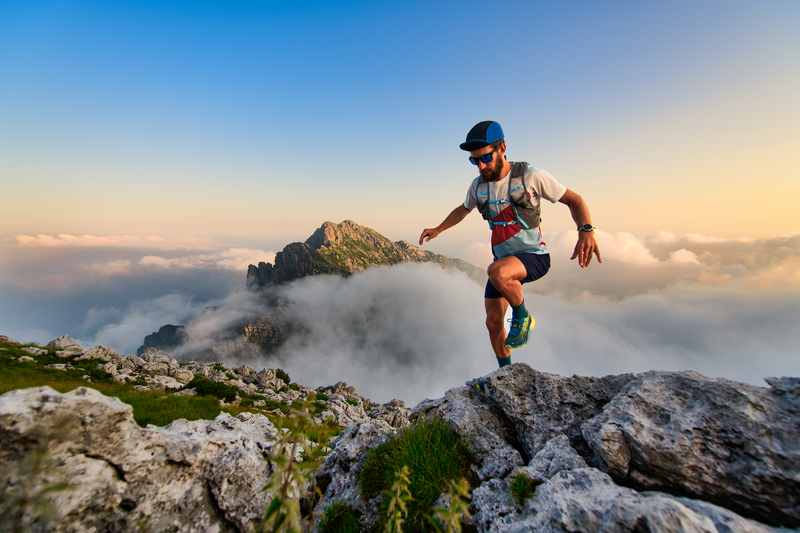
95% of researchers rate our articles as excellent or good
Learn more about the work of our research integrity team to safeguard the quality of each article we publish.
Find out more
ORIGINAL RESEARCH article
Front. Sustain. Food Syst. , 16 June 2022
Sec. Nutrition and Sustainable Diets
Volume 6 - 2022 | https://doi.org/10.3389/fsufs.2022.855788
This article is part of the Research Topic Sustaining Protein Nutrition through Plant-Based Foods: A Paradigm Shift View all 14 articles
Pulse varieties including Yellow Eye (YE) beans (Phaseolus vulgaris L.) are a rich source of protein (~26.5%) that can be utilized to create value-added protein concentrates. Pre-treatments including dehulling and germination have been shown to be effective at improving the nutritional and functional properties of extracted protein concentrates. However, the composition and functionality of these protein concentrates can vary depending on the pre-treatments and the method of extraction used (salt vs. alkaline). Furthermore, little is known about the impact of combining these different processing methods on the properties of YE bean protein concentrates. The objective of this study was to evaluate how germination and dehulling pre-treatments individually and when combined influence protein extraction efficiency, physiochemical properties (surface hydrophobicity and intrinsic fluorescence), and the functionality (solubility, oil and water holding capacities) of salt and alkaline extracted protein concentrates. Compared to the salt extracted concentrates, the alkaline protein concentrates exhibited higher protein recovery yields (16–23% vs. 43–56%) respectively. Conversely, the salt extracted protein concentrates exhibited superior functional properties as observed by improved water holding capacities and less variation in their solubilities at different pH values (4 to 10). When the pre-treatments were combined, the salt extracted concentrates exhibited improved extraction efficiencies and improved hydrophobicity and intrinsic fluorescence, whereas the opposite trend was observed in the alkaline protein concentrates. These observations were attributed to differences in the protein content and composition of the salt vs. alkaline protein concentrates. Overall, these findings suggest that dehulling and germination are potential processing methods that may be used to improve the physiochemical characteristics of salt extracted protein concentrates from yellow eye beans. Future research may investigate the potential application of these ingredients in different food formulations.
Over the past decade there has been increased efforts to reduce animal-based proteins and replace them with plant-based proteins to form healthier, more affordable, and more sustainable food products (Vainio et al., 2016). Underutilized pulse varieties, such as Nova Scotian Yellow Eye (YE) beans (Phaseolus vulgaris L.), have a high protein content (26.5%) and can serve as alternative protein ingredients in food production, however, their efficacy must be shown (English et al., 2019a).
Proteins in the form of concentrates (65–90% protein dry basis) or isolates (>90% protein dry basis) can be separated from different plant or animal sources and used as food ingredients (Ma et al., 2022). Traditionally, the preferred protein sources have been from soy, wheat, or dairy products (such as whey), however, the presence of allergens and certain dietary restrictions are making pulse proteins a more attractive alternative for food formulations (Soller et al., 2015; Nwachukwu and Aluko, 2021). Although there are different ways to define protein functionality, this terms most often refers to the ability of proteins to form and stabilize networks in food systems such as gels, foams, and gels, and includes physiochemical properties such as solubility, and water and fat binding abilities (Foegeding and Davis, 2011; Vogelsang-O'Dwyer et al., 2021). Indeed, pulse proteins have shown similar functional properties compared to soy proteins (Burger and Zhang, 2019). For example, common bean proteins have shown promising emulsifying and gelling properties (Shevkani et al., 2015; Rahmati et al., 2018), whereas pea proteins have exhibited high solubility and foaming properties. Knowledge of the functional properties of proteins is therefore important in selecting the most ideal ingredients for successful food formulations (Vogelsang-O'Dwyer et al., 2021). However, in terms of their functional properties not all proteins are equal, differences in the functional properties can be attributed to inherent variations between cultivars or differences in protein content, composition, and conformation (Rui et al., 2011). The type of protein extraction methodology used can also contribute to differences in functional properties because of: (1) changes in the protein fraction extraction affinity, and (2) conformational changes achieved during the extraction process (Karaca et al., 2011).
Currently, the techniques used to extract plant proteins may be grouped into dry and wet processes (Fernando, 2021; Yang et al., 2021). Dry processes achieve protein separation based on differences in density whereas in wet processes, proteins are extracted based on their solubilities in alkaline or salt solutions, followed by isoelectric or micellar precipitation, respectively (Stone et al., 2015; Tanger et al., 2020). The work in the present study has focused on wet processes since these methods are commonly used with pulses (Cui et al., 2020). Among the wet extraction methods, the alkaline extraction process is more commonly used because of its simple and cost-effective nature (Zhang et al., 2014; Momen et al., 2021). Although this approach primarily targets globulins and produces higher protein concentrate yields, the alkaline and acidic environments necessary with this approach can result in partial denaturation of the proteins which in turn reduces protein quality and functionality (Yang et al., 2021). Conversely, protein extraction techniques using salt solutions have been shown to produce a more complete protein profile (Karaca et al., 2011). However, compared to alkaline methods, salt extraction procedures can be time-consuming because an additional desalting step is required (Hadnadev et al., 2017). Determining how these two extraction techniques impact the functionality of YE bean protein concentrates is critical to the successful development and application of these ingredients.
Apart from the challenges related to protein extractions, the presence of undesirable components in pulses can impact the functionality and quality of pulse proteins. Antinutrients such as tannins have been linked with decreased protein bioavailability which in turn limits protein functionality (Samtiya et al., 2020). Various pre-treatments including germination and the subsequent enzymatic hydrolysis of indigestible proteins have helped to improve protein digestibility (Ali and Elozeiri, 2017). Dehulling (removal of the seed coat) and soaking have also been used to reduce anti-nutrients that impact protein adsorption (Wang, 2008). Many of the studies evaluating the effectiveness of pre-treatments have focused on flour samples with little emphasis on the protein isolates or concentrates. For example, Liu et al. (2018), demonstrated an increase in protein content and water absorption index in germinated mung bean flour over a 72-h period. In addition, Ghavidel and Prakash (2007) used a combination of pre-treatments (dehulling and germination) on lentils, chickpeas, green gram, and cowpeas and observed an increase in protein and protein digestibility. Indeed, our own observations with other food model systems containing yellow eye beans have shown that blending pre-treatments (germination and soaking) were more effective strategies for improving the functional properties and the aroma profiles of these pulse ingredients (English et al., 2019b). Although the use of combined pre-treatments is not a new approach the improvement in protein functionality suggests that there may be merit in further investigating this strategy.
Accordingly, the main objective of the present study was to evaluate the impact of dehulling and germination individually and a combination of these pre-treatments on Nova Scotia Yellow-eye bean protein concentrates. Protein extractions were conducted using alkaline and salt solutions, and the physiochemical (hydrophobicity and intrinsic fluorescence) and functional properties (solubility and oil and water binding capacity) of the different concentrates were evaluated. It was hypothesized that improved protein functionality and physiochemical properties may be observed in protein concentrates exposed to combined pre-treatments, since blending pre-treatments has been shown to have a greater impact on protein functionality (English et al., 2019b). Moreover, because salt extraction techniques have been demonstrated to reduce conformational changes in the extracted protein isolates (Grover and Ryall, 2005), it was further hypothesized that the salt extraction protein concentrates would have improved physiochemical properties compared to their alkaline extracted counterparts. Understanding the impact of these processes on the physiochemical and functional properties of yellow eye bean protein concentrates is important for the production and development of new pulse ingredients of high quality.
Yellow Eye beans (Phaseolus vulgaris L.) grown in Cambridge, Hants County, Nova Scotia, were purchased from a grocery store, Superstore in Antigonish, Nova Scotia and stored in a cool, dark cupboard until use. Bradford reagent, tris, and mini-PROTEAN electrophoresis gels were obtained from Bio-Rad (Mississauga, ON). Ethanol and sodium hypochlorite were purchased from VWR (Mississauga, ON). Sodium hydroxide, β-mercaptoethanol, and methanol were obtained from Fisher Scientific (Ottawa, ON). Sodium phosphate dibasic, sodium phosphate monobasic, and sodium dodecyl sulfate were purchased from Sigma-Aldrich (Oakville, ON). Hydrochloric acid and sodium hydroxide were purchased from Fisher Scientific (Ottawa Canada). All chemicals were reagent grade and did not require any additional purification.
A total of 5 bean variations were prepared for this study (Figure 1). Prior to flour preparation, beans were sorted and sanitized in a 0.07% (w/v) sodium hypochlorite solution for 30 min, then washed with distilled water until a pH of 7.0 was reached. Beans were soaked at a ratio of 1:4 of beans to distilled water at room temperature for 20 h. Once soaking was finished the remaining distilled water was decanted and excess water was removed from the beans. Whole/soaked (WS) beans were freeze dried and ground using a Blendtec 51-601-BHM Kitchen Mill. Untreated, raw beans (RB) were used as a control for this study and were milled using a Blendtec 51-601-BHM Kitchen Mill. The dehulled/soaked (DS) beans were first manually dehulled, then dried, ground, and stored as previously described.
Figure 1. Sample preparation of pre-treated (soaked, dehulled, germinated, and dehulled and germinated), Yellow Eye beans and the subsequent protein concentrates generated using salt and alkaline protein extraction solutions.
Variations described as whole/germinated (WG) and dehulled/germinated (DG) were germinated using a method adapted from Ma et al. (2018) and Xu et al. (2019). Beans were first sanitized and soaked following the method outlined in Section Preparation of Bean Flours for Protein Extraction. Twenty-two beans were placed into a sterile petri dish lined with Grade 1 Whatman® filter paper moistened with 1 mL of distilled water. The beans were covered with another piece of filter paper moistened with 500 μL of distilled water. Beans were germinated in a Memmert Humidity Chamber HCP (Büchenbach, Germany) at 20 °C and 95% relative humidity for a maximum of 72 h, with germinated beans being collected every 24 h. Beans were considered germinated when their radicles reached a length of over 2 mm. Beans that failed to germinate after the 72 h were discarded. Variation WG was then freeze dried and ground into flour, whereas variation DG was dehulled prior to freeze drying and grinding. All flours were stored in airtight containers at 4 °C until they were used.
Protein and ash analyses, and crude fat determination of all flours were determined at the Department of Agriculture and Food Operations Laboratory (Truro, Nova Scotia, Canada) using similar methods outlined in English et al. (2019a). Ash content was determined following the Association of Official Agricultural Chemists (AOAC) method 923.03 (AOAC, 2005). Protein content was determined using combustion analysis (Laboratory Services Analytical Laboratory, LSAL, Method 410) using a LECO CN828 macro combustion instrument (St. Joseph, KS, USA) using a protein to nitrogen conversion rate of 6.25 (Yang et al., 2021). Crude fat was calculated using a solvent extraction method. Carbohydrate content was determined by subtraction of the other chemical components from 100.
A total of five protein concentrates were generated using an alkaline extraction protocol (Figure 1). A modified alkaline extraction procedure was developed using a combination of reports from de Evangelho et al. (2017), Du et al. (2018), and Karaca et al. (2011). Briefly, bean flours were suspended in distilled water (1:10 w/v) and the pH of the solutions were adjusted to 9.5 with a 4N NaOH solution, after which the solutions were left to stir for 1 h. The slurries were centrifuged at 5,000 x g for 40 min and the supernatants collected. The remaining pellets were resuspended in distilled water (1:5 w/v) and centrifuged at 5,000 x g for 30 min. The supernatants were pooled together, and the pH was adjusted to 4.5 using 1M HCl to precipitate the protein. The samples were then centrifuged at 3,500 x g for 15 min and the pellets of precipitated protein was kept and adjusted to a pH of 7.0.
Five protein concentrates were prepared using a salt extraction protocol as shown in Figure 1. The salt extraction method described by Mundi and Aluko (2012) was followed with some modifications. Bean flours were suspended in 0.1 M Phosphate buffer (pH 8.0, 1:10 w/v) and stirred constantly for 2 h at 4 °C. The slurries were then centrifuged at 4,260 x g for 45 min, after which the supernatants were collected. Ammonium sulfate was added to the supernatants until 40% saturation had been achieved. The mixtures were stirred for an additional 2 h at 4 °C and centrifuged following the previously described conditions. The supernatants were collected once again, and ammonium sulfate added until 80% saturation was reached. The slurries were centrifuged one more time, after which the pellets were collected. The pH values of the extracted pellets were adjusted to 7.0 and diafiltrated with deionized water through a Vivaflow 200 polysulfone membrane with a molecular weight cut-off of 10 kDa at a volume concentration ratio (VCR) = 10.
Both protein extracts were ultrafiltered using a Vivaflow 200 polysulfone membrane with a molecular weight cut-off of 10 kDa. The ultrafiltration was repeated twice at VCR = 5. The collected protein solutions were freeze-dried and weighed to calculate the protein yield, and the protein recovery yields were calculated as a percentage of the isolate weight of the protein content found in the associated flour (Equations 1 and 2). Protein purity of each extracted protein concentrate (Equation 3) was determined by the Bradford protein assay (Bradford, 1976).
Sodium dodecyl sulfate polyacrylamide electrophoresis (SDS-PAGE) samples were prepared follow a method adapted from Aluko and McIntosh (2004) and English et al. (2019b). Concentrations of 10 mg/mL of each PI were prepared in Tris/HCl buffer (pH 8.0). Three point five microlitres of filtered protein extract was mixed with 10 μL of distilled water and 5 μL of protein sample buffer (pH 6.8, 1 M Tris-HCl, 5% SDS, 50% glycerol, 0.5% Bromphenol blue, and 10% β-mercaptoethanol) and heated in a boiling water bath for 10 min.
Fifteen microlitres of each sample were loaded onto 12% Mini-PROTEAN® TGXTM gels obtained from BioRad (Mississauga, ON). A pre-stained protein ladder (New England BioLabs, P77066; 10-250 kDa) was loaded in a 5 μL aliquot to estimate the molecular weights of proteins in the samples. Gels were loaded into a Mini-PROTEAN® Tetra Cell (Bio-Rad, Mississauga, ON) and the system was run for 1 hour at 170 V and 30 mA. Gels were stained for 30 min with a 0.1% Coomassie Brilliant Blue (R-250) staining solution and then de-stained overnight. A pre-stained protein marker (New England BioLabs, P77066, 10-250 kDa) was used to estimate the molecular weights of proteins. Images of the gels were captured using a BioRad Chemic DocTM MP imaging system.
The intrinsic fluorescence spectrums of the protein concentrates were measured following a modified method from He et al. (2020) and Yang et al. (2021). Concentrations of 0.2 mg/mL of protein solutions were made in 10 mM phosphate buffer (pH 7.2). The intrinsic fluorescence spectra of the protein solutions were measured spectrophotometrically using a QuantaMasterTM spectrofluorometer from Photon Technology International Inc. (London, ON). The excitation wavelength was set to 280 nm, and the emission spectra was recorded from 290–400 nm at a rate of 1 nm/s and a slit width of 2 nm.
A modified method by He et al. (2020) and Joshi et al. (2012), using 8 mM ANS prepared in 10 mM phosphate buffer (pH 7.2) as a probe, was followed. A concentration curve of 0.0–0.05% (w/v) and protein solutions were prepared using 10 mM phosphate buffer (pH 7.2). Briefly, 50 μL of ANS probe was added to 4 mL of each protein concentration, and then incubated in the dark for 5 min. The relative fluorescence intensity (RFI) of each dilution was then measured using a QuantaMasterTM spectrofluorometer from Photon Technology International Inc. (London, ON) with extraction/emission slits set to 5 nm, and excitation and emission wavelength set to 380 and 480 nm respectively. The net RFI was calculated using Equation 4:
The slope of the net RFI vs. protein concentration was calculated using linear regression analysis and used as an index of surface hydrophobicity (H0-ANS).
Protein solubility was determined using a combination of the methods described by Yin et al. (2010) and Ortiz and Wagner (2002). Protein solutions with concentrations of 10 mg/mL were prepared using 0.1 M phosphate buffers with pH values of 4.0, 6.0, 8.0, and 10.0. The samples were stirred for 45 min at room temperature and then centrifuged at 5,000 x g for 20 min. The protein concentrations of the supernatants were determined using the Bradford assay (1976) and the protein solubilities were determined using Equation 5:
Water holding capacity (WHC) and oil holding capacity (OHC) were determined following a modified version of the methods by Mohan and Mellem (2020). Briefly, 2.5 g of corn oil or distilled water were added to 0.25 g of sample to determine OHC and WHC, respectively. The samples were vortexed every 5 min for 10 sec for a total of 30 min. Afterwards, the samples were centrifuged for 15 min at 1800 x g. Finally, the supernatant was carefully pipetted off, and the remaining pellets were weighed. The following equation was used to calculate the OHC and WHC:
A minimum of three trials in triplicate for each sample were conducted for all protein concentrate experiments except for the bean flour samples (protein concentration determination and the ash analyses). The averaged means from the trials were calculated and reported as means ± standard deviation. Statistical differences were calculated using one-way analysis of variance (ANOVA) with Tukey's multiple comparison test at p < 0.05 in XLSTAT® software version 2020.2 (New York, NY). In addition, Pearson correlation (r) analysis was used to determine significant correlations between physicochemical properties and functional properties.
Protein content in the raw YE bean flour was 26.5%, and the highest value was observed in the flour from the dehulled/soaked beans (27.9%). Similar protein yields (20–30%) have been reported in the literature for other beans (Mundi and Aluko, 2012). The flour from the raw and soaked samples registered the highest ash content, 4.1 and 4.2%, respectively, whereas the lowest ash value of 3.6% was registered in flour samples from dehulled/soaked and dehulled/germinated seeds (Table 1).
Table 1. Ash and protein content of flour samples generated from treated and untreated yellow eye (YE) beans.
Extraction yields and protein recovery yields for alkaline extracted protein concentrates (APCs) ranged from 12.2 ± 0.5%−14.9 ± 0.28% and 43–56%, respectively (Table 2). Concentrate yields and protein recovery for salt extracted protein concentrates (SPCs) were much lower, ranging from 4.2–6.4% and 16–23% respectively. Ammonium sulfate because of its high solubility in water and negligible effects on protein has been the preferred salt used to extract protein fractions from other pulses including mungbeans, (Mendoza et al., 2001), and cowpea (Rangel et al., 2003). However, compared to alkaline extraction methods, protein yields from salt concentrates are often lower. Karaca et al. (2011) reported lower protein yields in SPCs for chickpeas and lentils. In addition, Yang et al. (2021) also reported lower protein yields in extracts from yellow peas, and these observations were attributed to greater carbohydrate solubility in salt solutions. Sathe (2002) emphasized that carbohydrates are the major non-protein components in beans; and their complete removal is required for adequate protein separation. Other factors including high salt concentrations as well as the presence of lipids have been shown to impact protein solubilization which in turn impacts the protein yield (Aluko, 2004; Deak et al., 2006). Table 2 shows the carbohydrate (59.3–64%) and lipid (0.8 to 1.4%) values reported in the present study which are similar to published results (Karaca et al., 2011). The low lipid contents in the flour samples, suggests that in this instance, lipids may not have a significant impact on the protein extraction yields, however, the interfering effect of carbohydrates cannot be ruled out.
Table 2. Protein extraction efficiency of untreated and pre-treated Yellow Eye bean protein concentrates prepared by alkaline and salt-extraction methods.
Both germination and dehulling pre-treatments impacted the yields of the protein concentrates generated from the alkaline and salt extractions. Compared to the raw beans used for alkaline extractions (RBA), germination decreased the yields in concentrates from beans that were germinated then alkaline extracted (WGA). Yields were also decreased in samples that were dehulled/germinated before undergoing a similar extraction process (DGA) (Table 2). The concentrate yield and recovery yield of the whole germinated seeds that underwent salt extractions (WGS) were also significantly decreased when compared to concentrates obtained from beans that were soaked followed by protein extraction using salts (WSS). These observations are opposite to the results of Sofi et al. (2020), who found a slight increase in yields from alkaline extracted protein concentrates generated from germinated chickpeas. This is potentially due to differences in germination time, as Sofi et al. (2020) used a shorter time of 48 h, whereas the beans in the present study were left to germinate for up to 72 h. Indeed, a study by Rumiyati and Jayasena (2012) highlighted a decrease in protein concentrate yield in dehulled/germination Australian sweet lupin APIs starting at 72 h of germination, and a continued decrease in concentrate yield from 17.6 ± 3.8% on day 3, to 4.7 ± 0.4% on day 9 of germination. Thus, it is likely that by 72 h, protein was being used as an energy source of germination in common beans (Ali and Elozeiri, 2017), which might offer a possible explanation for the lower extraction yields reported in the present study. For example, using the alkali method, the extraction yield registered for the germinated samples was 12.2 ± 0.49 % compared to 14.9± 0.28% for the raw YE bean (Table 2). Lower yields were observed in the salt extracted concentrates, 4.26 ± 0.31% for the raw beans vs. 4.31 ± 0.38% for the germinated samples. It is well known that beans are composed of different protein fractions, however, differences in the solubility of these fractions may have contributed to the lower yields obtained in the composite protein concentrates. Boyle et al. (2018) also highlighted that extraction procedures that combine more than one extraction solvent, can maximize solubilization of different protein fractions, which in turn improves extraction yields.
Dehulling did not improve the protein yields in the alkaline extracted concentrates, however, the dehulled salt concentrates showed a significant increase in yield compared to the concentrates generated from raw beans whose proteins were extracted with salt solutions (RBS). Seed coats primarily consist of carbohydrates and only contained 2–8% protein (Zhong et al., 2018). Therefore, removing the seed coat can increase the amount of protein to non-protein components, thus increasing concentrate yield.
Combined pre-treatments of dehulling and germination also improved protein extraction efficiency in the salt extracted samples (DGS). However, this was not observed for similar pre-treated beans that underwent alkaline extractions (DGA), in these samples, concentrate and protein recovery yields both decreased compared to concentrates from untreated beans (RBA) as well as those from beans that were soaked prior to alkali extractions, the WSA concentrates. These differences may be due to the presence of condensed tannins and protein-tannin interactions, which can form tannin-protein complexes and reduce protein solubility under alkaline conditions (Tajoddin et al., 2014; Shen et al., 2020). Because the seed coats were removed after germination in the present study, it is possible that these condensed tannins diffused into the cotyledon during the germination process (Chagas and Santoro, 1997). Condensed tannins can be extracted through alkaline methods and can form tannin-protein interactions which have been found to limit extraction yields at pH 8.0 (Brouwer et al., 2019). Moreover, the addition of salt solutions to proteins has been linked with decreased protein-tannin affinity (Kilmister et al., 2016).
Significant variations were observed in the physiochemical properties of the untreated and pre-treated concentrates obtained from salt and alkaline extractions. As hypothesized, the measured physiochemical properties of the salt extracted concentrates exhibited reduced conformational changes when compared to the alkaline extracted concentrates, which showed signs of protein denaturation. In addition, the pre-treatments resulted in changes to the tertiary structure of the protein concentrates and the exposure of more hydrophobic surface regions in the alkaline extracted concentrates, as indicated by the higher surface hydrophobicity values ranging from 398 ± 0.7–537 ± 9.6, (Figure 2). The opposite was observed in concentrates from salt extraction solutions where hydrophobicity values ranged from 77.6 ± 5.7 to 121.4 ± 5.3. Similar results were also reported by Yang et al. (2021) who recently showed that pea protein isolates extracted by an alkaline method exhibited a greater effect on protein conformation compared to protein extracted using salt solutions; higher surface hydrophobicity values (847.9 ± 32.9) were also reported for the alkaline extracted pea concentrates compared to the salt extracted counterparts (732.2 ± 42.8).
Figure 2. Surface hydrophobicity index (H0) of alkaline- and salt-extracted Yellow Eye bean protein concentrates (n = 3). Different letters (a to g) represent significant differences by Tukey's multiple comparison test (p < 0.05).
Variations in the physiochemical characteristics of the concentrates may be attributed to: (1) the types of proteins extracted and isolated during each extraction process and, (2) the conformation and structural changes to the proteins that occurred during the extraction process (Stone et al., 2015; Yang et al., 2021). Differences in the physiochemical properties may also occur because of the types of proteins extracted using salt vs. alkaline techniques. For example, salt extraction procedures typically isolate both globulins and albumins, whereas alkaline extraction methods primarily extract and precipitate the globulin fractions due to the pH of the precipitation step (Karaca et al., 2011; Tanger et al., 2020). Differences in protein fractions were also observed in the SDS-PAGE data, as the alkaline extracted concentrates had thicker bands because of the globulin subunits (40 and 45 kDa) whereas a smaller albumin band (27 kDa) was observed in the salt extracted concentrates (Figure 3). This alteration of the globulin/albumin ratio can influence the physiochemical characteristics of the protein. Importantly, since globulins tend to be more hydrophobic than albumins (Mundi and Aluko, 2012), this property can explain the higher surface hydrophobicity (H0-ANS) observed in alkaline concentrates compared to those from the salt extraction (Figure 3). In addition, globulins can also dissociate into their 11S legumin and 7S vicilin subunits under acidic and alkaline conditions, thus further exposing more hydrophobic side chains which in turn increases surface hydrophobicity (Papalamprou et al., 2010).
Figure 3. Sodium dodecyl sulfate-polyacrylamide gel electrophoresis profiles of Yellow Eye bean proteins extracted using salt- (A) and alkaline-extraction (B) techniques. Molecular distribution was compared to a New England BioLabs Precision Plus pre-stained protein marker (P77066, 10–250 kDa) in lane 1. The samples were separated using a 12% mini-Protean precast gel stained with Coomassie Brilliant Blue (0.1%).
The fluorescence spectra of the aromatic amino acids, phenylalanine, tryptophan, and tyrosine can be used as a tool to observe changes in the tertiary structure of proteins caused by solvents used for protein extraction as well as pre-treatments used before extraction (Johnson, 2006). Since bean protein isolates have been reported to contain ~ 6.0% phenylalanine, and 3.4% tyrosine, (and tryptophan not determined) (Fernández-Quintela et al., 1997; Boye et al., 2010), this intrinsic fluorescence variability may be used to detect differences in the treated and untreated protein concentrates. Similar approaches have been used by other researchers to evaluate structural changes in pea protein isolates (Yang et al., 2021) and black turtle bean protein isolates (He et al., 2020). The maximum fluorescence emission registered for alkaline extracted concentrates in the present study was much lower than that observed for the salt extracted concentrates (Figures 4A,B), indicating a decrease of exposed aromatic amino acid residues (Yang et al., 2021). Indeed, a negative correlation was observed between hydrophobicity and intrinsic fluorescence (r = −0.8725). This negative correlation may result from the formation of less compact structures which are induced by partial protein unfolding that is promoted by the alkaline conditions (Jiang et al., 2009; Yang et al., 2021). Decreased maximum fluorescence may also be a result of inter-intermolecular hydrophobic interactions of previously exposed tryptophan chromophores, thus promoting aggregation (Shen and Tang, 2012).
Figure 4. (A) Intrinsic fluorescence measurement of alkaline-extracted protein concentrates and (B) salt-extracted protein concentrates prepared from untreated and pre-treated Yellow Eye beans (0.2 mg/mL in 10 mM phosphate buffer, pH 7.2) (n = 3).
In addition, a shift toward higher wavelengths (red shift) was observed in the pre-treated concentrates obtained by alkaline extraction, and the maximum emission wavelength of the soaked, WSA (328 nm), germinated, WGA (329 nm), and dehulled concentrates, DGA (328 nm) increased compared to the alkaline concentrates from raw beans, RBA (327 nm). Conversely, the maximum emission wavelength (327 nm) of the alkaline protein concentrates from dehulled beans, DSA, remained the same (Figure 4A). The fluorescence intensity of the germinated alkaline protein concentrates also increased compared to the alkaline protein concentrates from raw beans, RBA. This observation indicates that germination and the required soaking process had created a more hydrophobic environment for the tryptophan residues, thereby increasing the surface availability of hydrophobic amino acids (Ghavidel and Prakash, 2007). Indeed, the surface hydrophobicity of all the pre-treated samples also increased compared to the alkaline protein concentrates from raw beans, RBA (Figure 2). All these measurements can be linked to the conformational changes of the extracted proteins (He et al., 2020). Specifically, alkaline protein concentrates from germinated beans, WGA as well as alkaline protein concentrates from dehulled and germinated beans, DGA, had significantly higher surface hydrophobicity compared to their ungerminated counterparts. This indicates an increase in exposed hydrophobic sites of amino acids. Enzymatic protein degradation is also one of the mechanisms initiated during germination which can alter protein conformation and/or denaturation and expose hydrophobic areas of the protein, thus increasing surface hydrophobicity (Ghavidel and Prakash, 2007; Zahir et al., 2021).
Figure 4B shows the impact of the various pre-treatments on the fluorescence patterns observed for the protein concentrates generated from salt extractions. A spectral shift toward lower wavelength (blue shift) was observed in the pre-treated, salt extracted protein concentrates, with decreased maximum emission wavelengths for the soaked, WSS (324 nm), germinated, WGS (325 nm), dehulled and germinated, DGS (325 nm) protein concentrates compared to those of the raw, RBS (327 nm) and dehulled salt extracted protein concentrates, DSS (327 nm) (Figure 5). In addition, the fluorescence intensity of the pre-treated salt extracted protein concentrates decreased compared to fluorescence intensity from the salt extracted concentrates from raw beans, RBS. This indicated an increase of tryptophan residues exposed to a hydrophilic environment, thus decreasing the surface availability of hydrophobic amino acids (He et al., 2020). In particular, the surface hydrophobicity of the soaked (WSS) and dehulled, (DSS) salt extracted protein concentrates decreased compared to the hydrophobicity of the salt extracted protein concentrates from raw beans, RBS, which is likely due to the leaching of insoluble proteins into the soaking water (Barak et al., 2014). However, it should be noted that the surface hydrophobicity registered for the salt extracted protein concentrates from the germinated, beans (WGS) was significantly higher when compared to the soaked samples (WSS). These changes can be linked to the degradation of nutrients used for energy during germination and the increased exposure of hydrophobic amino acids (Xu et al., 2020; Zahir et al., 2021).
Figure 5. (A) Protein solubility (% protein dissolved, 10 mg/mL in 0.1 M Phosphate buffer, pH values 4 to 10) of untreated and pre-treated Yellow Eye bean protein concentrates prepared using alkaline extraction; (B) and salt solutions (n = 3).
The conformation of a protein has long been established as an important property that can impact protein functionality (Boye et al., 2010). Contrary to the initial hypothesis, salt extracted protein concentrates exhibited decreased functionality when compared to the alkaline extracted concentrates, particularly relating to protein solubility. The solubilities of the alkaline extracted protein concentrates were significantly impacted by pH, as the concentrates precipitated at pH 4.0, and then solubility steadily increased as the pH of the concentrates also increased (Figure 5A). Interestingly, the impact of pH on salt extracted concentrates was less severe (Figure 5B). This can once again be attributed to the type of protein fractions extracted.
The isoelectric point of globulins is pH 4.5, which explains the insolubility of alkaline extracted protein concentrates at pH 4.0 (Tanger et al., 2020). The combination of albumin and globulin protein fractions extracted in the salt extracted protein concentrates would explain the lack of precipitation at pH 4.0, as albumin has been found to be more soluble than globulin at pH 4.0 (Makeri et al., 2017). It should be noted that the solubility of both alkaline and salt extracted protein concentrates in this study are typically lower than other pulse protein concentrates, particularly at more alkaline pH values (Mundi and Aluko, 2012; Shevkani et al., 2015; Ge et al., 2021). Paraman et al. (2007) attributed high insolubility to be a result of higher rates of interpeptide hydrophobic interactions or sulfhydryl-disulfide interactions. These changes can prevent protein-water interactions and thus reduce solubility. A better understanding of the bonds and linkages present in these bean protein concentrates would further elucidate these interactions.
Although the salt extracted concentrates had lower surface hydrophobicity values, these samples demonstrated lower solubility curves compared to raw beans extracted in salt solutions (RBS). Several factors including protein structure, surface charge, and the degree of aggregation can influence protein solubility (Hayakawa and Nakai, 1985). The lower solubility of the pre-treated salt extracted protein concentrates may be a result of intermolecular aggregation of its ß-sheets (Yang et al., 2021). On the other hand, the pre-treated alkaline extracted protein concentrates, particularly the germinated (WGA) and dehulled/germinated (DGA) samples, had increased solubility curves compared to the alkaline extracted protein concentrates from raw beans (RBA). This can be attributed once again to protein hydrolysis of globulins and the exposure of hydrophilic proteins for protein-water interactions (Cao et al., 2010).
Due to an increase in hydrophobic surface regions, the alkaline protein concentrates exhibited more hydrophobic functional characteristics, including increased OHC and decreased WHC when compared to the salt extracted concentrates. The higher OHC and lower WHC of alkaline protein concentrates compared to salt extracted concentrates can be explained by variations in protein conformation (Figures 6A,B). The exposure of hydrophobic side chains produced through the alkaline extraction process may be responsible for the higher OHC and the relatively low solubility of alkaline proteins (Figures 5A, 6A) compared to the literature (Karaca et al., 2011). In theory, an increase in hydrophobicity would result in a decrease of hydrophilic groups on the surface of the protein, thus, limiting the potential for protein-water interactions and decreasing WHC (Stone et al., 2015; Mohan and Mellem, 2020). Indeed, our own observations showed that surface hydrophobicity was positively correlated with OHC (r = 0.877) and negatively correlated with WHC (r = −0.618).
Figure 6. (A) Water holding capacity of alkaline- and salt-extracted Yellow Eye bean protein; (B) Oil holding capacity of alkaline- and salt-extracted Yellow Eye bean protein concentrates. Different letters (a to e) represent significant differences by Tukey's multiple comparison test (p < 0.05), (n = 3).
Variation in pre-treatment effects on OHC, WHC, and protein solubility were also observed. In general, pre-treated alkaline extracted protein concentrates had decreased WHC compared to similar concentrates from raw beans, RBA. This can be attributed to the increase in surface hydrophobicity properties. In addition, the removal of water-binding matrix components including starch and fiber have been found to decrease WHC in yellow field pea alkaline extracted protein concentrates (Agboola et al., 2010). It is also possible that the extracted protein was denatured during the extraction process, which can also correlate with decreased WHC (Mohan and Mellem, 2020). These phenomena are most likely responsible for the decreased WHC of all other pre-treated alkaline extracted protein concentrates compared to similar extracted concentrates from raw beans (RBA). Conversely, the germinated salt extracted protein concentrates (WGS) and the germinated/ dehulled salt extracted concentrates (DGS) had significantly higher WHCs compared to salt extracted concentrates from raw (RBS) and soaked (WSS) beans. This can be explained by the increase in soluble proteins generated during proteolysis (Sofi et al., 2020).
Despite increases in hydrophobic properties in the alkaline extracted protein concentrates, no significant differences in OHC were established between the concentrates from raw beans (RBA) and other alkaline extracted concentrates. However, the OHC of dehulled, alkaline extracted concentrates DSA was significantly lower than that of the soaked, WSA, germinated, WGA and dehulled DGA, alkaline extracted protein concentrates. Indeed, the surface hydrophobicity of the dehulled concentrates (DSA) was significantly lower than the dehulled/germinated (DGA) alkaline extracted concentrates, and there was no red shift in maximum emission wavelength. These changes can be attributed to a decrease in exposed hydrophobic amino acids, potentially due to tannin-protein interactions (Pal et al., 2017). Interestingly, despite the lower surface hydrophobicity of the dehulled sample (DSS), its OHC was significantly higher than that of the raw beans (RBS). The solubility curve of the salt extracted dehulled concentrate (DSS) was also decreased compared to concentrates from raw beans (RBS). Conformational changes that occurred during the extraction process may have attributed to these changes (Yang et al., 2021).
Although the salt extracted concentrates had lower surface hydrophobicity values, these samples demonstrated lower solubility curves compared to raw beans extracted in salt solutions (RBS). Several factors including protein structure, surface charge, and the degree of aggregation can influence protein solubility (Hayakawa and Nakai, 1985). The lower solubility of the pre-treated salt extracted protein concentrates may be a result of intermolecular aggregation of its ß-sheets (Yang et al., 2021). On the other hand, the pre-treated alkaline extracted protein concentrates, particularly the germinated (WGA) and dehulled/germinated (DGA) samples, had increased solubility curves compared to the alkaline extracted protein concentrates from raw beans (RBA). This can be attributed once again to protein hydrolysis of globulins and the exposure of hydrophilic proteins for protein-water interactions (Cao et al., 2010).
This study assessed the impact of dehulling and germination and a combination of these pre-treatments on the protein extraction efficiency, physiochemical properties, protein solubility and the water/oil holding capacities of alkaline, and salt extracted protein concentrates from YE beans. In general, alkaline extracted protein concentrates had a higher protein extraction efficiency compared to salt extraction samples, however the former was more susceptible to conformational changes as indicated by the increase in surface hydrophobicity. This increase in hydrophobic surface regions, observed in the alkaline extracted protein concentrates resulted in an increase in OHC and a decrease in the WHC when compared to the salt extracted concentrates. When applied individually, dehulling of beans resulted in protein concentrates with increased yields in the salt extraction solutions compared to the protein concentrates generated from raw beans when proteins were extracted in salt solutions. Conversely, beans that were germinated prior to protein extraction registered greater surface hydrophobicity when compared to the soaked samples. Moreover, the combination of dehulling and germination pre-treatment decreased protein extraction efficiency and functionality when compared to the untreated and soaked alkaline extracted protein concentrates. The combined pre-treatments also resulted in improved protein extraction yields, physiochemical properties, and the functionality of the salt extracted concentrates. The beneficial effect observed from the combined pre-treatment (dehulling/ germination) on the salt extracted protein concentrates suggests that these protein samples may be good potential candidates for further studies that evaluate their application in different food formulations.
The original contributions presented in the study are included in the article/supplementary material, further inquiries can be directed to the corresponding author.
LV co-designed the study, collected all data, interpreted results, drafted the manuscript, and approved the submission version. ME co-designed the study, supervision, and resources, edited, and proofread the manuscript, and approved the submission version. All authors contributed to the article and approved the submitted version.
The authors acknowledge the financial support of the Natural Sciences and Engineering Research Council of Canada (NSERC). NSERC Discovery Grant (#03195) to ME and NSERC Alexander Graham Bell Canada Graduate Scholarship to LV.
The authors declare that the research was conducted in the absence of any commercial or financial relationships that could be construed as a potential conflict of interest.
All claims expressed in this article are solely those of the authors and do not necessarily represent those of their affiliated organizations, or those of the publisher, the editors and the reviewers. Any product that may be evaluated in this article, or claim that may be made by its manufacturer, is not guaranteed or endorsed by the publisher.
Charles Forney (Agriculture and Agri Food Canada), Tamara Rodela (Saint Francis Xavier University), and Hiwot Haileslassie (University of Prince Edward Island) are gratefully acknowledged for their valuable input toward LV's graduate thesis.
Agboola, S., Mofolasayo, O., Watts, B., and Aluko, R. (2010). Functional properties of yellow field pea (Pisum sativum L) seed flours and the in vitro bioactive properties of their polyphenols. Food Res. Int. 43, 582–588. doi: 10.1016/j.foodres.2009.07.013
Ali, A. S., and Elozeiri, A. A. (2017). “Metabolic processes during seed germination”, in Advances in Seed Biology: Metabolic Processing during Seed Germination, ed J. C. Limenez-Lopez (Rijeka: Intech). doi: 10.5772/intechopen.70653
Aluko, R. (2004). Proteins: In Food Processing. Cambridge: Woodhead Publishing. p. 323–351. doi: 10.1533/9781855738379.2.323
Aluko, R., and McIntosh, T. (2004). Electrophoretic and functional properties of mustard seed meals and protein concentrates. J. Am. Oil Chem. Soc. 81, 679–683. doi: 10.1007/s11746-004-961-0
AOAC (2005). “Ash of Flour (Direct Method), Method 923.03”, in Official Methods of Analysis, 18th Edn. Gaithersburg: AOAC International Publisher.
Barak, S., Mudgil, D., and Khatkar, B. S. (2014). Effect of flour particle size and damaged starch on the quality of cookies. J. Food Sci. Technol. 51, 1342–1348. doi: 10.1007/s13197-012-0627-x
Boye, J., Zare, F., and Pletch, A. (2010). Pulse proteins: processing, characterization, functional properties and applications in food and feed. Food Res. Int. 43, 414–431. doi: 10.1016/j.foodres.2009.09.003
Boyle, C., Hansen, C., Hinnenkamp, C., and Ismail1, B. (2018). Emerging Camelina protein: Extraction, modification, and structural/functional characterization. J. Am. Oil Chem. Soc. 95, 1049–1062. doi: 10.1002/aocs.12045
Bradford, M. M. (1976). Rapid and sensitive method for quantification or microgram quantities of protein utilizing principle of protein dye binding. Anal. Biochem. 72, 248–254. doi: 10.1016/0003-2697(76)90527-3
Brouwer, P., Nierop, K. G. J., Huijgen, W. J. J., and Schluepmann, H. (2019). Aquatic weeds as novel protein sources: Alkaline extraction of tannin-rich Azolla. Biotechnol. Rep. 24, e00368. doi: 10.1016/j.btre.2019.e00368
Burger, T. G., and Zhang, Y. (2019). Recent progress in the utilization of pea protein as an emulsifier for food applications. Trends Food Sci. Technol. 86, 25–33. doi: 10.1016/j.tifs.2019.02.007
Cao, X., Li, C., Wen, H., and Gu, Z. (2010). Extraction technique and characteristics of soluble protein in germinated brown rice. Int. J. Food Prop. 13, 810–820. doi: 10.1080/10942910902895200
Chagas, E. P., and Santoro, L. G. (1997). Globulin and albumin proteins in dehulled seeds of three Phaseolus vulgaris cultivars. Plant Foods Hum. Nutri. 51, 17–26. doi: 10.1023/A:1007971329420
Cui, L., Bandillo, N., Wang, Y., Ohm, J., Chen, B., and Rao, J. (2020). Functionality and structure of yellow pea protein isolate as affected by cultivars and extraction pH. Food Hydrocoll. 108, 106008. doi: 10.1016/j.foodhyd.2020.106008
de Evangelho, J. A., Vanier, N. L., Pinto, V. Z., De Berrios, J. J., Dias, A. R. G., and Rosa Zavareze, E. (2017). Black beans (Phaseolus vulgaris L.) protein hydrolysates: Physiochemical and functional properties. Food Chem. 214, 460–467. doi: 10.1016/j.foodchem.2016.07.046
Deak, N. A., Murphy, P. A., and Johnson, L. A. (2006). Effects of NaCl concentration on salting-in and dilution during salting-out on soy protein fractionation. J. Food Sci. 71, C247–C254. doi: 10.1111/j.1750-3841.2006.00028.x
Du, M., Xie, J., Gong, B., Xu, X., Tang, W., Li, X., et al. (2018). Extraction, physiochemical characteristics and functional properties of mung bean protein. Food Hydrocolloids 76, 131–140. doi: 10.1016/j.foodhyd.2017.01.003
English, M. M., Viana, L., and McSweeney, M. B. (2019b). Effects of soaking on the functional properties of yellow-eyed beans flour and the acceptability of chocolate brownies. J. Food Sci. 84, 623–628. doi: 10.1111/1750-3841.14485
English, M. M., Viana, L., Michael, J., and Forney, C. F. (2019a). Characterizing the impact of soaking and germination on Yellow-Eyed bean flour. J. Food. Nutr. Sci. 1, 139–148. doi: 10.1057/jfns000018
Fernández-Quintela, A., Macarulla, M.T., Del Barrio, A.S., and Martínez, J.A. (1997). Composition and functional properties of protein isolates obtained from commercial legumes grown in northern Spain. Plant Foods Hum. Nutr. 51, 331–341. doi: 10.1023/A:1007936930354
Fernando, S. (2021). Pulse protein ingredient modification. J. Sci. Food Agric. 102, 892–897. doi: 10.1002/jsfa.11548
Foegeding, E., and Davis, J. (2011). Food protein functionality: a comprehensive approach. Food Hydrocoll. 25, 1853–1864. doi: 10.1016/j.foodhyd.2011.05.008
Ge, J., Sun, C.-X., Mata, A., Corke, H., Gan, R.-Y., and Fang, Y. (2021). Physicochemical and pH-dependent functional properties of proteins isolated from eight traditional Chinese beans. Food Hydrocoll. 112, 106288. doi: 10.1016/j.foodhyd.2020.106288
Ghavidel, R. A., and Prakash, J. (2007). The impact of germination and dehulling on nutrients, antinutrients, in vitro iron and calcium bioavailability and in vitro starch and protein digestibility of some legume seeds. LWT Food Sci. Technol. 40, 1292–1299. doi: 10.1016/j.lwt.2006.08.002
Grover, K., and Ryall, R. (2005). Critical appraisal of salting-out and its implications for chemical and biological sciences. Chem. Rev. 105, 1–10. doi: 10.1021/cr030454p
Hadnadev, M. S., Hadnadev, T. R. D., Pojić, M. M., Šarić, B. M., Mišan, A. C., Jovanov, P. T., et al. (2017). Progress in vegetable proteins isolation techniques: a review. Food Feed Res. 44, 11–21. doi: 10.5937/FFR1701011H
Hayakawa, S., and Nakai, S. (1985). Relationships of hydrophobicity and net charge to the solubility of milk and soy proteins. J. Food Sci. 50, 486–491. doi: 10.1111/j.1365-2621.1985.tb13433.x
He, S., Zhao, J., Cao, X., Ye, Y., Wu, Z., Yue, J., et al. (2020). Low pH-shifting treatment would improve functional properties of black turtle bean (Phaseolus vulgaris L.) protein isolate with immunoreactivity. Food Chem. 330, 127217. doi: 10.1016/j.foodchem.2020.127217
Jiang, J., Chen, J., and Xiong, Y. L. (2009). Structural and emulsifying properties of soy protein isolate subjected to acid and alkaline pH-shirting processes. J. Agric. Food Chem. 57, 7576–7583. doi: 10.1021/jf901585n
Johnson, A. E. (2006). Fluorescence approaches for determining protein conformations, interactions and mechanisms at membranes. Traffic. 6, 1078–1092. doi: 10.1111/j.1600-0854.2005.00340.x
Joshi, M., Adhikari, B., Aldred, P., Panozzo, J. F., Kasapis, S., and Barrow, C. J. (2012). Interfacial and emulsifying properties of lentil protein isolate. Food Chem. 134, 1343–1353. doi: 10.1016/j.foodchem.2012.03.029
Karaca, A. C., Low, N., and Nickerson, M. (2011). Emulsifying properties of chickpea, faba bean, lentil and pea proteins produced by isoelectric precipitation and salt extraction. Food Res. Int. 44, 2742–2750. doi: 10.1016/j.foodres.2011.06.012
Kilmister, R. L., Faulkner, P., Downey, M. O., Darby, S. J., and Falconer, R. J. (2016). The complexity of condensed tannin binding to bovine serum albumin – An isothermal titration calorimetry study. Food Chem. 190, 173–178. doi: 10.1016/j.foodchem.2015.04.144
Liu, Y., Xu, M., Wu, H., Jing, L., Gong, B., Gou, M., et al. (2018). The compositional, physicochemical, and functional properties of germinated mung bean flour and its addition on quality of wheat flour noodle. J. Food Sci. Technol. 55, 5142–5152. doi: 10.1007/s13197-018-3460-z
Ma, K., Greis, M., Lu, J., Nolden, A., McClements, D., and Kinchla, A. (2022). Functional performance of plant proteins. Foods 11, 594. doi: 10.3390/foods11040594
Ma, Z., Boye, J. I., and Hu, X. (2018). Nutritional quality and techno-functional changes in raw, germinated and fermented yellow field pea (Pisum sativum L.) upon pasteurization. LWT. 92, 147–154. doi: 10.1016/j.lwt.2018.02.018
Makeri, M. U., Mohamed, S. A., Karim, R., Ramakrishnan, Y., and Muhammad, K. (2017). Fractionation, physicochemical, and structural characterization of winged bean seed protein fractions with reference to soybean. Int. J. Food Prop. 20, 2220–2236. doi: 10.1080/10942912.2017.1369101
Mendoza, E., Adachi, M., Emiliana, A., Bernardo, N., and Utsumi, S. (2001). Mungbean (Vigna radiata (L.) Wilczek) Globulins: Purification and Characterization. J. Agric. Food Chem. 49, 1552–1558. doi: 10.1021/jf001041h
Mohan, N., and Mellem, J. J. (2020). Functional properties of the protein isolates of hyacinth bean [Lablab purpureus (L.) Sweet]: An effect of the used procedures. LWT 129, 109572. doi: 10.1016/j.lwt.2020.109572
Momen, S., Alavi, F., and Aider, A. (2021). Alkali-mediated treatments for extraction and functional modification of proteins: Critical and application review. Trends Food Sci. Technol. 110, 778–797. doi: 10.1016/j.tifs.2021.02.052
Mundi, S., and Aluko, R. E. (2012). Physicochemical and functional properties of kidney bean albumin and globulin protein fractions. Food Res. Int. 48, 299–306. doi: 10.1016/j.foodres.2012.04.006
Nwachukwu, I., and Aluko, R. (2021). “Food Protein Structures, Functionality and Product Development”, in Food Proteins and Peptides: Emerging Biofunctions, Food and Biomaterial Applications (London: The Royal Society of Chemistry), p. 1–33. doi: 10.1039/9781839163425-00001
Ortiz, S. E. M., and Wagner, J. R. (2002). Hydrolysates of native and modified soy protein isolates: structural characteristics, solubility and foaming properties. Food Res. Int. 35, 511–518. doi: 10.1016/S0963-9969(01)00149-1
Pal, R. S., Bhartiya, A., Yadav, P., Kant, L., Mishra, K. K., Aditya, J. P., et al. (2017). Effect of dehulling, germination and cooking on nutrients, anti-nutrients, fatty acid composition and antioxidant properties in lentil (Lens culinaris). J. Food Sci. Technol. 54, 909–920. doi: 10.1007/s13197-016-2351-4
Papalamprou, E. M., Doxastakis, G. I., and Kiosseoglou, V. (2010). Chickpea protein isolates obtained by wet extraction as emulsifying agents. J. Sci. Food Agric. 90, 304–313. doi: 10.1002/jsfa.3816
Paraman, I., Hettiarachchy, N. S., Schaefer, C., and Beck, M. I. (2007). Hydrophobicity, solubility, and emulsifying properties of enzyme-modified rice endosperm protein. Cereal Chem. 84, 343–349. doi: 10.1094/CCHEM-84-4-0343
Rahmati, N. F., Koocheki, A., Varidi, M., and Kadkhodaee, R. (2018). Introducing Speckled sugar bean (Phaseolus vulgaris) protein isolates as a new source of emulsifying agent. Food Hydrocoll. 79, 498–508. doi: 10.1016/j.foodhyd.2018.01.022
Rangel, A., Domont, G., Pedrosa, C., and Ferreira, S. (2003). Functional properties of purified vicilins from cowpea (Vigna unguiculata) and pea (Pisum sativum) and cowpea protein isolate. J. Agric. Food Chem. 51, 5792–5797. doi: 10.1021/jf0340052
Rui, X., Boye, J. I., Ribereau, S., Simpson, B. K., and Prasher, S. O. (2011). Comparative study of the composition and thermal properties of protein isolates prepared from nine Phaseolus vulgaris legume varieties. Food Res. Int. 44, 2497–2504. doi: 10.1016/j.foodres.2011.01.008
Rumiyati, A. P. J., and Jayasena, V. (2012). Effect of germination on the nutritional and protein profile of Australian sweet lupin (Lupinus angustifolius L). Food Nutr. Sci. 3, 19073. doi: 10.4236/fns.2012.35085
Samtiya, M., Aluko, R. E., and Dhewa, T. (2020). Plant food anti-nutritional factors and their reduction strategies: an overview. Food Prod. Process. Nutr. 2, 1–14. doi: 10.1186/s43014-020-0020-5
Sathe, S. (2002). Dry bean protein functionality. Crit. Rev. Biotechnol. 22, 175–223, doi: 10.1080/07388550290789487
Shen, L., and Tang, C.-H. (2012). Microfluidization as a potential technique to modify surface properties of soy protein isolate. Food Res. Int. 48, 108–118. doi: 10.1016/j.foodres.2012.03.006
Shen, P., Gao, Z., Xu, M., Rao, J., and Chen, B. (2020). Physicochemical and structural properties of proteins extracted from dehulled industrial hempseeds: Role of defatting process and precipitation pH. Food Hydrocoll. 108, 106065. doi: 10.1016/j.foodhyd.2020.106065
Shevkani, K., Singh, N., Kaur, A., and Rana, J. C. (2015). Structural and functional characterization of kidney bean and field pea protein isolates: A comparative study. Food Hydrocoll. 43, 679–689. doi: 10.1016/j.foodhyd.2014.07.024
Sofi, A. S., Singh, J., Muzaffar, K., Majid, D., and Dar, B. N. (2020). Physicochemical characteristics of protein isolates from native and germinated chickpea cultivars and their noodle quality. Int. J. Gastron. Food Sci. 22, 100258. doi: 10.1016/j.ijgfs.2020.100258
Soller, L., Ben-Shoshan, M., Harrington, D. W., Knoll, M., Fragapane, J., Joseph, L., et al. (2015). Prevalence and predictors of food allergy in Canada: A focus on vulnerable populations. J. Allergy Clin. Immunol. Pract. 3, 42–49. doi: 10.1016/j.jaip.2014.06.009
Stone, A. K., Nosworthy, M. G., Chiremba, C., House, J. D., and Nickerson, M. T. (2015). A comparative study of the functionality and protein quality of a variety of legume and cereal flours. Cereal Chem. 96, 1159–1169. doi: 10.1002/cche.10226
Tajoddin, M., Manohar, S., and Lalitha, J. (2014). Effect of soaking and germination on polyphenol content and polyphenol oxidase activity of mung bean (Phaseolus Aureus L.) cultivars differing in seed color. Int. J. Food Proper. 17, 782–790. doi: 10.1080/10942912.2012.654702
Tanger, C., Engel, J., and Kulozik, U. (2020). Influence of extraction conditions on the conformational alteration of pea protein extracted from pea flour. Food Hydrocoll. 107, 105949. doi: 10.1016/j.foodhyd.2020.105949
Vainio, A., Niva, M., Jallinoja, P., and Latvala, T. (2016). From beef to beans: Eating motives and the replacement of animal proteins with plant proteins among Finnish consumers. Appetite 106, 92–100. doi: 10.1016/j.appet.2016.03.002
Vogelsang-O'Dwyer, M., Zannini, E., and Arendt, E. (2021). Production of pulse protein ingredients and their application in plant-based milk alternatives. Trends Food Sci. Technol. 110 364–374. doi: 10.1016/j.tifs.2021.01.090
Wang, N. (2008). Effect of variety and crude protein content on dehulling quality and on the resulting chemical composition of red lentil (Lens culinaris). J. Sci. Food Agric. 88, 885–890. doi: 10.1002/jsfa.3165
Xu, M., Jin, Z., Gu, Z., Rao, J., and Chen, B. (2020). Changes in odor characteristics of pulse protein isolates from germinated chickpea, lentil, and yellow pea: Role of lipoxygenase and free radicals. Food Chem. 314, 126184. doi: 10.1016/j.foodchem.2020.126184
Xu, M., Jin, Z., Lan, Y., Rao, J., and Chen, B. (2019). HS-SPME-GC-MS/olfactory combined with chemometrics to assess the impact of germination on flavor attributed of chickpea, lentil, and yellow pea flours. Food Chem. 280, 83–95. doi: 10.1016/j.foodchem.2018.12.048
Yang, J., Zamani, S., Liang, L., and Chen, L. (2021). Extraction methods significantly impact pea protein composition, structure, and gelling properties. Food Hydrocolloids 117, 106678. doi: 10.1016/j.foodhyd.2021.106678
Yin, S. W., Tang, C. H., Wen, Q. B., and Yang, X. Q. (2010). Functional and conformational properties of phaeseolin (Phaseolus vulgaris L.) and kidney bean protein isolate: a comparative study. J. Sci. Food Agric. 90, 599–607. doi: 10.1002/jsfa.3856
Zahir, M., Fogliano, V., and Capuano, E. (2021). Soybean germination limits the role of well wall integrity in controlling protein physicochemical changes during cooking and improves protein digestibility. Food Res. Int. 143, 110254. doi: 10.1016/j.foodres.2021.110254
Zhang, C., Sanders, J. P. M., and Bruins, M. E. (2014). Critical parameters in cost-effective alkaline extraction for high protein yield from leaves. Biomass Bioenergy 67, 466–472. doi: 10.1016/j.biombioe.2014.05.020
Keywords: plant protein, germination, dehulling, protein extraction, protein concentrate
Citation: Viana L and English M (2022) The Impact of Dehulling and Germination on the Physiochemical, Protein Solubility and Water and Oil Holding Capacities of Yellow Eye Bean (Phaseolus vulgaris L.) Protein Concentrates. Front. Sustain. Food Syst. 6:855788. doi: 10.3389/fsufs.2022.855788
Received: 16 January 2022; Accepted: 19 April 2022;
Published: 16 June 2022.
Edited by:
Sapna Langyan, National Bureau of Plant Genetic Resources (ICAR), IndiaReviewed by:
Pranjal Yadava, Indian Agricultural Research Institute (ICAR), IndiaCopyright © 2022 Viana and English. This is an open-access article distributed under the terms of the Creative Commons Attribution License (CC BY). The use, distribution or reproduction in other forums is permitted, provided the original author(s) and the copyright owner(s) are credited and that the original publication in this journal is cited, in accordance with accepted academic practice. No use, distribution or reproduction is permitted which does not comply with these terms.
*Correspondence: Marcia English, bWVuZ2xpc2hAc3RmeC5jYQ==
Disclaimer: All claims expressed in this article are solely those of the authors and do not necessarily represent those of their affiliated organizations, or those of the publisher, the editors and the reviewers. Any product that may be evaluated in this article or claim that may be made by its manufacturer is not guaranteed or endorsed by the publisher.
Research integrity at Frontiers
Learn more about the work of our research integrity team to safeguard the quality of each article we publish.