- Department of Plant Science, McGill University, Montreal, QC, Canada
Currently, salinity is the second biggest challenge in the world after drought and affects all stages of plant growth. The use of environmentally friendly methods such as microorganisms and their derivatives can reduce the destructive effects of salinity stress. A growth chamber experiment was conducted to determine the effects of cell-free supernatant (CFS) from Bacillus strains on germination of corn under salinity stress. Corn seeds were subjected to three salinity levels (0, 100 and 150 mM of NaCl), cell-free supernatant of Bacillus strains (U35, U47, U48, U49, and U50) at two levels of dilution (1:50 and 1:250). Germination percentage and rate decreased with increasing salinity toward 150 mM NaCl all together leading to suppressed growth variables for corn seed seedlings including fresh and dry weight of radicle (47.71 and 52.63%, respectively), and shoot (49.52 and 49.25%, respectively), radicle and shoot lengths (39.90 and 66.07%, respectively). Seed vigor index also decreased by 63.04% at 150 mM NaCl. Contrary to salinity, the CFSs of Bacillus strains increased all the growth traits of corn seeds and reduced the negative effects of salinity, especially severe salinity. Ratios of 1:50 and 1: 250 gave best performance for CFSs from U35 and U50, respectively. In general, the highest seed vigor index was obtained by application of 1: 250 CFS from U50. Most germination traits and seed vigor index correlated significantly positive; however, mean germination time was negatively and significantly correlated with the seed vigor index of corn. The results showed that cell-free supernatant use, may as well-helped in changing the ratios of phytohormones, ROS, the activity of antioxidant enzymes and osmotic proteins, hence reduce the negative effects of salinity and improve seed vigor index which eventually increases the ability of plant seedling establishment under saline conditions.
Introduction
At this time, increasing crop production to match a rising global population is becoming increasingly difficult due to land degradation, water scarcity and pollution, soil salinity and climatic conditions (Tyczewska et al., 2018; Shahid et al., 2020; Shah et al., 2021). It has been estimated that by 2050 about 9 billion humans will live on Earth, implying that food insecurity, global hunger and food prices will become concerning possibilities for many more people (Payumo et al., 2018; Shah et al., 2021). With climate change, land erosion and desertification, extreme temperatures and poor-quality water, soils are losing crucial nutrients and minerals in addition to water-holding capacity (Tyczewska et al., 2018; Shahid et al., 2020). To counter these effects, farmers are using fertilizers to replenish soil mineral nutrients, and are irrigating agricultural lands; however, chemical inputs contribute to soil and water pollution, and poor-quality water used for irrigation increases soil salinity (Shahid et al., 2020).
Corn is an important cereal crop for both humans and livestock. It has nutritional and health benefits as it is a good source of carbohydrates and has medical value, plus it can be used as raw material for industry (Kumar and Narayan, 2013). Continued development in breeding and genetic engineering, makes corn today differ substantially from the plant our ancestors began domesticating many thousands of years ago. The combination of genetic modification coupled with agricultural technologies has improved significantly production of corn and other crops, and more recently, bio inoculants have begun to enter the market (Tyczewska et al., 2018).
Plant growth promoting rhizobacteria (PGPR) is known to improve crop production by enhancing plant growth and development, providing biotic and abiotic stress tolerance. PGPR helps plants in nutrients acquisition and reduce pathogenic microorganisms by competing for niche space and nutrients, increasing root surface area, boosting plant immunity and producing anti-pathogen compounds (Backer et al., 2018; Basu et al., 2021; Lyu et al., 2021a,b). In fact, PGPR can be considered as a new low-input, environmentally friendly technology set able to be deployed after the pesticides and fertilizers of the Green Revolution. PGPR have shown meaningful benefits to a variety of crops. For example, different bacterial strains have been shown to provide salt and drought resistance to corn, along with phytoremediation of heavy metals and improvement of seed germination (Basu et al., 2021). Many crops and terrestrial plants are glycophytes, a category of plants less adapted to salt stress, PGPR represent a viable solution, plus being eco-friendly, sustainable, biodegradable, safe to use, and economic (Kumari et al., 2019; Prasad et al., 2019; Shahid et al., 2020; Shah et al., 2021).
Plant growth promotion by PGPR would not be possible without interspecies signaling, given that communication in the rhizosphere is often key for the plant-microbe symbiosis establishment (Shah et al., 2021). Plant-to-microbe signaling has been involved in microorganism proliferation, gene expression and release of signals; likewise, microbe-to-plant signaling has influenced plant gene expression, physiology, immunity and intake of nutrients (Venturi and Keel, 2016).
For example during legume-rhizobia symbiosis establishment, the plant secretes flavonoids which trigger nod genes in the rhizobium bacteria. These genes allow the bacteria to produce lipo-chitooligosaccharides (LCOs), nodulation factors that are essential for root nodule formation. With chemoreceptors on the outermost root cells, plants can sense LCOs, which provoke mechanisms leading to formation of cells specialized in fixing atmospheric nitrogen (Venturi and Keel, 2016; Shah et al., 2021). Although plant-to-microbe and microbe-to-plant signaling has been elaborated in some circumstances, it is crucial to understand the initiation and whole process of a successful symbiosis. Having said that, the system relies on a fragile balance as temperature, lighting and soil type, age, pH, and fertility can affect exudation release, levels and recognition by others (Ortíz-Castro et al., 2009; Khan et al., 2020; Shah et al., 2021). There is more to discover in plant-microbe signaling, yet research in this area is flourishing as understanding interspecies chemical communication is likely to improve PGPR effects and expand their application in agricultural settings.
With the challenges to crop production systems resulting from climate change, PGPR is one of the prime option to overcome food security issues and contribute to the development of climate change resilient cropping systems. Nevertheless, PGPR represents a fragment of the products available on the market when compared to synthetic and chemical substances (Basu et al., 2021). Speculations are that the native soil and microbiome can affect the interactions and survival conditions of introduced microbes; however, researchers still lack a clear understanding of the modes of action once new microbes are introduced into the soil since rhizobacteria possess a range of potential mechanisms (Vejan et al., 2019; Basu et al., 2021; Shah et al., 2021). Finally, there are still many PGPR strains to discover given that researchers have only unfolded a corner of this complex living origami (Prasad et al., 2019).
Given the circumstances just described, this paper seeks to demonstrate that plant growth promoting rhizobacteria, and materials the produce, can improve germination of corn under saline stress conditions, by using a consortium of 5 Bacillus strains and creating medium and severe saline conditions with sodium chloride.
Materials and Methods
Experiment Design
Two separate experiments were conducted to study the effects of CFS of Bacillus strains on seed vigor index of corn (Zea mays L var DL1380) under saline stress and compared with a control treatment. Experiments were conducted in a growth chamber, at the Macdonald Campus of McGill University in 2021. The first experiment with a CFS dilution ratio of 1:50 (1 mL CFS of Bacillus strains and 50 mL distilled water) and the second experiment with a ratio of 1:250 (1 mL CFS of Bacillus strains and 250 mL distilled water) were performed simultaneously. The experiments were arranged as factorial, based on a completely randomized design with five replications, with three NaCl levels (0, 100, and 150 mM) and five Bacillus strains (U35, U47, U48, U49, and U50). The moisture content of the seed lot was determined as 5.7% by grinding the seeds and then drying at 103 ± 2°C for 17 h (ISTA 2010). Corn seeds were kept at 4°C for a minimum of 2 days in the refrigerator for stratification before sowing. After stratification, corn seeds were surface sterilized following a method by The seed sample was divided into six subsamples.
Bacterial Strains Growth Conditions
The consortium consists of 5 Bacillus strains (U35, U47, U48, U49, and U50) supplied by Ulysse Biotech (Trois-Rivières, Québec). Frozen glycerol stocks were revived, the cultures were used to inoculate 5 mL of growth mediums (M-13 liquid, plate count Agar) at an incubation temperature of 30°C. Autoclaving at 121°C for 20 min was completed to ensure sterilization of the growth mediums, and subsequently, solidification in Petri dishes was performed. Bacterial strains were transferred, with a streaking loop, onto the solid growth medium, and after an incubation of 3 days at 30°C, single colonies were cultured in broth (M-13 liquid) after a careful selection and sampling.
Harvesting CFS of Bacillus Strains
Cultures were collected after 4–5 days of incubation (after reaching 1 × 108 CFU). It was then transferred to centrifuge bottles and centrifuged at 11,000 rpm for 30 min. Retaining the pellet, the supernatant was passed through a sterile syringe filter unit (Fisherbrand, mixed cellulose esters, 0.22 μm and polyethersulfone: 0.22 μm, US) to obtain CFS.
Seed Germination Test Protocol
Ten sterilized corn seeds were placed in Petri dishes (sterile 100 x 15 mm polystyrene Petri dishes) with sheets of filter paper (Fisherbrand™–P8 Grade, Pittsburgh, US). moistened with either 5 mL of sterile distilled water (without CFS) acting as the control or solutions containing the CFS or the saline (NaCl) solutions. Petri dishes were put in a thin polyethylene bag to avoid drying caused by evaporation. Corn seeds were germinated in growth chambers at 25°C with a relative humidity of 70% and 24 h darkness, as it was determined to be the optimal temperature for corn seed germination tests, and in darkness, apart from times when germination was recorded. Seeds were observed several times daily and were considered to be germinated when the radical was over 0.2 cm long. Throughout the experiment, the germination percentage and time, root and shoot length, seedling fresh and dry weight, seed vigor index were recorded.
Mean Germination Time
Mean germination time (MGT) was calculated according to Ghassemi-Golezani et al. (2016) as:
Where n is the number of seeds germinated at the time of measurement, D is the number of days since the beginning of the experiment. Seedlings with short, thick, and spiral formed hypocotyls and stunted primary root was considered as abnormally germinated (ISTA, 2010). At the end of the germination experiments (2 weeks), radicles and shoots were cut from the cotyledons and then dried in an oven at 75 ± 2°C for 24 h.
Seed Vigor Index
The seedlings dry weight (SDW) obtained from the radicle and shoot was used to calculate seed vigor index (SVI) as follows:
Where MGT is mean germination time and SDW is the seedling dry weight.
Data Analysis
Data were analyzed using SAS 9.4, and differences between control and treatments were considered statistically significant at P < 0.05 using an LSD test. Excel software was used to draw figures.
Result
Germination
Thes CFS of Bacillus strains at both dilution ratios (1:50 and 1:250) in the absence of salinity stress increased seed germination and germination rate (Figure 1).
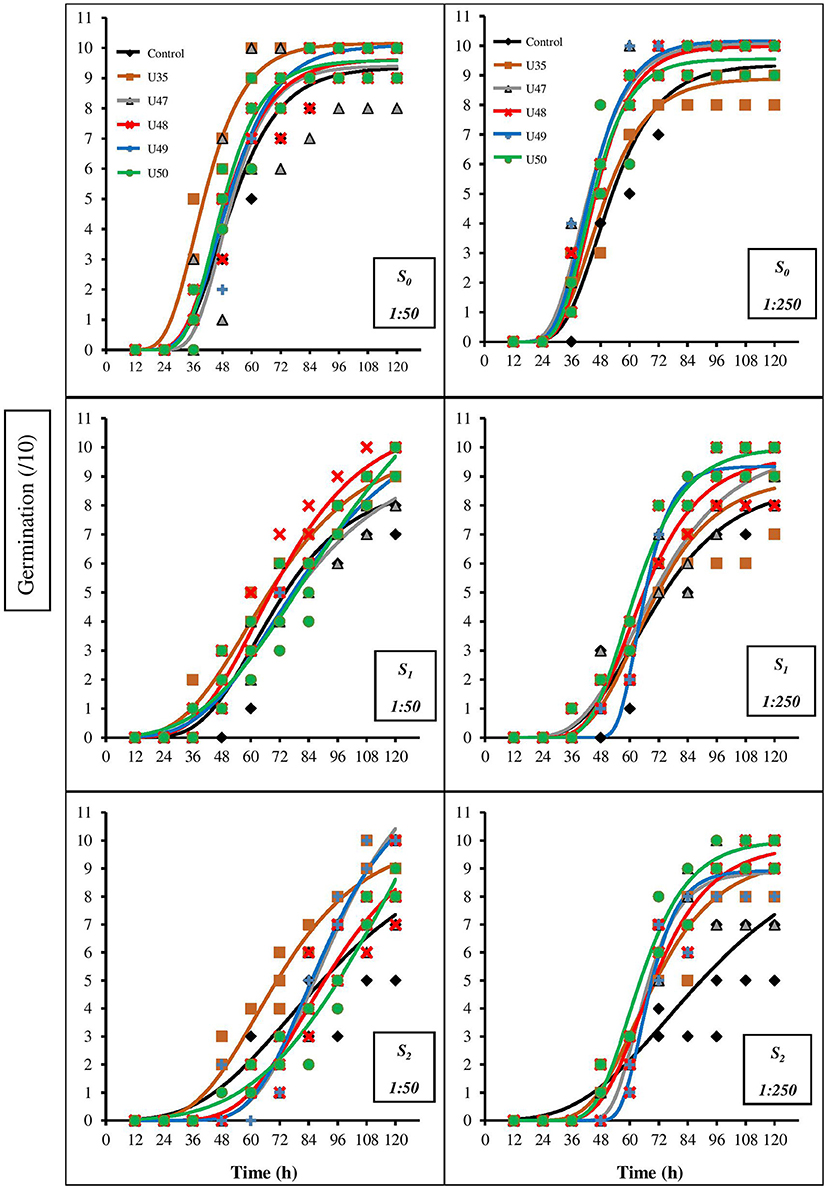
Figure 1. Changes in seed germination percentage for corn in response to salinity (0, 100, and 150 mM NaCl) and 1:50 and 1:250 dilutions of Bacillus strain CFSs (U35, 47, 48, 49, and 50). Values represent the means of five replicates ± SD. S0, S1, and S2 indicate 0, 100 and 150 mM NaCl, respectively. 1:50 dilution = 1 mL CFS of each Bacillus strain in 50 mL distilled water and 1:250 dilution = 1 mL cell-free supernatant of each Bacillus strain in 250 mL distilled water.
The highest germination and germination rate in the 1:50 and 1:250 dilutions were obtained as a result of the application of U35 and U49, respectively. Also, the best performance for CFS of strains 47, 48, 49 and 50 was at the 1:250 dilution, while the best efficiency of U35 was at the 1:50 dilution. Although moderate salinity (100 mM NaCl) has reduced germination and germination rate, the application of CFS from a range of Bacillus strains at both dilutions reduced the damaging effects of salinity (Figure 1). Comparison of the two graphs shows that the application of CFS at the 1:250 dilution had greater effects and strongly improved the germination process of treated seeds compared to control seeds; the greatest effect resulted from the application of U50 CFS.
The effects of severe salinity (150 mM NaCl) were significant and had adverse effects on germination, especially in CFS-free seeds. Although the application of Bacillus strain CFSs at high concentration (1:50 dilution) mitigated some of the negative effects of salinity the benefits were generally < the 1:250 dilution. At this concentration, the highest germination resulted from treatment with CFS from strains U47 and U49. CFS of strains in the more dilute concentration (1: 250 dilution) had stronger effects so that under severe salinity conditions, the germination of seeds treated with CFS was high in comparison to control seeds, especially seeds treated by CFS from strain U50.
Mean Germination Time
Our results indicated that salinity stress, especially severe salinity (150 mM NaCl) led to dramatically lengthened mean germination time for corn (Table 1). However, applying Bacillus strain CFSs to corn seed at all salinity levels reduced the mean germination time. At 1:50 and 1:250 CFS dilutions, the minimum germination time was observed in seed treated by CFS from U35 (2.4 days) and U47 (2.41 days).
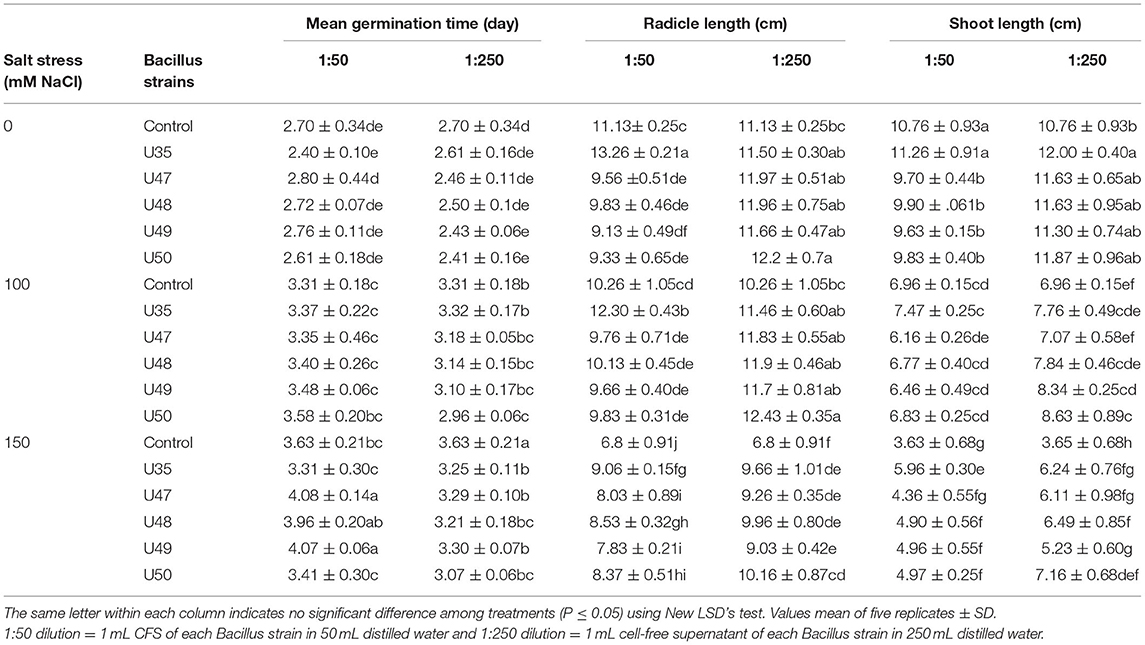
Table 1. Effect of Bacillus strain CFS dilution (1:50 and 1:250) and salinity on seed quality variables of corn.
Radicle Length
Salinity and Bacillus strain CFSs had significant effects on radicle length (Table 1). As expected, salinity stress significantly reduced radicle length, at moderate and severe salinity by 7.81 and 38.90%, respectively, compared with non-saline conditions. However, Bacillus strain CFSs caused significant increases in radicle length of corn, the highest of which was observed at the dilutions of 1:50 and 1: 250 for seeds treated with U35 (13.26 cm) and U50 (12.2 cm), respectively.
Shoot Length
Shoot length of corn seedlings was greatly affected by the salinity and Bacillus strain CFSs (Table 1). Although radicle length decreased from 10.76 to 3.62 cm under 150 mM NaCl compared to none-saline conditions, application Bacillus strain CFSs mitigated effect of salinity on radicle length while destructive effects of salinity on shoot length was specifically reduced by CFSs from U35 and U50 strains.
Radicle and Shoot Fresh Weight
Even though radicle fresh weight decreased under moderate (100 mM NaCl) and severe salinity (150 mM NaCl) by 34.68 and 52.99 %, respectively, seed treated with Bacillus strain CFSs at all salinity levels showed less adverse effects of salt stress. Also, under stress and non-stress conditions, the highest radicle fresh weights were associated with the application of CFSs from strains U35 (6.14 mg) and U50 (6.26 mg) (Figure 2).
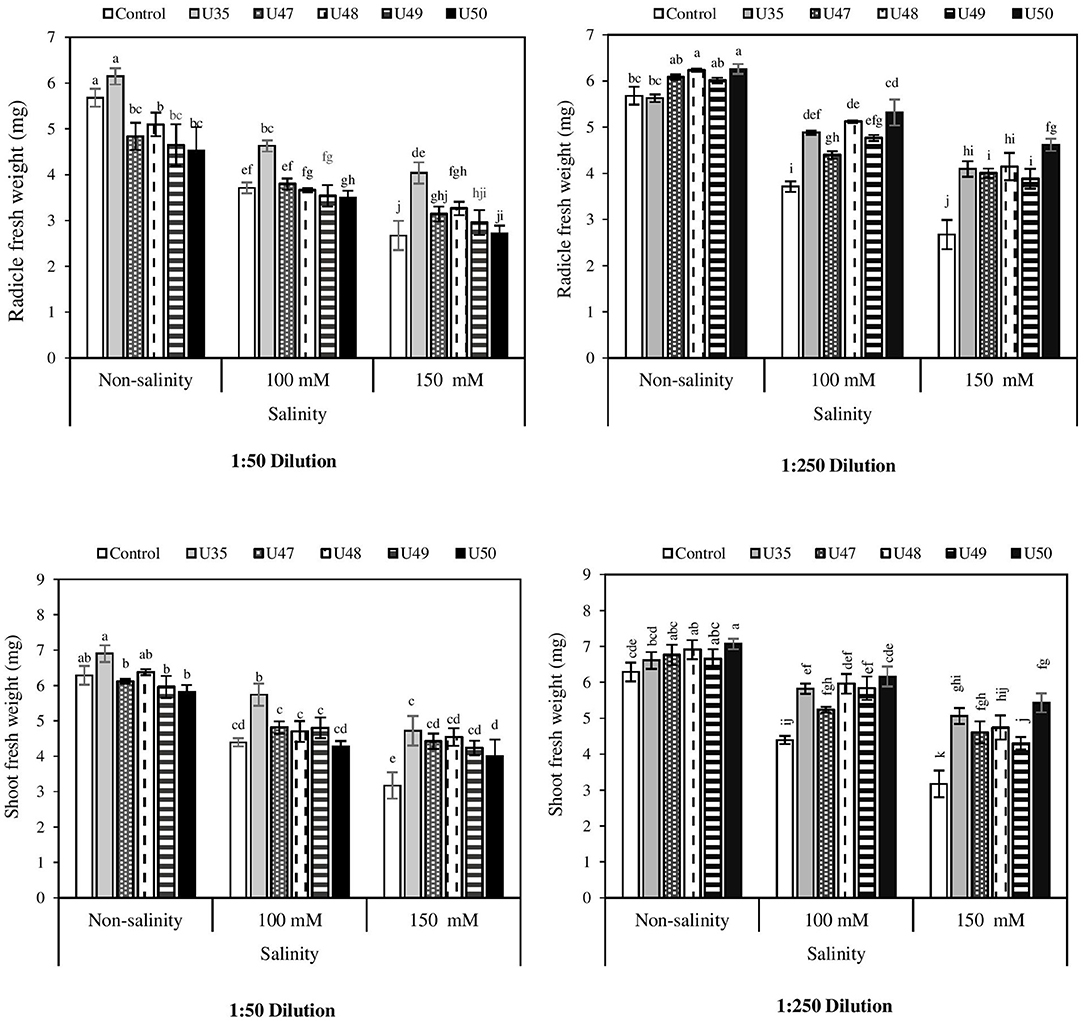
Figure 2. Effect of 1:50 and 1:250 dilutions of Bacillus strain CFSs (U35, 47, 48, 49, and 50) and salinity on radicle and shoot fresh weight of corn seedlings. Values represent the mean of five replicates ± SD. 1:50 = 1 mL Bacillus strain CFSs and 50 mL distilled water and 1:250 = 1 mL Bacillus strain CFSs and 250 mL distilled water.
Our findings demonstrated that salinity and Bacillus strain CFSs had significant and opposite effects on shoot fresh weight. Germination under saline conditions resulted in the production of shoots with lower weights, so that the mean fresh weight of the shoots in non-stress conditions was 6.28 mg, moderate salinity 4.39 mg and severe salinity 3.17 mg. However, application of Bacillus strain CFSs improved shoot fresh weight at 1:50 and 1:250 dilutions. Seeds treated with CFS from U35 and U50 strains increased the shoot fresh weight by 9.87 and 12.58%, respectively (Figure 2).
Radicle and Shoot Dry Weight
The effect of salinity and Bacillus strain CFSs on radicle dry weight is shown in Figure 3. As expected, salinity caused a decrease in radicle dry weight of corn seedlings, and this reduction, in moderate and severe salinity was 34.68 and 52.63% respectively. While application of Bacillus strain CFSs, at both dilutions (1:50 and 1:250), to corn seeds mitigated the negative effects of salinity.
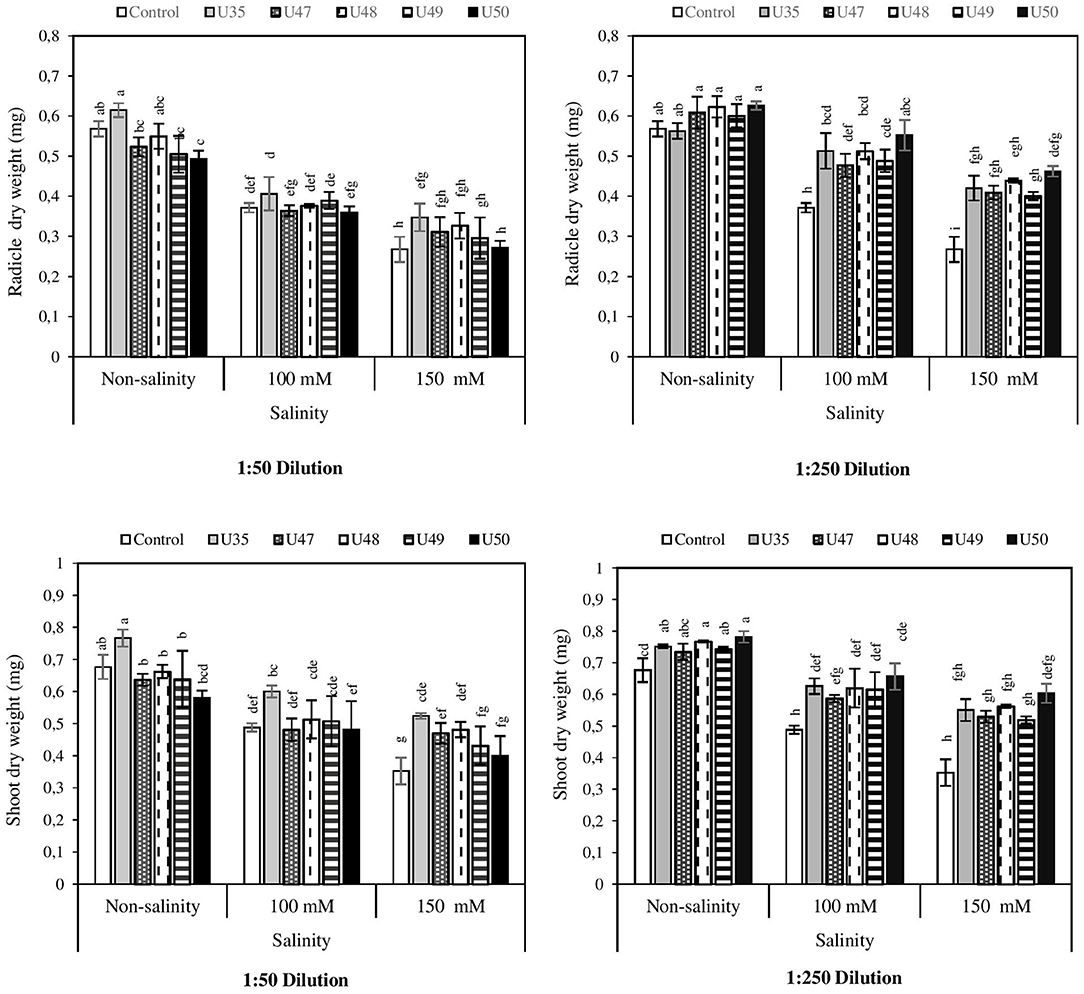
Figure 3. Effect of 1:50 and 1:250 dilutions of Bacillus strain CFSs (U35, 47, 48, 49, and 50) and salinity on radicle and shoot dry weight in corn. Values represent the mean of five replicates ± SD. 1:50 =1 mL Bacillus strain CFSs and 50 mL distilled water and 1:250 = 1 mL Bacillus strain CFSs and 250 mL distilled water.
Figure 3 indicates that CFS improved the shoot dry weight at all levels of salinity stress and also all Bacillus strains had positive effects on shoot dry weight. Strain U35 had the largest effect at the 1:50 dilution, while the strongest impacts of strains U47, U48, U49, and U50 on shoot dry weight of corn were observed at the 1:250 dilution.
Seed Vigor Index
Figure 4 indicates that increasing salinity had a negative influence on seed vigor index. Although the seed vigor index was 0.46 under non-saline conditions, in moderate and severe salinity decreased to 0.26 and 0.17, respectively. Seed treated Bacillus strain CFSs increased the seed index at both dilutions (1:50 and 1:250), and the highest efficicacy was observed as a result of treatment with CFSs from strains U50, U35, and U48, in order of effectiveness.
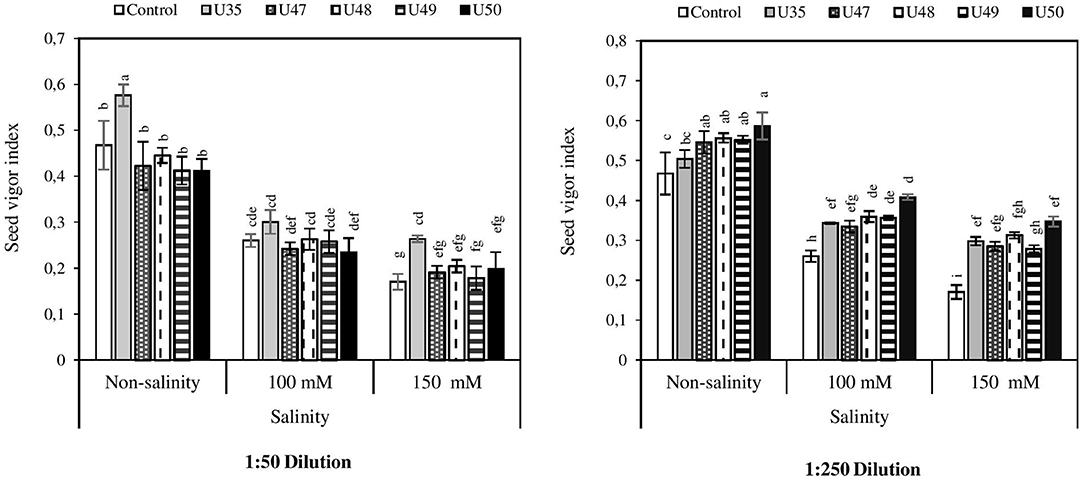
Figure 4. Effect of 1:50 and 1:250 dilutions of Bacillus strain CFSs (U35, 47, 48, 49, and 50) and salinity on corn seed vigor index. Values mean of five replicates ± SD. 1:50 = 1 mL Bacillus strain CFSs and 50 mL distilled water and 1:250 = 1 mL Bacillus strain CFSs and 250 mL distilled water.
Correlation
Correlation coefficients of measured variables and seed vigor index (Table 2) showed germination variables and seed vigor index correlated positively and significantly. However, mean germination time was negatively and significantly correlated with corn seed vigor index. At the 1:50 dilution on ther other, the highest and lowest positive correlations with seed vigor index were for radicle dry weight and germination, respectively. Furthermore, at 1:250 dilution radicle fresh weight and seed germination, respectively, were most and least correlated with seed vigor index.
Discussion
The overall objective of this study was to identify biologically efficient methods to reduce the adverse effects of salinity stress. The effects of salinity at the early stages of germination affect subsequent growth, and since seed germination is the first step in plant growth and final performance, selection of the appropriate biological methods to reduce the damaging effects of salinity stress is important for crop seed germination (Rajabi Dehnavi et al., 2020). Our findings confirm that salinity is one of the limiting factors for germination, which significantly reduces the germination percentage and rate of corn seeds. Similar results have been published previously for other crops, such as sorghum (Rajabi Dehnavi et al., 2020), rice (Liu et al., 2018), soybean (Shu et al., 2017), and canola (Li et al., 2017), illustrating that salinity stress reduces germination percentage for a wide range of crop plants. Although it has been shown that the negative relationships between salinity and germination percentage and rate vary with salt levels. Due to the sensitivity of plants to salt, moderate and severe salinity reduced seed germination through dormancy and inhibition of seedling development (Rajabi Dehnavi et al., 2020). Salinity stress negatively alters germination percentage and rate by affecting the amount and composition of phytohormones, osmotic proteins, changes in the activity of antioxidant enzymes and reactive oxygen species (ROS) (Farhangi-Abriz and Torabian, 2017; Liu et al., 2018). However, treatment of corn seed with microbial CFS from Bacillus strains reduced, to an extent, the negative effects of salinity stress and improved germination percentage and rate at all levels of salinity stress. It was clear that strains U35 and U50 at 1:50 and 1:250 dilutions had the largest positive effects at all salinity levels.
Enzymes involved in seed germination are referred to as Hydrolytic enzymes (Delshadi et al., 2017) and PGPR has been shown to increase their activity, thereby improving germination (Nuncio-Orta et al., 2015). Similar results have been reported by Li et al. (2017). One of the important factors in seed germination is the phytohormone abscisic acid (ABA), an inhibitory hormone for germination and growth under biotic and abiotic stresses, its amount increases under stress conditions; in addition, the presence of this hormone plays a vital role in seed dormancy (Atia et al., 2009; Shu et al., 2016, 2017; Née et al., 2017). It also controls the activity of growth phytohormones such as gibberellin (Gallardo et al., 2002; Ayele et al., 2012; Liu et al., 2018), thus reducing the rate of germination and increasing the duration of germination via those pathways. As mentioned previously, the application of Bacillus strains can greatly reduce the negative effects of salinity stress and will reduce germination time by increasing the germination rate (Delshadi et al., 2017). Thus, CFSs of Bacillus strain could influence the amount of ABA to be reduced, and germination occurs faster.
The results of the present study showed that salinity, in addition to limiting the germination percentage, rate and time, caused a significant reduction in radicle and shoot length, and the shortest radicle and shoot length occurred at the greatest salinity level. Because the first organ that is exposed to salinity is the radicle and the embryonic shoot reductions in their length is a common phenomenon when salinity stress is present and occurs in all plants (Rajabi Dehnavi et al., 2020). In addition to the phytohormonal issues mentioned previously, salinity can make the ionic balance in the cell volatile and this ionic disorder will have negative consequences on the structure of the cell wall (Hanin et al., 2016; Zhu, 2016). Because salinity through disrupting the K+/Na+ ratio (Yaghoubian et al., 2021), will reduce the strength of the cell wall, and this ion toxicity will cause the production of ROS (Farhangi-Abriz and Torabian, 2017). Aggregation of ROS can disrupt metabolic activity by damaging the cell wall (Demidchik et al., 2014) and storage proteins in the seed, which are the main sources of nutrition for radicle and shoot, greatly reducing the longitudinal growth of radicle and shoot. There is a positive relationship between the length of plant organs and their fresh and dry weights. Salinity reduced the radicle and shoot length of corn (Table 1) and this longitudinal reduction led to reduced radicle and shoot fresh and dry weight, especially under severe salinity, where the lowest value was recorded.
Salinity, by reducing gibberellin and increasing the production of ABA and ethylene, and also increasing accumulation of ROS, reduced radicle and shoot access to nutrients stored in the seed and thus reduced the fresh and dry weight of these organs. These results are consistent those of other researchers (Li et al., 2016; Shu et al., 2017). However, one way to improve germination indices under salinity conditions is the deployment of PGPR (Habib et al., 2016; Hussein and Joo, 2018; Ha-Tran et al., 2021); these effects were also observed in our results as a result of application of CFS. At moderate and severe salinity, the highest amount of organ fresh and dry weight was obtained as a result of application of Bacillus strain CFSs, especially strains U50, U35, and U48. In general, the greatest effects were at the 1:250 dilution. Previous reports have shown that the PGPR largely balances the effects of stress and improves the growth of tomatoes (Akram et al., 2019; Vaishnav et al., 2020) and soybean (Yaghoubian et al., 2021). PGPR enhances plant growth by changing phytohormone ratios and increasing the ratio of growth phytohormones to growth inhibitors. has been reported that, accumulation of ethylene, a growth inhibitory phytohormone, is limited by bacteria. Because the primary precursor of ethylene (amino cyclopropane-1-carboxylate deaminase) is used by bacteria as a source for nitrogen fixation (Backer et al., 2018). As a result of reduced ethylene, the negative effects of stress are reduced (Nascimento et al., 2018). The application of PGPR also prevents the accumulation of ROS under stress by improving the activity of antioxidant enzymes, so that stored proteins of seed are less damaged (Zhang et al., 2017). As a result, growing of radicle and shoot will improve. Similar results have been reported for rice (Rêgo et al., 2014), bean (Korir et al., 2017), and chickpea (Fierro-Coronado et al., 2014).
Seed vigor index, the most important indicator for evaluating seed production capacity, had a similar pattern of a relationship with other measured variables and was negatively affected by salinity stress. The first determining factor for germination, after seed survival, is the presence of water and its adequate uptake by the seed. When the plant is exposed to salinity stress, the osmotic potential caused by salt prevents water uptake (Misra and Gupta, 2005) and conditions similar to water deficiency occur, thus reducing seed vigor (Delshadi et al., 2017). Salinity also reduces seed vigor index by reducing the amount of radicle and shoot dry weight (Figure 3) as well as increasing the germination time (Table 1). Rajabi Dehnavi et al. (2020) reported similar results for sorghum. Findings of this study showed that application of Bacillus strain CFSs at both 1:50 and 1:250 dilutions mitigated the adverse effects of salinity stress. Strains 35, 50 and 48 caused clear increases over control seeds and in general, all strains except 35 were more effective at the 1:250 dilution. Similar results have been reported for positive effects of PGPR on tomato (Konappa et al., 2020) and soybean (De Gregorio et al., 2017).
Conclusions
Studies involving the effects of CFS on plant growth promotion remain scarce and even the few reports have remained mainly conducted under a controlled environment and under optimum conditions as reviewed by Pellegrini et al. (2020). This study aimed at understanding the potential positive effects of CFS on adverse effects of salinity on seed germination percentage, rate, radicle length, shoot development, and finally seed vigor index. Findings obtained in this study have provided new insights on the effects of CFSs from Bacillus on the seed vigor index of corn under salinity. Clearly, the study has indicated that at 1:50 concentration of CFSs can have both positive and negative effects on germination. Furthermore, 1: 250 dilutions especially U50 improved most growth indices such as germination percentage and rate, as well as seed vigor index and greatly reduced the negative effects of salinity stress and improved seed vigor index of corn. It can be argued that CFSs of Bacillus strains have reduced the negative effects of salinity on the involved factors in germination such as Hydrolytic enzymes, phytohormones, ROS and ionic balance. It would also be of interest to study the chemical composition of the studied CFSs which caused positive effects.
Data Availability Statement
The raw data supporting the conclusions of this article will be made available by the authors, without undue reservation.
Author Contributions
IY developed the original idea, conducted research, analyzed data, and wrote the manuscript. LM abstracted and reviewed the manuscript. DS provided critical revision of the manuscript and overall intellectual context. All authors contributed to the article and approved the submitted version.
Funding
This work was funded through a Collaborative Research Development Grant from the Natural Science and Engineering Research Council of Canada, number G247037.
Conflict of Interest
The authors declare that the research was conducted in the absence of any commercial or financial relationships that could be construed as a potential conflict of interest.
Publisher's Note
All claims expressed in this article are solely those of the authors and do not necessarily represent those of their affiliated organizations, or those of the publisher, the editors and the reviewers. Any product that may be evaluated in this article, or claim that may be made by its manufacturer, is not guaranteed or endorsed by the publisher.
References
Akram, W., Aslam, H., Ahmad, S. R., Anjum, T., Yasin, N. A., Khan, W. U., et al. (2019). Bacillus megaterium strain A12 ameliorates salinity stress in tomato plants through multiple mechanisms. J. Plant Interact. 14, 506–518. doi: 10.1080/17429145.2019.1662497
Atia, A., Debez, A., Barhoumi, Z., Smaoui, A., and Abdelly, C. (2009). ABA, GA3, and nitrate may control seed germination of Crithmum maritimum (Apiaceae) under saline conditions. C. R. Biol. 332, 704–710. doi: 10.1016/j.crvi.2009.03.009
Ayele, B. T., Ozga, J. A., Wickramarathna, A. D., and Reinecke, D. M. (2012). Gibberellin metabolism and transport during germination and young seedling growth of pea (Pisum sativum L.). J. Plant Growth Regul. 31, 235–252. doi: 10.1007/s00344-011-9234-8
Backer, R., Rokem, J. S., Ilangumaran, G., Lamont, J., Praslickova, D., Ricci, E., et al. (2018). Plant growth-promoting rhizobacteria: context, mechanisms of action, and roadmap to commercialization of biostimulants for sustainable agriculture. Front Plant Sci. 9, 1473. doi: 10.3389/fpls.2018.01473
Basu, A., Prasad, P., Das, S. N., Kalam, S., Sayyed, R. Z., Reddy, M. S., et al. (2021). Plant growth promoting rhizobacteria (PGPR) as green bioinoculants: recent developments, constraints, and prospects. Sustainability 13, 1140. doi: 10.3390/su13031140
De Gregorio, P. R., Michavila, G., Ricciardi Muller, L., de Souza Borges, C., Pomares, M. F., Saccol de Sá, E. L., et al. (2017). Beneficial rhizobacteria immobilized in nanofibers for potential application as soybean seed bioinoculants. PLoS ONE. 12, e0176930. doi: 10.1371/journal.pone.0176930
Delshadi, S., Ebrahimi, M., and Shirmohammadi, E. (2017). Influence of plant-growth-promoting bacteria on germination, growth and nutrients' uptake of Onobrychis sativa L. under drought stress. J. Plant Interact. 12, 200–208. doi: 10.1080/17429145.2017.1316527
Demidchik, V., Straltsova, D., Medvedev, S. S., Pozhvanov, G. A., Sokolik, A., and Yurin, V. (2014). Stress-induced electrolyte leakage: the role of K+−permeable channels and involvement in programmed cell death and metabolic adjustment. J. Exp. Bot. 65, 1259–1270. doi: 10.1093/jxb/eru004
Farhangi-Abriz, S., and Torabian, S. (2017). Antioxidant enzyme and osmotic adjustment changes in bean seedlings as affected by biochar under salt stress. Ecotoxicol. Environ. Saf. 137, 64–70. doi: 10.1016/j.ecoenv.2016.11.029
Fierro-Coronado, R. A., Quiroz-Figueroa, F. R., García-Pérez, L. M., Ramírez-Chávez, E., Molina-Torres, J., and Maldonado-Mendoza, I. E. (2014). IAA-producing rhizobacteria from chickpea (Cicer arietinum L.) induce changes in root architecture and increase root biomass. Can. J. Microbiol. 60, 639–648. doi: 10.1139/cjm-2014-0399
Gallardo, K., Job, C., Groot, S. P., Puype, M., Demol, H., Vandekerckhove, J., et al. (2002). Proteomics of Arabidopsis seed germination. A comparative study of wild-type and gibberellin-deficient seeds. Plant Physiol. 129, 823–837. doi: 10.1104/pp.002816
Ghassemi-Golezani, K., Yaghoubian, I., and Raei, Y. (2016). The impact of hydro-priming duration on seed invigoration and field emergence of milk thistle. J. Biodivers. Environ. Sci 9, 229–234.
Habib, S. H., Kausar, H., and Saud, H. M. (2016). Plant growth-promoting rhizobacteria enhance salinity stress tolerance in okra through ROS-scavenging enzymes. Biomed Res. Int. 2016, 6284547. doi: 10.1155/2016/6284547
Hanin, M., Ebel, C., Ngom, M., Laplaze, L., and Masmoudi, K. (2016). New insights on plant salt tolerance mechanisms and their potential use for breeding. Front. Plant Sci. 7, 1787. doi: 10.3389/fpls.2016.01787
Ha-Tran, D. M., Nguyen, T. T. M., Hung, S. H., Huang, E., and Huang, C. C. (2021). Roles of plant growth-promoting rhizobacteria (PGPR) in stimulating salinity stress defense in plants: a review. Int. J. Mol. Sci. 22, 3154. doi: 10.3390/ijms22063154
Hussein, K. A., and Joo, J. H. (2018). Plant growth-promoting rhizobacteria improved salinity tolerance of Lactuca sativa and Raphanus sativus. J. Microbiol. Biotechnol. 28, 938–945. doi: 10.4014/jmb.1712.12027
ISTA. (2010). International Rules for Seed Testing. Bassersdorf: International Seed Testing Association.
Khan, N., Bano, A., Ali, S., and Babar, M. A. (2020). Crosstalk amongst phytohormones from planta and PGPR under biotic and abiotic stresses. Plant Growth Regul. 90, 189–203. doi: 10.1007/s10725-020-00571-x
Konappa, N., Krishnamurthy, S., Arakere, U. C., Chowdappa, S., and Ramachandrappa, N. S. (2020). Efficacy of indigenous plant growth-promoting rhizobacteria and Trichoderma strains in eliciting resistance against bacterial wilt in a tomato. Egypt J. Biol. Pest Control. 30, 1–13. doi: 10.1186/s41938-020-00303-3
Korir, H., Mungai, N. W., Thuita, M., Hamba, Y., and Masso, C. (2017). Co-inoculation effect of rhizobia and plant growth promoting rhizobacteria on common bean growth in a low phosphorus soil. Front. Plant Sci. 8, 141. doi: 10.3389/fpls.2017.00141
Kumar, D., and Narayan, A. (2013). Mini review paper nutritional, medicinal and economical importance of corn: a mini review. Res. J. Pharm. Sci. 2, 7–8.
Kumari, B., Mallick, M. A., Solanki, M. K., Solanki, A. C., Hora, A., and Guo, W. (2019). “Plant growth promoting rhizobacteria (PGPR): modern prospects for sustainable agriculture,” in Plant Health Under Biotic Stress: Volume 2: Microbial Interactions (Springer), 109–127. doi: 10.1007/978-981-13-6040-4_6
Li, H., Lei, P., Pang, X., Li, S., Xu, H., Xu, Z., et al. (2017). Enhanced tolerance to salt stress in canola (Brassica napus L.) seedlings inoculated with the halotolerant Enterobacter cloacae HSNJ4. Agric. Ecosyst. Environ. Appl. Soil Ecol. 119, 26–34. doi: 10.1016/j.apsoil.2017.05.033
Li, W., Yamaguchi, S., Khan, M. A., An, P., Liu, X., and Tran, L. S. P. (2016). Roles of gibberellins and abscisic acid in regulating germination of Suaeda salsa dimorphic seeds under salt stress. Front. Plant Sci. 6, 1235. doi: 10.3389/fpls.2015.01235
Liu, L., Xia, W., Li, H., Zeng, H., Wei, B., Han, S., et al. (2018). Salinity inhibits rice seed germination by reducing α-amylase activity via decreased bioactive gibberellin content. Front. Plant Sci. 9, 275. doi: 10.3389/fpls.2018.00275
Lyu, D., Msimbira, L. A., Nazari, M., Antar, M., Pagé, A., Shah, A., et al. (2021a). The coevolution of plants and microbes underpins sustainable agriculture. Microorganisms. 9, 1036. doi: 10.3390/microorganisms9051036
Lyu, D., Zajonc, J., Pagé, A., Tanney, C. A. S., Shah, A., Monjezi, N., et al. (2021b). Plant holobiont theory: the phytomicrobiome plays a central role in evolution and success. Microorganisms 9, 675. doi: 10.3390/microorganisms9040675
Misra, N., and Gupta, A. K. (2005). Effect of salt stress on proline metabolism in two high yielding genotypes of green gram. Plant Sci. 169, 331–339. doi: 10.1016/j.plantsci.2005.02.013
Nascimento, F. X., Rossi, M. J., and Glick, B. R. (2018). Ethylene and 1-aminocyclopropane-1-carboxylate (ACC) in plant–bacterial interactions. Front. Plant Sci. 9, 114. doi: 10.3389/fpls.2018.00114
Née, G., Xiang, Y., and Soppe, W. J. (2017). The release of dormancy, a wake-up call for seeds to germinate. Curr. Opin. Plant Biol. 35, 8–14. doi: 10.1016/j.pbi.2016.09.002
Nuncio-Orta, G., Mendoza-Villarreal, R., Robledo-Torres, V., VazquezBadillo, M., and Almaraz Suarez, J. J. (2015). Influence of rhizobacteria on seed germination and vigor of seeds chili jalapeno (Capsicum annuum L. ‘var. Grande'). ITEA Inform. Tecn. Econ Agraria. 111, 18–33. doi: 10.12706/itea.2015.002
Ortíz-Castro, R., Contreras-Cornejo, H. A., Macías-Rodríguez, L., and López-Bucio, J. (2009). The role of microbial signals in plant growth and development. Plant Signal. Behav. 4, 701–712. doi: 10.4161/psb.4.8.9047
Payumo, J. G., Assem, S., Bhooshan, N., Galhena, H., Mbabazi, R., and Maredia, K. (2018). Managing agricultural research for prosperity and food security in 2050: comparison of performance, innovation models and prospects. Open Agr. J. 12, 20. doi: 10.2174/1874331501812010020
Pellegrini, M., Pagnani, G., Bernardi, M., Mattedi, A., Spera, D. M., and Gallo, M. D. (2020). Cell-Free supernatants of plant growth-promoting bacteria: a review of their use as biostimulant and microbial biocontrol agents in sustainable agriculture. Sustainability 12, 9917. doi: 10.3390/su12239917
Prasad, M., Srinivasan, R., Chaudhary, M., Choudhary, M., and Jat, L. K. (2019). “Chapter seven—plant growth promoting rhizobacteria (PGPR) for sustainable agriculture: perspectives and challenges,” in PGPR Amelioration in Sustainable Agriculture, eds A. K. Singh, A. Kumar, and P. K. Singh (Jhansi: Woodhead Publishing), 129–157. doi: 10.1016/B978-0-12-815879-1.00007-0
Rajabi Dehnavi, A., Zahedi, M., Ludwiczak, A., Cardenas Perez, S., and Piernik, A. (2020). Effect of salinity on seed germination and seedling development of sorghum [Sorghum bicolor (L.) Moench] genotypes. Agronomy 10, 859. doi: 10.3390/agronomy10060859
Rêgo, M. C. F., Ilkiu-Borges, F., de Filippi, M. C. C., Gonçalves, L. A., and da Silva, G. B. (2014). Morphoanatomical and biochemical changes in the roots of rice plants induced by plant growth-promoting microorganisms. J. Bot. 2014:818797. doi: 10.1155/2014/818797
Shah, A., Nazari, M., Antar, M., Msimbira, L. A., Naamala, J., Lyu, D., et al. (2021). PGPR in agriculture: a sustainable approach to increasing climate change resilience. Front. Sustain. Food Syst. 5, 211. doi: 10.3389/fsufs.2021.667546
Shahid, M. A., Sarkhosh, A., Khan, N., Balal, R. M., Ali, S., Rossi, L., et al. (2020). Insights into the physiological and biochemical impacts of salt stress on plant growth and development. Agronomy 10, 938. doi: 10.3390/agronomy10070938
Shu, K., Chen, Q., Wu, Y., Liu, R., Zhang, H., Wang, P., et al. (2016). ABI 4 mediates antagonistic effects of abscisic acid and gibberellins at transcript and protein levels. Plant J. 85, 348–361. doi: 10.1111/tpj.13109
Shu, K., Qi, Y., Chen, F., Meng, Y., Luo, X., Shuai, H., et al. (2017). Salt stress represses soybean seed germination by negatively regulating GA biosynthesis while positively mediating ABA biosynthesis. Front. Plant Sci. 8, 1372. doi: 10.3389/fpls.2017.01372
Tyczewska, A., Wozniak, E., Gracz, J., Kuczyński, J., and Twardowski, T. (2018). Towards food security: current state and future prospects of agrobiotechnology. Trends Biotechnol. 36, 1219–1229. doi: 10.1016/j.tibtech.2018.07.008
Vaishnav, A., Singh, J., Singh, P., Rajput, R. S., Singh, H. B., and Sarma, B. K. (2020). Sphingobacterium sp. BHU-AV3 induces salt tolerance in tomato by enhancing antioxidant activities and energy metabolism. Front. Microbiol. 11, 443. doi: 10.3389/fmicb.2020.00443
Vejan, P., Khadiran, T., Abdullah, R., Ismail, S., and Dadrasnia, A. (2019). Encapsulation of plant growth promoting rhizobacteria—prospects and potential in agricultural sector: a review. J. Plant Nutr. 42, 2600–2623. doi: 10.1080/01904167.2019.1659330
Venturi, V., and Keel, C. (2016). Signaling in the rhizosphere. Trends Plant Sci. 21, 187–198. doi: 10.1016/j.tplants.2016.01.005
Yaghoubian, I., Ghassemi, S., Nazari, M., Raei, Y., and Smith, D. L. (2021). Response of physiological traits, antioxidant enzymes and nutrient uptake of soybean to azotobacter chroococcum and zinc sulfate under salinity. S. Afr. J. Bot. 143, 42–51. doi: 10.1016/j.sajb.2021.07.037
Zhang, N., Zhang, H. J., Sun, Q. Q., Cao, Y. Y., Li, X., Zhao, B., et al. (2017). Proteomic analysis reveals a role of melatonin in promoting cucumber seed germination under high salinity by regulating energy production. Sci. Rep. 7, 503. doi: 10.1038/s41598-017-00566-1
Keywords: Bacillus strains, cell-free supernatant, corn, salinity, seed germination, seed vigor index
Citation: Yaghoubian I, Msimbira LA and Smith DL (2022) Cell-Free Supernatant of Bacillus Strains can Improve Seed Vigor Index of Corn (Zea mays L.) Under Salinity Stress. Front. Sustain. Food Syst. 6:857643. doi: 10.3389/fsufs.2022.857643
Received: 18 January 2022; Accepted: 21 February 2022;
Published: 21 March 2022.
Edited by:
Everlon Cid Rigobelo, São Paulo State University, BrazilReviewed by:
Swapan Kumar Paul, Bangladesh Agricultural University, BangladeshHadi Pirasteh-Anosheh, Agricultural Research, Education and Extension Organization, Iran
Copyright © 2022 Yaghoubian, Msimbira and Smith. This is an open-access article distributed under the terms of the Creative Commons Attribution License (CC BY). The use, distribution or reproduction in other forums is permitted, provided the original author(s) and the copyright owner(s) are credited and that the original publication in this journal is cited, in accordance with accepted academic practice. No use, distribution or reproduction is permitted which does not comply with these terms.
*Correspondence: Donald Lawrence Smith, ZG9uYWxkLnNtaXRoQG1jZ2lsbC5jYQ==