Colelli, G. (2018). Hyperspectral imaging and multivariate accelerated shelf-life testing (MASLT) approach for determining shelf life of rocket leaves. J. Food Eng. 238, 122–133. doi: 10.1016/j.jfoodeng.2018.06.017
Dubois, M., Gilles, K. A., Hamilton, J. K., Rebers, P. T., and Smith, F. (1956). Colorimetric method for determination of sugars and related substances. Anal. Chem. 28, 350–356. doi: 10.1021/ac60111a017
Elharadallou, S. B., Khalid, I. I., Gobouri, A. A., and Abdel-Hafez, S. H. (2015). Amino acid composition of cowpea (Vigna ungiculata L. Walp) flour and its protein isolates. Food Nutr. Sci. 6:790. doi: 10.4236/fns.2015.69082
FAO (2019). Available online at: www.fao.org/faostat/en/#data/QC
Feizollahi, E., Mirmahdi, R. S., Zoghi, A., Zijlstra, R. T., Roopesh, M. S., and Vasanthan, T. (2021). Review of the beneficial and anti-nutritional qualities of phytic acid, and procedures for removing it from food products. Food Res. Int. 143:110284. doi: 10.1016/j.foodres.2021.110284
Ghavidel, R. A., and Prakash, J. (2007). The impact of germination and dehulling on nutrients, antinutrients, in vitro iron and calcium bioavailability and in vitro starch and protein digestibility of some legume seeds. LWT. 40, 1292–1299. doi: 10.1016/j.lwt.2006.08.002
Giridhar, K., Raju, P. S., Pushpalatha, G., and Patra, C. (2020). Effects of plant density on yield parameters of cowpea (Vigna unguiculata L.). Int. J. Chem. Stud. 8, 344–347. doi: 10.22271/chemi.2020.v8.i4f.10090
Gonçalves, A., Goufo, P., Barros, A., Domínguez-Perles, R., Trindade, H., Rosa, E. A., et al. (2016). Cowpea (Vigna unguiculata L. Walp), a renewed multipurpose crop for a more sustainable agri-food system: nutritional advantages and constraints. J. Sci. Food Agric. 96, 2941–2951. doi: 10.1002/jsfa.7644
Hall, C., Hillen, C., and Garden Robinson, J. (2017). Composition, nutritional value, and health benefits of pulses. Cereal. Chem. 94, 11–31. doi: 10.1094/CCHEM-03-16-0069-FI
Harlan, J. R (1971). Agricultural origins: centres and noncentres. Science 174, 468–474. doi: 10.1126/science.174.4008.468
Hoover, R., Hughes, T., Chung, H. J., and Liu, Q. (2010). Composition, molecular structure, properties, and modification of pulse starches: a review. Food Res. Int. 43, 399–413. doi: 10.1016/j.foodres.2009.09.001
Jayathilake, C., Visvanathan, R., Deen, A., Bangamuwage, R., Jayawardana, B. C., Nammi, S., et al. (2018). Cowpea: an overview on its nutritional facts and health benefits. J. Sci. Food Agric. 98, 4793–4806. doi: 10.1002/jsfa.9074
Joint, W. H. O., and Consultation, F. E. (2018). Diet, Nutrition and the Prevention of Chronic Diseases. WHO Technical Report Series: Geneva.
Kalogeropoulos, N., Chiou, A., Ioannou, M., Karathanos, V. T., Hassapidou, M., and Andrikopoulos, N. K. (2010). Nutritional evaluation and bioactive microconstituents (phytosterols, tocopherols, polyphenols, triterpenic acids) in cooked dry legumes usually consumed in the Mediterranean countries. Food Chem. 121, 682–690. doi: 10.1016/j.foodchem.2010.01.005
Kapravelou, G., Martínez, R., Martino, J., Porres, J. M., and Fernández-Fígares, I. (2020). Natural fermentation of cowpea (Vigna unguiculata) flour improves the nutritive utilization of indispensable amino acids and phosphorus by growing rats. Nutr. 12:2186. doi: 10.3390/nu12082186
Kim, Y. Y., Woo, K. S., and Chung, H. J. (2018). Starch characteristics of cowpea and mungbean cultivars grown in Korea. Food Chem. 263, 104–111. doi: 10.1016/j.foodchem.2018.04.114
Kumar, V., Rani, A., Solanki, S., and Hussain, S. M. (2006). Influence of growing environment on the biochemical composition and physical characteristics of soybean seed. J. Food Compos. Anal. 19, 188–195. doi: 10.1016/j.jfca.2005.06.005
Longvah, T., Anantan, I., Bhaskarachary, K., Venkaiah, K, and Longvah, T. (2017). Indian Food Composition Tables. Hyderabad: National Institute of Nutrition, Indian Council of Medical Research, 2–58.
Madodé, Y. E., Houssou, P. A., Linnemann, A. R., Hounhouigan, D. J., Nout, M. J. R., and Van Boekel, M. A. J. S. (2011). Preparation, consumption, and nutritional composition of West African cowpea dishes. Ecol. Food Nutr. 50, 115–136. doi: 10.1080/03670244.2011.552371
Mamiro, P. S., Mbwaga, A. M., Mamiro, D. P., Mwanri, A. W., and Kinabo, J. L. (2011). Nutritional quality and utilization of local and improved cowpea varieties in some regions in Tanzania. African J. Food Agric. Nutr. Dev. 11:65876. doi: 10.4314/ajfand.v11i1.65876
Mbuma, N. W., Gerrano, A. S., Lebaka, N., Amoo, S., Mofokeng, A., and Labuschagne, M. (2021). Variability in the concentration of mineral elements and phytochemical contents of cowpea genotypes for crop improvement. Acta Agric. Scand. B Soil Plant Sci. 71, 132–144. doi: 10.1080/09064710.2020.1859609
Michałowski, T., Asuero, A. G., and Wybraniec, S. (2013). The titration in the kjeldahl method of nitrogen determination: base or acid as titrant. J. Chem. Educ. 90, 191–197. doi: 10.1021/ed200863p
Naiker, T. S., Gerrano, A., and Mellem, J. (2019). Physicochemical properties of flour produced from different cowpea (Vigna unguiculata) cultivars of Southern African origin. J. Food Sci. Technol. 56, 1541–1550. doi: 10.1007/s13197-019-03649-1
Nassourou, M. A., Noubissié, T. J. B., Njintang, Y. N., and Bell, J. M. (2017). Diallel analyses of soluble sugar content in cowpea (Vigna unguiculata L. Walp.). Crop J. 5, 553–559. doi: 10.1016/j.cj.2017.05.005
Nikmaram, N., Leong, S. Y., Koubaa, M., Zhu, Z., Barba, F. J., Greiner, R., et al. (2017). Effect of extrusion on the anti-nutritional factors of food products: an overview. Food Control 79, 62–73. doi: 10.1016/j.foodcont.2017.03.027
Nkhata, S. G., Ayua, E., Kamau, E. H., and Shingiro, J. B. (2018). Fermentation and germination improve nutritional value of cereals and legumes through activation of endogenous enzymes. Food Sci. Nutr. 6, 2446–2458. doi: 10.1002/fsn3.846
Oghbaei, M., and Prakash, J. (2016). Effect of primary processing of cereals and legumes on its nutritional quality: a comprehensive review. Cogent. Food Agric. 2:1136015. doi: 10.1080/23311932.2015.1136015
Omomowo, O. I., and Babalola, O. O. (2021). Constraints and prospects of improving cowpea productivity to ensure food, nutritional security and environmental sustainability. Front. Plant Sci. 12:751731. doi: 10.3389/fpls.2021.751731
Perez, C. M., and Juliano, B. O. (1978). Modification of the simplified amylose test for milled rice. Starch-Stärke. 30, 424–426. doi: 10.1002/star.19780301206
Petroni, K., Landoni, M., Tomay, F., Calvenzani, V., Simonelli, C., and Cormegna, M. (2017). Proximate composition, polyphenol content and anti-inflammatory properties of white and pigmented Italian rice varieties. Univers. J. Agric. Res. 5, 312–321. doi: 10.13189/ujar.2017.050509
Phillips, R. D., Saalia, F. K., and Affrifah, N. S. (2022). Cowpea composition, processing, and products. Dry Beans Pulses 13, 331–358. doi: 10.1002/9781119776802.ch13
Pramitha, J. L., Rana, S., Aggarwal, P. R., Ravikesavan, R., Joel, A. J., and Muthamilarasan, M. (2021). Diverse role of phytic acid in plants and approaches to develop low-phytate grains to enhance bioavailability of micronutrients. Adv. Genet. 107, 89–120. doi: 10.1016/bs.adgen.2020.11.003
Ren, Y., Yuan, T. Z., Chigwedere, C. M., and Ai, Y. (2021). A current review of structure, functional properties, and industrial applications of pulse starches for value-added utilization. Compr. Rev. Food Sci. Food Saf. 20, 3061–3092. doi: 10.1111/1541-4337.12735
Singleton, V. L., Orthofer, R., and Lamuela-Raventós, R. M. (1999). Analysis of total phenols and other oxidation substrates and antioxidants by means of folin-ciocalteu reagent. Methods Enzymol. 299, 152–178. doi: 10.1016/S0076-6879(99)99017-1
Sivakumaran, K., Wansapala, J., and Herath, T. (2018). Total phosphorous, phytate phosphorous contents and the correlation of phytates with amylose in selected edible beans in Sri Lanka. Potravinarstvo 12:812. doi: 10.5219/812
Tamang, J. P., Thapa, N., Bhalla, T. C., and Savitri (2016). “Ethnic fermented foods and beverages of India,” in Ethnic Fermented Foods and Alcoholic Beverages of Asia, ed J. Tamang (New Delhi: Springer). doi: 10.1007/978-81-322-2800-4_2
The Jamovi Project (2020). Jamovi. (Version 1.6.9) [Computer Software] 2020. Available online at: https://www.jamovi.org (accessed September 2021).
Tomar, M., Bhardwaj, R., Kumar, M., Singh, S. P., Krishnan, V., Kansal, R., et al. (2021). Nutritional composition patterns and application of multivariate analysis to evaluate indigenous Pearl millet [Pennisetum glaucum (L.) R. Br.] germplasm. J. Food Compos. Anal. 103:104086. doi: 10.1016/j.jfca.2021.104086
Wani, A. A., Singh, P., Shah, M. A., Schweiggert-Weisz, U., Gul, K., and Wani, I. A. (2012). Rice starch diversity: effects on structural, morphological, thermal, and physicochemical properties—a review. Compr. Rev. Food Sci. Food Saf. 11, 417–436. doi: 10.1111/j.1541-4337.2012.00193.x
Weng, Y., Ravelombola, W. S., Yang, W., Qin, J., Zhou, W., Wang, Y. J., et al. (2018). Screening of seed soluble sugar content in cowpea [Vigna unguiculata (L.) Walp]. Am. J. Plant Sci. 9, 1455–1466. doi: 10.4236/ajps.2018.97106
Yu, W., Tan, X., Zou, W., Hu, Z., Fox, G. P., Gidley, M. J., et al. (2017). Relationships between protein content, starch molecular structure and grain size in barley. Carbohydr. Polym. 155, 271–279. doi: 10.1016/j.carbpol.2016.08.078
Zhang, B., Deng, Z., Ramdath, D. D., Tang, Y., Chen, P. X., Liu, R., et al. (2015). Phenolic profiles of 20 Canadian lentil cultivars and their contribution to antioxidant activity and inhibitory effects on α-glucosidase and pancreatic lipase. Food Chem. 172, 862–872. doi: 10.1016/j.foodchem.2014.09.144
Zhu, F (2015). Interactions between starch and phenolic compound. Trends. Food Sci. Technol. 43, 129–143. doi: 10.1016/j.tifs.2015.02.003
Keywords: legumes, nutritional profiling, diversity, HCA, PCA, NIRS assisted sample selection
Citation: Padhi SR, Bartwal A, John R, Tripathi K, Gupta K, Wankhede DP, Mishra GP, Kumar S, Archak S and Bhardwaj R (2022) Evaluation and Multivariate Analysis of Cowpea [Vigna unguiculata (L.) Walp] Germplasm for Selected Nutrients—Mining for Nutri-Dense Accessions. Front. Sustain. Food Syst. 6:888041. doi: 10.3389/fsufs.2022.888041
Received: 02 March 2022; Accepted: 18 April 2022;
Published: 19 May 2022.
Edited by:
Uma Tiwari, Technological University Dublin, IrelandReviewed by:
Vijay Kumar Yadav, Indian Grassland and Fodder Research Institute (ICAR), IndiaAjit Singh, University of Nottingham Malaysia Campus, Malaysia
Copyright © 2022 Padhi, Bartwal, John, Tripathi, Gupta, Wankhede, Mishra, Kumar, Archak and Bhardwaj. This is an open-access article distributed under the terms of the Creative Commons Attribution License (CC BY). The use, distribution or reproduction in other forums is permitted, provided the original author(s) and the copyright owner(s) are credited and that the original publication in this journal is cited, in accordance with accepted academic practice. No use, distribution or reproduction is permitted which does not comply with these terms.
*Correspondence: Rakesh Bhardwaj, cmFrZXNoLmJoYXJkd2FqMUBpY2FyLmdvdi5pbg==