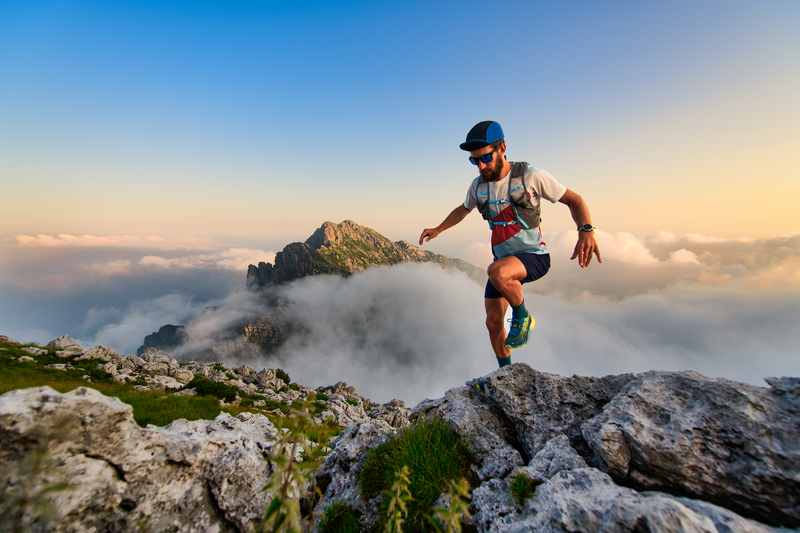
95% of researchers rate our articles as excellent or good
Learn more about the work of our research integrity team to safeguard the quality of each article we publish.
Find out more
ORIGINAL RESEARCH article
Front. Sustain. Food Syst. , 13 July 2022
Sec. Waste Management in Agroecosystems
Volume 6 - 2022 | https://doi.org/10.3389/fsufs.2022.838635
This article is part of the Research Topic Upcycling Organic Waste for the Sustainable Management of Soilborne Pests and Pathogens in Agri-Food Systems View all 15 articles
Anaerobic soil disinfestation (ASD) has been demonstrated as an effective alternative to pre-plant chemical soil fumigation (CSF) commonly used to control soilborne pathogens. However, the ASD effects on spatial and temporal changes in soil microbial communities remain poorly understood in production systems with low soilborne disease pressure. The objective of this study was to assess the influence of ASD treatments on soil microbial community composition at different soil depths during the spring tomato production season in Florida. Soil treatments included ASD using 6.9 m3 ha−1 of molasses with 11 Mg ha−1 of composted poultry litter (CPL) (ASD0.5), ASD with 13.9 m3 ha−1 of molasses and 22 Mg ha−1 CPL (ASD1.0), and chemical soil fumigation (CSF) using a mixture of 1,3-dichloropropene and chloropicrin. Soil microbial community composition was measured at soil depths of 0–15 and 15–30 cm using phospholipid fatty acid (PLFA) analysis at 0, 36, 76, and 99 days after transplanting (DAT). Fatty acid methyl esters were categorized into biomarker groups including total microbial biomass (TMB), G+ bacteria (G+), G− bacteria (G−), actinomycetes (Actino), arbuscular mycorrhizal fungi (AMF), protozoa, and general fungi (F). Soil concentrations of G+, Actino, F, AMF, and the ratio of F:bacteria (B) were significantly impacted by a soil treatment × soil depth × sampling time three-way interaction. All the microbial biomarkers were significantly affected by soil treatment × sampling depth two-way interactions except for protozoa and F:B ratio. Concentrations of TMB, Actino, AMF, F, G+, and G− bacteria were significantly increased in ASD treated soils at both 0–15 and 15–30 cm soil depths across different sampling times compared with CSF. In addition, the concentrations of G+ and G− bacteria, AMF, F, and TMB were higher at 0–15 vs. 15–30 cm soil depth under ASD treatments, whereas no soil depth differences were observed in CSF. Discriminant analysis further confirmed that soil microbial community composition was distinctly different in CSF compared with ASD treatments. The soil microbial profile was well-differentiated between the two soil depths under ASD treatments but not in CSF, while the enhancement of PLFA biomarkers by ASD decreased with increasing soil depth.
Tomato (Solanum lycopersicum) is an important high-value vegetable crop worldwide. In the United States, Florida ranks first in the production of fresh market tomato with a reported production area of 9,308 ha and production value of over $323 million [United States Department of Agriculture, National Agricultural Statistics Service (USDA-NASS), 2022]. Following the phase-out of methyl bromide for soil fumigation, alternative chemical soil fumigants have been identified for use in tomato production prior to field transplanting to aid in suppression of weeds and soilborne pathogens. However, fumigants such as 1,3-dichloropropene (Group II, halogenated hydrocarbon) are subject to stringent environmental regulations and often lack adequate efficacy (Poret-Peterson et al., 2019). Commonly used pre-and post-plant non-triazine herbicides including glyphosate, acetolactate synthase (ALS) inhibitors, and acetyl coenzyme A carboxylase (ACC) inhibitors have resulted in rapid growth of selective herbicide resistance (LeBaron and Hill, 2008). In addition, increasing public health awareness and consumer demand for sustainable food products provide a unique incentive for the development of environmentally sustainable agricultural practices. Thus, to promote the long-term sustainability of vegetable production systems, there is a need to explore non-chemical alternatives for managing soilborne pests (Rosskopf et al., 2005, 2020; Shi et al., 2019) while carefully considering economic viability, environmental impact, and social acceptability.
Anaerobic soil disinfestation (ASD) has been reported as an effective, environmentally benign alternative to pre-plant chemical soil fumigation for managing soilborne pathogens in various high-value crops across a range of production systems (Momma et al., 2013; Mazzola et al., 2018). The ASD method involves incorporation of a labile carbon (C) source, followed by irrigation to saturate soil pores and covering the soil with gas impermeable film for ~3 weeks (Butler et al., 2014; Paudel et al., 2020). Some commonly used C sources include rice or wheat bran (Strauss and Kluepfel, 2015), liquid or dried molasses (Butler et al., 2012b; Shrestha et al., 2018), and ethanol (Momma et al., 2010). In greenhouse studies, Butler et al. (2012b) also investigated the use of some warm-season cover crops as a C source for ASD on the suppression of Fusarium oxysporum, yellow nutsedge (Cyperus esculentus) tubers, and root-knot nematode (Meloidogyne incognita) eggs and juveniles. In Florida, ASD has been successfully demonstrated to manage weeds, plant parasitic nematodes, and some soilborne pathogens in eggplant (Solanum melongena), pepper (Capsicum annuum), tomato, and strawberry (Fragaria × ananassa) production while improving crop yield (Butler et al., 2012a,b; Di Gioia et al., 2016, 2020; Guo et al., 2017; Paudel et al., 2020). The mechanisms of ASD are likely related to shifts in soil community composition from aerobic organisms to facultative and obligate anaerobic organisms, production of volatile organic compounds, release of organic acids, and generation of metal ions (Momma, 2008; Strauss and Kluepfel, 2015; Hewavitharana et al., 2019; Rosskopf et al., 2020). However, information regarding the response of soil microbial communities to ASD during the cropping season remains limited.
van Agtmaal et al. (2015) assessed the impact of stress-induced changes in soil microbial community composition on microbially produced volatile organic compounds (VOCs) for suppression of Pythium intermedium in the production of hyacinth flower bulbs using pyrosequencing of 16S ribosomal gene fragments. At 3 months after ASD treatment, an increase in the relative abundance of the phylum Bacteroidetes and a significant decrease of the phyla Acidobacteria, Planctomycetes, Nitrospirae, Chloroflexi, and Chlorobi were observed. In two separate field studies, Poret-Peterson et al. (2019) also investigated shifts in bacterial communities after ASD soil treatments with different C sources. In their study, ASD treatments using molasses, mustard seed meal, tomato pomace, and rice bran led to increases in the abundances of Bacteroidales, Clostridiales, Selenomonadales, and Enterobacteriales compared with untreated controls. It was also found that the phylogenetic and taxonomic composition of communities in ASD treated soils with different C sources did not show pronounced differences. However, the authors did not investigate in-season microbial community composition dynamics, as no crops were grown during their study. In a previous study using phospholipid fatty acid (PLFA) analysis, Guo et al. (2018) examined the influence of two ASD soil treatments [6.9 m3 ha−1 molasses (M) + 11 Mg ha−1 composted poultry litter (CPL) and 13.9 m3 ha−1 M + 22 Mg ha−1 CPL] in contrast with chemical soil fumigation (CSF; a mixture of 1,3-dichloropropene and chloropicrin) on dynamic changes of soil microbial communities at 0–15 cm soil depth in a tomato production system to identify possible legacy effects of ASD on soil microbial community composition during the tomato growing season. It was observed that soil microbial groups were depleted in CSF treatment compared with ASD treatments in bulk and rhizosphere soils, while the composition of soil microbial communities was similar between ASD treated soils. Additionally, greater concentrations of total microbial biomass (TMB), actinomycetes, and G− bacteria were detected in ASD treated soils as opposed to CSF at 0, 36, 76, and 99 days after tomato transplanting. However, it is unclear whether and how the impact may be altered at soil depths beyond 0–15 cm. Therefore, the objective of this follow-up study was to compare the spatial and temporal changes of soil microbial communities between 0–15 and 15–30 cm soil depths in response to ASD soil treatments during the field-tomato production season in an effort to clarify possible legacy effects on soil microbial community composition.
The field experiment was conducted at the University of Florida Plant Science Research and Education Unit in Citra, FL from August to December 2015, with the soil type as Gainesville loamy sand (Hyperthermic, coated Typic Quartzipsamments). The field had prominent levels of weed infestation (primarily nutsedge) and root-knot nematodes (Guo et al., 2018). A thorough rototilling at 15 cm below the soil line was conducted in the experimental plots at the time of field preparation. The field trial was arranged in a split plot design with four replications. The pre-plant soil treatments were included in the whole plots following a randomized complete block design with four blocks, with herbicide treatments in the subplots. The soil treatments consisted of ASD with 6.9 m3 ha−1 of molasses (Agricultural Carbon Source, TerraFeed, LLC, Plant City, FL, USA) and 11 Mg ha−1 of composted poultry litter (CPL) (ASD0.5), ASD with 13.9 m3 ha−1 of molasses and 22 Mg ha−1 (ASD1.0), and chemical soil fumigation [CSF; Pic-Clor 60 (Soil Chemical Corporation, Hollister, CA, USA) applied at 224 kg ha−1, containing 1,3-dichloropropene (39.0%) and chloropicrin (59.6%)]. The herbicide treatments included application of halosulfuron-containing Sandea® (Gowan Company, Yuma, AZ, USA) with the rate of 70 g ha−1 and the no-herbicide control.
Three raised beds (24.4 m long, 0.9 m wide, 0.30 m high, and 1.8 m between centers) were made in each of the four blocks (replications) on 26 September 2018 and randomly assigned to ASD0.5, ASD1.0, or CSF. Each bed received an application of the pre-plant compound fertilizer (10N-10P2O5-10K2O) at a rate of 560 kg ha−1. A 1:1 (v:v) water dilution of molasses and CPL was used to set up ASD0.5 and ASD1.0 treatments. The mixture was applied to the top of the bed and tilled at the soil depth of 15 cm using a rotary cultivator, evenly amending the soil. The 24.4 m-long bed was divided in two 12.2 m-long sections (each serving as a subplot) for each whole plot. A random assignment of the herbicide Sandea® treatment and the no-herbicide control were applied in each half of the bed plots. Following application of the herbicide, the CSF treatment was applied via shank injection. A 0.025 mm white (on black) VaporSafe® TIF (Raven Industries Inc., Sioux Falls, SD, USA) polyethylene mulch with an ethylene vinyl alcohol (EVOH) barrier layer was used to cover all the beds. Each bed was irrigated through two drip lines positioned about 2.5 cm beneath the soil surface under the mulch. The beds undergoing ASD were the only treatments irrigated one time applying 68.9 kPa water pressure for a 4-h period. The soil pore space in the upper 10 cm of the bed (5-cm irrigation) was saturated to promote anaerobic conditions (Butler et al., 2012a). Tomato transplanting took place 3 weeks after the soil treatments were initiated.
On 3 September, 2015, tomato cultivar ‘Tribute’ (Sakata Seed America, Morgan Hill, USA) was transplanted at the four-true-leaf stage. Twenty-six plants with in-row spacing of 0.45 m were planted per subplot. A timer-controlled drip irrigation system was used to water plants twice daily. Initially, irrigation time was set to 30 min and later adjusted as plants matured. A weekly injection through the drip tape of fertilizer 6N-0P2O5-8K2O plus micro blend (2% Ca, 0.4% Mg, 0.02% Zn, and 0.02% B; Mayo Fertilizer Inc., Mayo, FL, USA) began 7 days after transplanting (DAT), with in-season application rates of N and K2O by fertigation at 161 and 215 kg ha−1, respectively.
Bulk soil samples were collected four times from the soil depth of 0–15 and 15–30 cm for soil microbial analysis during the tomato season: 3 September, 2015 (0 DAT), 9 October, 2015 (36 DAT), 18 November, 2015 (76 DAT), and 11 December, 2015 (99 DAT). Six bulk soil samples were collected from each subplot using a handheld soil probe (1.75 cm internal diameter) at each sampling time. The six soil samples were then combined and homogenized and kept at −20°C until microbial community analysis.
Soil microbial communities for each treatment were characterized using PLFA analysis outlined by Guo et al. (2018). All collected soil samples passed through a 2-mm sieve to remove root and fresh litter materials, and then were freeze-dried before further analysis. Fatty acid methyl esters (FAMEs) were extracted from the freeze-dried soil samples using high throughput procedures described by Buyer and Sasser (2012). After thawing to room temperature, samples were used to extract PLFAs. A Bligh-Dyer extractant (chloroform/methanol/phosphate buffer, 1:2:0.8, v/v/v, 50 mM, pH 7.4; 4.0 mL) with an internal standard 19:0 (1,2-Dimyristoyl-sn-glycero-3-phosphocholine) was used for PLFA extraction. Lipid classes were isolated by solid phase extraction (SPE) with a 96-well SPE plate containing 50 mg of silica per well (Phenomenex, Torrance, CA, USA). The FAMEs were analyzed using an Agilent 7890N gas chromatography system (Agilent Technologies, Wilmington, DE, USA), which was equipped with an autosampler and flame ionization detector, and was controlled with MIDI Sherlock® software and Agilent ChemStation (Microbial ID, Inc., Newark, DE, USA). The FAMEs were classified and placed into six biomarker groups: Gram positive (G+) bacteria, iso and anteiso saturated branched fatty acids; Gram negative (G−) bacteria, monounsaturated fatty acids, and cyclopropyl 17:0 and 19:0; actinomycetes, 10-methyl fatty acids; arbuscular mycorrhizal fungi (AMF), 16:1ω5c; general fungi, 18:2ω6c; protozoa, 20:3ω6c and 20:4ω6c. Total microbial biomass (TMB) was determined as a sum of all quantified PLFAs in each sample. The concentrations of different biomarker groups and total microbial biomass in the soil were expressed in the unit of nmol PLFAs g−1 soil. In addition, the ratios of fungi:bacteria (F:B) and G+ bacteria:G− bacteria (G+:G−) were calculated.
Prior to statistical analysis, data were checked for normality and log transformed when necessary to meet assumptions of linear mixed models. All data and results demonstrated in tables and figures present non-transformed values. Data were analyzed using a linear mixed model in the GLIMMIX procedure in SAS (Version 9.3; SAS Institute, Cary, NC, USA). Soil treatment, herbicide treatment, sampling time, and soil depth were analyzed as fixed effects. Block and soil treatment, soil treatment × herbicide treatment, soil treatment × herbicide treatment × sampling time within the block were considered as random effects. Soil treatment × herbicide treatment × soil depth within the block was analyzed as random residual effects by fitting a first-order autoregressive [AR(1)] model to account for the repeat measures of sampling time, using the “slice” statement to compare the composition of soil microbial communities over time for each soil depth. Multiple comparisons for each microbial biomarker were conducted using Tukey's Honest Significant Difference (HSD) test at α = 0.05. The influence of soil treatment and soil depth combinations on soil microbial community structure was further examined using discriminant analysis (DA; JMP V.15.0.0; SAS Institute). Specific microbial biomarkers with the greatest impact on treatment segregation were identified with DA. Canonical discriminant analysis was performed on the ensuing discriminant model. The quantity of elements used to differentiate treatment groups was found through the number of significant (P ≤ 0.05) canonical discriminant functions (linear combinations of important microbial biomarkers identified in discriminant analysis).
Herbicide application had no significant main effect on any of the soil microbial biomarkers measured, while soil treatment significantly impacted all PLFA biomarker groups (Table 1). Soil depth showed significant main effects on all the microbial parameters except for G+:G− bacteria ratio and actinomycetes, while sampling time showed significant effects on all microbial biomarkers except for protozoa. Each of the PLFA biomarkers were significantly affected by the soil treatment × soil depth interaction except for F:B ratio and protozoa. The soil treatment × sampling time interaction had significant effects on all the biomarkers except for TMB and G− bacteria. Only protozoa were significantly affected by the soil treatment × soil herbicide interaction (Table 1). Furthermore, concentrations of G+ bacteria, actinomycetes, general fungi, AMF, and F:B ratio during the tomato production season were significantly influenced by a three-way interaction of soil treatment, soil depth, and sampling date (Tables 1, 2).
Table 1. Analysis of variance of the effects of soil treatment, soil depth, sampling time, and herbicide application, and their two-way and three-way interactions on microbial group concentrations.
Table 2. Microbial biomarker concentrations (nmol g−1) and ratio of fungi:bacteria in the bulk soil as affected by the three-way interaction of soil treatment, sampling date, and soil depth.
Within 0–15 cm soil depth, both ASD treatments had significantly higher levels of G+ bacteria compared with CSF at all DATs, whereas no significant differences were observed between ASD 0.5 and ASD 1.0 at each DAT (Table 2). Similarly, there were no significant differences between the two ASD treatments at each DAT within 15–30 cm soil depth, but the ASD treatments led to significantly higher levels of G+ bacteria at all DATs except for 99 DAT when compared with CSF. When comparing G+ bacteria concentrations between the two soil depths within each soil treatment, both ASD treatments showed significantly higher levels at 0–15 cm compared with 15–30 cm soil depth at all DATs, but no differences were found between depths in CSF (Table 2).
The main effects of soil treatment, soil depth, and sampling time were significant for the concentrations of G− bacteria, while only the soil treatment × soil depth interaction significantly affected G− bacteria (Table 1). Both ASD treatments showed significantly higher concentrations of G− bacteria at 0–15 cm soil depth compared with 15–30 cm soil depth, while no significant differences between soil depths were observed for CSF (Figure 1A). At both soil depths, both ASD treated soils showed higher concentrations of G− bacteria compared with CSF.
Figure 1. Bulk soil PLFA concentrations of G− bacteria (A), ratio of G+:G− (B), and PLFA concentrations of TMB (C) as affected by the two-way interaction of soil treatment and soil depth across sampling dates. Error bars represent standard error. Within a soil treatment, bars sharing the same uppercase letter are not significantly different at P ≤ 0.05 according to Tukey's HSD test. Within a sampling depth, bars sharing the same lowercase letter are not significantly different at P ≤ 0.05 according to Tukey's HSD test. CSF, chemical soil fumigation control with Pic-Clor 60 at a rate of 224 kg ha−1; ASD0.5, anaerobic soil disinfestation with 6.9 m3 ha−1 of molasses and 11 Mg ha−1 of composted poultry litter; ASD1.0, anaerobic soil disinfestation with 13.9 m3 ha−1 of molasses and 22 Mg ha−1 of composted poultry litter. G−, Gram negative bacteria; G+:G−, ratio of Gram positive bacteria to Gram negative bacteria; TMB, total microbial biomass.
Regarding G+:G− bacteria ratio, at 0 DAT, it was significantly higher in CSF compared with ASD0.5, but it was similar between CSF and ASD1.0 (Figure 2A). However, the ratio of G+:G− bacteria were significantly greater in CSF treatment compared to both ASD treatments at the other DATs. There were no significant differences between ASD0.5 and ASD1.0 at 0, 36, and 76 DAT, whereas ASD0.5 showed significantly higher concentration of G+:G− bacteria compared with ASD1.0 at 99 DAT. Within each soil treatment, it showed significantly higher ratio of G+:G− bacteria at 0 DAT compared to the other DATs (Figure 2A). The ratio of G+:G− bacteria did not significantly differ between soil depths under ASD0.5, ASD1.0, and CSF, respectively (Figure 1B). Within both soil depths, CSF exhibited a higher ratio of G+:G− bacteria compared with ASD0.5 and ASD1.0, while the difference between CSF and ASD1.0 appeared to be greater at the soil depth of 15–30 vs. 0–15 cm.
Figure 2. Ratio of G+:G− (A) and PLFA concentrations of protozoa (B) in the bulk soil. As affected by two-way interaction of soil treatment and sampling date across soil depths. Error bars represent standard error. Within a soil treatment, bars sharing the same uppercase letter are not significantly different at P ≤ 0.05 according to Tukey's HSD. Within a sampling date, bars sharing the same lowercase letter are not significantly different at P ≤ 0.05 according to Tukey's HSD test. CSF, chemical soil fumigation control with Pic-Clor 60 at a rate of 224 kg ha−1; ASD0.5, anaerobic soil disinfestation with 6.9 m3 ha−1 of molasses and 11 Mg ha−1 of composted poultry litter; ASD1.0, anaerobic soil disinfestation with 13.9 m3 ha−1 of molasses and 22 Mg ha−1 of composted poultry litter. G+:G−, ratio of Gram positive bacteria to Gram negative bacteria; DAT, days after transplanting.
In terms of protozoa, soil treatment and soil depth showed significant effects, while the interaction effect of soil treatment × sampling time was significant as well (Table 1). At 0, 36, and 99 DAT, the concentrations of protozoa in ASD0.5 and ASD1.0 were significantly higher compared with CSF (Figure 2B). The concentration of protozoa steadily decreased from 0 to 99 DAT under ASD0.5, resulting in a significant difference between 0 and 99 DAT. However, there were no significant differences among different DATs for ASD1.0 and CSF treatments (Figure 2B). Across soil treatments and sampling dates, the comparison between the two soil depths revealed a significantly higher concentration of protozoa within the 0–15 cm soil depth than in the deeper soil at 15–30 cm (data not shown).
With respect to the concentration of actinomycetes, within both 0–15 and 15–30 cm soil depths, ASD0.5 had significantly higher levels of actinomycetes than CSF at each DAT, while ASD1.0 showed significantly higher levels at 36, 76, and 99 DAT (Table 2). Only at 0 DAT, ASD0.5 showed a significantly higher level of actinomycetes compared with ASD1.0 at both soil depths. When comparing the levels of actinomycetes between the two soil depths, higher levels were found at 0–15 vs. 15–30 cm in ASD0.5 at 36 DAT and ASD1.0 at 99 DAT, but no significant differences were observed in CSF at each DAT (Table 2).
The concentration of AMF was positively affected by both ASD soil treatments. Although no significant differences were observed at 0 DAT, the concentrations of AMF in the ASD1.0 and ASD0.5 treated soils were significantly higher compared with CSF at both soil depths at 36, 76, and 99 DAT (Table 2). Furthermore, at each sampling date the concentration of AMF under CSF did not differ significantly between soil depths, whereas higher concentrations of AMF were observed at the 0–15 cm soil depth compared with 15–30 cm soil depth under both ASD treatments at 36, 76, and 99 DAT. At 0 DAT, higher concentrations of AMF were observed at the 15–30 cm soil depth compared with 0–15 cm soil depth for ASD1.0.
The concentrations of general fungi significantly increased at 0 DAT in both ASD soil treatments compared with CSF at the 0–15 cm soil depth, while no differences were observed at later sampling dates. At the 15–30 cm soil depth, the concentrations of general fungi did not differ significantly among soil treatments at each DAT (Table 2). With respect to the comparison between soil depths, significantly higher levels of fungi were observed at 0–15 cm than at 15–30 cm under both ASD treatments at each DAT, while such a difference was only observed at 36 and 99 DAT for CSF (Table 2).
Under CSF, the F:B ratio significantly increased from 0 to 36 DAT and then remained relatively stable until 99 DAT at both 0–15 and 15–30 cm soil depths (Table 2). No significant changes were observed in the F:B ratio under the two ASD treatments despite soil depth and DAT except that a significant increase from 0 to 36 DAT was found at the 15–30 cm soil depth in ASD1.0 (Table 2). The ratio of F:B was significantly greater at 0–15 vs. 15–30 cm soil depth at 0, and 76 DAT for ASD0.5, while a similar trend was observed in ASD1.0 at 0 and 99 DAT, and in CSF at 36 and 99 DAT (Table 2).
The soil treatment × soil depth interaction had a significant impact on TMB (Table 1; Figure 1C). Across all sampling dates, both ASD treatments demonstrated significantly higher levels of TMB at the 0–15 cm soil depth in contrast to the deeper soil at 15–30 cm, whereas no difference between the soil depths was observed in CSF. The two ASD treatments resulted in significantly greater concentrations of TMB than CSF at both soil depths, but to a lesser extent in the deeper soil at 15–30 cm (Figure 1C). The main effect of sampling time also significantly impacted TMB as reflected by a significantly higher concentration of TMB at 0 DAT than that at 36, 76, and 99 DAT, and no significant differences were observed at these 3 later sampling dates (data not shown).
Different microbial biomarkers (Table 1) were included in the discriminant analysis to characterize changes in overall soil microbial community structure in response to the interaction of soil treatment and soil depth. Canonical discriminant analysis indicated two significant discriminant functions accounting for 94.0% of the total variance. The first canonical axis explained 64.5% of the variability, while 29.5% of the variability was explained by the second canonical axis (Figure 3). Microbial biomarkers positively correlated to the first canonical component included G+ bacteria, G− bacteria, and actinomycetes (in order of strongest to weakest correlation). In contrast, a negative correlation was observed between the first canonical component and TMB, ratio of G+:G− bacteria, AMF, fungi, protozoa, and F:B (in order of strongest to weakest correlation). The second canonical component was positively correlated with G− bacteria, ratio of G+:G− bacteria, fungi, TMB, and protozoa (in order of strongest to weakest correlation), while it was negatively correlated with G+ bacteria, actinomycetes, AMF, and F:B (in order of strongest to weakest correlation).
Figure 3. Canonical discriminant analysis of PLFA biomarkers for the two-way interaction of soil treatment and soil depth across sampling dates. Vectors represent standardized canonical coefficients and indicate the relative contribution of each biomarker group to each canonical variate. Ellipses represent 95% confidence region of the mean. CSF, chemical soil fumigation control with Pic-Clor 60 at a rate of 224 kg ha−1; ASD0.5, anaerobic soil disinfestation with 6.9 m3 ha−1 of molasses and 11 Mg ha−1 of composted poultry litter; ASD1.0, anaerobic soil disinfestation with 13.9 m3 ha−1 of molasses and 22 Mg ha−1 of composted poultry litter. G+:G−, ratio of Gram positive bacteria to Gram negative bacteria; F:B, ratio of fungi:bacteria; TMB, total microbial biomass; G+, Gram positive bacteria; Prot, protozoa; G−, Gram negative bacteria; Actino, actinomycetes; AMF, arbuscular mycorrhizal fungi; ASD0.5 d1, ASD0.5 at 0–15 cm soil depth; ASD0.5 d2, ASD0.5 at 15–30 cm soil depth; ASD1.0 d1, ASD1.0 at 0–15 cm soil depth; ASD1.0 d2, ASD1.0 at 15–30 cm soil depth; CSF d1, chemical soil fumigation control at 0–15 cm soil depth; CSF d2, chemical soil fumigation control at 15–30 cm soil depth.
The canonical discriminant analysis results further demonstrated that the microbial community composition characterizing the two ASD treatments were clearly different from that of CSF (Figure 3). Particularly, TMB, G+ bacteria, and G− bacteria were the key biomarkers differentiating CSF from ASD0.5 and ASD1.0 along canonical axis 1. CSF at both soil depths showed similar microbial biomarker characteristics according to canonical axis 1 and canonical axis 2, whereas the ASD treatments at 0–15 and 15–30 cm soil depths were well-separated along canonical axis 2. Moreover, the difference in microbial biomarkers between ASD0.5 and ASD1.0 appeared to be more pronounced at the soil depth of 0–15 cm than at 15–30 cm. The G+ bacteria played a more important role among other biomarkers in differentiating the two ASD treatments, while the G− bacteria tended to be more important in differentiating soil microbial composition between the two soil depths across the ASD treatments.
Soil fumigants are used extensively in Florida to manage soilborne pests and pathogens prior to growing strawberries, tomatoes, and other high-value crops. Although the effects of fumigants on beneficial non-target organisms at the field scale remain largely unknown (Jackson et al., 2013; Liu et al., 2015), previous studies have demonstrated fumigants including dimethyl disulfide (DMDS), Telone (1,3- dichloropropene or 1,3-D), and chloropicrin (CP) exhibit broad biocidal activity against non-target soil organisms (Dangi et al., 2015). The present study showed that shank-injected Pic-Clor 60 at a rate of 224 kg ha−1 to a soil depth of 30 cm (CSF) resulted in a reduction in the relative abundance of several soil microbial PLFA biomarkers. In general, concentrations of TMB, actinomycetes, AMF, G+, and G− bacteria, and protozoa were reduced at both 0–15 and 15–30 cm soil depths under CSF compared with ASD treated soils. Specifically, concentrations of protozoa under ASD0.5 and ASD1.0 increased exponentially at 0–15 cm soil depth compared with CSF, while concentrations of G− bacteria increased by more than 200% at 0–15 cm soil depth under ASD treated soils compared with CSF. At 15–30 cm soil depth, concentration of G− bacteria increased by 83% under ASD0.5 and by 108% under ASD1.0 compared with CSF. These results agree with findings from Dangi et al. (2015) who reported that microbial communities including G+ bacteria, G− bacteria, fungi, and AMF under fumigated soils were significantly lower compared with non-fumigated control plots. Other studies have also observed a decline in total microbial biomass after fumigation (Klose et al., 2006; Ge et al., 2021). However, previous studies on shifts in bacterial populations are inconsistent. While Yao et al. (2006) suggested G− bacteria may recover more rapidly following fumigation, others have reported that concentrations of G+ bacteria recover preferentially (Ibekwe et al., 2001). These inconsistencies in the literature may also be related to the availability and diversity of C-rich substrates (Hewavitharana et al., 2019).
The ratio of G+:G− bacteria was significantly greater under CSF vs. ASD treatments (except for the similar level between CSF and ASD1.0 at 0 DAT) at both soil depths, which may be primarily linked to the marked increase of G− bacteria populations in the ASD soil treatments. These results are in line with a previous study by Breulmann et al. (2014) who reported an increase in G+:G− bacteria ratio with decreasing labile C substrates along the soil profile. Gram negative bacteria generally utilize more labile, plant derived C sources, while G+ bacteria use C sources derived from soil organic matter or recalcitrant sources (Fanin et al., 2019). Thus, the structure and function of soil microbial composition are affected by substrate availability. In the present study, CSF soil treatment did not receive composted poultry litter or molasses as a source of mineral N and labile C substrate, respectively. Gram negative bacteria exhibit r-selected Monod growth kinetics, enabling rapid growth and reproduction in nutrient rich environments. It is likely that G− bacteria concentrations were promoted by the addition of molasses and CPL. In addition, some studies have reported on the influence of soil nutrient availability on soil microbial community composition. For example, Demoling et al. (2008) demonstrated that the addition of N changed microbial community composition compared with unfertilized plots. In their study, fungal growth rates were less negatively affected by fertilization compared with bacterial growth rates, while overall fungal biomass decreased more compared with bacterial biomass as a result of N fertilization. In our study, the addition of CPL and molasses contributed 372.5 and 745 kg ha−1 N and 4,907.5 and 9,815 kg ha−1 C for ASD0.5 and ASD1.0, respectively (Di Gioia et al., 2017). Thus, the observed differences in soil microbial community composition could be linked to creating an anaerobic environment during the ASD treatment period and the change in soil C and nutrient pools resulting from the incorporation of organic amendments.
The top 30 cm of soil is generally considered to be the root zone of many horticultural crops. The ability of soil microorganisms to establish and function within this zone after soil treatment is critical for maintaining productive soils. The canonical discriminant analysis revealed that for either ASD treatment, the soil microbial profile was well-differentiated between the two soil depths, whereas there was a lack of differentiation in the CSF treatment. In general, the concentrations of G+ and G− bacteria, AMF, fungi, and TMB were higher at 0–15 cm compared with 15–30 cm soil depth under ASD treatments, whereas no consistent differences were observed between 0–15 and 15–30 cm soil depth under CSF treatment. Regardless of soil treatment, the level of protozoa also decreased with increasing soil depth. One previous study that examined the effects of ASD in tree-crop nursery conditions in California reported soil microbial community changes as a function of soil depth (down to 76.2 cm) in ASD treated soils when the C source was only incorporated to a soil depth of 15.2–20.3 cm. The reduction of soilborne plant pathogens was significantly greater at the 15.2 cm soil depth, likely due to enhanced microbial metabolic activity resulting from the higher concentration of the C source at that soil depth (Strauss et al., 2017). This might be the similar case in our study, as molasses and CPL amended to a soil depth of ~15 cm, thus higher concentrations of several microbial biomarkers at 0–15 cm soil depth were observed.
The investigation of soil microbial community composition following ASD treated soils compared with fumigated soils at different soil depths is not well-documented in the literature. In a previous study, Guo et al. (2018) reported soil microbial community structure differed substantially between ASD and fumigated soils at 0–15 cm soil depth. Similarly, in the present study, canonical discriminant analysis of PLFA microbial biomarkers in CSF and ASD soil treatments at 0–15 and 15–30 cm soil depths clearly indicates differences in soil microbial community composition between ASD treated soils and fumigated soil. Soil microbial communities in ASD treated soils were distinctly different from fumigated plots, regardless of soil depth. The difference in soil microbial community structure between ASD and fumigated soils may be due to high toxicity of many fumigants to soil organisms (Ibekwe et al., 2001), while the addition of CPL and labile C sources in ASD soils likely promote a greater abundance of soil microbial populations (Guo et al., 2018). Mazzola et al. (2018) also observed distinctly different bacterial and fungal communities in ASD treated soils using rice bran at 20 Mg ha−1 or molasses at 20 Mg ha−1 compared with methyl bromide-chloropicrin soil fumigation. However, in their study soil samples were examined only to a depth of 0–15 cm.
In the present study, the concentration of selected microbial biomarkers including G+ bacteria, actinomycetes, general fungi, arbuscular mycorrhizal fungi, and fungi:bacteria ratio were impacted by the three-way interaction of soil treatment, sampling time, and soil depth while all of the microbial biomarkers were affected by the interaction of soil treatment and soil depth except protozoa and fungi:bacteria ratio. In general, ASD treatments increased the overall abundance of total microbial biomass, actinomycetes, arbuscular mycorrhizal fungi, protozoa, G+, and G− bacteria compared with CSF at both 0–15 and 15–30 cm soil depths across multiple sampling dates. Moreover, the concentrations of G+ and G− bacteria, arbuscular mycorrhizal fungi (except for at 0 DAT), general fungi, and total microbial biomass were higher at 0–15 cm compared with 15–30 cm soil depth under ASD treatments, whereas no differences were observed between 0–15 and 15–30 cm soil depths under CSF treatment. Overall, the soil microbial profile was well-differentiated between the two soil depths under ASD treatments but there is a lack of soil depth differences in CSF. These findings suggest that ASD soil treatments as an alternative to CSF may also exhibit potential for promoting soil health over the long-run in vegetable production systems, particularly within the area of the crop rhizosphere. In order to pinpoint specific players in different soil microbial functional groups, future research may use high-throughput DNA sequencing or other advanced approaches to elucidate soil microbial community composition in response to ASD application.
The original contributions presented in the study are included in the article, further inquiries can be directed to the corresponding author/s.
This present field study was conducted by HG, XZ, FD, JH, and ER. Statistical data analyses were performed by IV, NX, BP, JC, and XZ. Lab analyses of PLFAs and FAMEs were conducted by DM. Manuscript drafting and compilation were completed by IV. NX, LA, XZ, JH, FD, and ER contributed to editing and finalizing the manuscript. All authors contributed to the article and approved the submitted version.
The research presented here was funded in part by the USDA, ARS, Areawide Project on Anaerobic Soil Disinfestation.
The authors declare that the research was conducted in the absence of any commercial or financial relationships that could be construed as a potential conflict of interest.
All claims expressed in this article are solely those of the authors and do not necessarily represent those of their affiliated organizations, or those of the publisher, the editors and the reviewers. Any product that may be evaluated in this article, or claim that may be made by its manufacturer, is not guaranteed or endorsed by the publisher.
We gratefully acknowledge the helpful comments by the two reviewers. The authors wish to acknowledge Joseph V. Kupper for fatty acid methyl esters extraction and the substantial contributions of Zachary Black, Buck Nelson, Wesley Schonborn, Michael Hensley, Peter D'Auito, and Na Ding.
Breulmann, M., Masyutenko, N. P., Kogut, B. M., Schroll, R., Dörfler, U., Buscot, F., et al. (2014). Short-term bioavailability of carbon in soil organic matter fractions of different particle sizes and densities in grassland ecosystems. Sci. Tot. Environ. 497, 29–37. doi: 10.1016/j.scitotenv.2014.07.080
Butler, D. M., Kokalis-Burelle, N., Albano, J. P., Mccollum, T. G., Muramoto, J., Shennan, C., et al. (2014). Anaerobic soil disinfestation (ASD) combined with soil solarization as a methyl bromide alternative: vegetable crop performance and soil nutrient dynamics. Plant Soil 378, 365–381. doi: 10.1007/s11104-014-2030-z
Butler, D. M., Kokalis-Burelle, N., Muramoto, J., Shennan, C., McCollum, T. G., and Rosskopf, E. N. (2012a). Impact of anaerobic soil disinfestation combined with soil solarization on plant- parasitic nematodes and introduced inoculum of soilborne plant pathogens in raised-bed vegetable production. Crop Protect. 39, 33–40. doi: 10.1016/j.cropro.2012.03.019
Butler, D. M., Rosskopf, E. N., Kokalis-Burelle, N., Albano, J. P., Muramoto, J., and Shennan, C. (2012b). Exploring warm-season cover crops as carbon sources for anaerobic soil disinfestation (ASD). Plant Soil 355, 149–165. doi: 10.1007/s11104-011-1088-0
Buyer, J. S., and Sasser, M. (2012). High throughput phospholipid fatty acid analysis of soils. Appl. Soil Ecol. 61, 127–130. doi: 10.1016/j.apsoil.2012.06.005
Dangi, S. R., Gerik, J. S., Tirado-Corbal,á, R., and Ajwa, H. (2015). Soil microbial community structure and target organisms under different fumigation treatments. Appl. Environ. Soil Sci. 15, 1–8. doi: 10.1155/2015/673264
Demoling, F., Nilsson, L. O., and Bååth, E. (2008). Bacterial and fungal response to nitrogen fertilization in three coniferous forest soils. Soil Biol. Biochem. 40, 370–379. doi: 10.1016/j.soilbio.2007.08.019
Di Gioia, F., Hong, J. C., Ozores-Hampton, M., Zhao, X., Wilson, C., Thomas, J., et al. (2020). Anaerobic soil disinfestation: nutrient cycling and potential environmental impact. Acta Hortic. 1270, 51–62. doi: 10.17660/ActaHortic.2020.1270.6
Di Gioia, F., Ozores-Hampton, M., Hong, J., Kokalis-Burelle, N., Albano, J., Zhao, X., et al. (2016). The effects of anaerobic soil disinfestation on weed and nematode control, fruit yield, and quality of Florida fresh-market tomato. HortScience 51, 703–711. doi: 10.21273/HORTSCI.51.6.703
Di Gioia, F., Ozores-Hampton, M., Zhao, X., Thomas, J., Wilson, P., Li, Z., et al. (2017). Anaerobic soil disinfestation impact on soil nutrients dynamics and nitrous oxide emissions in fresh-market tomato. Agric. Ecosyst. Environ. 240, 194–205. doi: 10.1016/j.agee.2017.02.025
Fanin, N., Kardol, P., Farrell, M., Nilsson, M. C., Gundale, M. J., and Wardle, D. A. (2019). The ratio of Gram-positive to Gram-negative bacterial PLFA markers as an indicator of carbon availability in organic soils. Soil Biol. Biochem. 128, 111–114. doi: 10.1016/j.soilbio.2018.10.010
Ge, A. H., Liang, Z. H., Xiao, J. L., Zhang, Y., Zeng, Q., Xiong, C., et al. (2021). Microbial assembly and association network in watermelon rhizosphere after soil fumigation for Fusarium wilt control. Agric. Ecosyst. Environ. 312:107336. doi: 10.1016/j.agee.2021.107336
Guo, H., Di Gioia, F., Zhao, X., Ozores-Hampton, M., Swisher, M. E., Hong, J., et al. (2017). Optimizing anaerobic soil disinfestation for fresh market tomato production: nematode and weed control, yield, and fruit quality. Sci. Hortic. 218, 105–116. doi: 10.1016/j.scienta.2017.01.054
Guo, H., Zhao, X., Rosskopf, E. N., Di Gioia, F., Hong, J. C., and McNear, D. H. Jr. (2018). Impacts of anaerobic soil disinfestation and chemical fumigation on soil microbial communities in field tomato production system. Appl. Soil Ecol. 126, 165–173. doi: 10.1016/j.apsoil.2017.12.018
Hewavitharana, S. S., Klarer, E., Reed, A. J., Leisso, R., Poirier, B., Honaas, L., et al. (2019). Temporal dynamics of the soil metabolome and microbiome during simulated anaerobic soil disinfestation. Front. Microbiol. 10:2365. doi: 10.3389/fmicb.2019.02365
Ibekwe, A. M., Papiernik, S. K., Gan, J., Yates, S. R., Yang, C. H., and Crowley, D. E. (2001). Impact of fumigants on soil microbial communities. Appl. Environ. Microbiol. 67, 3245–3257. doi: 10.1128/AEM.67.7.3245-3257.2001
Jackson, L. E., Brussaard, L., de Ruiter, P. C., Pascual, U., Perrings, C., and Bawa, K. (2013). “Agrobiodiversity,” in Encyclopedia of Biodiversity, 2nd Edn, ed S. A. Levin (Waltham, MA: Academic Press), 126–135.
Klose, S., Acosta-Martínez, V., and Ajwa, H. A. (2006). Microbial community composition and enzyme activities in a sandy loam soil after fumigation with methyl bromide or alternative biocides. Soil Biol. Biochem. 38, 1243–1254. doi: 10.1016/j.soilbio.2005.09.025
LeBaron, H. M., and Hill, E. R. (2008). “Weeds resistant to nontriazine classes of herbicides,” in The Triazine Herbicides, eds H. M. Lebaron, J. E. McFarland, and O. C. Burnside (San Diego, CA: Elsevier), 133–151. doi: 10.1016/B978-044451167-6.50014-3
Liu, X., Cheng, X., Wang, H., Wang, K., and Qiao, K. (2015). Effect of fumigation with 1,3-dichloropropene on soil bacterial communities. Chemosphere 139, 379–385. doi: 10.1016/j.chemosphere.2015.07.034
Mazzola, M., Muramoto, J., and Shennan, C. (2018). Anaerobic disinfestation induced changes to the soil microbiome, disease incidence and strawberry fruit yields in California field trials. Appl. Soil Ecol. 127, 74–86. doi: 10.1016/j.apsoil.2018.03.009
Momma, N. (2008). Biological soil disinfestation (BSD) of soilborne pathogens and its possible mechanisms. Jpn. Agric. Res. Quart. 42, 7–12. doi: 10.6090/jarq.42.7
Momma, N., Kobara, Y., Uematsu, S., Kita, N., and Shinmura, A. (2013). Development of biological soil disinfestations in Japan. Appl. Microbiol. Biotechnol. 97, 3801–3809. doi: 10.1007/s00253-013-4826-9
Momma, N., Momma, M., and Kobara, Y. (2010). Biological soil disinfestation using ethanol: effect on Fusarium oxysporum f. sp. lycopersici and soil microorganisms. J. Gen. Plant Pathol. 76, 336–344. doi: 10.1007/s10327-010-0252-3
Paudel, B. R., Di Gioia, F., Zhao, X., Ozores-Hampton, M., Hong, J. C., Kokalis-Burelle, N., et al. (2020). Evaluating anaerobic soil disinfestation and other biological soil management strategies for open-field tomato production in Florida. Renew. Agric. Food Syst. 35, 274–285. doi: 10.1017/S1742170518000571
Poret-Peterson, A. T., Albu, S., McClean, A. E., and Kluepfel, D. A. (2019). Shifts in soil bacterial communities as a function of carbon source used during anaerobic soil disinfestation. Front. Environ. Sci. 6:160. doi: 10.3389/fenvs.2018.00160
Rosskopf, E., Di Gioia, F., Hong, J. C., Pisani, C., and Kokalis-Burelle, N. (2020). Organic amendments for pathogen and nematode control. Annu. Rev. Phytopathol. 58, 277–311. doi: 10.1146/annurev-phyto-080516-035608
Rosskopf, E. N., Chellemi, D. O., Kokalis-Burelle, N., and Church, G. T. (2005). Alternatives to methyl bromide: a Florida perspective. Plant Health Prog. 6:19. doi: 10.1094/PHP-2005-1027-01-RV
Shi, L., Wang, J., Gao, Z., Zhao, X., Di Gioia, F., Guo, H., et al. (2019). Economic analysis of anaerobic soil disinfestation for open-field fresh-market tomato production in Southwest and North Florida. HortTechnology 29, 777–787. doi: 10.21273/HORTTECH04332-19
Shrestha, U., Dee, M. E., Ownley, B. H., and Butler, D. M. (2018). Anaerobic soil disinfestation reduces germination and affects colonization of Sclerotium rolfsii sclerotia. Phytopathology 108, 342–351. doi: 10.1094/PHYTO-04-17-0152-R
Strauss, S. L., Greenhut, R. F., McClean, A. E., and Kluepfel, D. A. (2017). Effect of anaerobic soil disinfestation on the bacterial community and key soilborne phytopathogenic agents under walnut tree-crop nursery conditions. Plant Soil 415, 493–506. doi: 10.1007/s11104-016-3126-4
Strauss, S. L., and Kluepfel, D. A. (2015). Anaerobic soil disinfestation: a chemical-independent approach to pre-plant control of plant pathogens. J. Integr. Agric. 14, 2309–2318. doi: 10.1016/S2095-3119(15)61118-2
United States Department of Agriculture, National Agricultural Statistics Service (USDA-NASS) (2022). Data and Statistics. Washington, DC: USDA-NASS. Available online at: https://www.nass.usda.gov/Quick_Stats/Ag_Overview/stateOverview.php?state=FLORIDA
van Agtmaal, M., van Os, G. J., Hol, W. H., Hundscheid, M. P., Runia, W. T., Hordijk, C. A., et al. (2015). Legacy effects of anaerobic soil disinfestation on soil bacterial community composition and production of pathogen-suppressing volatiles. Front. Microbiol. 6:701. doi: 10.3389/fmicb.2015.00701
Keywords: ASD, chemical soil fumigation, discriminant analysis, FAME, microbial biomarker, PLFA, soil depth
Citation: Vincent IR, Paudel BR, Guo H, Rosskopf EN, Di Gioia F, Hong JC, McNear DH Jr, Xu N, Anrecio L, Colee J and Zhao X (2022) Spatial and Temporal Changes of Soil Microbial Communities in Field Tomato Production as Affected by Anaerobic Soil Disinfestation. Front. Sustain. Food Syst. 6:838635. doi: 10.3389/fsufs.2022.838635
Received: 18 December 2021; Accepted: 17 May 2022;
Published: 13 July 2022.
Edited by:
Engracia Madejon, Institute of Natural Resources and Agrobiology of Seville (CSIC), SpainReviewed by:
Juan Fernando Hirzel, Instituto de Investigaciones Agropecuarias, ChileCopyright © 2022 Vincent, Paudel, Guo, Rosskopf, Di Gioia, Hong, McNear, Xu, Anrecio, Colee and Zhao. This is an open-access article distributed under the terms of the Creative Commons Attribution License (CC BY). The use, distribution or reproduction in other forums is permitted, provided the original author(s) and the copyright owner(s) are credited and that the original publication in this journal is cited, in accordance with accepted academic practice. No use, distribution or reproduction is permitted which does not comply with these terms.
*Correspondence: Xin Zhao, enhpbkB1ZmwuZWR1
Disclaimer: All claims expressed in this article are solely those of the authors and do not necessarily represent those of their affiliated organizations, or those of the publisher, the editors and the reviewers. Any product that may be evaluated in this article or claim that may be made by its manufacturer is not guaranteed or endorsed by the publisher.
Research integrity at Frontiers
Learn more about the work of our research integrity team to safeguard the quality of each article we publish.