- 1Department of Industrial Engineering, University of Salerno, Fisciano, Italy
- 2ProdAl Scarl–University of Salerno, Fisciano, Italy
This study was focused on the optimization of the pulsed electric fields (PEF)-assisted extraction process using central composite design for response surface methodology from response surface methodology (RSM) with the aim to sustainably intensify the extractability of phenolic compounds from white grape pomace. The cell disintegration index (Zp) was used as response variable to identify the optimal PEF pre-treatment conditions of grape pomace in terms of field strength (E = 0.5–5 kV/cm) and energy input (WT = 1–20 kJ/kg), to be applied prior to the subsequent solid-liquid extraction (SLE) process. for both untreated and PEF-treated samples SLE process was optimized to determine the most effective combination of extraction temperature (20–50°C), extraction time (30–300 min), and solvent concentration (0–100% ethanol in water). Total phenolic content (TPC), flavonoid content (FC), and antioxidant activity (FRAP) of the obtained extracts were determined. The extracted compounds from untreated and PEF-treated samples at the optimal conditions were analyzed via HPLC-PDA analysis. Results revealed that, at a fixed extraction temperature (50°C), the application of PEF at optimal processing conditions (E = 3.8 kV/cm, WT = 10 kJ/kg) prior to SLE has the potential to reduce the solvent consumption (3–12%) and shorten the extraction time (23–103 min) to obtain the same recovery yield of phenolic compounds. Under optimized conditions, the extracts derived from PEF-treated samples showed significantly higher TPC (8%), FC (31%), and FRAP (36%) values, as compared to the control extraction. HPLC analyses revealed that epicatechin, p-coumaric acid, and quercetin were among the main phenolic compounds extracted, and no degradation phenomena occurred due to PEF application.
Introduction
Grapes of the cultivars Vitis vinifera are the basis of the majority of globally produced wines, with an annual production that, in 2018, has overcome 77 million tons (OIV International Organization of Vine Wine, 2019). The majority of cropped grapes is processed in wineries (Kammerer et al., 2014; Beres et al., 2017; Hogervorst et al., 2017; Maroun et al., 2017) with a global wine production accounting for 292 MhL in 2018 (OIV International Organization of Vine Wine, 2019).
However, during the winemaking process a large amount of grape processing wastes and by-products are also generated, including grape stalk, grape pomace or marc (skins and seeds), and wine lees/sediments, which account for up to 10–30% of the processed raw material (Kammerer et al., 2014; Barba et al., 2016; Sirohi et al., 2020). Among them, grape pomace are the major winery by-products, with a production of 20 kg for each hectoliter of wine (Buttol et al., 2018; Coelho et al., 2020), representing about 20–25% of the processed raw material (Chowdhary et al., 2021).
These by-products represent a major disposal problem for winery (Barba et al., 2016), where they currently find low-added value uses as animal feed or fertilizers (Casazza et al., 2010; Juri et al., 2019; Thirumdas et al., 2020), or used to recover renewable energy through anaerobic digestion of semi-solid wastes like lees and vinasses (Moletta, 2005; Barba et al., 2016), or sent to the distillery for the production of brandy from marc distillation (Muhlack et al., 2018).
However, they still represent a rich source of bioactive molecules including polyphenols, anthocyanins, tannins, and vitamins, which possess superior antioxidant activity (Andrade et al., 2019), as well as antimicrobial, anti-inflammatory, and anti-aging properties (Zhu et al., 2019; Da Rocha and Noreña, 2020; Kato-Schwartz et al., 2020; Sirohi et al., 2020).
Therefore, the valorization of grape by-products is a challenge, since it might improve the sustainability of wine production and create economic and social benefits, especially through the recovery of a gamut of bioactive compounds with high commercial value, for their potential applications as natural additives or active ingredients in food, cosmetic, and pharmaceutical products (Puértolas and Barba, 2016; Hogervorst et al., 2017; Maroun et al., 2017; Andrade et al., 2019; Sette et al., 2020).
The recovery of these bioactive compounds via conventional solid-liquid extraction (SLE) techniques is limited by the presence of the cell envelope (membranes and wall) in plant tissues, which exert a significant resistance against mass transfer phenomena of solvents and target intracellular compounds, slowing down the solvent extraction process (Corrales et al., 2008; Donsì et al., 2010a; Agati et al., 2012). This is motivating scientists to explore the use of cell disruption pre-treatment of plant residues that induces weakening or rupture of cell envelops, thus enhancing the extractability of target intracellular compounds from plant matrices, with reduced solvent, time, and energy consumption (Barba et al., 2015b).
In this frame, pulsed electric fields (PEF) is gaining great interest as gentle and scalable cell disruption technique of plant biomass (Carpentieri et al., 2022). PEF process consists in exposing plant tissues in wet form, placed between two metal electrodes, to repetitive short duration pulses (1 μ-1 ms) of moderate electric field (0.5–10 kV/cm) and relatively low energy input (1–20 kJ/kg), which leads to the permeabilization of cell membranes by pores formation, referred to as electroporation or electropermeabilization (Raso et al., 2016).
This has showed great potential to intensify the selective recovery of target intracellular compounds from various plant matrices derived from processing of fruit and vegetable (Puértolas and Barba, 2016), while lowering the energy and solvent consumption, and reducing the treatment time (Barba et al., 2015a; Frontuto et al., 2019; Pataro et al., 2020; Carpentieri et al., 2021, 2022).
However, to date, only few works demonstrated the feasibility of PEF technology to intensify the recovery yield of phenolic compounds from winery by-products (Corrales et al., 2008; Boussetta et al., 2009; Barba et al., 2015a; Brianceau et al., 2015), but none of them focused on the extractability of phenolic compounds from white grape pomace.
Furthermore, it should be emphasized that, in order to full exploit the potential benefits and advantages deriving from the application of PEF-assisted extraction over the conventional SLE process, an optimization step is required. To this regard, response surface methodology (RSM) is a statistical tool that, by means of appropriate design and analysis of experiments, enables to gain insight on the effect of the different processing variables and their interactions on target response variables, while minimizing the count of experimental runs. RSM has been successfully applied for developing, improving, and optimizing processes related to food systems (Srikanth et al., 2020; Lal et al., 2021; Pravitha et al., 2021).
Nevertheless, as per literature survey, only in few works RSM was used for the optimization of the main variables (time, liquid to solid ratio, temperature, solvent concentration) involved in the conventional SLE process of bioactive compounds from grape residues (Casazza et al., 2012; Rajha et al., 2013; Melo et al., 2015; Caldas et al., 2018; Kwiatkowski et al., 2020). However, no study has been published yet on the optimization of the innovative extraction process made of a PEF pre-treatment stage followed by a subsequent SLE step.
The main aim of this study was to investigate the potential of PEF pre-treatment to intensify the extractability of bioactive compounds, such as total phenolic compounds and flavonoids, with high antioxidant activity from white grape pomace extracts, based on the optimization of the whole PEF-assisted extraction process. Specifically, RSM was used to optimize PEF processing conditions, evaluating the effect of different combinations of electric field strength (E) and total specific energy input (WT) on the cell disintegration index (Zp) of grape pomace tissues, with the aim to define the minimum treatment severity that maximize Zp to be applied before the subsequent SLE step. The selected solvents for the SLE process were water and ethanol, widely used in grape extraction process due to their biocompatibility and availability in wineries (Brazinha et al., 2014). RSM was also used to optimize the extraction process of the bioactive compounds from grape pomace with regard to extraction time, ethanol concentration, and extraction temperature, during both conventional SLE and PEF-assisted extraction process. Finally, the composition of polyphenols in the obtained extracts was evaluated using HPLC-PDA analyses.
Materials and Methods
Chemicals and Raw Materials
Ethanol, and all reagents and standards involved in the analyses were purchased from Sigma Aldrich (Steinheim, Germany).
Fresh white grape pomace of the “Fiano” variety, mainly composed of skins and seeds, were provided by a local winery (Tenuta Sarno 1860, Avellino, Italy). A picture of the grape pomace is reported in Supplementary Figure 1. The samples were collected during winemaking process, transported to the laboratories of ProdAl Scarl (Fisciano, Italy), and stored under refrigerated conditions (T = 4°C) until use. The moisture content on wet basis of grape pomace, evaluated on arrival at the laboratory, was found to be 69 ± 1.2%.
PEF Apparatus
PEF treatments of white grape pomace, before either impedance analysis or solvent extraction process, were performed using a laboratory-scale batch system previously described elsewhere (Donsì et al., 2010a). Briefly, the system consisted of a treatment chamber made of two parallel plate electrodes of stainless steel separated by a Teflon spacer. The distance between the two electrodes was 2 cm, and their area was 75 cm2. A high voltage cable connected the treatment chamber with a high voltage pulsed power (25 kV−500 A) generator (Modulator PG, ScandiNova, Uppsala, Sweden) able to deliver monopolar square wave pulses with different pulse width (3–25 μs) and frequency (1–450 Hz) through the plant tissue placed between the two electrodes. The actual voltage and current signals at the treatment chamber were measured, respectively, by a high voltage probe (Tektronix, P6015A, Wilsonville, OR, USA) and a Rogowsky coil (2-0.1, Stangenes, Inc., USA), connected to a 300 MHz oscilloscope (Tektronix, TDS 3034B, Wilsonville, OR, USA). The maximum electric field intensity (E, in kV/cm) and the total specific energy input (WT, in kJ/kg of plant tissues) were evaluated according to Carpentieri et al. (2021).
Quantification of PEF-Induced Cell Membrane Permeabilization
The cell disintegration index (Zp) was determined to quantify the degree of cell membrane permeabilization of grape pomace tissue induced by PEF treatment before the subsequent SLE process. The determination of Zp via impedance analyses was carried out according to the method described by Bobinait et al. (2015).
Measurements of electrical complex impedance of untreated and PEF-treated samples were performed by loading 5 g of grape pomace into a measuring cell along with 1 mL of distilled water, to guarantee electrical continuity between the electrodes. The measuring cell consisted of two parallel plate cylindrical electrodes (3 cm in diameter) separated by a polycarbonate tube (1 cm electrode gap). The electrodes were connected to an impedance analyzer (Solartron 1260, UK), which was working in the frequency range of 102-107 Hz. PEF treatments were carried out at a constant pulse width (20 μs) and frequency (5 Hz) and for different combinations of field strength (E = 0.5–5 kV/cm) and energy input (WT = 1–20 kJ/kg), as derived from the experimental design described in Table 1. The initial temperature of the samples was set at 20 ± 1°C and no remarkable temperature increase was observed due to the relatively low energy input delivered during the treatment.
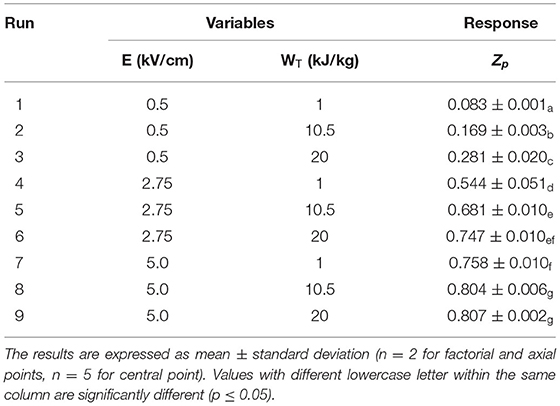
Table 1. Actual values of the two independent variables investigated and response of the dependent variable (Zp) of the PEF treated grape pomace tissues.
For each PEF treatment condition, the Zp value was calculated on the basis of the measurement of the absolute value of the complex impedance |Z|of untreated (|Zuntr|) and treated tissue (|Ztr|) in the low (0.1 kHz) and high (1 MHz) frequency ranges, using the Equation (1) (Donsì et al., 2010b).
The Zp varies between 0 (for intact tissues) and 1 (for fully permeabilized tissue). All the measurements were carried out in triplicate.
The achieved Zp values were used to define optimal treatment conditions in terms of field strength (Eopt) and energy input (WT,opt), which allowed the accomplishment of the highest degree of cell membrane permeabilization with the minimum treatment severity (Bobinait et al., 2015; Frontuto et al., 2019; Pataro et al., 2019; Carpentieri et al., 2021). These optimal conditions were applied during the subsequent PEF-assisted extraction experiments.
PEF-Assisted Extraction Experiments
For PEF-assisted extraction experiments, ~5 g (on average) of grape pomace were loaded into the treatment chamber and PEF pre-treated under the optimal conditions (Eopt, WT,opt) previously defined through the Zp determinations. After the electro-permeabilization treatment, the samples were immediately subjected to solvent extraction process by placing them into 100 mL Pyrex flasks where a water-ethanol mixture was added at a constant solid to liquid ratio (1:10 g/mL). The flasks were then introduced in an orbital incubator S150 (PBI international, Milan, Italy) where the extraction process was carried out under constant shaking at 160 rpm for different times (0–165 min), temperatures (20–50°C), and ethanol concentration (0–100%), as derived from the experimental design (Table 3). For the sake of comparison, the same experimental design and extraction protocol was used for untreated (control) grape pomace subjected to conventional SLE process.
The extracts achieved from untreated, and PEF treated samples were then centrifuged at 5289 x g (PK130R model, ALC International, Cologno Monzese, IT) for 10 min at 4°C to separate the supernatants. The final extract was then stored at 4°C until further analysis.
Experimental Design
Response surface methodology was used to establish the relationship between response variables and process variables, to determine the optimal conditions of PEF pre-treatment (Eopt in kV/cm, WT,opt in kJ/kg), which maximize the Zp value, as well as the optimal conditions of the subsequent SLE process that maximize the extraction yield of total phenolic compounds, flavonoids, and antioxidant activity of grape pomace extracts from untreated (control) and PEF-treated samples.
A three-factors face-centered central composite design (FC-CCD) with six center points was used to investigate the effects of the electric field strength (X1, 0.5–5 kV/cm) and total specific energy input (X2, 1–20 kJ/kg) on the permeabilization degree of grape pomace tissues induced by PEF. Cell permeabilization index (Y1) of PEF-treated samples was used as response variable. The experimental design consisted of 9 runs (Table 1). A second-order polynomial model reported in Equation (2) was used to predict the response variable as a function of the investigated independent factors:
where Yk is the predicted response variable; Xi and Xj are the independent variables; β0, βi, βii, and βij are the intercept, regression coefficients of the linear, quadratic, and interaction terms of the model, respectively.
The same FC-CCD was also used to evaluate the effect of ethanol percentage (X3, 0–100%, v/v) in a water-ethanol solvent mixture, extraction time (X4, 30–165 min), and extraction temperature (X5, 20–50°C). Total phenolic content (Y2), flavonoid content (Y3), and antioxidant activity (Y4), of untreated and PEF-treated samples were used as response variables. The experimental design consisted of 15 runs of extraction conditions including five replicates of central points (Table 3). A third-order polynomial model reported in Equation (3) was used to predict the response variables as a function of the investigated independent factors:
where Yk is the predicted response variables; Xi and Xj are the independent variables; α0, αi, αii, αij, αiij, and αijj are the intercept, regression coefficients of the linear, quadratic, and interaction terms of the model, respectively.
Analysis of the Extracts
Determination of Total Phenolic Content
The total phenolic content (TPC) of grape pomace extracts was determined using the Folin-Ciocalteau method as previously described by Bobinait et al. (2015), with some modifications. Briefly, 1 mL of extract, which was diluted when required, was mixed with 5 mL of 10% (v/v) Folin-Ciocalteau reagent and then added with 4 mL of sodium carbonate (7.5%, w/v). The absorbance of the reacting mixture was measured after incubation for 60 min at room temperature in a dark place at 765 nm using a UV/Vis spectrophotometer (V-650, Jasco Inc. Easton, MD, USA). Gallic acid dissolved in ethanol/water mixtures of different concentrations (0%, 50%, 100%, v/v) was used to generate five-point external standard calibration curve in a concentration range comprised between 1 and 100 mg/L. The concentration of TPC were expressed as milligrams of gallic acid equivalents (GAE) per g of dry weight (gDM) grape pomace.
Determination of Flavonoid Content
Aluminum-chloride colorimetric assay was used to determine the flavonoid content (FC) of grape pomace extracts as previously reported by Agbo et al. (2015). Briefly, 1 mL of extract was mixed with 4 mL of distilled water in a 10 mL plastic tube and then added with 0.3 mL of 5% (w/v) sodium nitrite. After 5 min in a dark place, 0.3 mL of 10% (w/v) AlCl3·6H2O solution was added to the mixture, followed by the addition of 2 mL of 1.0 M NaOH after another 5 min of storage in a dark place. Then the mixture was diluted to the mark with distilled water. A set of standard solutions of quercetin (20, 40, 60, 80, 100 μg/mL) in ethanol/water mixtures of different concentrations (0%, 50%, 100%, v/v), were prepared and used as standard for the calibration curves. The absorbance of the samples and standard solutions were measured against the reagent blank at 510 nm with the UV/Vis spectrophotometer. The FC was determined from the calibration curve and expressed as mg of quercetin equivalent (QE) per gDM of grape pomace.
Evaluation of Ferric Reducing Antioxidant Power
FRAP assay of grape pomace extracts from both untreated and PEF treated samples was performed according to the method described by Benzie and Strain (1996) with slight modifications. Briefly, 2.5 mL of freshly prepared FRAP working solution and 0.5 mL of diluted extract (0.25 mL extract + 0.25 mL ethanol-water solution) were mixed and incubated for 10 min at ambient temperature. The absorbance of the reacting mixture was then measured at 593 nm with the UV/Vis spectrophotometer. Ascorbic acid dissolved in ethanol/water mixtures of different concentrations (0%, 50%, 100%, v/v) was used to generate five-point external standard calibration curve in a concentration range comprised between 0 and 2 mmol/L. The FRAP values were expressed as mg of ascorbic acid equivalents (mg AAE) per gDM of grape pomace.
HPLC-PDA Analyses of the Extracts
The High-Performance Liquid Chromatography - Photodiode Array Detection (HPLC-PDA) analyses of the grape pomace extracts obtained at the optimal extraction conditions were performed using a Waters 1525 Separation Module equipped with a photodiode array detector Water 2996 (Waters Corporation, USA), via the methodology described by Frontuto et al. (2019), with some modifications. Analytical separation of the analyzed compounds was carried out in a Waters Spherisorb C18 reverse phase column (5 μm ODS2, 4.6 × 250 mm, Water Corporation, USA). Prior to HPLC analysis, the extracts were filtered with 0.20 μm filters and then diluted with the ethanol/water solution [50% (v/v)]. The mobile phase consisted of (A) phosphoric acid in water (0.1 %, v/v), and (B) methanol. For compounds separation, the following gradient was used: 0–30 min from 5% B to 80% B, 30–33 min 80% B, 33–35 min from 80% B to 5% B. The injection volume and the flow rate of the mobile phase were 5 μL and 0.8 mL/min, respectively. The signal for each identified compound was recorded at its wavelength of maximum absorbance. In particular, wavelengths of λ = 280, 310, and 360 nm were used for the absorbance detector.
Phenolic compounds, in the extracts were identified according to the HPLC-PDA retention times (RT) and UV absorbance maximum, as compared to commercial standards, namely epicatechin, p-coumaric acid, and quercetin.
All commercial standards were dissolved into the extraction solvent to generate 6 points standard calibration curves (R2 = 0.999). The results were expressed as mg of the target compound/gDM of grape pomace.
Statistical Analysis
All the experiments and analyses of the obtained extracts were performed in triplicate and the results reported as means ± standard deviations. Differences among mean values were analyzed by one-way variance (ANOVA) using SPSS 20 (SPSS IBM, Chicago, USA) statistical package. Tukey test was carried out to determine statistically significant differences (p < 0.05). The software package Design Expert Version 12 (Minneapolis, MN) was used to define the experimental design (FC-CCD), as well as to perform the analysis of the data including the generated 3D response surfaces, and the determination of the optimal combination of process parameters that maximize either Zp or the extractability of the compounds of interest. Five replicates of the optimal conditions were performed to validate the models. The Pearson product-moment correlation coefficient (r) was used to evaluate the strength of the linear correlation between response variables.
Results and Discussion
Effect of PEF Treatment on the Cell Membrane Permeabilization of Grape Pomace Tissues
Measurements of the changes in the electrophysical properties, such as complex electrical impedance of untreated and PEF treated biological materials, have been suggested as a simple and reliable method to obtaining a measurement of the extent of damaged cells (Donsì et al., 2010b; Pataro et al., 2011). Results depicted in Supplementary Figure 2, reported the absolute value of the complex electrical impedance of intact and PEF-treated grape pomace tissues as a function of the electrical frequency and for different combination of field strength E (0.5–5 kV/cm) and total specific energy input WT (1–20 kJ/kg).
In this work, data of Supplementary Figure 1 were used to assess the cell disintegration index (Zp), which has been successfully employed as a reliable macroscopic indicator of the cell membrane permeabilization degree induced upon the application of PEF treatment in diverse fruits and vegetables tissues (Battipaglia et al., 2009; Donsì et al., 2010a; Puértolas et al., 2013; Luengo et al., 2014; Bobinait et al., 2015; Frontuto et al., 2019; Pataro et al., 2019), including grape processing by-products (Boussetta et al., 2009; Barba et al., 2015a; Brianceau et al., 2015).
According to the constructed experimental FC-CCD, Table 1 shows the effect of the independent variables, namely electric field strength and energy inputs, on the Zp of PEF-treated grape pomace tissue. Results show that, increasing the electric field strength and energy input the Zp increased up to 0.80, which was the highest value recorded when applying the most intense treatment conditions (5 kV/cm and 10–20 kJ/kg). However, it can be observed that the permeabilization degree shows a great dependence on the electric field strength, whereas the effect of energy input appears more pronounced when applying lower field strengths. Specifically, results show that at any energy input investigated, the Zp values exhibited a statistically significant increase (p ≤ 0.05) when the field strength was increased within the investigated range (0.5–5 kV/cm). At fixed field strength applied, the Zp value also increased when the energy input was increasing. However, statistically significant differences (p ≤ 0.05) were detected only when the lowest field strength was applied, while at field strength of 3 and 5 kV/cm significant differences (p ≤ 0.05) were observed only when the energy input was changed from 1 to 10.5 kJ/kg. Further increments of WT above this value scarcely affected Zp, which tended to level off to a constant value.
The increment of Zp values with increasing intensity of PEF treatment observed in this research is in good agreement with findings previously reported by other scientists on different plant matrices, such as orange peels (Luengo et al., 2013), tomato peels (Luengo et al., 2014; Pataro et al., 2020), potato peels (Frontuto et al., 2019), oregano and wild thyme tissues (Carpentieri et al., 2021), including grape processing by-products (Boussetta et al., 2009; Brianceau et al., 2015). As an example, in the study of Boussetta et al. (2009), it was observed that the permeabilization index of PEF-treated grape skin derived from winemaking processing of white grapes (Vitis Vinifera L. cv. “Chardonnay”), grew under the PEF treatment and reached a saturation level already at the electric field strength of 1.3 kV/cm and at long treatment times >1 s.
Model Fitting, RSM Analysis and Optimization of PEF Treatment Conditions
The data obtained from the FC-CCD were fitted to a second-order polynomial equation (p ≤ 0.05). The values and significance of the regression coefficients of the predicted polynomial models and corresponding p values for each variable are reported in Table 2.
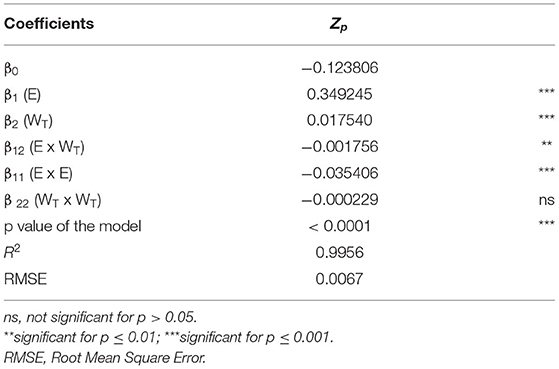
Table 2. Analysis of variance (ANOVA) of the second polynomial model for the cell disintegration index (Zp) of PEF treated grape pomace tissues.
Even though the two investigated factors showed a statistically significant effect on Zp (p ≤ 0.05), the electric field strength was the factor that mostly affected the observed response, resulting in a significant linear and quadratic effect on Zp, as compared to the non-significant (p > 0.05) quadratic term of the energy input. Results also show that, the interaction between single factors was significant (p ≤ 0.01), suggesting that the combination of both field strength and energy input amplified the permeabilization effect of PEF. Moreover, the significance negative value of the squared factors (β11) indicates the existence of optimum value of field strength maximizing the response variable.
The coefficients of the equation used for the fitting and the statistics used to test the adequacy of the model, are demonstrated to be well fitted by the second order polynomial equation expressing the relationship between the experimental parameters and the response variable (Zp). The p-value of the model is <0.05, which indicates that the model is significant, and therefore the independent variables of the model have a significant effect on the responses. The model has a satisfactory level of adequacy (R2= 0.9956), indicating a good agreement between the experimental data and the predicted values.
Response surface plot reported in Figure 1 shows the interactions between field strength and energy input on the Zp of PEF pre-treated grape pomace tissues. In the whole investigated domain, the increase in the PEF treatment intensity induced an increase in the extent of cellular damages of the membrane permeabilization of grape pomace tissues. More specifically, results clearly show that Zp gradually increased with increasing the energy input, even though the field strength was the factor that mostly influenced the observed response.
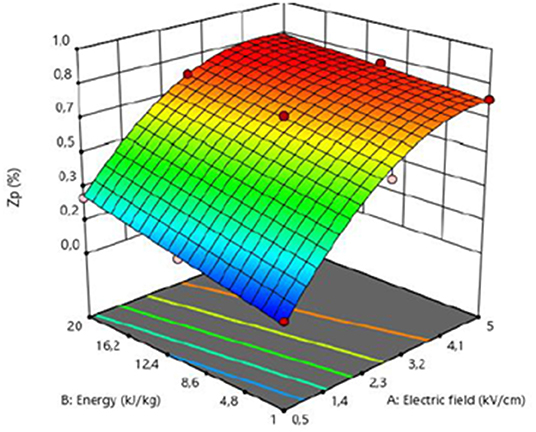
Figure 1. Response surface for the permeabilization index (Zp) of PEF treated grape pomace tissues as a function of electric field strength (kV/cm) and the energy input (kJ/kg).
From these results, optimal PEF treatment conditions, with regard to the minimal electric field strength (Eopt, kV/cm) and total specific energy input (WT,opt, kJ/kg) that enabled the achievement of the highest degree of cell membrane permeabilization, were defined. In particular, the maximum Zp value (0.75) was detected for the PEF-treated samples at 3.8 kV/cm and 10 kJ/kg. Moreover, it can be concluded that PEF pre-treatment was able to efficiently induce the cell membrane permeabilization of white grape pomace tissues, at an extent mostly dependent on the electric field strength applied.
According to these results, further investigations of PEF pre-treatment on the extractability of bioactive compounds from grape pomace were performed under the defined optimal conditions (3.8 kV/cm−10 kJ/kg).
Effect of PEF-Assisted Extraction on Total Phenolic Content, Flavonoid Content, and Antioxidant Activity of the Extracts
Model Fitting
In this work, the experimental FC-CCD was created to evaluate the effect of three factors, namely extraction time, extraction temperature and ethanol concentration, on total phenolic content (TPC), flavonoid content (FC), and antioxidant activity (FRAP) of white grape pomace extracts, obtained from either conventional SLE or PEF-assisted extraction process (Table 3).
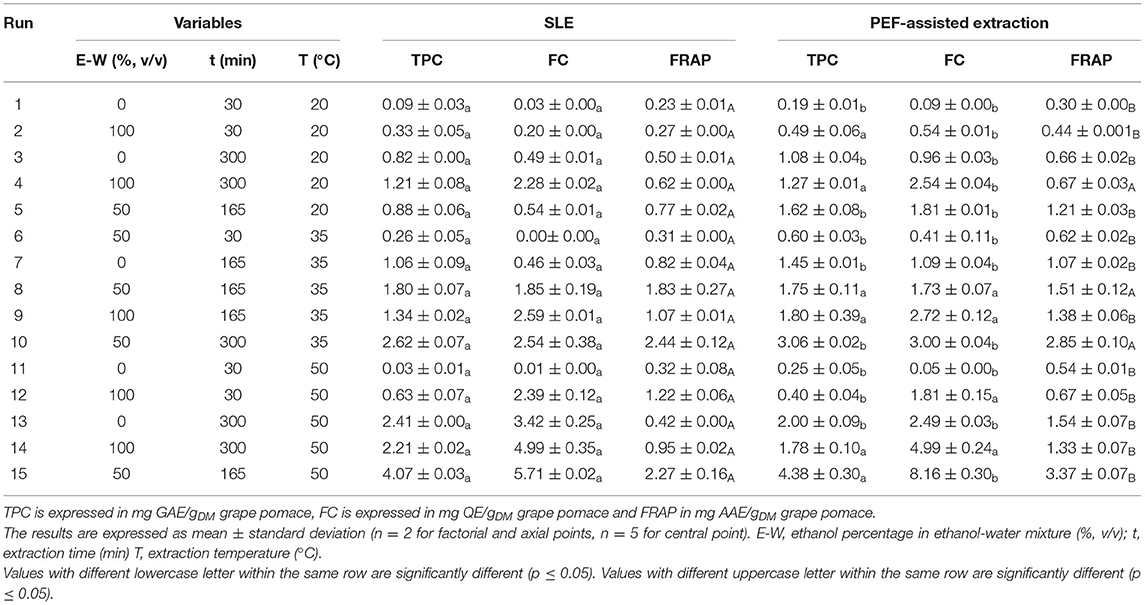
Table 3. Actual values of the three independent variables investigated and responses of the dependent variable (TPC, FC, and FRAP) in grape pomace extracts from either conventional SLE or PEF (3.9 kV/cm, 10 kJ/kg)-assisted extraction process.
In general, results demonstrate that all the investigated variables greatly influenced the concentrations of TPC, FC, and FRAP values. In particular, regardless the application of PEF pre-treatment, the highest levels of response variables were achieved at high extraction time and medium values of ethanol concentration within the investigated range, and especially at higher temperature. This is in agreement with previous research (Melo et al., 2015), suggesting that an increase in the extraction temperature resulted in an increase in the studied responses. Moreover, results also show that the application of PEF pre-treatment to white grape pomace, at the previously defined optimal conditions (3.8 kV/cm−10 kJ/kg), markedly enhanced the extraction of TPC (8%) and FC (31%), while leading to higher antioxidant activity (36%) of the extracts, as compared with the control extraction, with significant differences detected especially when water or ethanol-water mixtures were used as a solvent.
The data obtained from the FC-CCD for all the response variables were fitted to a reduced third-order polynomial equation (Equation 3). Since from the statistical analyses some of the terms of the equation resulted not significant, these terms were not taken into consideration for the general evaluation of the model, thus reducing the general complexity of the mathematical relationship between independent and response variables. The values and significance of the regression coefficients of the predicted polynomial models and corresponding p-values, the determination coefficient (R2) for each variable, and the Root Mean Square Error (RMSE) are reported in Table 4.
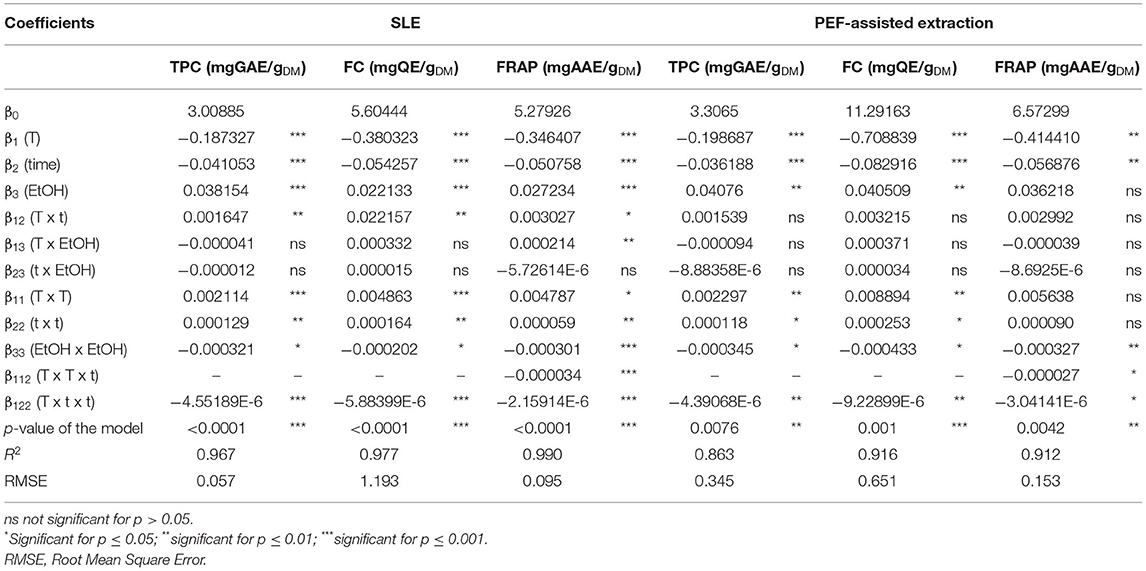
Table 4. Analysis of variance (ANOVA) of the third polynomial models for the TPC, FC, and antioxidant activity (FRAP) in grape pomace extracts from either conventional SLE or PEF (3.9 kV/cm, 10 kJ/kg)-assisted extraction process.
Results show that, for the extracts of either untreated or PEF-treated samples, the significance of the different factors on the investigated response variables (TPC, FC, and FRAP) appeared similar, with only little exceptions. In particular, all the investigated factors resulted in a statistically significant linear and quadratic effect on most of the response variables. However, differently than untreated samples, a non-significant effect was detected for the linear term of ethanol concentration, and for the quadratic terms of temperature and time in the case of FRAP values of PEF treated samples. Moreover, it is worth noting that, regardless the response variables, the negative quadratic term of the ethanol concentration leads to a parabolic trend, which indicates the existence of a maximum response value.
On the other hand, regardless the investigated responses, all the interactions between single factors were not significant in the case of PEF-treated samples, while only the dependency of the extraction temperature on the diffusion time was detected in the case of untreated samples. This suggests that, in the investigated variables domain, PEF pre-treatment reduced the influence of extraction temperature on diffusion time, in comparison with the untreated samples. Similar results were found by Frontuto et al. (2019) when analyzing the interaction between the extraction temperature and time on the TPC in PEF-treated potato peel extracts.
The ANOVA results (Table 4) show that the RSME values were comprised in the range between 0.007 and 1.19, and that relationship between response variables and the extraction parameters had determination coefficient (R2) values ranged between 0.863 and 0.990, thus denoting a good correlation between observed and predicted data. Additionally, analysis of variance indicated that the model used was significant (p ≤ 0.01) for all the responses supporting the predictive efficacy of the selected model.
RSM Analysis and Optimization of the Extraction Process
Response surface graphs reported in Figures 2, 3 for both extracts from untreated and PEF-treated samples depict the complex interactions among extraction temperature (20–50°C), extraction time (30–300 min), and ethanol concentration (0–100%, v/v) on the level of TPC and FC of the obtained extracts.
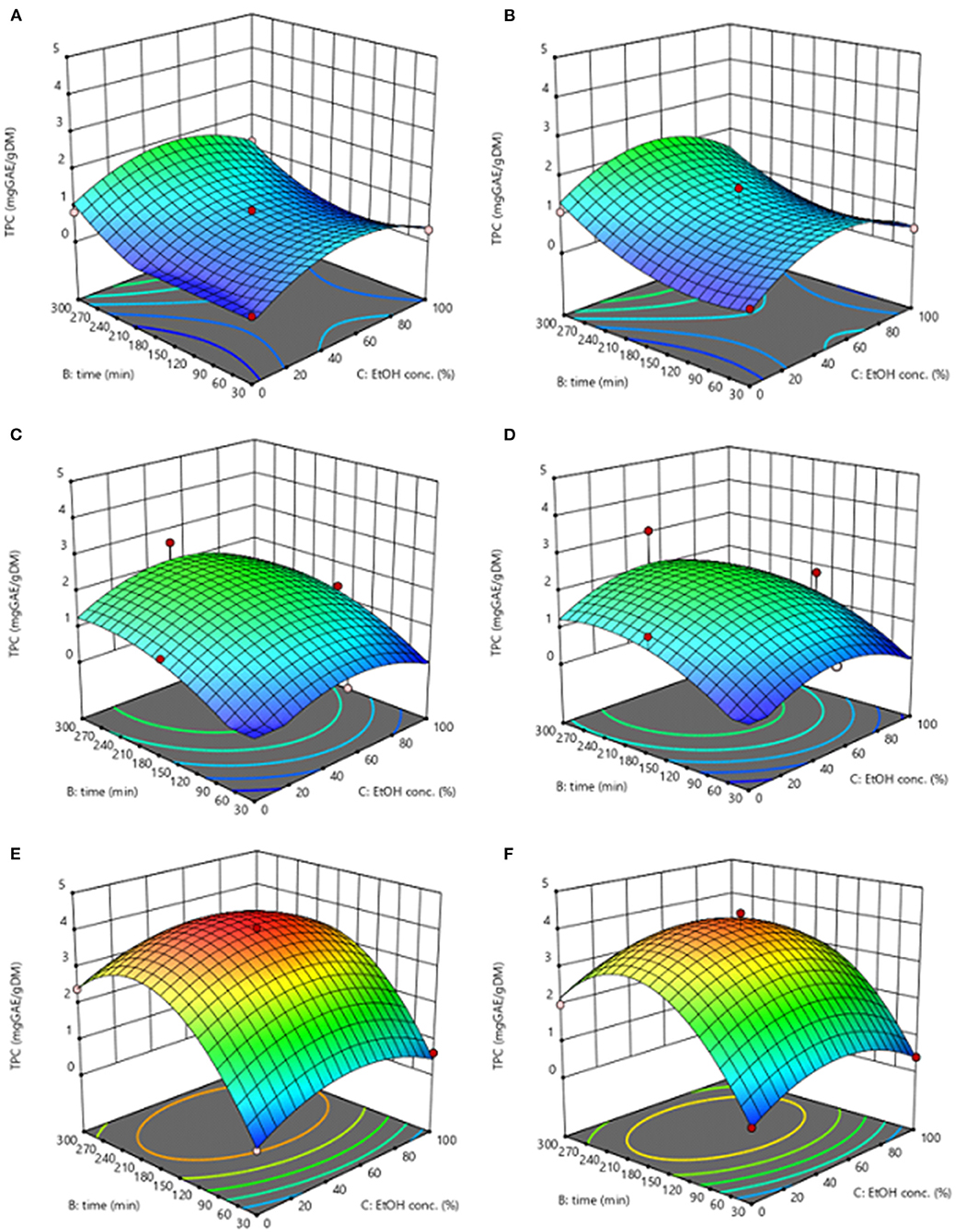
Figure 2. Response surfaces for TPC of extracts obtained from untreated (Control) (A,C,E) and PEF-treated (E = 3.8 kV/cm; WT = 10 kJ/kg) (B,D,F) grape pomace as a function of extraction time and ethanol concentration, with the extraction temperature set at 20°C (A,B), 35°C (C,D), 50°C (E,F) as extraction temperature.
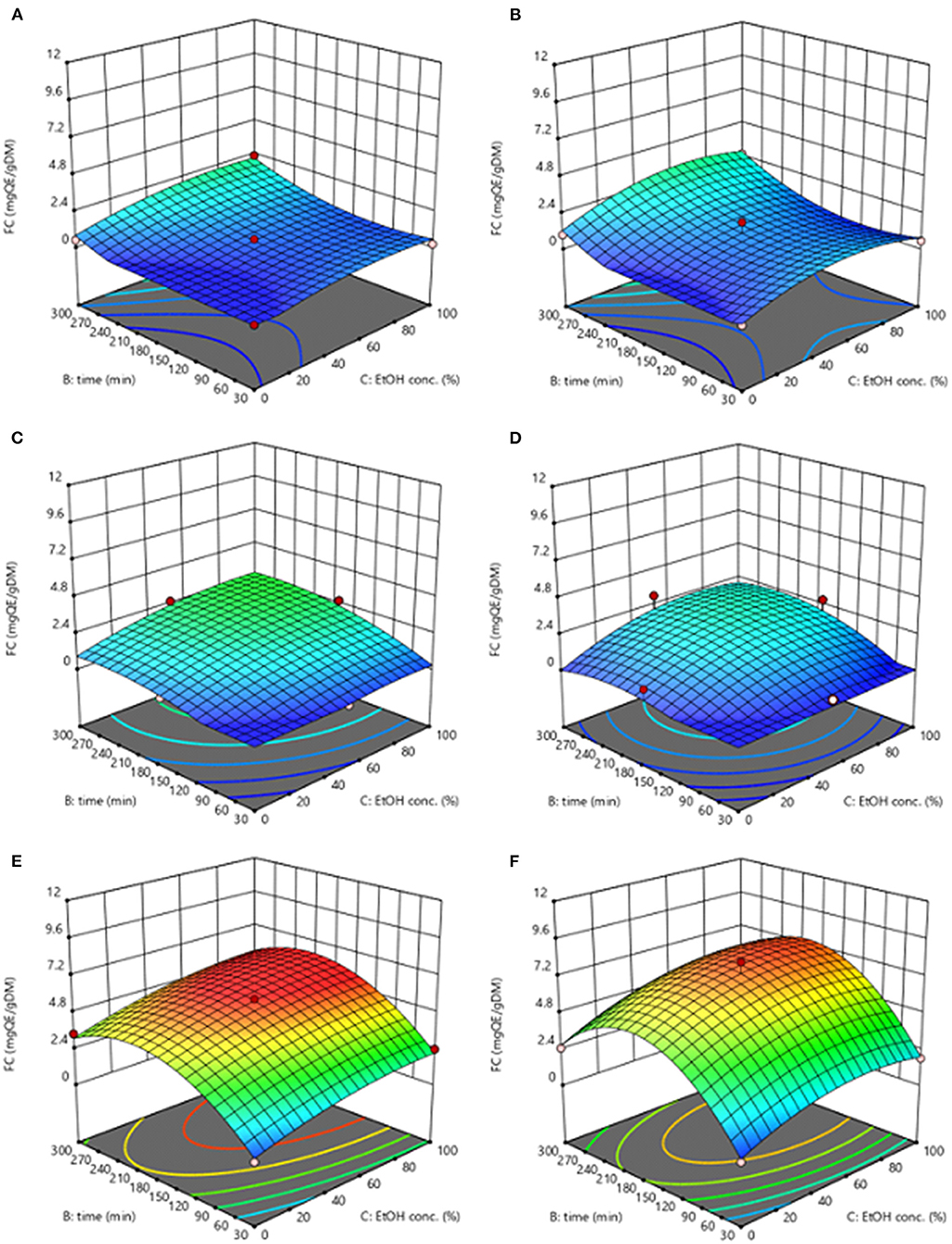
Figure 3. Response surfaces for total flavonoids of extracts obtained from untreated (Control) (A,C,E) and PEF-treated (E = 3.8 kV/cm; WT = 10 kJ/kg) (B,D,F) grape pomace as a function of extraction time and ethanol concentration, with the extraction temperature set at 20°C (A,B), 35°C (C,D), 50°C (E,F).
In the investigated variables domain, PEF treatment increased the concentration of the phenolic content in the extracts as compared with the untreated samples. Although all the investigated variables had a statistically significant (p ≤ 0.05) effect on TPC and FC, the extraction temperature appeared as the factor that mostly affected the response variables. The higher the extraction temperature, the greater the recovery yield of phenolic compounds. This is also corroborated by the higher value of the extraction temperature linear coefficient than those of ethanol concentration and diffusion time. The positive effect of the extraction temperature on the extraction efficiency of phenolic compounds from grape by-products was previously observed by other researchers when studying the extractability of phenolic compounds from different winery by-products (Spigno et al., 2007; Rajha et al., 2013; Melo et al., 2015). This behavior could be explained considering that the higher temperature ameliorates the mass transfer, by enhancing solubility and diffusivity of intracellular compounds in the solvent and reducing the surface tension and viscosity (Rajha et al., 2013; Melo et al., 2015). Moreover, mild heating of plant tissues may also contributes to make the lipid bilayer of the cell membrane more sensitive to breakage under the PEF treatment (Pataro et al., 2018), thus further intensifying the extractability of intracellular compounds. On the other hand, subjecting grape pomace to high temperatures above 50°C, might lead to the release of certain phenolic compounds while simultaneously triggering possible thermal oxidation or degradation of others (Spigno et al., 2007; Rajha et al., 2013).
In agreement with previous findings (Rajha et al., 2013), the TPC and FC values reached its peak at 50°C, which is the highest temperature tested in this work.
Additionally, regardless the application of the PEF pre-treatment, results of Figure 2 revealed that, at constant temperature, both extraction time and ethanol concentration significantly influenced the TPC and FC, showing a quadratic effect on the extraction yield, which was more evident only at processing temperature higher than 20°C.
In particular, regarding the extraction time, results revealed that, at room temperature, TPC and FC slightly increased with increasing the diffusion time within the investigated range. This is partially in contrasts with results found by Casazza et al. (2012), who found that the response surface for total phenolic yield showed a parabolic trend already at 25°C, with a maximum TPC in ethanol extracts achieved after 19 h.
However, at higher temperature, a prolonged extraction time resulted in a decrease of TPC value, with the maximum reached at an intermediate extraction time (190–223 min) depending on extraction method and response variable (Figure 2). This is somehow consistent with the findings previously reported by other scientists. For example, when Rajha et al. (2013) compared the water extraction yield of phenolic compounds form Cabernet Sauvignon grape by-products in a range of temperature between 33 and 50°C, they found that diffusion time had a quadratic significant negative effect on TPC, showing a maximum TPC values at 30 h. This is because long extraction time could lead to a reversed effect on the phenolic compounds likely due to oxidation or degradation reactions triggered by oxygen present in the head space of test flasks, which would be accelerated at higher temperature (Rajha et al., 2013).
On the other hand, ethanol concentration, with its parabolic trend, significantly influenced the observed response (Figure 2). This behavior could be ascribed to the effect of the ethanol concentration on the polarity of the solvent mixture, thus enabling the extractability of phenolic compounds with a wide range of polarities (Frontuto et al., 2019). Additionally, it is known that ethanol may affect the barrier properties of the cell envelop of the plant tissues by acting on the phospholipid bilayer of biological membranes (Puértolas et al., 2013), thus facilitating the capacity of penetration or diffusion of the solvent and the subsequent release of solubilized intracellular compounds.
The optimal conditions for maximizing the TPC were shown by the adopted model to be 50°C, 53% ethanol-water mixture, and 213 min of extraction time for control samples (4.07 mgGAE/gDM), and 50°C, 50% ethanol-water mixture, and 190 min extraction time for PEF-treated samples (4.38 mgGAE/gDM, + 8% over SLE). Similarly, the highest FC value was achieved at 50°C, 88% ethanol, and 223 min for control samples (5.98 mg AA/gDW, +31% over SLE).
Although any comparison of the extraction efficiency of valuable compounds from different plant matrices is very difficult being currently based on literature data achieved using different types of plant materials, equipment and experimental protocols, our results appear consistent with those achieved by other scientists. In particular, the optimal extraction temperature determined in our study (50°C) was the same detected by Rajha et al. (2013) during the SLE of phenolic compounds from grape by-products. Similarly, the optimal concentration of ethanol (53%) found in the present work was only slightly higher than that (50%) detected in work carried out by Caldas et al. (2018) upon SLE of polyphenols from red grape skins. Moreover, optimal flavonoid concentration achieved in this work was greatly in agreement with the FC of ethanol extracts from grape pomace found by other scientists (Zhang and Zhu, 2015; Guaita and Bosso, 2019).
Interestingly, the application of PEF prior to SLE can be successfully used not only to increase the amount of phenolic compounds in the extract (by 8%), but also to lower both duration of the extraction process (by 23–103 min) and the ethanol concentration (by 3–12%) to achieve the desired extraction yield. These results could be explained by the electroporation effect induced by PEF pre-treatment. According with data reported in Table 1, in fact, PEF pre-treatment is able to induce the permeabilization of cell membrane of the grape pomace tissues (Zp = 0.75), leading to a remarkable increase of the capacity of penetration of the solvent into the solid matrix, thus intensifying the recovery of phenolic compounds. On the other hand, it is worth noting that the capability of temperature and ethanol to further enhancing solubility and the capacity of penetration of the solvent into the plant tissues, as well as their interaction with the electrical parameters, may have acted in improving the extractability of phenolic compounds.
It is also worth noting that the value of the quadratic term of the ethanol concentration (−0.000202) for FC response model of untreated samples was approximately half the value determined for PEF-treated samples (−0.000433), which denotes a less pronounced negative effect of ethanol concentration on the FC yield upon SLE process. Such behavior is of particular interest, since phenolic compounds belonging to the TF group were found to have high antioxidant activity (Van de Wiel et al., 2001).
The response surface of antioxidant activity (FRAP) of extracts from untreated and PEF-treated grape pomace, is shown in Figure 4. In agreement with previous findings (Caldas et al., 2018), the behavior of the antioxidant activity within the region under investigation of the independent variables, appeared very similar to that achieved for TPC and FC. This similarity indicates, as expected, that phenolic compounds present in the grape pomace extract are probably the main responsible for its antioxidant capacity.
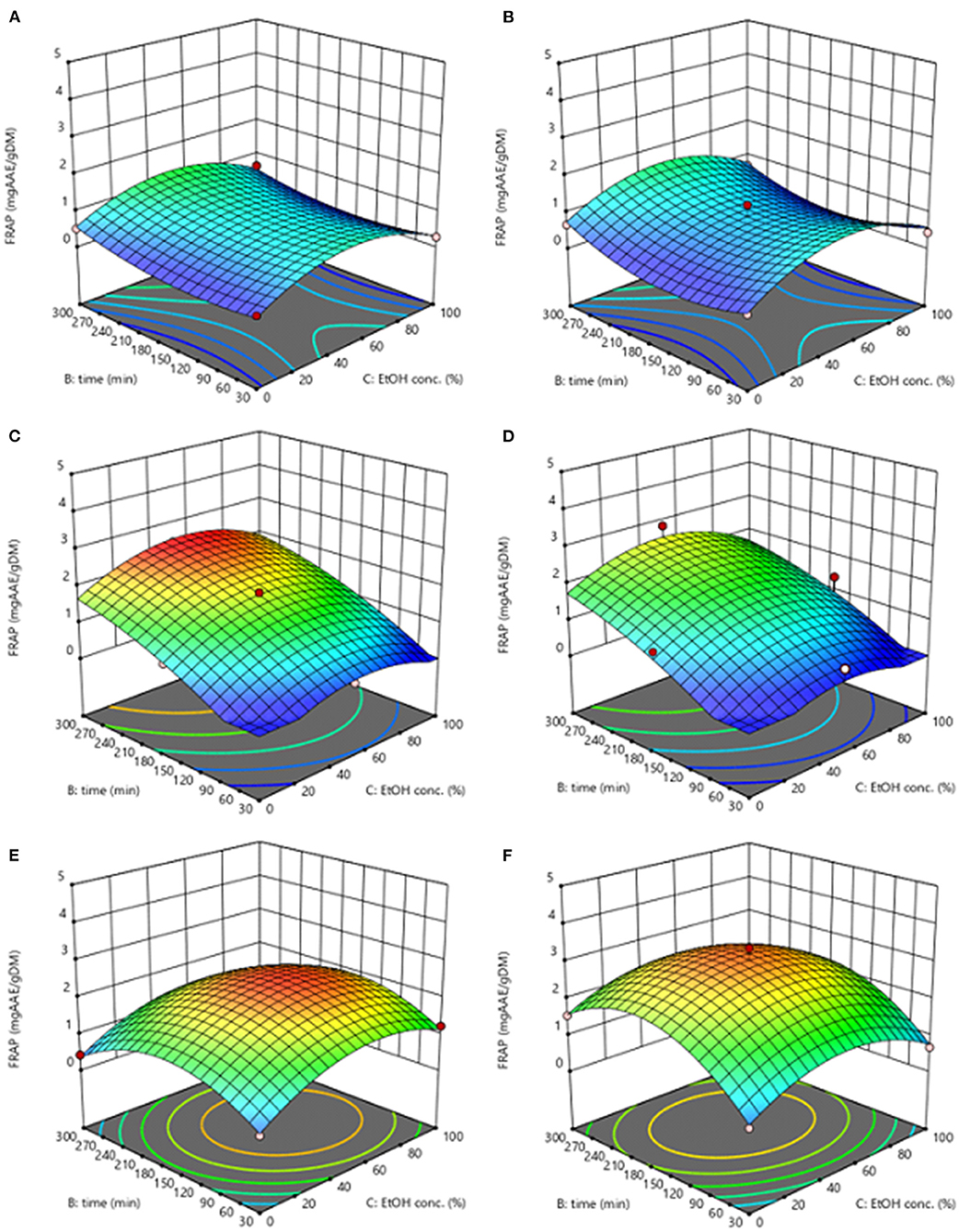
Figure 4. Response surfaces for antioxidant activity (FRAP) of extracts obtained from untreated (Control) (A,C,E) and PEF-treated (E = 3.8 kV/cm; WT = 10 kJ/kg) (B,D,F) grape pomace as a function of extraction time and ethanol concentration, with the extraction temperature set at 20°C (A,B), 35°C (C,D), 50°C (E,F).
In particular, from the results illustrated in Figure 4, it clearly appears that the extracts derived from the PEF-treated grape pomace possessed a higher antioxidant activity than that detected in the control extracts, especially at the selected optimal extraction temperature (T = 50°C), which was likely due to the high amount of phenolic compounds recovered upon the application of PEF pre-treatment. Moreover, the quadratic term of ethanol concentration possessed a high effect on the antioxidant activity of the extracts that increased as increasing the ethanol concentration until reaching a maximum value at intermediate ethanol concentration within the investigated range and then decreased, thus reflecting the trend observed for TPC and FC.
This appears somehow consistent with the finding of Melo et al. (2015), who observed that the highest levels of antioxidant capacity in extracts obtained from different varieties of grape pomace were obtained at moderate ethanol concentrations (40–60%, v/v) and higher temperatures.
Furthermore, in agreement with previous findings (Casazza et al., 2012), a strong positive correlation was found between the TPC and FC and antioxidant activity, with a Pearson correlation coefficient in the range 0.840–0.979 for TPC and 0.723–0.846 for FC, indicating that the global antioxidant activity of the grape pomace extracts is mostly associated to the phenolic compounds.
The values of the factors that maximize antioxidant activity were 50°C, 62% ethanol-water mixture, 293 min for control extracts, and 50°C, 50% ethanol-water mixture, 190 min for PEF extracts, which were similar to those identified for phenolic compounds, resulting in an antioxidant activity of 2.58 mgAAE/gDM and 3.38 mgAAE/gDM, respectively.
Therefore, likewise TPC and FC, the application of PEF prior to SLE can be successfully used not only to increase the antioxidant power of the extract but also to reduce both duration and temperature of the extraction process, as well as the ethanol concentration to achieve the desired FRAP value.
Based on the results achieved so far, for further analysis the optimal extraction conditions 50°C, 50% ethanol-water mixture, 190 min extraction time, were selected, being the minimal PEF-assisted extraction conditions enabling the enhancement of the release of all the detected target compounds with the minimum use of ethanol and extraction time.
Quantification of the Main Phenolic Compounds via HPLC-PDA Analysis
The composition of the extracts obtained from untreated and PEF-treated white grape pomace at the previously optimized PEF-assisted extraction conditions (3.8 kV/cm−10 kJ/kg; 50°C−50% ethanol concentration-190 min), was assessed via HPLC-PDA analysis. The resulting chromatogram profiles are presented in Figure 5.
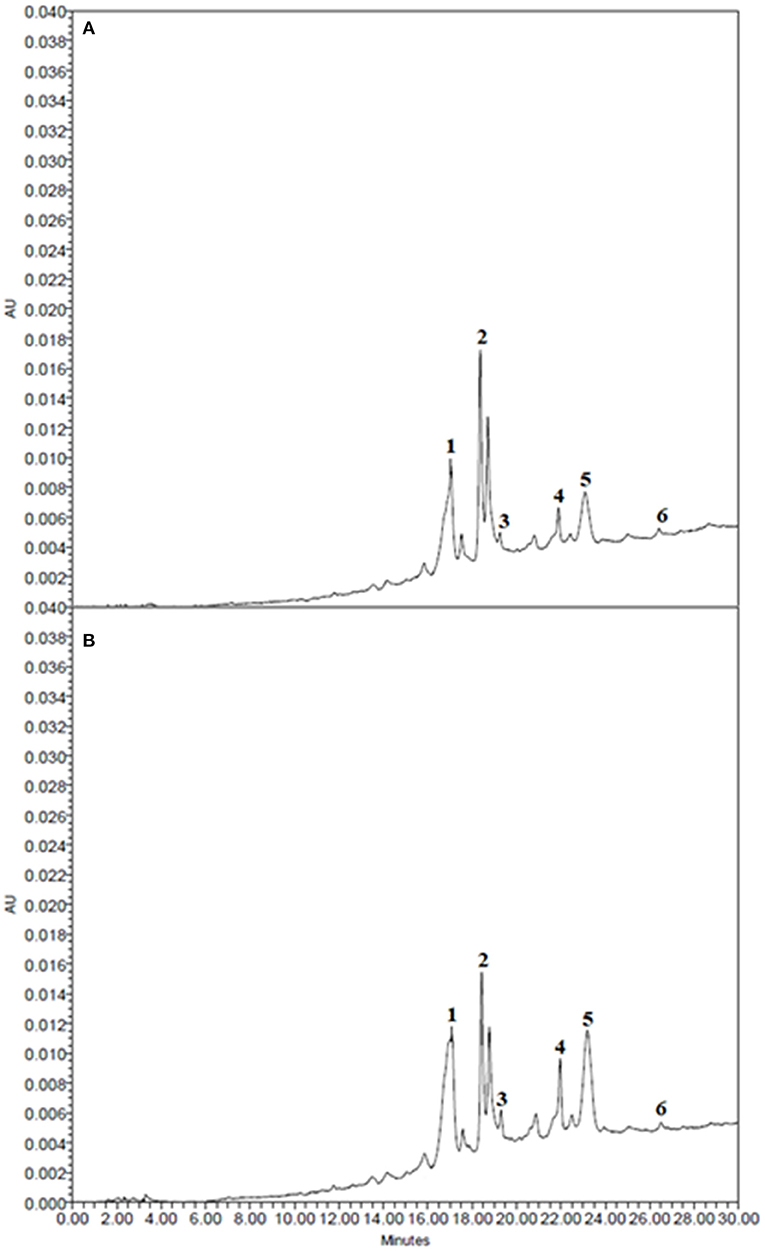
Figure 5. HPLC-PDA chromatograms of 50% (v/v) ethanol-water extracts obtained after 190 min of extraction at 50°C from (A) untreated (Control) and (B) PEF-treated (Eopt =3.8 kV/cm; WT,opt = 10 kJ/kg) white grape pomace. Peak identification: epicatechin (1); unidentified compound (2); p-coumaric acid (3); unidentified compounds (4), and (5); quercetin (6).
Results show that, regardless the application of a PEF pre-treatment, one of the major phenolic compounds corresponding to epicatechin (peak 1), followed by p-coumaric acid (peak 3), and quercetin (peak 6) were detected at an elution time of 17.06, 19.25, and 26.5 min, respectively. Additionally, at least three other unidentified compounds were also detected after the elution time of epicatechin. The latter is known to possess strong antioxidant effects and is present in grapes and wine at the highest levels of all flavonoids (Mikeš et al., 2008).
It is worth noting that, the extracts obtained from untreated, and PEF pre-treated grape pomace showed similar phenolic profiles (Figure 5), confirming that, under the mild PEF treatment conditions applied no degradation/modification of individual phenolic compounds occurred. This is consistent with the observation reported by other scientists for the extraction of phenolic compounds from different plant matrices (López et al., 2009; Luengo et al., 2013; Bobinait et al., 2015; Pataro et al., 2017; Frontuto et al., 2019).
Moreover, HPLC-PDA analysis showed that the concentrations of epicatechin, p-coumaric acid and quercetin detected in ethanol-water extracts of untreated samples were 1.11 ± 0.06, 0.035 ± 0.01, and 0.061 ± 0.001 mg/gDW, respectively. The application of the PEF pre-treatment markedly increased the recovery yield of these compounds leading to a final concentration in the extracts of 1.79 ± 0.2, 0.075 ± 0.01, and 0.064 ± 0.001 mg/gDW, respectively. It is worth nothing that the concentration of epicatechin in ethanolic extracts detected in this work were consistent with those found by other authors, with slight differences depending on the variety, geographical location, viniculture technologies or stress factors occurring during growing and ripening of the grapes, as well as on the mode of vinification (Yilmaz and Toledo, 2004; Rockenbach et al., 2011). As an example, Rockenbach et al. (2011) found that catechins were the most abundant non-anthocyanin compounds identified in the grape pomace (1.5 mg/gDW) for all the varieties investigated, with the epicatechin being the most abundant catechin (0.96 mg/gDW).
Results reported in Figure 5 are also consistent somehow with the observations reported Boussetta et al. (2009) when carried out the identification of phenolic compounds via HPLC analyses in extracts achieved from PEF-treated white grape skins. The authors, in fact, detected the presence of epicatechin and quercetin, even though no significant differences were detected compared to the extracts from untreated white grape skins.
Furthermore, these results seem to confirm that the permeabilization effect of cell membrane (Zp = 0.75) induced by PEF pre-treatment applied at the optimized conditions was effective to increase the extraction yield of phenolic compounds, and especially of epicatechin and p-coumaric acid up to a concentration 61 and 114%, respectively, higher that detected for control extraction.
Conclusions
The results of this work have demonstrated that the optimization of the experimental parameters of the PEF-assisted extraction process enabled to sustainably enhance the extraction yield of valuable compounds, such as total phenolic content (TPC, +8%), flavonoid content (FC, +31%), as well as the antioxidant activity (FRAP, +36%) of the extracts obtained from white grape pomace.
The effects of the PEF process parameters, namely field strength and energy input, on the cell permeabilization of grape pomace tissue (Zp), as well as the effects of SLE conditions, such as temperature, diffusion time, and ethanol concentration, on TPC, FC and FRAP values, were determined using a FC-CCD and response surface methodology (RSM). The variables were significant, and the quadratic and third order model accurately predicted the investigated responses for PEF treatment and extraction process, respectively.
Interestingly, optimum processing parameters of PEF-assisted extraction can either intensify the extractability of valuable molecules (polyphenols) with high antioxidant power or reduce the extraction time (by 23–103 min) and the solvent consumption (by 3–12%), compared to conventional SLE. Moreover, it was shown that moderate heating (50°C) and an intermediate ethanol concentration (50%) could be selected as the most efficient parameters in order to maximize TPC, FC and FRAP values while reducing solvent disposal and process costs.
The HPLC analyses also revealed that PEF pre-treatment further enhanced the extraction yield of the identified phenolic compounds, namely, p-coumaric acid, and quercetin, and no degradation of phenolic compounds occurred upon the application of PEF.
Overall, these promising results confirm the potential of optimized PEF-assisted extraction process to promote the valorization grape processing by-products through sustainable recover with high yield of valuable compounds with antioxidant proprieties. However, further studies are necessary to validate these results at pilot and pre-industrial scale in order to confirm the benefits of assisting phenolic extraction with PEF.
Data Availability Statement
The original contributions presented in the study are included in the article/Supplementary Material, further inquiries can be directed to the corresponding author/s.
Author Contributions
GP and GF contributed conception, design of the study, and supervised the study. SC was in charge of performing chemical and statistical analysis and wrote the first draft of the manuscript. GP and SC performed the experiments. All authors contributed to manuscript revision, read, and approved the submitted version.
Funding
This research was supported by the project Pasta for fun (No. F/200122/01-02/X45) and by the research project ValICET (B45F20003170005).
Conflict of Interest
The authors declare that the research was conducted in the absence of any commercial or financial relationships that could be construed as a potential conflict of interest.
Publisher's Note
All claims expressed in this article are solely those of the authors and do not necessarily represent those of their affiliated organizations, or those of the publisher, the editors and the reviewers. Any product that may be evaluated in this article, or claim that may be made by its manufacturer, is not guaranteed or endorsed by the publisher.
Acknowledgments
The authors wish to thank Eng. Alessandro Russo for his invaluable help with chemical analyses.
Supplementary Material
The Supplementary Material for this article can be found online at: https://www.frontiersin.org/articles/10.3389/fsufs.2022.854968/full#supplementary-material
References
Agati, G., Azzarello, E., Pollastri, S., and Tattini, M. (2012). Flavonoids as antioxidants in plants: Location and functional significance. Plant Sci. 196, 67–76. doi: 10.1016/j.plantsci.2012.07.014
Agbo, M. O., Uzor, P. F., Akazie-Nneji, U. N., Eze-Odurukwe, C. U., Ogbatue, U. B., and Mbaoji, E. C. (2015). Antioxidant, total phenolic and flavonoid content of selected Nigerian medicinal plants. Dhaka University J. Pharmaceut. Sci. 14, 35–41. doi: 10.3329/dujps.v14i1.23733
Andrade, M. A., Lima, V., Sanches Silva, A., Vilarinho, F., Castilho, M. C., Khwaldia, K., et al. (2019). Pomegranate and grape by-products and their active compounds: Are they a valuable source for food applications? Trends Food Sci. Technol. 86, 68–84. doi: 10.1016/j.tifs.2019.02.010
Barba, F. J., Brianceau, S., Turk, M., Boussetta, N., and Vorobiev, E. (2015a). Effect of alternative physical treatments (ultrasounds, pulsed electric fields, and high-voltage electrical discharges) on selective recovery of bio-compounds from fermented grape pomace. Food Bioprocess Technol. 8, 1139–1148. doi: 10.1007/s11947-015-1482-3
Barba, F. J., Grimi, N., and Vorobiev, E. (2015b). New approaches for the use of non-conventional cell disruption technologies to extract potential food additives and nutraceuticals from microalgae. Food Eng. Rev. 7, 45–62. doi: 10.1007/s12393-014-9095-6
Barba, F. J., Zhu, Z., Koubaa, M., Sant'Ana, A. S., and Orlien, V. (2016). Green alternative methods for the extraction of antioxidant bioactive compounds from winery wastes and by-products: A review. Trends Food Sci. Technol. 49, 96–109. doi: 10.1016/j.tifs.2016.01.006
Battipaglia, G., De Vito, F., Dons,ì, F., Pataro, G., and Ferrari, G. (2009). “Enhancement of polyphenols extraction from involucral bracts of artichokes,” in: Proceeding of the International Conference Bio & Food Electrotechnologies – BFE 2009, Université de Technologie de Compiegne, Compiegne, France.
Benzie, I. F. F., and Strain, J. J. (1996). The ferric reducing ability of plasma (FRAP) as a measure of antioxidant power: The FRAP assay. Analyt. Biochem. 239, 70–76. doi: 10.1006/abio.1996.0292
Beres, C., Costa, G. N. S., Cabezudo, I., da Silva-James, N. K., Teles, A. S. C., Cruz, A. P. G., et al. (2017). Towards integral utilization of grape pomace from winemaking process: A review. Waste Manage. 68, 581–594. doi: 10.1016/j.wasman.2017.07.017
Bobinaitė, R., Pataro, G., Lamanauskas, N., Šatkauskas, S., Viškelis, P., and Ferrari, G. (2015). Application of pulsed electric field in the production of juice and extraction of bioactive compounds from blueberry fruits and their by-products. J. Food Sci. Technol. 52, 5898–5905. doi: 10.1007/s13197-014-1668-0
Boussetta, N., Lebovka, N., Vorobiev, E., Adenier, H., Bedel-Cloutour, C., and Lanoisell,é, J. L. (2009). Electrically assisted extraction of soluble matter from chardonnay grape skins for polyphenol recovery. J. Agric. Food Chem. 57, 1491–1497. doi: 10.1021/jf802579x
Brazinha, C., Cadima, M., and Crespo, J. G. (2014). Optimization of extraction of bioactive compounds from different types of grape pomace produced at wineries and distilleries. J. Food Sci. 79, 12476. doi: 10.1111/1750-3841.12476
Brianceau, S., Turk, M., Vitrac, X., and Vorobiev, E. (2015). Combined densification and pulsed electric field treatment for selective polyphenols recovery from fermented grape pomace. Innovative Food Sci. Emerg. Technol. 29, 2–8. doi: 10.1016/j.ifset.2014.07.010
Buttol, P., Creo, C., Cutaia, L., Benedetto, E., Di Nobili, P., Pentassuglia, R., et al. (2018). Uso efficiente delle risorse nelle imprese olivicole olearie. ENEA Agenzia nazionale per le nuove tecnologie, l'energia e lo sviluppo economico sostenibile.
Caldas, T. W., Mazza, K. E. L., Teles, A. S. C., Mattos, G. N., Brígida, A. I. S., Conte-Junior, C. A., et al. (2018). Phenolic compounds recovery from grape skin using conventional and non-conventional extraction methods. Ind. Crops Prod. 111, 86–91. doi: 10.1016/j.indcrop.2017.10.012
Carpentieri, S., Mazza, L., Nutrizio, M., Jambrak, A. R., Ferrari, G., and Pataro, G. (2021). Pulsed electric fields- and ultrasound-assisted green extraction of valuable compounds from Origanum vulgare L. and Thymus serpyllum L. Int. J. Food Sci. Technol. 2021, 1–9. doi: 10.1111/IJFS.15159/v2/response1
Carpentieri, S., ReŽek Jambrak, A., Ferrari, G., and Pataro, G. (2022). Pulsed electric field-assisted extraction of aroma and bioactive compounds from aromatic plants and food by-products. Front. Nutr. 8, e792203. doi: 10.3389/fnut.2021.792203
Casazza, A. A., Aliakbarian, B., De Faveri, D., Fiori, L., and Perego, P. (2012). Antioxidants from winemaking wastes: A study on extraction parameters using response surface methodology. J. Food Biochem. 36, 28–37. doi: 10.1111/j.1745-4514.2010.00511.x
Casazza, A. A., Aliakbarian, B., Mantegna, S., Cravotto, G., and Perego, P. (2010). Extraction of phenolics from Vitis vinifera wastes using non-conventional techniques. J. Food Eng. 100, 50–55. doi: 10.1016/j.jfoodeng.2010.03.026
Chowdhary, P., Gupta, A., Gnansounou, E., Pandey, A., and Chaturvedi, P. (2021). Current trends and possibilities for exploitation of Grape pomace as a potential source for value addition. Environ. Pollut. 278, 116796. doi: 10.1016/j.envpol.2021.116796
Coelho, M. C., Pereira, R. N., Rodrigues, A. S., Teixeira, J. A., and Pintado, M. E. (2020). The use of emergent technologies to extract added value compounds from grape by-products. Trends Food Sci. Technol. 106, 182–197. doi: 10.1016/j.tifs.2020.09.028
Corrales, M., Toepfl, S., Butz, P., Knorr, D., and Tauscher, B. (2008). Extraction of anthocyanins from grape by-products assisted by ultrasonics, high hydrostatic pressure or pulsed electric fields: A comparison. Innovative Food Sci. Emerg. Technol. 9, 85–91. doi: 10.1016/j.ifset.2007.06.002
Da Rocha, C. B., and Noreña, C. P. Z. (2020). Microwave-assisted extraction and ultrasound-assisted extraction of bioactive compounds from grape pomace. Int. J. Food Eng. 16, 1–10. doi: 10.1515/ijfe-2019-0191
Donsì, F., Ferrari, G., Fruilo, M., and Pataro, G. (2010a). Pulsed electric field-assisted vinification of aglianico and piedirosso grapes. J. Agric. Food Chem. 58, 11606–11615. doi: 10.1021/jf102065v
Donsì, F., Ferrari, G., and Pataro, G. (2010b). Applications of pulsed electric field treatments for the enhancement of mass transfer from vegetable tissue. Food Eng. Rev. 2, 109–130. doi: 10.1007/s12393-010-9015-3
Frontuto, D., Carullo, D., Harrison, S. M., Brunton, N. P., Ferrari, G., Lyng, J. G., et al. (2019). Optimization of pulsed electric fields-assisted extraction of polyphenols from potato peels using response surface methodology. Food Bioprocess Technol. 12, 1708–1720. doi: 10.1007/s11947-019-02320-z
Guaita, M., and Bosso, A. (2019). Polyphenolic characterization of grape skins and seeds of four Italian red cultivars at harvest and after fermentative maceration. Foods 8, 395. doi: 10.3390/foods8090395
Hogervorst, J. C., Miljić, U., and Puškaš, V. (2017). Extraction of Bioactive Compounds From Grape Processing By-Products. C. M., Galanakis. London: Elsevier Inc. doi: 10.1016/B978-0-12-809870-7.00005-3
Juri,ć, S., Ferrari, G., Velikov, K. P., and Dons,ì, F. (2019). High-pressure homogenization treatment to recover bioactive compounds from tomato peels. J. Food Eng. 262, 170–180. doi: 10.1016/j.jfoodeng.2019.06.011
Kammerer, D. R., Kammerer, J., Valet, R., and Carle, R. (2014). Recovery of polyphenols from the by-products of plant food processing and application as valuable food ingredients. Food Res. Int. 65, 2–12. doi: 10.1016/j.foodres.2014.06.012
Kato-Schwartz, C. G., Corrêa, R. C. G., de Souza Lima, D., de Sá-Nakanishi, A. B., de Almeida Gonçalves, G., Seixas, F. A. V., et al. (2020). Potential anti-diabetic properties of Merlot grape pomace extract: An in vitro, in silico and in vivo study of α-amylase and α-glucosidase inhibition. Food Res. Int. 137, 109462. doi: 10.1016/j.foodres.2020.109462
Kwiatkowski, M., Kravchuk, O., Skouroumounis, G. K., and Taylor, D. K. (2020). Response surface parallel optimization of extraction of total phenolics from separate white and red grape skin mixtures with microwave-assisted and conventional thermal methods. J. Clean. Prod. 251, 119563. doi: 10.1016/j.jclepro.2019.119563
Lal, A. M. N., Prince, M. V., Kothakota, A., Pandiselvam, R., Thirumdas, R., Mahanti, N. K., et al. (2021). Pulsed electric field combined with microwave-assisted extraction of pectin polysaccharide from jackfruit waste. Innovative Food Sci. Emerg. Technol. 74, 102844. doi: 10.1016/j.ifset.2021.102844
López, N., Puértolas, E., Hernández-Orte, P., Álvarez, I., and Raso, J. (2009). Effect of a pulsed electric field treatment on the anthocyanins composition and other quality parameters of Cabernet Sauvignon freshly fermented model wines obtained after different maceration times. LWT - Food Sci. Technol. 42, 1225–1231. doi: 10.1016/j.lwt.2009.03.009
Luengo, E., Álvarez, I., and Raso, J. (2013). Improving the pressing extraction of polyphenols of orange peel by pulsed electric fields. Innovative Food Sci. Emerg. Technol. 17, 79–84. doi: 10.1016/j.ifset.2012.10.005
Luengo, E., Condón-Abanto, S., Álvarez, I., and Raso, J. (2014). Effect of pulsed electric field treatments on permeabilization and extraction of pigments from chlorella vulgaris. J. Membrane Biol. 247, 1269–1277. doi: 10.1007/s00232-014-9688-2
Maroun, R. G., Rajha, H. N., Vorobiev, E., and Louka, N. (2017). Emerging Technologies for the Recovery of Valuable Compounds From Grape Processing By-Products. Handbook of Grape Processing By-Products: Sustainable Solutions. London: Elsevier Inc. doi: 10.1016/B978-0-12-809870-7.00007-7
Melo, P. S., Massarioli, A. P., Denny, C., Dos Santos, L. F., Franchin, M., Pereira, G. E., et al. (2015). Winery by-products: Extraction optimization, phenolic composition and cytotoxic evaluation to act as a new source of scavenging of reactive oxygen species. Food Chem. 181, 160–169. doi: 10.1016/j.foodchem.2015.02.087
Mikeš, O., Vrchotová, N., Tríska, J., Kyseláková, M., and Šmidrkal, J. (2008). Distribution of major polyphenolic compounds in vine grapes of different cultivars growing in south moravian vineyards. Czech J. Food Sci. 26, 182–189. doi: 10.17221/1591-CJFS
Moletta, R. (2005). Winery and distillery wastewater treatment by anaerobic digestion. Water Sci. Technol. 51, 137–144. doi: 10.2166/wst.2005.0017
Muhlack, R. A., Potumarthi, R., and Jeffery, D. W. (2018). Sustainable wineries through waste valorisation: A review of grape marc utilisation for value-added products. Waste Manage. 72, 99–118. doi: 10.1016/j.wasman.2017.11.011
OIV International Organization of Vine and Wine (2019). Statistical Report on World Vitiviniculture. 2019 Statistical Report on World Vitiviniculture. Available online at: http://www.oiv.int/public/medias/5029/world-vitiviniculture-situation-2016.pdf (accessed January 10, 2022).
Pataro, G., Bobinaite, R., Bobinas, C., Šatkauskas, S., Raudonis, R., Visockis, M., et al. (2017). Improving the extraction of juice and anthocyanins from blueberry fruits and their by-products by application of pulsed electric fields. Food Bioprocess Technol. 10, 1595–1605. doi: 10.1007/s11947-017-1928-x
Pataro, G., Carullo, D., Bakar Siddique, M. A., Falcone, M., Dons,ì, F., and Ferrari, G. (2018). Improved extractability of carotenoids from tomato peels as side benefits of PEF treatment of tomato fruit for more energy-efficient steam-assisted peeling. J. Food Eng. 233, 65–73. doi: 10.1016/j.jfoodeng.2018.03.029
Pataro, G., Carullo, D., Falcone, M., and Ferrari, G. (2020). Recovery of lycopene from industrially derived tomato processing by-products by pulsed electric fields-assisted extraction. Innovative Food Sci. Emerg. Technol. 63, 102369. doi: 10.1016/j.ifset.2020.102369
Pataro, G., Carullo, D., and Ferrari, G. (2019). PEF-assisted supercritical CO2 extraction of pigments from microalgae nannochloropsis oceanica in a continuous flow system. Chem. Eng. Transact. 74, 97–102. doi: 10.3303/CET1974017
Pataro, G., Ferrari, G., and Donsi, F. (2011). Mass transfer enhancement by means of electroporation. Mass Transfer Chem. Eng. Processes. 8, 151–176. doi: 10.5772/22386
Pravitha, M., Manikantan, M. R., Ajesh Kumar, V., Beegum, S., and Pandiselvam, R. (2021). Optimization of process parameters for the production of jaggery infused osmo-dehydrated coconut chips. Lwt 146, 111441. doi: 10.1016/j.lwt.2021.111441
Puértolas, E., and Barba, F. J. (2016). Electrotechnologies applied to valorization of by-products from food industry: Main findings, energy and economic cost of their industrialization. Food Bioproducts Processing 100, 172–184. doi: 10.1016/j.fbp.2016.06.020
Puértolas, E., Cregenzán, O., Luengo, E., Álvarez, I., and Raso, J. (2013). Pulsed-electric-field-assisted extraction of anthocyanins from purple-fleshed potato. Food Chem. 136, 1330–1336. doi: 10.1016/j.foodchem.2012.09.080
Rajha, H., Darra, N., El Vorobiev, E., Louka, N., and Maroun, R. G. (2013). An environment friendly, low-cost extraction process of phenolic compounds from grape byproducts. Optimization by multi-response surface methodology. Food Nutr. Sci. 4, 650–659. doi: 10.4236/fns.2013.46084
Raso, J., Frey, W., Ferrari, G., Pataro, G., Knorr, D., Teissie, J., et al. (2016). Recommendations guidelines on the key information to be reported in studies of application of PEF technology in food and biotechnological processes. Innovative Food Sci. Emerg. Technol. 37, 312–321. doi: 10.1016/j.ifset.2016.08.003
Rockenbach, I. I., Rodrigues, E., Gonzaga, L. V., Caliari, V., Genovese, M. I., De Souza Schmidt Gonçalves, A. E., et al. (2011). Phenolic compounds content and antioxidant activity in pomace from selected red grapes (Vitis vinifera L. and Vitis labrusca L.) widely produced in Brazil. Food Chem. 127, 174–179. doi: 10.1016/j.foodchem.2010.12.137
Sette, P., Fernandez, A., Soria, J., Rodriguez, R., Salvatori, D., and Mazza, G. (2020). Integral valorization of fruit waste from wine and cider industries. J. Clean. Prod. 242, e118486. doi: 10.1016/j.jclepro.2019.118486
Sirohi, R., Tarafdar, A., Singh, S., Negi, T., Gaur, V. K., Gnansounou, E., et al. (2020). Green processing and biotechnological potential of grape pomace: Current trends and opportunities for sustainable biorefinery. Bioresour. Technol. 314, 123771. doi: 10.1016/j.biortech.2020.123771
Spigno, G., Tramelli, L., and De Faveri, D. M. (2007). Effects of extraction time, temperature and solvent on concentration and antioxidant activity of grape marc phenolics. J. Food Eng. 81, 200–208. doi: 10.1016/j.jfoodeng.2006.10.021
Srikanth, V., Rajesh, G. K., Kothakota, A., Pandiselvam, R., Sagarika, N., Manikantan, M. R., et al. (2020). Modeling and optimization of developed cocoa beans extractor parameters using box behnken design and artificial neural network. Comp. Elect. Agri. 177, 105715. doi: 10.1016/j.compag.2020.105715
Thirumdas, R., Sarangapani, C., and Barba, F. J. (2020). Pulsed electric field applications for the extraction of compounds and fractions (fruit juices, winery, oils, by-products, etc.). Pulsed Electric Fields Obtain Healthier Sustain. Food Tomorrow 10, 227–246. doi: 10.1016/B978-0-12-816402-0.00010-0
Van de Wiel, A., Van Golde, P. H. M., and Hart, H. C. (2001). Blessings of the grape. Eur. J. Intern. Med. 12, 484–489. doi: 10.1016/S0953-6205(01)00170-4
Yilmaz, Y., and Toledo, R. T. (2004). Major flavonoids in grape seeds and skins : antioxidant capacity of catechin, epicatechin, and gallic acid. J. Agric. Food Chem. 52, 255–260. doi: 10.1021/jf030117h
Zhang, S., and Zhu, M. J. (2015). Characterization of polyphenolics in grape pomace extracts using ESI Q-TOF MS/MS. J. Food Sci. Nutr. 1, 001. doi: 10.24966/FSN-1076/100001
Keywords: pulsed electric fields, extraction, green solvents, response surface methodology, HPLC-PDA, grape by-products, bioactive compounds
Citation: Carpentieri S, Ferrari G and Pataro G (2022) Optimization of Pulsed Electric Fields-Assisted Extraction of Phenolic Compounds From White Grape Pomace Using Response Surface Methodology. Front. Sustain. Food Syst. 6:854968. doi: 10.3389/fsufs.2022.854968
Received: 14 January 2022; Accepted: 14 March 2022;
Published: 13 April 2022.
Edited by:
Ricardo N. Pereira, University of Minho, PortugalReviewed by:
Lilia Ahrné, University of Copenhagen, DenmarkR. Pandiselvam, Central Plantation Crops Research Institute (ICAR), India
Pedro Ferreira Santos, University of Minho, Portugal
Copyright © 2022 Carpentieri, Ferrari and Pataro. This is an open-access article distributed under the terms of the Creative Commons Attribution License (CC BY). The use, distribution or reproduction in other forums is permitted, provided the original author(s) and the copyright owner(s) are credited and that the original publication in this journal is cited, in accordance with accepted academic practice. No use, distribution or reproduction is permitted which does not comply with these terms.
*Correspondence: Gianpiero Pataro, Z3BhdGFyb0B1bmlzYS5pdA==