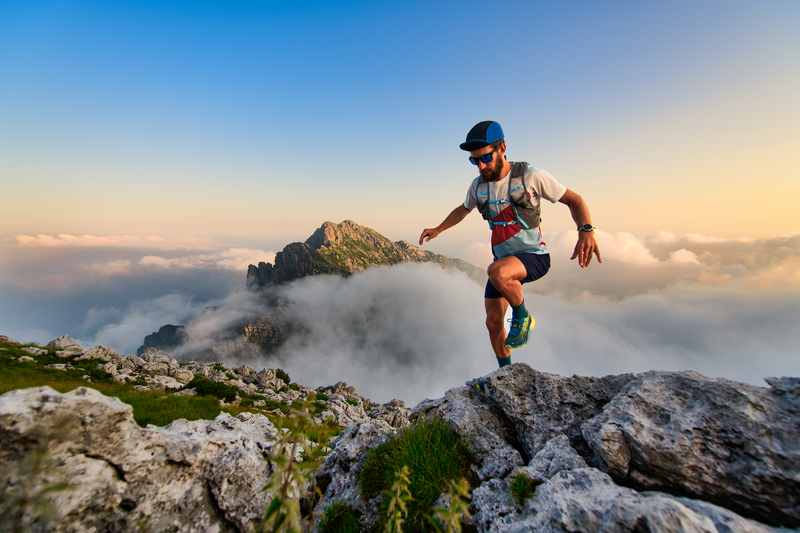
95% of researchers rate our articles as excellent or good
Learn more about the work of our research integrity team to safeguard the quality of each article we publish.
Find out more
ORIGINAL RESEARCH article
Front. Sustain. Food Syst. , 29 March 2022
Sec. Urban Agriculture
Volume 6 - 2022 | https://doi.org/10.3389/fsufs.2022.849304
This article is part of the Research Topic Rethinking Food Systems: Circular Economy and Urban Agriculture View all 4 articles
Vertical farms have expanded rapidly in urban areas to support food system resilience. However, many of these systems source a substantial share of their material and energy requirements outside their urban environments. As urban areas produce significant shares of residual material and energy streams, there is considerable potential to explore the utilization of these streams for urban agriculture in addition to the possibility of employing underutilized urban spaces in residential and commercial buildings. This study aims to explore and assess the potential for developing more circular vertical farming systems which integrate with buildings and utilize residual material and energy streams. We focus on the symbiotic development of a hypothetical urban farm located in the basement of a residential building in Stockholm. Life cycle assessment is used to quantify the environmental performance of synergies related to energy integration and circular material use. Energy-related scenarios include the integration of the farm's waste heat with the host building's heating system and the utilization of solar PV. Circular material synergies include growing media and fertilizers based on residual materials from a local brewery and biogas plant. Finally, a local pick-up system is studied to reduce transportation. The results point to large benefits from integrating the urban farm with the building energy system, reducing the vertical farm's GHG emissions up to 40%. Synergies with the brewery also result in GHG emissions reductions of roughly 20%. No significant change in the environmental impacts was found from the use of solar energy, while the local pick-up system reduces environmental impacts from logistics, although this does not substantially lower the overall environmental impacts. However, there are some trade-offs where scenarios with added infrastructure can also increase material and water resource depletion. The results from the synergies reviewed suggest that proximity and host-building synergies can improve the material and energy efficiency of urban vertical farms. The results provide insights to residential building owners on the benefits of employing residual space for urban food provisioning and knowledge to expand the use of vertical farming and circular economy principles in an urban context.
Urban farming has been identified by a number of authors to provide promising solutions to secure food supplies, produce more sustainable food, and reduce pressure on agricultural land by shifting food production to urban environments and buildings (Cockrall-King, 2012; Thomaier et al., 2014; Eigenbrod and Gruda, 2015; Goldstein et al., 2016; Bustamante, 2018). Urban-vertical farming is primarily promoted for its potential to extend the seasonal availability of regional foods, especially in Northern Europe (Graamans et al., 2018; Martin and Molin, 2019; Martin et al., 2019; Orsini et al., 2020). Examples of urban-vertical farming have seen a dramatic increase in recent years, attracting considerable interest and funding (Weidner et al., 2019; Orsini et al., 2020; S2G, 2020; Agritecture, 2021).
These systems are relatively new in the context of urban food supply, and thus it is an expanding subject of inquiry. Much of the literature has focused on technological possibilities and feasibility. Many authors promote these systems as a sustainable solution for urban areas (Despommier, 2009; Benke and Tomkins, 2017; Van Delden et al., 2021). However, evaluations of their benefits remain limited in the literature, with few studies assessing the sustainability of the systems (Romeo et al., 2018; Sanjuan-Delmás et al., 2018; Gentry, 2019; Martin and Molin, 2019). Furthermore, despite some studies addressing the potential benefits of including these systems in urban environments (Eigenbrod and Gruda, 2015; Kozai and Niu, 2016; Eaves and Eaves, 2018), the literature remains limited.
Many previous studies and current commercial vertical farms employ and outline linear approaches, relying on imported materials from outside their immediate regions without any recycling or reuse, e.g., growing media and fertilizer. With these systems rapidly expanding worldwide in urban environments, the potential for integration with their urban surroundings and utilizing urban residual material and energy is vital to promote a circular economy (Chance et al., 2018; Martin and Harris, 2018; Martin et al., 2019). In recent years increased interest in the academic literature has been directed to address this topic, with studies identifying how different urban farming techniques can be integrated with urban residual flows and infrastructure; see, e.g., Mohareb et al. (2017), Chance et al. (2018), Marchi et al. (2018), Sanjuan-Delmás et al. (2018), Gentry (2019), Martin et al. (2019), and Dorr et al. (2021). However, the literature is abundant with examples of integration with urban systems by employing vacant plots for urban gardening and rooftop greenhouses (Thomaier et al., 2014; Goldstein et al., 2016; Dorr et al., 2017; Sanjuan-Delmás et al., 2018; Jones and Franck, 2019; Orsini et al., 2020; Pulighe and Lupia, 2020). Despite their prevalence, such examples are scarce in the context of Northern Europe, the focus in this article, and few examples have been highlighted for the integration of urban vertical farming and urban residuals (Chance et al., 2018; Martin et al., 2019). Instead, many of the larger-scale urban-vertical farms are primarily confined to indoor environments through controlled environment agriculture (CEA) techniques, with many examples of vertical farms in underutilized rooms, vacant buildings, underground in parking garages, basements, storage rooms, in containers in parking lots, and displays in shops and commercial environments (Bustamante, 2020; Martin and Bustamante, 2021).
A host of literature suggests a substantial potential to reduce the environmental impacts through symbiotic developments (Dong et al., 2013; Kim et al., 2017; Martin and Harris, 2018; Martin, 2020). However, there are few assessments of the implications of integrating indoor farms with urban building energy systems, taking advantage of the building envelope, and synergies with surrounding businesses. As such, sustainable solutions for more integrated food, water, energy, and transportation of food are needed, with urban agriculture highlighted as a possible solution to integrate these systems (Specht et al., 2014; Martin et al., 2019; Rufí-Salís et al., 2020). Furthermore, with cities becoming important as a driving force for the circular economy and as a critical stakeholder for developing and improving food security, studying and quantifying the potential of urban food production from urban vertical farms in different urban contexts is essential for understanding and planning for future urban food systems.
The aim of this study is to explore and assess the potential for developing circular urban vertical farming systems which integrate with buildings and employ residual material and energy streams from regional firms. The article offers novel insights on the symbiotic development of vertical farms within buildings and urban environments, bridging the literature on urban agriculture, vertical farming, sustainable urban development, and industrial and urban symbiosis.
In the following sections, we first describe the methodology used to assess the environmental implications (Section Methodology). Thereafter, we present the hypothetical case study area to give background, followed by a description of the baseline system assessed, followed by a description of the different synergies with the host building and synergies with external actors. We then highlight results from our life cycle assessment of the different scenarios and further analyze the results and their sensitivity (Section Results and Analysis). We then discuss the results and future research opportunities (Section Discussion), followed by a concluding discussion (Section Conclusions).
Life cycle assessment was employed to assess the environmental sustainability of the different synergistic options. The functional unit used for the environmental assessment is the annual production of plants from the hypothetical vertical farm (roughly 185 000 plants per year with an edible portion weight of ~5 500 kg) available to consumers. It is assumed that the farm will produce a mix of leafy greens and herbs. Furthermore, to show the impacts related to the plants, the environmental impacts per kg edible portion of the plants are also highlighted. The study is limited to the production and final availability of the product to consumers. As such, the study was conducted using a cradle-to-gate perspective, including all upstream processes in the cultivation, energy use, transportation of materials, and distribution of the plants to regional supermarkets. No waste handling for the final product after consumption was modeled as it was considered outside the scope of the assessment. This includes the pots and growing media in the potted plants. However, waste handling from any waste generated at the farm was included in the assessment, including biowaste, plastics, and water. A depiction of the system boundaries is available in Figure 1.
Figure 1. System boundaries of the study, including all material and energy inputs, and avoided processes from by-products of the vertical farm.
For the LCA of symbiotic exchanges, this article employs a system expansion methodology (i.e., semi-consequential approach). This is done to allow for the depiction of the benefits of integration with the building and surrounding businesses and motivated by the scope of the study, to explore the implications of symbiotic exchanges with the host building and residual flows from regional systems to improve the environmental performance of the vertical farm, see, e.g., guidelines in Martin et al. (2015) and Brandao et al. (2017). As such, the vertical farm is of primary importance, and the benefits provided to surrounding systems (other than the building with which it is integrated) through the use of residual materials, etc., are excluded. Any intermediate processes for the synergies, e.g., for the use of new materials, are included in the assessment as outlined in the section below for different synergies.
The LCA for the different scenarios was conducted in the OpenLCA software v 1.10.3 (Greendelta, 2018). For this study, the International Reference Life Cycle Data System (ILCD, 2011) midpoint life cycle impact assessment (LCIA) method was employed as it is widely regarded as a consistent and robust method for exploring the implications of products and services in the European market (JRC, 2010). The impact categories included in the main text are Global warming potential (measured in kg CO2-eq) and denoted (GHG), Water resource depletion (WRD) measured in m3 water, Acidification Potential (AP) measured in molc H+ eq, Freshwater Eutrophication (FE) measured in kg P-eq, and Mineral, fossil, and renewable resource depletion (RD)measured in kg Sb-eq. These particular impact categories were chosen as they provide a review of the regional, global, and resource implications of food systems and their importance in the changes studied, i.e., altering the energy demand and sources, fertilizers, material inputs, and waste handling methods. These are also of particular interest for our comparisons, and similar impact categories are commonly used in a number of studies on agricultural systems and urban farming applications. However, the ILCD method contains 16 indicators, and results from the study for those indicators not highlighted in the main text are provided in Supplementary Material for further information.
All life cycle inventory (LCI) data were obtained from the LCI database Ecoinvent v. 3.6 (Ecoinvent, 2019). Further details are also provided in the subsequent sections for all scenarios assessed. See further details in Supplementary Material for corresponding specific datasets, references for all processes and materials used, in addition to further descriptions on modeling choices.
This study focuses on a prospective assessment of a hypothetical vertical farm located in the Hammarby Sjöstad, a city district within Stockholm, Sweden. The Hammarby Sjöstad district is located on the southern edge of Stockholm with ~25,000 inhabitants. It has been a testbed for the experimentation of innovative environmental technologies and urban symbiosis strategies (Pandis Iveroth et al., 2013). Recent investigations have also addressed issues related to food, photovoltaics, and circular development in the region (ElectriCity, 2021). We chose this area to investigate the potential for the symbiotic development of a vertical farm with the building envelope and surrounding businesses and infrastructure given the background and interest, as it offers synergies with local actors. Furthermore, it was selected as the case study area in the funded project.
To model the potential of symbiotic exchanges with the host building and surrounding businesses, a reference scenario is created to model the hypothetical vertical farm, i.e., with no connections to other systems, labeled Reference. This vertical farm is modeled as a small-scale hypothetical farm employing a hydroponic nutrient film technique (NFT). It is located in a vacant storage room in the basement of a residential building in the case study area. The building is assumed to be an average residential building in the Hammarby Sjöstad area. These are typically around five stories high and have a footprint of up to 7 000 m2. The total area for the small-scale vertical farm and other associated processes within the residual space is roughly 100 m2. The plants are grown on vertically stacked structures. Each tray has a footprint of ~3 m2, with four layers of trays for each rack structure. In total, 50 m2 is used as a footprint for the stacked structures1. The plants are assumed to be grown in pots made of polystyrene with a growing media comprised of an equal mix, by weight, of peat and coir. The plants are cultivated manually by removing the plants in their pots for manual packaging; as such, no infrastructure for their cultivation is included. The final products are sold as potted plants in a polyethylene cover with labels. All material waste from the farm is assumed to be sent to local waste incineration.
For lighting and temperature control, several assumptions were made. Light-emitting diode (LED) lights are used to provide adequate photosynthetically active radiation for plant growth according to the plant's growth stage. On average, the plants are assumed to receive around 216 μmol/m2-s for 16 h with a power consumption of 111 W/m2. The air in the facility was considered to be constantly conditioned to ensure a conducive environment for plant growth; the temperature is kept at 25°C (19°C during the dark period) and 73% relative humidity (83% during the dark period); see, e.g., Graamans et al. (2017). A fraction of the air is conveyed via fans through an air handling unit, consisting of a heat recovery section, a water-cooled chiller, and a heat pump. The electricity consumption of compressors, fans, and pumps is modeled for the photo and dark period on a monthly basis. The cooling load was derived from modeling the plant evapotranspiration, which, together with the waste heat from the LEDs, determines the air conditioning requirements. A schematic, detailed explanation, and all equations and assumptions for this are provided in Supplementary Material.
All infrastructure and supporting systems for the vertical farm are included in the assessment. This consists of the structures, LED armatures, pumps, pipes and hoses, tanks, and other electronics required. Furthermore, all transportation for materials and infrastructural components needed, in addition to all transportation of outputs (including final products and waste), are included in the study. For all material and infrastructure transportation, it is assumed that the transportation is conducted by truck. As a small vertical farm, all deliveries of the product in the Reference scenario are assumed to be conducted by a mid-sized vehicle, i.e., a van.
Table 1 provides an overview of all material and energy inputs and outputs to the system. Further details on all assumptions for material, utilities, and the infrastructure are provided in Supplementary Material.
From the Reference scenario vertical farm, prospective synergies with surrounding businesses and the host building are assessed. The different synergies are illustrated in Figure 2 and further described in the subsequent sections. These are outlined and assessed as separate synergies, while an additional scenario with several synergies integrated is also evaluated.
Figure 2. Depiction of material and energy exchanges between the building, the vertical farm, and connected systems for the different synergistic scenarios. These include (1) integration with rooftop photovoltaics (PV) in the PV15 and PV30 scenarios, (2) utilizing biogas digestate fractions as a biofertilizer (Biofert.), (3) employing local market delivery services in the Local Market scenario, (4) utilizing brewers spent grain (BSG) and Carbon dioxide (CO2) from a local brewery in the BrewInteg scenario, and (5) integrating waste heat from the LEDs with the heating system of the host building in the Bldng Int. HP-WWTP and Bldng. Int. DH-W2E scenarios.
Vertical farms are often suggested to have substantial energy demand, contributing to a large share of their environmental impacts, even in low-carbon energy systems such as Sweden (Gentry, 2019; Martin and Molin, 2019; Weidner et al., 2021). In order to supplement the energy demand, and due to the interest in the specific case study region (ElectriCity, 2021), the installation of a photovoltaic (PV) system on the roof of the building was assessed to provide energy to the vertical farm. In these scenarios, the environmental implications of their integration with the vertical farm were evaluated.
For the installation, a total roof area of roughly 250 m2 was assumed to be available in the modeled building. This includes assessing the effect of including the PV installation with a capacity of 15 kW and 30 kW, where two scenarios are assessed. These are labeled PV15 and PV30, respectively. All electricity from the PV arrays is assumed to be used solely for the vertical farm. As the solar radiation varies throughout the year, the production fluctuates, with less electricity produced in winter and more in summer. The system includes the installation of the solar PV infrastructure, with an assumed lifetime of 30 years. As such, all impacts from the infrastructure are included in the datasets employed; see Supplementary Material. As the system cannot provide the required electricity demand throughout the year, additional electricity is needed. Further details on the PV system, installation parameters, and monthly capacity are provided in Supplementary Material.
Conventional fertilizers are often used in the vertical farming industry. In this scenario, labeled Biofert., the environmental implications of employing the biofertilizer from anaerobic digestion plants were assessed (Tampio et al., 2016). The use of biogas digestate as a biofertilizer is becoming increasingly studied for hydroponic systems, see, e.g., Martin et al. (2019) and Lind et al. (2021). For this scenario, it is assumed that the biofertilizer is from a concentrated fertilizer solution developed from biogas digestate. The biofertilizer was assumed to be sourced from a biogas plant outside Stockholm. However, the figures are developed based on Eriksson and Runevad (2016) and Strandmark (2019) for digestate from a co-digestion plant in Sweden, which uses a large share of household food waste. For the assessment, we assumed that the required nutrients for the vertical farm would be similar to the Reference scenario. The nutrient content in the biofertilizer, however, is not similar to the conventional fertilizer blend. As such, additional macro and micronutrients are required and were included as additional inputs; see Supplementary Material for further details. Intermediate processing to obtain the biofertilizer from the liquid fraction of the digestate and transportation of the biofertilizer were included. This included a decanting centrifuge to extract the liquid, a new fertilizer reservoir, biofilm carriers, and an auxiliary pump for the nitrification process. The transportation distance was assumed to be 30 km from the nearest co-digestion plant. Further details on the nutrient amounts and intermediate processing can be found in Supplementary Material.
In order to model the potential for creating a local demand/market for the products, requiring no wholesaler, and employing new business models for providing the produced plants for the neighborhood, a scenario was created to assess the implications of selling the products through a local pick-up depot, labeled Local Market. In this scenario, it is assumed that the plants are sold directly to consumers from the farm through a local pick-up depot and directly in local retail locations, requiring no additional transportation. It is assumed that the pick-up depot is located in the vicinity of the building housing the vertical farm. The local pick-up depot is assumed to be a self-service system, requiring a mobile device to enter a code and open a box with the order. These are popular options in all larger grocery store chains in Sweden; see Axfoods (2020) and McKinsey (2020). The modeling of this system is based on a similar modular locker system for renting goods through a mobile application as outlined in Martin et al. (2021) and information from Keba (2020). As the throughput for this local pick-up depot may not be as large as the weekly output of the farm, it is assumed that all additional plants are sold in conventional retail at local retail locations requiring no transportation. Further details on the modeling of the pick-up depot are provided in Supplementary Material.
In close vicinity to the building and vertical farm is a brewery producing roughly 1 million liters of beer per year (NC, 2021). Furthermore, the brewing process generates large volumes of brewers' spent grains (BSG), carbon dioxide from fermentation, heat, and other materials, e.g., spent yeast and hop cake, which often goes unused, wasted, or used in low-value applications (Thiago et al., 2014). For this study, only the BSG and CO2 were explored for synergies with the vertical farm in proximity with the brewery. Previous assessments have also highlighted the potential of utilizing residual brewing materials in the vertical farming system, see, e.g., Martin et al. (2019). In this scenario, integration with the brewery was explored, labeled BrewInteg.
BSG used as a growing medium was explored in this study to replace peat used in the pots and combined with coir, thus as a supplement in growing media. An intermediate process of drying and pasteurizing the grains is included in the assessment to allow for the BSG to be used in the farm to increase its shelf life and applicability as a growing medium (Tang et al., 2005). Furthermore, it was assumed that the transportation of BSG to the vertical farm was no further than 10 km round-trip due to geographical proximity. The CO2 was considered to be captured and compressed into tanks and employed for carbon enrichment in the vertical farm to expedite growing conditions. All intermediate processing for the CO2 is included in the impacts for the CO2, including compressing and transportation. Further details are provided in Supplementary Material.
Urban-vertical farms, especially those in Sweden, are often located within a building envelope, for example, in the basement, parking garage, or other residual space in the building, and often not integrated. In this scenario, labeled Bldng Int. the integration of the heating system of the building with the vertical farm is analyzed to address how the host building can benefit from the vertical farm and vice versa.
Residential buildings in the Hammarby Sjöstad area use district heat to heat the rooms during the colder months (space heating). The air conditioning system (AC) of the vertical farm transfers heat generated from the LEDs and moisture from the plants away from the facility (cooling), rejecting it elsewhere. In the reference scenario, the heat is rejected to the outside air through a rooftop air cooler. For the integrated case, the waste heat generated was considered to be used for space heating, thus replacing district heat. This was calculated on a monthly basis, considering space heating needs and accounting for the electricity savings of not requiring a rooftop air cooler.
The district heat for the case study area is derived from a wastewater treatment plant that lifts water at 30°C with a heat pump to the target distribution temperature, a relatively novel practice (Stockholm Exergi, 2019). In order to model the results of this particular case with those more common in Stockholm, and Sweden in general, two scenarios are used to study the effect of replacing district heating. One scenario considers the replacement of the current district heating system employed based on a heat pump system in the local wastewater treatment plant [saving electricity for the heat pump (HP)] and labeled Bldng Int. HP-WWTP and another case considers the replacement of district heat derived from municipal waste-to-energy (W2E) plants which are more common throughout Sweden, labeled Bldng. Int. DH-W2E. Further details on the modeling for the energy system integration are provided in Supplementary Material.
Employing the results of the individual synergies assessed above, and based on those synergies showing the largest potential environmental impact improvements, a scenario labeled Optimized synergies (Opti. Integ.) is also studied. In this scenario, the scenarios with the three largest GHG emissions reduction potential were included, i.e., the building energy integration scenario, synergies with the local brewery, and employing a local market system, as outlined in subsequent sections.
From the results, it was found that there can be sensitivity to certain datasets and assumptions employed in the assessment. For these datasets and assumptions, the Sensitivity Analysis section below analyzes the influence of these datasets and assumptions. A description of the comparisons is described in these sections, and further details can also be found in Supplementary Material.
The following sections outline the results. These are presented by first reviewing the impacts of the reference scenario to provide context. Thereafter, the influences of the symbiotic scenarios are assessed, followed by an analysis of the contributions of different processes, the influence on the final product, sensitivity to modeling choices, and datasets.
As illustrated in Figure 3, for the reference scenario, in nearly all environmental impact categories, energy contributes to the largest share of the environmental impacts. This is primarily a result of the electricity use for the LEDs and the HVAC system employed, contributing to over 50% of the GHG emissions and acidification and freshwater eutrophication impacts. Furthermore, the majority of the resource and water depletion impacts, over 98%, were due to electricity demand.
Figure 3. Contribution of different processes to the annual environmental impacts in the Reference Scenario, shown in the contribution (percentage) to each impact category.
Roughly 20% of the GHG and acidification impacts are due to the pots and growing media. Transportation, for both inputs and of the products to market, had only a small contribution to the environmental impacts. Together, transportation of inputs and final products to the market accounted for <8% of all GHG emissions in addition to acidification and eutrophication impacts. For freshwater eutrophication, GHG emissions, and acidification, packaging also has a minor contribution to these impact categories, accounting for roughly 8% in all aforementioned impact categories, which was primarily a result of the polyethylene packaging.
The results illustrate that all scenarios, except those with PV arrays, were found to have lower GHG emissions. GHG emissions are reduced largely through integration with residual streams from the local brewery. In this scenario, these two options, i.e., the use of BSG as a growing media and using the CO2, are illustrated in Figure 4 to reduce the impacts from the Pots and Media and CO2 processes. The scenario including the use of biofertilizer had a slight reduction in impacts, again primarily due to the reduction of conventional fertilizers required.
Figure 4. Annual GHG emissions for different scenarios illustrating the contribution of different processes and the overall impacts (shown in kg CO2-eq annually).
As illustrated in Figure 4, the scenarios with the largest reduction in environmental impacts are those of building energy system integration and synergies with a local brewery. For the integrated building energy scenarios, i.e., Bldng Int. HP-WWTP and Bldng Int. DH-W2E, despite no large changes to the overall farm-based emissions, the credits for the use of heat extracted from the LEDs were illustrated to have large potential benefits. For the latter scenario, replacing district heating from waste-to-energy sources had the largest benefit for the system. Finally, when combining the most beneficial scenarios, i.e., in the Opt. Integ, the overall GHG emissions are further reduced.
The scenarios with PV arrays were illustrated to have higher overall GHG emissions compared to all other scenarios. This was partly due to an increase in infrastructure emissions for installing the PVs for the vertical farm, see Figure 4 above. Upon further analysis, this was also found to be due to higher GHG emissions per kWh from photovoltaics compared to the chosen electricity source. For example, while the chosen LCI dataset for electricity from the Swedish electricity mix had GHG emissions of roughly 45 g CO2-eq per kWh, the resulting GHG emissions from PV sourced electricity was roughly 76 g CO2-eq per kWh.
However, despite lowering GHG emissions, as Table 2 illustrates, there may be trade-offs with the symbiotic scenarios, where other impact categories assessed may increase. As an example, the local market scenario was illustrated to have lower GHG emissions and acidification impacts compared to the Reference scenario, due in part to a reduction in transportation. However, the resource and water resource depletion impacts were slightly higher, owing to the added infrastructure required for the local pick-up depot, e.g., metals and electronics. The biofertilizer scenario showed reductions in GHG emissions and acidification impacts from reducing conventional fertilizer application, though no notable changes in resource depletion or water resource depletion were illustrated.
Table 2. Comparison of the environmental impacts of different scenarios compared to the reference scenario.
While the scenarios with PVs were found to have increased GHG emissions and acidification emissions, in comparison to the Reference scenario, they were found to have lower resource and water resource depletion. This was primarily a result of less demand for electricity from the grid despite added infrastructure for the PVs.
The building integration scenarios had lower environmental impacts in all categories. The scenario Building Integ-W2E was illustrated to substantially reduce GHG emissions, acidification, and resource depletion, owing primarily to the credits provided from the avoidance of district heating from waste incineration. However, the water resource depletion was only reduced slightly considering the low impact of the avoided heat for water resource depletion.
As illustrated in Figure 4, there are considerable reductions possible when the synergies are integrated. Integration of the three scenarios with the largest reductions in environmental impacts, i.e., the BrewInteg, Building Integ-W2E, and Local Market scenarios, was also assessed to analyze the development of more than one synergy and create a symbiotic network between the building, farm, and regional market and businesses.
The previous results highlight the annual impacts. However, as illustrated in Figure 5, the GHG emissions per kg of edible mass from the plants are largely influenced by the synergies. The results show how the carbon footprint associated with the product can be reduced through more circular development, with carbon footprint reductions of over 50% possible.
Figure 5. GHG emissions per kg edible plant for all scenarios. Results are shown in kg CO2-eq per kg edible portion of the plant.
The results point to the scenarios with energy system building integration to have the largest environmental impact reductions. This was primarily due to the credits provided from the avoided energy consumption for heat production, as illustrated in Figure 4 above, avoiding electricity or district heating in the Bldg Int. HP-WWTP and Bldg Int. DH-W2E scenarios, respectively. These two scenarios reflect the situation in Stockholm. However, the heat source of district heating (as a co-product from electricity generation) might be different in other locations compared to the context studied, i.e., Sweden. Hence, a sensitivity analysis was conducted to analyze the influence of the type of district heating employed. Figure 6 shows the influence of different district heating types on GHG emissions.
Figure 6. Illustration of the sensitivity to choices made on the annual GHG emissions for the Bldng Int- scenarios, illustrating the contribution of different processes. Additional scenarios describe district heating from the co-production of electricity and heat in typical power plants fuelled by coal (COA), natural gas (NGS), oil (OIL) or biomass (BIO).
Table 3 further exemplifies that the credits from energy savings through integration with the building energy system are sensitive to the choice of the replaced energy system. The current electricity and district heating systems in Stockholm are already relatively low-carbon. Thus, when replacing more carbon-intensive co-produced heat, such as from coal or natural gas power plants, the attributed benefits increase drastically. This even leads to overall negative impacts of integrated vertical farms in some categories, most notably global warming.
As the energy demand was highlighted as an important contributor to all environmental impacts, primarily electricity, the study can be highly sensitive to the choice of electricity. Table 4 analyzes the influence of the electricity system. The chosen mix (Swedish electricity mix) was compared with a dataset for a Nordic electricity mix, which may contain a larger share of fossil fuels. In addition, two other datasets with only wind and hydropower-produced electricity are also compared, as many firms in Sweden choose electricity with renewable certificates from wind and hydropower.
Table 4. Sensitivity to the choice of electricity system employed on the different environmental impacts compared to reference scenario (Swedish), shown in percentage.
Comparing results of the reference scenario, which employs a Swedish electricity mix, with the use of a Nordic mix of electricity increased the GHG emissions. In contrast, employing electricity from windpower reduced the GHG emissions, while electricity from hydropower had no substantial change. However, in nearly all other environmental impact categories, the Swedish mix performed worse or similar to the Nordic electricity mix. Water resource depletion was lower for the use of wind power. Hydro and wind power also reduced acidification and eutrophication impacts, while also reducing both water resource and resource depletion. This suggests that the results are sensitive to the choice of electricity datasets and can be considered an important choice when choosing the electricity provider for the farm.
As the use of BSG as a growing media is studied as an exploratory development, the sensitivity to its use as a replacement of peat was analyzed. This includes reducing the share with which it can replace peat, and the assumptions on the energy required for its intermediate processing to ensure it is sterile for use as a hydroponic growing media.
From the BrewInteg scenario outlined above, two additional scenarios are included. The scenario BrewInteg-BSG/Peat is included, where only half of the peat is assumed to be replaced by the BSG as it is not certain that the replacement has direct equivalence. Additionally, in order to ensure the BSG does not contain contaminants, an additional sterilization process is included before being blended with other growing media. This scenario is labeled BrewInteg-Added Proc. In this scenario, additional energy requirements and equipment for an autoclave are included. Further details and assumptions are provided in Supplementary Material.
As shown in Table 5, no significant change in the environmental impacts are seen from different assumptions on the use of BSG and its added processing. The reduction of the amount of BSG in the growing media, and subsequent reintroduction of peat, increased the GHG emissions, but only slightly. Added processing, despite the added energy requirements, had not large effect on the environmental impacts.
Table 5. Testing the sensitivity to different assumptions for the use of brewers' spent grain (BSG).
This study highlights the potential of developing symbiotic systems for vertical farms through the exploration of building integration and synergies with regional businesses and markets. The following sections provide further developments on the results and discuss these in relation to other studies, in addition to highlighting limitations and potential improvement areas for developing more circular vertical farming systems.
The results of this study indicate that symbiotic development, more specifically urban symbiosis, through synergies with the host building and surrounding businesses, can lead to large environmental performance improvements. The results are consistent with the findings from several previous studies that also show that synergies with regional systems can improve the environmental performance of vertical farms by moving away from a linear approach, see, e.g., Martin et al. (2019) and Arcas-Pilz et al. (2022). However, this greatly depends on the selected synergies. While several synergies have a more considerable beneficial contribution, others have less influence on the environmental performance. Furthermore, while many of the environmental impact categories are improved, there are some trade-offs between the environmental impact categories.
As the results highlight, synergies to improve the energy demand of the host building through the use of residual heat from the vertical farm showed the most promise in reducing GHG emissions. These results also concur with earlier studies that find environmental performance benefits for urban farms through symbiotic development with energy systems (Sanjuan-Delmás et al., 2018; Sanyé-Mengual et al., 2018; Gentry, 2019). Additionally, several further studies have also suggested similar potential, despite not assessing the environmental performance, see, e.g., Thomaier et al. (2014), Eigenbrod and Gruda (2015), and Chance et al. (2018). However, for some synergies, resource depletion and water resource depletion impacts may also increase due to increased infrastructure, as shown in the Local market and BrewInteg scenario. Such concerns can be an essential aspect of the sustainability of symbiotic networks (Martin, 2020).
Developing a local market for the products also reduced the environmental impacts. In the local market scenario, the reduced transportation and logistics required for product deliveries largely reduced these farm-to-gate emissions from transport. This does partially concur with the discourse used to promote vertical urban farms (Specht et al., 2014). However, this is only applicable for more localized markets for the products with little to no distribution logistics. A number of previous studies have shown that the transportation of foods has a relatively minor impact on the overall impacts of foods (Edwards-Jones et al., 2008; Coley et al., 2009), and specifically vertical farms (Martin and Molin, 2019). Nonetheless, for vertical farms, the close proximity to consumers can also influence the choice of crops grown, which can be selected for specific attributes such as flavor and taste, which is not always possible in conventional varieties found in retail (Bogomolova et al., 2018; Harada and Whitlow, 2020).
While the study only addresses the environmental performance, the benefits also apply to other sustainability aspects, including economic performance and socio-economic development. Urban symbiosis can provide many opportunities for a number of socio-economic benefits, which may be hard to quantify. This can include shared learning, leading to human and social capital development (Velenturf and Jensen, 2016; Chance et al., 2018). For example, a previous study by Chance et al. (2018) studied the material flows and social implications of an urban symbiotic network for hydroponic systems. Furthermore, Marchi et al. (2018) also explore the possibility of symbiotic networks to improve horticulture, suggesting potential economic performance benefits. Nonetheless, few assessments of the environmental performance of the role of urban symbiosis for improving the sustainability of vertical farming systems are available in the literature, pointing to the novel results provided in this study.
As the results suggest, developing circular systems also benefits the environmental performance of the products. The impacts may be halved with more circular development compared to a reference scenario. Similar results for the potential improvements have been outlined in previous studies, see, e.g., Martin et al. (2015) and Martin (2020). However, few other studies have assessed the implications on the environmental impacts of products from vertical farms, and there are few assessments to compare with. In a recent study, however, Orsini et al. (2020) found that greenhouse production methods have reported carbon footprints from 0.2 to 3.2 kg CO2-eq per kg edible product, while vertical farms may have between 10 and 20 kg CO2-eq per kg edible for salad. Previous studies of vertical farms from a Swedish context have also shown environmental impacts from roughly 2.6–5.3 kg CO2-eq per kg edible portion for mixed herbs and leafy greens from vertical farms (Martin et al., 2019; Barge, 2020). As such, the findings of this study are in line with these figures, and symbiotic development has been shown to reduce the impacts, where the reference scenario has a carbon footprint of 3.2 kg CO2-eq per kg edible while the best case for symbiotic development halves these emissions to roughly 1.5 kg CO2-eq per kg edible.
While the results of the photovoltaic scenarios are slightly higher than the reference scenario, such results are not unexpected in Sweden, where the energy system has low impacts. Similar findings have been outlined for different regions in Sweden, where the mitigation potential of solar microgrids had higher emissions than the regional electricity system; see e.g., Papageorgiou et al. (2020). This is also due in part to the datasets employed. Ecoinvent data available for photovoltaic energy may be outdated, although the GHG emissions of photovoltaic electricity are similar to those outlined in the aforementioned study. Despite this, and despite the minor contribution the photovoltaics may have to supply the entire demand of the system, it is important also to recognize that this would require a much larger surface. However, this may not negate the emissions associated with the photovoltaic production compared to other sources from regional electricity, i.e., the Swedish electricity mix. Recent studies have also outlined the potential of photovoltaics, suggesting it can cover the energy demand of vertical farms depending on the configuration and sizing of the growing area, see, e.g., Rehman (2021), although further research into the use of photovoltaics in urban environments is needed.
The results point to utilizing biofertilizers as an alternative to conventional fertilizers. Despite only minor reductions in the overall impacts of the vertical farm, the use of biofertilizers largely reduced impacts from the fertilizer use. While this study makes assumptions on their replacement, based primarily on comparing macronutrients, biofertilizer from anaerobic digestion has been tested by several of the regional vertical farms in the Stockholm region. Additionally, in several recent studies, biofertilizers from anaerobic digestion have also been shown to be viable alternatives with added infrastructure for nitrification for these vertical farms, see, e.g., Bergstrand et al. (2020) and Lind et al. (2021). The use of biofertilizers in vertical farms in urban environments can create new markets for regional biogas plants, as the economic viability and use of biofertilizers have been identified as a bottleneck in biogas production systems, see, e.g., Olsson and Fallde (2014) and Martin (2015).
This study outlines several synergies with brewing industry residual products. Replacing conventional soil containing a combination of coir and peat with BSG as a growing media was illustrated to reduce the environmental impacts. These results concur with those suggested in Barrett et al. (2016), who highlight that utilizing industrial by-products can improve the sustainability of soilless plant cultivation systems, and also outlined in an exploratory study by Martin et al. (2019). Furthermore, a number of studies highlight the viability of BSG and other fibrous residual products for different horticultural applications with minimal effect on the plant production (Chong, 2005; Verhagen and Boon, 2008; Christoulaki et al., 2014; Barrett et al., 2016; Chrysargyris et al., 2018). Nonetheless, the use of BSG in this study is conducted only to explore its potential and the viability has not been tested experimentally. Another promising synergy from the brewing industry is the use of carbon dioxide from fermentation for carbon enrichment. This was also illustrated to reduce environmental impacts. However, carbon dioxide extraction from the brewing industry is rare in Sweden. Despite this, ethanol producers in Sweden currently capture CO2. Carbon capture and its use for carbon enrichment for greenhouses have been conducted in other areas on industrial scales, see, e.g., Short et al. (2014).
While this study has highlighted the possibilities for the use of residual products from the brewing industry, these are not currently explored to their fullest potential. This is especially true for small-scale breweries, which have grown extensively in recent years (SBA, 2020), and may not have handling processes to deliver by-products and wastes in an efficient manner. Many large-scale brewers currently dispose of their by-products, e.g., BSG, for low-value applications, i.e., to produce animal feed or even fuel for boilers (San Martin et al., 2020). Furthermore, there are a large number of urban breweries in Sweden, which lack efficient handling methods for their by-products (Stockholm Brewing, 2021), which ultimately end up in municipal waste streams. Many of these lack efficient handling methods for their by-products, increasing the need to manage resources more sustainably. The scale of the exchange in this study, i.e., the share of BSG used annually for the vertical farm, is relatively minor. As such, effects from changing markets for BSG are considered negligible. Such flows have also been outlined in a number of studies to be ripe for valorization (Santos et al., 2003; Enweremadu et al., 2008; Gmoser et al., 2020; San Martin et al., 2020), although added processing to ensure they do not contain pathogens is critical (Mycoterra Farm, 2014) and further viability and validity of its use as a growing medium is needed. The results of the sensitivity analysis show that this would not significantly change the environmental impacts of the system as the growing media does not largely influence the overall environmental impacts.
Furthermore, while this study does not assess the economic potential of these residual streams, their use in new areas may provide new revenue streams. Still, it may also require added infrastructure and processes to upgrade these to a marketable product. As such, this may incur added costs for the vertical farms, which often struggle with economic viability (Shao et al., 2016) and may impede their development and realization to progress the industry toward synergies as outlined in this study.
The results point to energy demand, primarily electricity, for the different processes as a major influencing input. This stems largely from electricity demand from LED lighting and the HVAC system. These results concur with a number of previous studies, suggesting that energy consumption for artificially maintained climatic and light regimes contribute significantly to the environmental impacts of such systems; see, e.g., Chance et al. (2018), Romeo et al. (2018), and Martin and Molin (2019). This was also apparent in the sensitivity analysis, where the choice of electricity heavily influenced the results.
The results indicate that benefits derived from using waste heat for space heating were substantial. There are two reasons for this. First, virtually all electricity used is converted to heat (through LED waste heat, reflection, and plant evapotranspiration), which is eventually extracted through the AC unit and absorbed by the refrigerant. The majority of this heat can be used for space heating due to adequate temperature levels. As the COP of the AC unit was considered to be between 3 and 4, this leads to a higher quantity in kilowatt terms of heat theoretically available for building integration (189 630 kWh) compared with the total electricity input (130 240 kWh for LEDs and 44 640 kWh for the AC). In practice, this value is not achieved as space heating requirements are seasonal and heat transfer losses occur (106 720 kWh of heat effectively replaced). Second, in the negative impact scenarios, renewable electricity indirectly replaces heat from fossil fuels, which means the inputs are much less carbon-intensive than the replaced products. These indicative values need to be carefully considered. In this study, roughly 15% heat losses were assumed. However, this needs to be validated in practice as the heat loss through walls might be higher depending upon the building envelope. On the other hand, the replacement of hot water heating was not considered as additional heat pumps would be required to lift temperatures to adequate levels (roughly 60°C). This would increase the symbiosis potential as waste heat in summer could be partially utilized. In locations where electricity is generated by burning fossil fuels, the GHG emissions for energy would be proportionally higher (compared with ~50 g CO2/kWh assumed in this study). Similar assertions have also been highlighted for the use of heat from the vertical farm by Barge (2020). Similar findings from energy-integrated rooftops greenhouses have also been found to save energy and heating requirements for buildings (Bass and Baskaran, 2001; Sanjuan-Delmás et al., 2018; Muñoz Liesa et al., 2020).
While the study addresses building energy integration, further synergies have also been outlined in the literature to integrate vertical farms in buildings. In a recent study, Shao et al. (2021) also highlight the potential of employing vertical farms to reduce CO2 concentration and reduce ventilation energy consumption in commercial buildings. Furthermore, additional studies have also highlighted the use of optical fibers in buildings to reduce the energy consumption for LEDs; see e.g., Asiabanpour et al. (2018). Thus, further research could be focused on the potential of further integration with buildings to reduce energy and resource consumption for the farm and buildings.
Once again, the results of this study point to the benefits of including vertical farming in buildings and the potential of urban symbiosis to link with local energy systems and surrounding businesses. Such information can provide evidence to support the integration of urban agriculture and vertical farms in existing buildings and in new developments. Integrating vertical farms with their buildings can be used to progress toward decarbonizing buildings, which is needed to reduce the environmental implications of the building sector; see stipulations in Castleton et al. (2010) and Mata et al. (2022). This study also highlights the potential of utilizing residual spaces in urban areas and buildings. While a number of studies have also reviewed the use of vacant lots, few study the use of indoor vacant spaces; see, e.g., Grewal and Grewal (2012). As such, the benefits of employing residual spaces for producing food through vertical farms can add to the rental returns for real estate owners, improve the quality of the spaces, and provide new business opportunities for food production and markets (Martin and Bustamante, 2021; Shao et al., 2021), providing insights to residential building owners on the benefits of employing residual space for urban food provisioning. As the results of this study show, these systems can be multi-functional by providing energy savings for the building, offering locally produced food, utilizing and valorizing residual streams, and increasing awareness of food production in urban areas. Similar assertions have been highlighted in previous studies (Ferreira et al., 2018; Rufí-Salís et al., 2020; Arcas-Pilz et al., 2022). Such development should be encouraged in urban areas to promote urban agriculture, food systems and promote circular economy development in urban areas (Specht et al., 2014; Petit-Boix and Leipold, 2018; Arcas-Pilz et al., 2022).
The synergistic development of a vertical farm with its host building and surrounding businesses for more circular urban farming systems was found to have large potential benefits for the vertical farm. Furthermore, the benefits extend to the building host, where there are large energy savings from utilizing residual heat from the vertical farm for space heating, avoiding conventional heating sources. It was found that integration of the vertical farm with the building energy system can reduce GHG emissions of the vertical farm up to 40%. Once again, this is mainly due to the context location of the study, i.e., Sweden. Furthermore, the use of residual materials from the brewery, including spent grains and carbon dioxide, led to GHG emissions reductions of roughly 20%. The local market scenario, with a self-service location for distributing the products, and the scenario employing biofertilizer from a local biogas plant, were found to have no large reductions of GHG emissions overall for the vertical farm, despite reducing emissions from transportation and fertilizer application. Furthermore, in these scenarios, some trade-offs were identified, where added infrastructure can also increase material and water resource depletion. We also found that the results are sensitive to assumptions made on, e.g., the replaced heating sources and electricity mix.
The results from the assessment suggest that synergies with the host-building synergies and further synergies with businesses in proximity to the vertical farm can improve the material and energy efficiency of urban vertical farms. Furthermore, the results provide insights to residential building owners on the benefits of employing residual space for urban food provisioning and lowering energy demand. The knowledge produced from this study can therefore contribute to the understanding of the environmental implications of including vertical farming in urban environments and buildings to bridge disciplines such as architecture, urban design, horticulture, and industrial ecology. It provides novel results on the environmental implications of the circular development of vertical farms. However, further research should focus on more applied studies with urban farms to provide empirical evidence of the potential, viability, feasibility, and potential of additional synergies.
The original contributions presented in the study are included in the article/Supplementary Material, further inquiries can be directed to the corresponding author/s.
MM and CG conceived of the project idea and funding. MM and TW contributed to the concept of the study, its data collection and analysis, and writing of the manuscript. All authors contributed with editing and inputs for revisions.
The research has been funded through several grants. The primary financial support for the study was provided through a grant from the Strategic Innovation Programme, Viable Cities, funded through the Swedish Innovation Agency (Vinnova), Swedish Research Council for Sustainable Development (FORMAS), and the Swedish Energy Agency (Energimyndigheten). Funding for the writing and visitation of TW was provided through a grant from the Swedish Innovation Agency (Vinnova), grant 2019-03178 in the project Urban farming for resilient and sustainable food production in urban areas.
CG was employed by SWECO Architects.
The remaining authors declare that the research was conducted in the absence of any commercial or financial relationships that could be construed as a potential conflict of interest.
All claims expressed in this article are solely those of the authors and do not necessarily represent those of their affiliated organizations, or those of the publisher, the editors and the reviewers. Any product that may be evaluated in this article, or claim that may be made by its manufacturer, is not guaranteed or endorsed by the publisher.
We would like to thank the vertical farms which provided information on their farming systems. We would also like to thank our colleagues in the project for support and guidance and the reviewers for feedback leading toward the development and increased transparency of this article.
The Supplementary Material for this article can be found online at: https://www.frontiersin.org/articles/10.3389/fsufs.2022.849304/full#supplementary-material
1. ^This system may be considered small, but is common in residual spaces within Stockholm (Barge, 2020; Ljungberg, 2020).
Arcas-Pilz, V., Parada, F., Rufí-Salis, M., Stringari, G., González, R., Villalba, G., et al. (2022). Extended use and optimization of struvite in hydroponic cultivation systems. Resour. Conserv. Recycl. 179, 106130. doi: 10.1016/j.resconrec.2021.106130
Asiabanpour, B., Estrada, A., Ramirez, R., and Downey, M. S. (2018). Optimizing natural light distribution for indoor plant growth using PMMA optical fiber: simulation and empirical study. J. Renew. Energy 2018, 9429867. doi: 10.1155/2018/9429867
Axfoods. (2020). Upphämtning av matkassar boomar: Nu öppnar Willys hämtastatoner på CircleK. Available online at: https://www.axfood.se/nyhetsrum/pressmeddelanden/2020/05/upphamtning-av-matkassar-boomar–nu-oppnar-willys-hamta-stationer-pa-circle-k/ (accessed March 19, 2021).
Barge, U. (2020). Analyzing the environmental sustainability of an urban vertical hydroponic system (Master Thesis). UPTEC W 20025. Uppsala University, Uppsala, Sweden.
Barrett, G. E., Alexander, P. D., Robinson, J. S., and Bragg, N. C. (2016). Achieving environmentally sustainable growing media for soilless plant cultivation systems – a review. Sci. Horticul. 212, 220–234. doi: 10.1016/j.scienta.2016.09.030
Bass, B., and Baskaran, B. (2001). Evaluating rooftop and vertical gardens as an adaptation strategy for urban areas. NRCC Report 46737. Institute for Research in Construction, National Research Council Canada.
Benke, K., and Tomkins, B. (2017). Future food-production systems: vertical farming and controlled-environment agriculture. Sustain. Sci. Pract. Policy 13, 13–26. doi: 10.1080/15487733.2017.1394054
Bergstrand, K.-J., Asp, H., and Hultberg, M. (2020). Utilizing anaerobic digestates as nutrient solutions in hydroponic production systems. Sustainability 12, 10076. doi: 10.3390/su122310076
Bogomolova, S., Loch, A., Lockshin, L., and Buckley, J. (2018). Consumer factors associated with purchasing local vs. global value chain foods. Renew. Agric. Food Syst. 33, 33–46. doi: 10.1017/S1742170516000375
Brandao, M., Martin, M., Cowie, A., Hamelin, L., and Zamagni, A. (2017). Consequential life cycle assessment: what, how, and why? Ref. Module Earth Syst. Environ. Sci. 277–284. doi: 10.1016/B978-0-12-409548-9.10068-5
Bustamante, M. (2018). “AgTech and the city: the case of vertical farming and shaping a market for urban-produced food,” in Managing Digital Transformation BrandFactory, eds P. M. S. Anersson, M. Mähring, R. Teigland, and K. Wennberg (Sweden: Gothenburg), 281–298.
Bustamante, M. J. (2020). Using sustainability-oriented process innovation to shape product markets. Int. J. Innov. Manag. 24, 2040001. doi: 10.1142/S1363919620400010
Castleton, H. F., Stovin, V., Beck, S. B. M., and Davinson, J. B. (2010). Green roofs: building energy savings and the potential for retrofit. Energy Build. 42, 1582–1591. doi: 10.1016/j.enbuild.2010.05.004
Chance, E., Ashton, W., Pereira, J., Mulrow, J., Norberto, J., Derrible, S., et al. (2018). The plant—an experiment in urban food sustainability. Environ. Progress Sust. Energy 37, 82–90. doi: 10.1002/ep.12712
Chong, C. (2005). Experiences with wastes and composts in nursery substrates. Hort Technol. 15, 739. doi: 10.21273/HORTTECH.15.4.0739
Christoulaki, M., Gouma, S., Manios, T., and Tzortzakis, N. (2014). Deployment of sawdust as substrate medium in hydroponically grown lettuce. J. Plant Nutr. 37, 1304–1315. doi: 10.1080/01904167.2014.881870
Chrysargyris, A., Stavrinides, M., Moustakas, K., and Tzortzakis, N. (2018). Utilization of paper waste as growing media for potted ornamental plants. Clean Technol. Environ. Policy. 21, 1937–1948. doi: 10.1007/s10098-018-1647-7
Cockrall-King, J. (2012). Food and The City: Urban Agriculture and the New Food Revolution, Prometheus Books. New York, NY.
Coley, D., Howard, M., and Winter, M. (2009). Local food, food miles and carbon emissions: a comparison of farm shop and mass distribution approaches. Food Policy 34, 150–155. doi: 10.1016/j.foodpol.2008.11.001
Despommier, D. (2009). The rise of vertical farms. Sci. Am. 301, 80–87. doi: 10.1038/scientificamerican1109-80
Dong, L., Zhang, H., Fujita, T., Ohnishi, S., Li, H., Fujii, M., et al. (2013). Environmental and economic gains of industrial symbiosis for Chinese iron/steel industry: Kawasaki's experience and practice in Liuzhou and Jinan. J. Clean. Prod. 59, 226–238. doi: 10.1016/j.jclepro.2013.06.048
Dorr, E., Koegler, M., Gabrielle, B., and Aubry, C. (2021). Life cycle assessment of a circular, urban mushroom farm. J. Clean. Prod. 288, 125668. doi: 10.1016/j.jclepro.2020.125668
Dorr, E., Sanyé-Mengual, E., Gabrielle, B., Baptiste, G., and Aubry, C. (2017). Proper selection of substrates and crops enhances the sustainability of Paris rooftop garden. Agron. Sust. Dev. 37, 51. doi: 10.1007/s13593-017-0459-1
Eaves, J., and Eaves, S. (2018). Comparing the profitability of a greenhouse to a vertical farm in Quebec. Can. J. Agric. Econ. Rev. Can. Agroecon. 66, 43–54. doi: 10.1111/cjag.12161
Edwards-Jones, G., Milà I Canals, L., Hounsome, N., Truninger, M., Koerber, G., Hounsome, B., et al. (2008). Testing the assertion that “local food is best”: the challenges of an evidence-based approach. Trends Food Sci. Technol. 19, 265–274. doi: 10.1016/j.tifs.2008.01.008
Eigenbrod, C., and Gruda, N. (2015). Urban vegetable for food security in cities. A review. Agron. Sust. Dev. 35, 483–498. doi: 10.1007/s13593-014-0273-y
ElectriCity. (2021). Reference to PV installation studies in Hammarby Stort intresse för solceller i Hammarby Sjöstad. Available online at: https://electricityinnovation.se/okategoriserad/10627/ (accessed August 10, 2021).
Enweremadu, C. C., Waheed, M. A., Adekunle, A. A., and Adeala, A. (2008). The energy potential of Brewer's spent grain for breweries in Nigeria. J. Eng. Appl. Sci. 3, 175–177.
Eriksson, L., and Runevad, D. (2016). Evaluating digestate processing methods at Linköping biogas plant : A resource efficient perspective (Master Thesis). Linköping University, Linköping, Sweden.
Ferreira, A. J. D., Guilherme, R. I. M. M., Ferreira, C. S. S., and Oliveira, M. D. F. M. L. D. (2018). Urban agriculture, a tool towards more resilient urban communities? Curr. Opin. Environ. Sci. Health 5, 93–97. doi: 10.1016/j.coesh.2018.06.004
Gentry, M. (2019). Local heat, local food: integrating vertical hydroponic farming with district heating in Sweden. Energy 174, 191–197. doi: 10.1016/j.energy.2019.02.119
Gmoser, R., Fristedt, R., Larsson, K., Undeland, I., Taherzadeh, M. J., and Lennartsson, P. R. (2020). From stale bread and brewers spent grain to a new food source using edible filamentous fungi. Bioengineered 11, 582–598. doi: 10.1080/21655979.2020.1768694
Goldstein, B., Hauschild, M., Fernández, J., and Birkved, M. (2016). Testing the environmental performance of urban agriculture as a food supply in northern climates. J. Clean. Prod. 135, 984–994. doi: 10.1016/j.jclepro.2016.07.004
Graamans, L., Baeza, E., van den Dobbelsteen, A., Tsafaras, I., and Stanghellini, C. (2018). Plant factories versus greenhouses: comparison of resource use efficiency. Agric. Syst. 160, 31–43. doi: 10.1016/j.agsy.2017.11.003
Graamans, L., Van Den Dobbelsteen, A., Meinen, E., and Stanghellini, C. (2017). Plant factories; crop transpiration and energy balance. Agric. Syst. 153, 138–147. doi: 10.1016/j.agsy.2017.01.003
Grewal, S. S., and Grewal, P. S. (2012). Can cities become self-reliant in food? Cities 29, 1–11. doi: 10.1016/j.cities.2011.06.003
Harada, Y., and Whitlow, T. H. (2020). Urban rooftop agriculture: challenges to science and practice. Front. Sustain. Food Syst. 4,76. doi: 10.3389/fsufs.2020.00076
ILCD (2011). Joint Research Centre - Institute for Environment and Sustainability: International Reference Life Cycle Data System (ILCD) Handbook - Recommendations for Life Cycle Impact Assessment in the European Context. EUR 24571 EN. Luxembourg Publications Office of the European Union. doi: 10.2788/33030
Jones, J. C., and Franck, K. A. (2019). “A brewery in a foundry, a winery in a strip mall: adaptive reuse by food enterprises.” Urban Design Int. 24, 108–117. doi: 10.1057/s41289-019-00085-7
JRC (2010). International Reference Life Cycle Data System (ILCD) Handbook. General guide for Life Cycle Assessment - Detailed guidance: Joint Research Centre, Institute for Environment and Sustainability, European Commission.
Keba (2020). KePol FS-09. The Rugged Outdoor Parcel Locker With More Box' for Your Bucks. Available online at: https://www.keba.com/download/x/702c6e5184/kepol_fs-09_datasheet_en.pdf (accessed February 10, 2021).
Kim, H.-W., Dong, L., Choi, A. E., Fujii, M., Fujita, T., and Park, H.-S. (2017). Co-benefit potential of industrial and urban symbiosis using waste heat from industrial park in Ulsan, Korea. Resour. Conserv. Recycl. 135, 225–234. doi: 10.1016/j.resconrec.2017.09.027
Kozai, T., and Niu, G. (2016). “Chapter 1 - introduction,” in Plant Factory, eds T. Kozai, G. Niu, and M. Takagaki (San Diego, Academic Press), 3–5. doi: 10.1016/B978-0-12-801775-3.00001-9
Lind, O., Hultberg, M., Bergstrand, K.-J., Larsson Jönsson, H., Caspersen, S., and Asp, H. (2021). Biogas digestate in vegetable hydroponic production: pH dynamics and pH management by controlled nitrification. Waste Biomass Valoriz. 12, 123–133. doi: 10.1007/s12649-020-00965-y
Ljungberg, A. (2020). Omställning till hållbara livsmedelssystem. Kommersiell stadsodling i Stockholm (Master Thesis). UPTEC W20046, Uppsala University.
Marchi, B., Zanoni, S., and Pasetti, M. (2018). Industrial symbiosis for greener horticulture practices: the CO2 enrichment from energy intensive industrial processes. Proc. CIRP 69, 562–567. doi: 10.1016/j.procir.2017.11.117
Martin, M. (2015). Potential of biogas expansion in Sweden: identifying the gap between potential studies and producer perspectives. Biofuels 6, 233–240. doi: 10.1080/17597269.2015.1090769
Martin, M. (2020). Evaluating the environmental performance of producing soil and surfaces through industrial symbiosis. J. Indus. Ecol. 24, 626–638. doi: 10.1111/jiec.12941
Martin, M., and Bustamante, M. J. (2021). Growing-service systems: new business models for modular urban-vertical farming. Front. Sust. Food Syst. 5, 787281. doi: 10.3389/fsufs.2021.787281
Martin, M., and Harris, S. (2018). Prospecting the sustainability implications of an emerging industrial symbiosis network. Resour. Conserv. Recycl. 138, 246–256. doi: 10.1016/j.resconrec.2018.07.026
Martin, M., Heiska, M., and Björklund, A. (2021). Environmental assessment of a product-service system for renting electric-powered tools. J. Clean. Prod. 281, 125245. doi: 10.1016/j.jclepro.2020.125245
Martin, M., and Molin, E. (2019). Environmental assessment of an urban vertical hydroponic farming system in Sweden. Sustainability 11, 4124. doi: 10.3390/su11154124
Martin, M., Poulikidou, S., and Molin, E. (2019). Exploring the environmental performance of urban symbiosis for vertical hydroponic farming. Sustainability 11, 6724. doi: 10.3390/su11236724
Martin, M., Svensson, N., and Eklund, M. (2015). Who gets the benefits? An approach for assessing the environmental performance of industrial symbiosis. J. Clean. Prod. 98, 263–271. doi: 10.1016/j.jclepro.2013.06.024
Mata, É., Kihila, J. M., Wanemark, J., Cheng, S. H., Harris, S., Sandkvist, F., et al. (2022). Non-technological and behavioral options for decarbonizing buildings – a review of global topics, trends, gaps, and potentials. Sust. Prod. Consump. 29, 529–545. doi: 10.1016/j.spc.2021.10.013
McKinsey. (2020). Digital disruption at the grocery store. Available online at: https://www.mckinsey.com/industries/retail/our-insights/digital-disruption-at-the-grocery-store# (accessed March 20, 2021).
Mohareb, E., Heller, M., Novak, P., Goldstein, B., Fonoll, X., and Raskin, L. (2017). Considerations for reducing food system energy demand while scaling up urban agriculture. Environ. Res. Lett. 12, 125004. doi: 10.1088/1748-9326/aa889b
Muñoz Liesa, J., Royapoor, M., Lopez-Capel, E., Cuerva, E., Rufí-Salís, M., Gassó, S., et al. (2020). Quantifying energy symbiosis of building-integrated agriculture in a mediterranean rooftop greenhouse. Renew. Energy 156, 696–709. doi: 10.1016/j.renene.2020.04.098
Mycoterra Farm. (2014). Cultivation of gourmet mushrooms using brewer's spent grain. SARE research report FNE14-795. Available online at: https://projects.sare.org/?export=1andproject_report=475693 (accessed 12 Feb 2021).
NC. (2021). Nya Carnegie Bryggeriet. The Brewery. Available online at: https://www.nyacarnegiebryggeriet.se/en/brewery/ (accessed October 2, 2021).
Olsson, L., and Fallde, M. (2014). Waste(d) potential: a socio-technical analysis of biogas production and use in Sweden. J. Clean. Prod. 98, 107–115. doi: 10.1016/j.jclepro.2014.02.015
Orsini, F., Pennisi, G., Michelon, N., Minelli, A., Bazzocchi, G., Sanyé-Mengual, E., et al. (2020). Features and functions of multifunctional urban agriculture in the Global North: a review. Front. Sust. Food Syst. 4, 562513. doi: 10.3389/fsufs.2020.562513
Pandis Iveroth, S., Vernay, A.-L., Mulder, K. F., and Brandt, N. (2013). Implications of systems integration at the urban level: the case of Hammarby Sjöstad, Stockholm. J. Clean. Prod. 48, 220–231. doi: 10.1016/j.jclepro.2012.09.012
Papageorgiou, A., Ashok, A., Hashemi Farzad, T., and Sundberg, C. (2020). Climate change impact of integrating a solar microgrid system into the Swedish electricity grid. Appl. Energy 268, 114981. doi: 10.1016/j.apenergy.2020.114981
Petit-Boix, A., and Leipold, S. (2018). Circular economy in cities: reviewing how environmental research aligns with local practices. J. Clean. Prod. 195, 1270–1281. doi: 10.1016/j.jclepro.2018.05.281
Pulighe, G., and Lupia, F. (2020). Food first: COVID-19 outbreak and cities lockdown a booster for a wider vision on urban agriculture. Sustainability 12, 5012. doi: 10.3390/su12125012
Rehman, N. U. (2021). Vertical farms with integrated solar photovoltaics. J. Sol. Energy Eng. 144, 011007 doi: 10.1115/1.4052055
Romeo, D., Vea, E. B., and Thomsen, M. (2018). Environmental impacts of urban hydroponics in Europe: a case study in Lyon. Proc. CIRP 69, 540–545. doi: 10.1016/j.procir.2017.11.048
Rufí-Salís, M., Petit-Boix, A., Villalba, G., Gabarrell Durany, X., and Leipold, S. (2020). Combining LCA and circularity assessments in complex production systems: the case of urban agriculture. Resour. Conserv. Recycl. 166, 105359. doi: 10.1016/j.resconrec.2020.105359
S2G. (2020). S2G Ventures. Growing Beyond the Hype: Controlled Environment Agriculture (CAGR of GH market 20-25% and VF market 50% over next 5 years, 1B$ investment into VF). Available online at: https://www.s2gventures.com/reports/growing-beyond-the-hype%3A–controlled-environment-agriculture (accessed 2 December 2021).
San Martin, D., Orive, M., Iñarra, B., Castelo, J., Estevez, A., Nazzaro, J., et al. (2020). Brewers' spent yeast and grain protein hydrolysates as second-generation feedstuff for aquaculture feed. Waste Biomass Valoriz. 11, 5307–5320. doi: 10.1007/s12649-020-01145-8
Sanjuan-Delmás, D., Llorach-Massana, P., Nadal, A., Ercilla-Montserrat, M., Muñoz, P., Montero, J. I., et al. (2018). Environmental assessment of an integrated rooftop greenhouse for food production in cities. J. Clean. Prod. 177, 326–337. doi: 10.1016/j.jclepro.2017.12.147
Santos, M., Jiménez, J. J., Bartolomé, B., Gómez-Cordovés, C., and Del Nozal, M. J. (2003). Variability of brewer's spent grain within a brewery. Food Chem. 80, 17–21. doi: 10.1016/S0308-8146(02)00229-7
Sanyé-Mengual, E., Martinez-Blanco, J., Finkbeiner, M., Cerdà, M., Camargo, M., Ometto, A. R., et al. (2018). Urban horticulture in retail parks: environmental assessment of the potential implementation of rooftop greenhouses in European and South American cities. J. Clean. Prod. 172, 3081–3091. doi: 10.1016/j.jclepro.2017.11.103
SBA. (2020). Swedish Brewers Association (SBA), Bryggeristatistik. Available online at: https://sverigesbryggerier.se/statistik/ (accessed June 10, 2020).
Shao, Y., Heath, T., and Zhu, Y. (2016). Developing an economic estimation system for vertical farms. Int. J. Agric. Environ. Inform. Syst. 7, 26–51. doi: 10.4018/IJAEIS.2016040102
Shao, Y., Li, J., Zhou, Z., Hu, Z., Zhang, F., Cui, Y., et al. (2021). The effects of vertical farming on indoor carbon dioxide concentration and fresh air energy consumption in office buildings. Build. Environ. 195, 107766. doi: 10.1016/j.buildenv.2021.107766
Short, S. W., Bocken, N. M. P., Barlow, C. Y., and Chertow, M. R. (2014). From refining sugar to growing tomatoes: industrial ecology and business model evolution. J. Indus. Ecol. 18, 603–618. doi: 10.1111/jiec.12171
Specht, K., Siebert, R., Hartmann, I., Freisinger, U. B., Sawicka, M., Werner, A., et al. (2014). Urban agriculture of the future: an overview of sustainability aspects of food production in and on buildings. Agric. Hum. Val. 31, 33–51. doi: 10.1007/s10460-013-9448-4
Stockholm Exergi. (2019). Hammarbyverket. Stockholm Exergi, miljörapport 2018. Available online at: www.stockholmexergi.se/content/uploads/2019/05/Miljörapport-Hammarbyverket-2018.pdf (accessed October 10, 2020).
Strandmark, E. (2019). Biogödsel som kvävekälla i hydroponisk tomatproduktion, SLU, Sveriges lantbruksuniversitet. Fakulteten för landskapsarkitektur, trädgårds- och växtproduktionsvetenskap. Institutionen för biosystem och teknologi.
Tampio, E., Marttinen, S., and Rintala, J. (2016). Liquid fertilizer products from anaerobic digestion of food waste: mass, nutrient and energy balance of four digestate liquid treatment systems. J. Clean. Prod. 125, 22–32. doi: 10.1016/j.jclepro.2016.03.127
Tang, Z., Cenkowski, S., and Izydorczyk, M. (2005). Thin-layer drying of spent grains in superheated steam. J. Food Eng. 67, 457–465. doi: 10.1016/j.jfoodeng.2004.04.040
Thiago, R., Pedro, P., and Servulo, E. (2014). Solid wastes in brewing process: a review. J Brew Distill. 5, 1–9. doi: 10.5897/JBD2014.0043
Thomaier, S., Specht, K., Henckel, D., Dierich, A., Siebert, R., Freisinger, U. B., et al. (2014). Farming in and on urban buildings: Present practice and specific novelties of Zero-Acreage Farming (ZFarming). Renew. Agric. Food Syst. 30, 43–54. doi: 10.1017/S1742170514000143
Van Delden, S. H., Sharathkumar, M., Butturini, M., Graamans, L. J. A., Heuvelink, E., Kacira, M., et al. (2021). Current status and future challenges in implementing and upscaling vertical farming systems. Nat. Food. 2, 944–956. doi: 10.1038/s43016-021-00402-w
Velenturf, A. P. M., and Jensen, P. D. (2016). Promoting industrial symbiosis: using the concept of proximity to explore social network development. J. Indus. Ecol. 20, 700–709. doi: 10.1111/jiec.12315
Verhagen, H., and Boon, H. T. M. (2008). Classification of growing media on their environmental profile. Acta Hortic. 779, 231–238. doi: 10.17660/ActaHortic.2008.779.28
Weidner, T., Yang, A., and Hamm, M. W. (2019). Consolidating the current knowledge on urban agriculture in productive urban food systems: learnings, gaps and outlook. J. Clean. Prod. 209, 1637–1655. doi: 10.1016/j.jclepro.2018.11.004
Keywords: building integrated agriculture, vertical farming (VF), industrial symbiosis (IS), urban symbiosis, circular economy (CE), urban food, life cycle assessment (LCA), urban agriculture
Citation: Martin M, Weidner T and Gullström C (2022) Estimating the Potential of Building Integration and Regional Synergies to Improve the Environmental Performance of Urban Vertical Farming. Front. Sustain. Food Syst. 6:849304. doi: 10.3389/fsufs.2022.849304
Received: 05 January 2022; Accepted: 02 March 2022;
Published: 29 March 2022.
Edited by:
Monika Egerer, Technical University of Munich, GermanyReviewed by:
Sergiy Smetana, German Institute of Food Technologies, GermanyCopyright © 2022 Martin, Weidner and Gullström. This is an open-access article distributed under the terms of the Creative Commons Attribution License (CC BY). The use, distribution or reproduction in other forums is permitted, provided the original author(s) and the copyright owner(s) are credited and that the original publication in this journal is cited, in accordance with accepted academic practice. No use, distribution or reproduction is permitted which does not comply with these terms.
*Correspondence: Michael Martin, bWljaGFlbC5tYXJ0aW5AaXZsLnNl
Disclaimer: All claims expressed in this article are solely those of the authors and do not necessarily represent those of their affiliated organizations, or those of the publisher, the editors and the reviewers. Any product that may be evaluated in this article or claim that may be made by its manufacturer is not guaranteed or endorsed by the publisher.
Research integrity at Frontiers
Learn more about the work of our research integrity team to safeguard the quality of each article we publish.