- 1Institute of Plant Breeding and Genetic Resources, Hellenic Agricultural Organization DEMETER, Thermi, Greece
- 2School of Agriculture, Aristotle University of Thessaloniki, Thessaloniki, Greece
In the forthcoming era of climate change and ecosystem degradation, fostering the use of beneficial microbiota in agroecosystems represents a major challenge toward sustainability. Some plant-associated bacteria, called Plant Growth Promoting Rhizobacteria (PGPR), may confer growth-promoting advantages to the plant host, through enhancing nutrient uptake, altering hormone homeostasis, and/or improving tolerance to abiotic stress factors and phytopathogens. In this regard, exploring the key ecological and evolutionary interactions between plants and their microbiomes is perquisite to develop innovative approaches and novel natural products that will complement conventional farming techniques. Recently, details of the molecular aspects of PGPR-mediated tolerance to various stress factors have come to light. At the same time the integration of the recent advances in the field of plant-microbiome crosstalk with novel -omic approaches will soon allow us to develop a holistic approach to “prime” plants against unfavorable environments. This mini review highlights the current state of the art on seed biopriming, focusing on the identification and application of novel PGPR in cultivated plant species under conditions where crop productivity is limited. The potential challenges of commercializing these PGPR as biostimulants to improve crop production under multiple environmental constraints of plant growth, as well as concerns about PGPR application and their impact on ecosystems, are also discussed.
Introduction
As climate change is rearing up, the significant rise in global temperature has caused detrimental effects not only on crop production, but also on cultivable area worldwide (Mohanty et al., 2021; Shah et al., 2021). During the decades of industrial revolution, humankind has continuously unbalanced its environment, with the massive use of chemical inputs, in order to stimulate crop production (Delitte et al., 2021). The intensive anthropogenic activities in combination with climate change has resulted to the systematic reduction in soil fertility and plant biomass production (Oleńska et al., 2020). In this regard, plants growing under field conditions meet a plethora of environmental challenges, among others drought, salinity, high temperature, and nutrient deficiency, that usually appear jointly to augment stress severity (Srivastava et al., 2021). Apart from these stresses, the over-accumulation of toxic metals such as Mn, Pb, Cd, and Cr in the soil environment due to rapid industrialization and population expansion represents another important threat limiting crop productivity (Singh et al., 2018). The majority of cultivated plants are not able to grow adequately under harsh environmental conditions, as they have not evolved the plasticity to cope with extreme environmental pressures (Shah et al., 2021). In fact, environmental stresses account for the highest losses in crop yield (~50%), according to the National Climate Assessment (NCA)-USDA. Based on the current climate change scenaria, the application of environmentally friendly strategies toward improving resource use efficiency while reducing non-sustainable agrochemical inputs, represents a major challenge for global food production and security. Since the first appearance of Plant Growth Promoting Rhizobacteria (PGPR) at early 80s (Kloepper et al., 1980), a broad number of beneficial microbes have been isolated and characterized (Leontidou et al., 2020; Mohanty et al., 2021). The increased demand for alternatives to synthetic chemicals has provided prominent opportunities for the extended use of PGPR formulations (Jiao et al., 2021), representing a significant scientific breakthrough toward securing future food production in a sustainable manner.
Plant rhizosphere represents one of the most delicate and dynamic ecosystems, hosting numerous microorganisms with multifaceted function in plant growth and survival (Pérez-Jaramillo et al., 2018; Genitsaris et al., 2020). A large number of diverse and emblematic PGPR have been isolated and characterized, including the taxa Pseudomonas, Bacillus, Enterobacter, Klebsiella, Agrobacterium, Erwinia, Azotobacter, Burkholderia, Bradyrhizobium, Rhizobium, and Serratia (Grover et al., 2021; Ullah et al., 2021). However, the efficiency of PGPR application on plant growth enhancement may depend on various factors, such as plant growth stage, plant species, cultivation method, soil ecology, as well as experimentation conditions. In the light of recent advances in the field of plant-microbe interactions, this mini review focuses on the mechanisms underlying PGPR-mediated abiotic stress tolerance and discusses the challenges of PGPR application as biopriming agents to improve crop production under changing climate conditions.
Prominent Mechanisms of PGPR-Mediated Alleviation of Abiotic Stress
Rhizosphere represents the zone for intense interactions between plant roots and soil microbial communities, that include bacteria, archaea, fungi, and viruses (Paez-Espino et al., 2016). Among them, bacteria are considered as the most abundant taxonomic group (Mohanty et al., 2021). The concept of soil metagenome to interpret the dynamics of gene microbes of the resulting soil metaphenome remains challenging as the nature of plant-microbe interplay is highly dynamic and very complex (Jansson and Hofmockel, 2018). In this regard, the delicate network of interkingdom signaling between microbes and their plants symbionts is regulated by a broad number of secondary metabolites and root exudates, rich in sugars, amino acids, organic acids and flavonoids, acting as rhizospheric chemo-attractants either to attract other beneficial soil microbes, or to repulse microbial phytopathogens (Ullah et al., 2021). An overview of different PGP traits affecting plant growth and stress tolerance, as well as their underlying mechanisms against drought and salt stress, is illustrated in Figure 1.
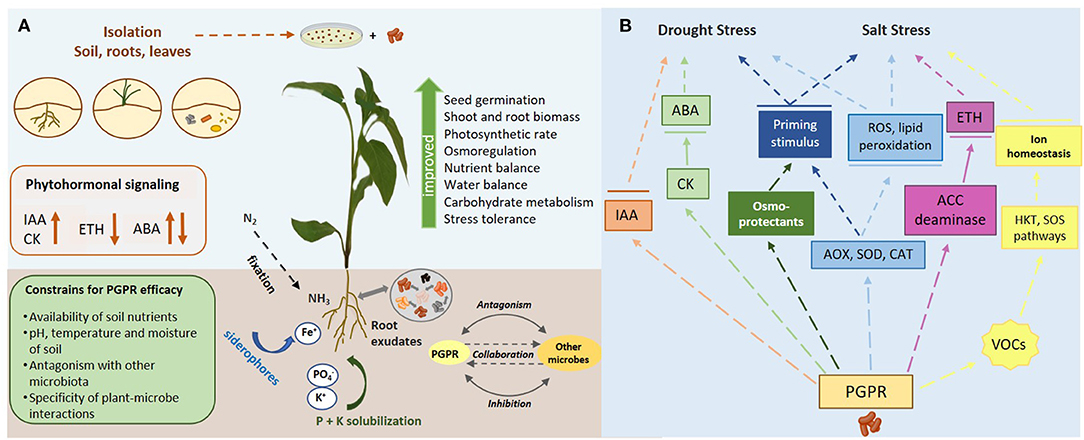
Figure 1. (A) An overview of diverse PGP traits affecting plant growth and stress tolerance. (B) A diagrammatic representation of the mechanisms underlying PGPR-mediated stress tolerance against drought and salt stress.
Several PGPR have been found to be able to decrease the need for nitrogen supplementation required for plant growth, either directly through nitrogen (N) fixation and mobilization, or indirectly by helping the N-fixers through their secretions and by root anatomic alterations (Vacheron et al., 2013; Jiao et al., 2021; Shah et al., 2021). Previously, it has been demonstrated that PGPR of different Bacillus sp., which are considered as free-living N-fixers (dos Santos et al., 2020), can trigger the expression levels of the Arabidopsis nitrate and ammonium transporters, leading to higher nutrient uptake (Calvo et al., 2019), an attribute that could be vital under nutrient deficiency stress. Additionally, some PGPR can improve nitrogen bioavailability indirectly by increasing root surface area and root morphology (Oleńska et al., 2020).
On the other hand, inorganic P solubilizing PGPR, belonging to the genera of Azotobacter, Bacillus, Burkholderia, Erwinia, Pseudomonas, Serratia, and Rhizobium, serve as another target for biofertilizer development, since over 99% of naturally available P exists in inorganic form (Oleńska et al., 2020). Bacteria-mediated solubilization of P is predominantly achieved by soil acidification from organic acid released by bacteria. These rhizobacteria are able to produce organic acids like gluconic acid and 2-ketogluconic acid, which can chelate the cations bound to phosphate, thus making it accessible for plant roots (Riaz et al., 2021).
PGPR can also contribute in iron acquisition by producing organic compounds of lower molecular weight compounds under iron-deficient conditions, called siderophores (Mohanty et al., 2021). Siderophores also can help plants inhibit disease development by depriving iron supply to pathogens (Backer et al., 2018). Several bacterial species are capable of producing siderophores, including, Azospirillum, Klebsiella, Pantoea, Pseudomonas, Azotobacter, Bacillus, and Serratia (dos Santos et al., 2020; Leontidou et al., 2020). Under metal stress, PGPR producing siderophores are valuable for plant survival, by reducing metal ion availability, thus preventing from the toxic accumulation of metals in the soil (Dimkpa et al., 2009) (Table 1). Further, uptake of other metals such as Zn is enhanced in the presence of PGP strains.
The majority of PGPR can also produce phytohormones, as well as associated metabolites and signaling molecules, resembling plant-synthesized hormones (Jiao et al., 2021; Shah et al., 2021). These included the hormones of idole acetic acid (IAA), gibberellic acid, cytokinins, and abscisic acid (ABA) (Ullah et al., 2021). Among them, IAA is the most popular hormone produced by nearly 80% of soil microbes, including the majority of Pseudomonas sp., Bacillus sp., Bulkoderia sp., and Rhizobium sp. (dos Santos et al., 2020; Khan et al., 2020). There are plenty of reports demonstrating the significance of certain IAA-producing PGPR in stimulating plant growth and crop yield under saline conditions, generated due to the extensive utilization of agrochemicals (Singh and Jha, 2017; Sarkar et al., 2018; Mellidou et al., 2021), or under water-deficit conditions (Jochum et al., 2019; Kumar et al., 2019) (Table 1). Several IAA-producing PGPR have been also reported to promote root length, root surface area, and root biomass, indirectly enhancing nutrient uptake and plant growth under nutrient-deficient conditions (Oleńska et al., 2020). In fact, at high amounts, IAA promote the formation of lateral roots, enhancing mineral absorption, and further fuel the production of root exudates improving bacterial colonization.
Cytokinins regulating a wide range of functions in plant growth and development, playa crucial role in root elongation, root meristem activity, lateral root and nodule formation. A wide range of bacteria genera such as Arthrobacter, Azotobacter, Bacillus, Pseudomonas, and Pantoea, are to synthesize and release cytokinins (Shah et al., 2021), thus improving nutrient supply, and also regulating host defense responses against pathogens (Akhtar et al., 2020). On the other hand, the gibberellin-releasing PGPR can also stimulate plant growth and yield, and include the species of Azospirillum, Shingomonas, Bacillus amyloliquafaciens, and Bacillus pumilus (dos Santos et al., 2020). Although not yet elucidated, beneficial effects resulted from PGPR producing phytohormones are exerted in different plant species, such as maize (Lucas et al., 2018), rice (Lee et al., 2015), or soybean (Kang et al., 2019).
Additionally, some PGPR possess the enzyme of 1-aminocyclopropane-1-carboxylate (ACC) deaminase that is able to scavenge plant ACC (Orozco-Mosqueda et al., 2020). These bacteria can hydrolyze ACC to ammonia and α-ketobutyrate, thereby limiting substrate availability for ethylene production and monitoring excess ethylene levels. PGPR that possess the enzyme, i.e., some strains of Pseudomonas oryzihabitans, Bacillus amyloliquefaciens and Burkholderia phytofirmans, are capable of promoting root elongation of their host plants (Oleńska et al., 2020). Inoculation with ACC-deaminase possessing strains has been reported to enhance plant growth under salt stress in rice (Nautiyal et al., 2013; Sarkar et al., 2018), rapeseed (Li et al., 2017), wheat (Singh and Jha, 2017), bean (Gupta and Pandey, 2019), and tomato (Mellidou et al., 2021), or under drought stress in artichoke (Namwongsa et al., 2019), maize (Danish et al., 2020), and millet (Chandra et al., 2020), or even under flooding such as in Rumex palustris (Ravanbakhsh et al., 2017), and rice (Etesami and Alikhani, 2016) (Table 1). Notably, a mutation in ACC deaminase gene has been correlated with low nitrogenase activity and inability to enhance plant growth of rice upon salt stress (Han et al., 2015).
Some PGPR may also secrete ABA, that acts antagonistically to ethylene in the control of plant development, as well as under abiotic stress, presumably through controlling stomatal conductance (Müller, 2021). Studies so far generated contrasting results on the mechanisms of ABA-mediated stress tolerance that seem to be dependent on both plant- and bacteria-species. In particular, some PGPR have been reported to act as ABA-producers, including strains of Azospirillium brasiliense, Achromobacter xylosoxidans, B. lycheniformis, B. pumilus, and Rhizobium spp. (Egamberdieva et al., 2017), or ABA stimulators (Salomon et al., 2014) under water-deficit stress (Cohen et al., 2015) (Table 1). Similarly, under excess salt, the ABA-induced ROS accumulation mediated by PGPR can activate Ca+2 channels inducing stomatal closure, thus help plants reducing water loss(Goswami and Deka, 2020; Grover et al., 2021).
By contrast, it has been also suggested that some PGPR can use ABA as carbon and energy source, thus reducing ABA accumulation within plant tissues (Oleńska et al., 2020). In accordance to this theory, inoculation of wheat with psychrophilic Bacillus spp. strains, improved cold stress tolerance, by modulating, among others, the expression levels of ABA-related genes (Zubair et al., 2019). Similarly, suppression of ABA signaling in tomato plants inoculated with a P. oryzihabitans strain has been demonstrated after exposure to salt stress (Mellidou et al., 2021). Conceivably, these results indicated alterations in ABA-mediated signaling pathways can orchestrate the tolerance mechanisms of host plants to various abiotic stress factors, triggering diverse signaling genes. A fewer PGPR can also produce polyamines, and in this regard, they can enhance root architecture, as well as stimulate stomatal conductance and photosynthesis, as recorded in Arabidopsis plants inoculated with the spermidine-producing B. megaterium strain in which ROS production was reduced, and ABA biosynthesis were enhanced (Zhou et al., 2016) (Table 1).
There are thousands of non-hormonal signal compounds mediating a complex network of plant-to- microbe communication and vice versa, governing diverse aspects of growth and metabolism of both holobiont partners (plant and its related microbiome). Among the secondary metabolites and root exudates that act as rhizospheric chemo-attractants, volatile compounds (VOCs) represent a class of signaling molecules, secreted by microbes such as terpenes, N- and sulfur-compounds, and pyrazines, that can regulate plant growth and modulate plant and soil health (Mohanty et al., 2021; Ullah et al., 2021). Furthermore, PGPR releasing extracellular polymeric substances (EPS) can help plants recuperate from heavy metal stress by limiting their availability in the soil (Mishra et al., 2017).
Apart from their well-characterized PGP properties, some PGPR strains, including the species of Pseudomonas, Enterobacter, Bacillus, Azotobacter, and Burkholderia, have been found to be able to re-program the redox state of plants, training them to withstand pathogen attacks and adverse environmental stimuli (Mellidou et al., 2021; Mitra et al., 2021). Inoculations with such strains, can impose plants to a primed state, at which they are able to activate more robustly their defense responses against abiotic stimuli. In support to this theory, it has been demonstrated that tomato seedlings, inoculated with the ACC deaminase possessing strain P. oryzihabitans AXSa06, undergo a mild oxidative stress, as indicated by increased lipid peroxidation, just enough to augment their alertness for efficient activation of antioxidant machinery prior to salt stress application (Mellidou et al., 2021). Similarly, several ACC-deaminase-possessing strains have been found to efficiently enhance stress tolerance of tomato and pepper (Mayak et al., 2004), or wheat (Chandra et al., 2020), under water-deficient conditions (Table 1). The putative priming effect of such strains may be related to enhanced catalase, ascorbate peroxidase or glutathione peroxidase activity in inoculated plants (Mellidou et al., 2021; Swain et al., 2021). By contrast, in some other studies, PGPR inoculation resulted to suppressed expression of stress-responsive genes or genes encoding ROS-scavenging enzymes involved in the protection of plant upon stress application (Goswami and Deka, 2020). In tomato, decreased lipid peroxidation, accompanied by high glutathione levels and low antioxidant enzyme activities have been reported in plants inoculated with the strain Sphingomonas sp. (LK11) upon salt stress (Halo et al., 2015). It seems that different “contradictory” alterations in plant functions can be undertaken from inoculated plants under stress, and this probably is depended on the PGPR modes of action, and reflecting the multifactorial processes simultaneously regulated under stress conditions. Further experimentation to unravel the crosstalk between PGPR-mediated signal and metabolic/molecular re-programming responsible for the enhancement of plant tolerance under diverse environmental cues needs to be undertaken.
Challenges and Ecological Concerns of PGPR Application From Lab to the Field
While the technology of PGPR inoculation is centuries old, there are several bottlenecks that need to be overcome prior to their application to the field (Sessitsch et al., 2019). This is mainly because the development of PGPR inocula is based on in vitro assays of their biochemical properties and functions. Conceptually, there is need to develop PGPR formulations appropriate for field conditions, as it is difficult to predict their in vitro responses (Backer et al., 2018). Another consideration that should not be overlooked is that PGPR may exert their plant-growth effect at different timepoints after application. In this context, the effect of acute (applied once) vs. chronic (applied several times) application of PGPR, as well as the target of application, must be considered. In fact, not all bacterial species can survive for long in the soil, thus they must be artificially mass propagated with regard to their biological activity under field conditions (Mohanty et al., 2021).
Multiple factors, such as soil properties, plant species, and agricultural practices, that determine the predominant microbial taxa which can survive and evolve (dos Santos et al., 2020). For example, soil temperature is a predominant factor limiting microbe performance. In this regard, some microbes can form biofilms consisted of polysaccharides, that facilitate their plant symbionts to isolate stimuli from changes in their environment, such as soil temperature or pH variations (Shah et al., 2021). On the other hand, rich soils can also shape microbiome communities and affect PGPR efficacy. In particular, at the excess of nutrient availability, there is lower antagonism for nutrients from other microbes probably resulting to higher diversity and more complex interactions that suppress antagonist microbes, but at the same time it can eliminates the mutualistic benefits of the symbiosis between PGPR and plants.
The stability of PGPR can be also affected by their production (culture media and growth conditions), formulation (in liquid form, or as seed coating and pellets), and transportation conditions (Sessitsch et al., 2019; Jiao et al., 2021). In this regard, the development of efficient microbial inocula, single or as consortia, with high colonization ability in-planta and long shelf-life in soil is challenging. The ideal PGPR for commercialization should possess as many beneficial traits as possible, propagating rapidly in vitro for massive production, having high capacity for rhizosphere colonization, being environmentally friendly, compatible with other soil microbiota and tolerant to environmental stresses (Jiao et al., 2021).
Another aspect that should not be underestimated in the efficacy of PGPR is plant host specificity, as root exudates synthesized and released by roots can attract symbiotic partners due to the specificity of interactions (Keet et al., 2017). At the same time, PGPR may face antagonism from the indigenous population of soil microbes prior to their inoculation, especially under low nutrient and energy resources, thus limiting their root colonization efficacy (Shah et al., 2021). In this perspective, the use of well-formulated microbial consortia may be a better strategy than the use of single strains, as the compatible partners of a consortium can interact synergistically with each other by facilitating colonization, and further displaying diverse resistance to environmental stimuli, thereby having a better probability to adapt and survive.
Future studies should also consider the ecological concerns of the large-scale release of PGPR in the field, as well as the effect that they may have on perturbing natural microbial diversity (Mohanty et al., 2021). In this frame, plant species usually cultivated under controlled greenhouse condition, such as vegetables, could serve as an excellent alternative to investigate the potential of successful implementation of PGPR using limited resources.
Until recently, the regulatory framework for registering and commercializing PGPR as biostimulants is tremendously complicated, requiring numerous safety documentation (Backer et al., 2018). This is presumably because each country has its own regulatory procedures and norms for biobased products, usually accompanied by high fees for registrations and long-lasting on-farm evaluation protocols (Shah et al., 2021). From ecological perspective, the development of commercial PGPR products, naturally existing in ecosystems, rises issues of intellectual property, as only formulations and uses can be patented, and not the actual life forms (Matthews and Cuchiara, 2014). Nonetheless, as climate change is a global issue, collaboration between academic, industrial and governmental partners is obligatory for a successful product development from the isolation of microbes with PGP traits to their licensing as biostimulants, and also for the implementation of simpler registration policies. For these reasons, EU constitutes a world leader in the recognition of these products as a separate category, and efforts have been made to harmonize rules concerning the placing of these products on the market of the individual Member States. Regulation 2019/1009 of the European Council, which lays down rules on the disposal of fertilizer products, introduces for the first time a distinct product category of plant biostimulants, divided into two subcategories (i) biostimulants with microorganisms and (ii) biostimulants without microorganisms. The regulation will enter into force on 16 July 2022, although compliance with harmonized rules would therefore remain optional, because of the local nature of certain product markets.
Another obstacle in the wide utilization of microbe-based techniques in the field is farmer's mindset (Shah et al., 2021). For farmers, it is difficult to quit utilization of common agrochemical that have served as an efficient strategy for over 50 years, even in small doses. But more important is the disadvantage of high costs of choosing a PGPR-based strategy over the common chemicals. Keeping this in mind, it is very challenging to properly educate producers regarding the benefits of bioproducts in the upcoming limiting resources era due to climate change.
During their long co-evolution with plants, phytomicrobes can affect plant phenotypic plasticity through modifying plant growth and responses to environmental stimuli (Jiao et al., 2021). According to the emerging holobiont concept, most organisms exist as “superorganisms”, an entity consisting of the host organism and all its related microbes (Nogales et al., 2016). In this frame, the symbiotic microbial community that has the ability to adapt faster than the host plants, would facilitate the holobiont partners to acclimate and survive under rapidly changing environmental conditions, serving as a rich source of stress-mitigating bacteria (Backer et al., 2018). Conceivably, overlooking the contribution of rhizospheric communities to the overall plant phenotype can decrease the efficiency of agricultural strategies toward sustainability. Despite the tremendous advances in plant genetic improvement, it is becoming evident that plant and its symbiotic microbiome should be treated as a heritable life-long partners (Ravanbakhsh et al., 2021). The integration of -omic approaches from both plants and microbes would enable the re-evaluation of the next generation plant breeding approaches, on the basis of genetic variation offered by plant microbiome, that can be also inherited from parent plant to seed and then to the seedlings (Gopal and Gupta, 2016).
Concluding Remarks
Scientific communities and commercial enterprises should take advantage of the growing public concern around the use of agrochemicals, thereby promoting the benefits of alternative technologies such as the utilization of phytomicrobiome in the form of “plant probiotics”. There are myriads of studies on important crop species unraveling beneficial microbes for plant growth, especially under harsh environments. Nonetheless, more systematic and large-scale studies are required to detect and characterize novel potential microbial biostimulants, and to elucidate their functions in host plants as consortia, to fill in important knowledge gaps before proceeding to their commercialization. Additionally, coupling innovative-omic approaches with computational biology will resolve technical challenges associated with the unpredicted plant–microbiome behavior under a continuously fluctuating environment, driving innovations in the microbiome application in the agriculture sector.
Author Contributions
IM conceived the original article idea, performed the main topic review, and wrote the original manuscript. KK contributed with literature review, manuscript writing, and feedback throughout the manuscript development. All authors contributed to the article and approved the submitted version.
Funding
This project was funded by the Hellenic Foundation for Research and Innovation (HFRI) and the General Secretariat for Research and Innovation (GSRI), under the Grant Agreement No. 705, awarded to IM.
Conflict of Interest
The authors declare that the research was conducted in the absence of any commercial or financial relationships that could be construed as a potential conflict of interest.
Publisher's Note
All claims expressed in this article are solely those of the authors and do not necessarily represent those of their affiliated organizations, or those of the publisher, the editors and the reviewers. Any product that may be evaluated in this article, or claim that may be made by its manufacturer, is not guaranteed or endorsed by the publisher.
References
Akhtar, S. S., Mekureyaw, M. F., Pandey, C., and Roitsch, T. (2020). Role of Cytokinins for Interactions of Plants with Microbial Pathogens and Pest Insects. Front. Plant Sci. 10, 1777. doi: 10.3389/fpls.2019.01777
Backer, R., Rokem, J. S., Ilangumaran, G., Lamont, J., Praslickova, D., Ricci, E., et al. (2018). Plant growth-promoting rhizobacteria: context, mechanisms of action, and roadmap to commercialization of biostimulants for sustainable agriculture. Front. Plant Sci. 871, 1–17. doi: 10.3389/fpls.2018.01473
Calvo, P., Zebelo, S., McNear, D., Kloepper, J., and Fadamiro, H. (2019). Plant growth-promoting rhizobacteria induce changes in Arabidopsis thaliana gene expression of nitrate and ammonium uptake genes. J. Plant Interact. 14, 224–231. doi: 10.1080/17429145.2019.1602887
Chandra, D., Srivastava, R., Glick, B. R., and Sharma, A. K. (2020). Rhizobacteria producing ACC deaminase mitigate water-stress response in finger millet (Eleusine coracana (L.) Gaertn.). 3 Biotech 10, 65. doi: 10.1007/s13205-019-2046-4
Cohen, A. C., Bottini, R., Pontin, M., Berli, F. J., Moreno, D., Boccanlandro, H., et al. (2015). Azospirillum brasilense ameliorates the response of Arabidopsis thaliana to drought mainly via enhancement of ABA levels. Physiol. Plant. 153, 79–90. doi: 10.1111/ppl.12221
Danish, S., Zafar-Ul-Hye, M., Mohsin, F., and Hussain, M. (2020). ACC-deaminase producing plant growth promoting rhizobacteria and biochar mitigate adverse effects of drought stress on maize growth. PLoS ONE 15, 1–14. doi: 10.1371/journal.pone.0230615
Delitte, M., Caulier, S., Bragard, C., and Desoignies, N. (2021). Plant microbiota beyond farming practices: a review. Front. Sustain. Food Syst. 5, 1–14. doi: 10.3389/fsufs.2021.624203
Dimkpa, C. O., Merten, D., Svatoš, A., Büchel, G., and Kothe, E. (2009). Metal-induced oxidative stress impacting plant growth in contaminated soil is alleviated by microbial siderophores. Soil Biol. Biochem. 41, 154–162. doi: 10.1016/j.soilbio.2008.10.010
dos Santos, R. M., Diaz, P. A. E., Lobo, L. L. B., and Rigobelo, E. C. (2020). Use of plant growth-promoting rhizobacteria in maize and sugarcane: characteristics and applications. Front. Sustain. Food Syst. 4, 1–15. doi: 10.3389/fsufs.2020.00136
Egamberdieva, D., Wirth, S. J., Alqarawi, A. A., Abd-Allah, E. F., and Hashem, A. (2017). Phytohormones and beneficial microbes: essential components for plants to balance stress and fitness. Front. Microbiol. 8, e02104. doi: 10.3389/fmicb.2017.02104
Etesami, H., and Alikhani, H. A. (2016). Co-inoculation with endophytic and rhizosphere bacteria allows reduced application rates of N-fertilizer for rice plant. Rhizosphere 2, 5–12. doi: 10.1016/j.rhisph.2016.09.003
Genitsaris, S., Stefanidou, N., Leontidou, K., Matsi, T., Karamanoli, K., and Mellidou, I. (2020). Bacterial communities in the rhizosphere and phyllosphere of halophytes and drought-tolerant plants in mediterranean ecosystems. Microorganisms 8,1708. doi: 10.3390/microorganisms8111708
Gopal, M., and Gupta, A. (2016). Microbiome selection could spur next-generation plant breeding strategies. Front. Microbiol. 7, 1971. doi: 10.3389/fmicb.2016.01971
Goswami, M., and Deka, S. (2020). Plant growth-promoting rhizobacteria—alleviators of abiotic stresses in soil: a review. Pedosphere 30, 40–61. doi: 10.1016/S1002-0160(19)60839-8
Grover, M., Bodhankar, S., Sharma, A., Sharma, P., Singh, J., and Nain, L. (2021). PGPR mediated alterations in root traits: way toward sustainable crop production. Front. Sustain. Food Syst. 4, 1–28. doi: 10.3389/fsufs.2020.618230
Gupta, S., and Pandey, S. (2019). ACC deaminase producing bacteria with multifarious plant growth promoting traits alleviates salinity stress in French bean (Phaseolus vulgaris) plants. Front. Microbiol. 10, 1–17. doi: 10.3389/fmicb.2019.01506
Halo, B. A., Khan, A. L., Waqas, M., Al-Harrasi, A., Hussain, J., Ali, L., et al. (2015). Endophytic bacteria (Sphingomonas sp. LK11) and gibberellin can improve Solanum lycopersicum growth and oxidative stress under salinity. J. Plant Interact. 10, 117–125. doi: 10.1080/17429145.2015.1033659
Han, Y., Wang, R., Yang, Z., Zhan, Y., Ma, Y., Ping, S., et al. (2015). 1-Aminocyclopropane-1-carboxylate deaminase from Pseudomonas stutzeri A1501 facilitates the growth of rice in the presence of salt or heavy metals. J. Microbiol. Biotechnol. 25, 1119–1128. doi: 10.4014/jmb.1412.12053
Jansson, J. K., and Hofmockel, K. S. (2018). The soil microbiome—from metagenomics to metaphenomics. Curr. Opin. Microbiol. 43, 162–168. doi: 10.1016/j.mib.2018.01.013
Jiao, X., Takishita, Y., Zhou, G., and Smith, D. L. (2021). Plant associated rhizobacteria for biocontrol and plant growth enhancement. Front. Plant Sci. 12, e634796. doi: 10.3389/fpls.2021.634796
Jochum, M. D., McWilliams, K. L., Borrego, E. J., Kolomiets, M. V., Niu, G., Pierson, E. A., et al. (2019). Bioprospecting plant growth-promoting rhizobacteria that mitigate drought stress in grasses. Front. Microbiol. 10, 1–9. doi: 10.3389/fmicb.2019.02106
Kang, S.-M., Khan, A. L., Waqas, M., Asaf, S., Lee, K.-E., Park, Y.-G., et al. (2019). Integrated phytohormone production by the plant growth-promoting rhizobacterium Bacillus tequilensis SSB07 induced thermotolerance in soybean. J. Plant Interact. 14, 416–423. doi: 10.1080/17429145.2019.1640294
Keet, J.-H., Ellis, A. G., Hui, C., and Le Roux, J. J. (2017). Legume–rhizobium symbiotic promiscuity and effectiveness do not affect plant invasiveness. Ann. Bot. 119, 1319–1331. doi: 10.1093/aob/mcx028
Khan, N., Bano, A., Ali, S., and Babar, M. A. (2020). Crosstalk amongst phytohormones from planta and PGPR under biotic and abiotic stresses. Plant Growth Regul. 90, 189–203. doi: 10.1007/s10725-020-00571-x
Kloepper, J. W., Leong, J., Teintze, M., and Schroth, M. N. (1980). Enhanced plant growth by siderophores produced by plant growth-promoting rhizobacteria. Nature 286, 885–886. doi: 10.1038/286885a0
Kumar, A., Patel, J. S., Meena, V. S., and Ramteke, P. W. (2019). Plant growth-promoting rhizobacteria: strategies to improve abiotic stresses under sustainable agriculture. J. Plant Nutr. 42, 1402–1415. doi: 10.1080/01904167.2019.1616757
Lee, K.-E., Radhakrishnan, R., Kang, S.-M., You, Y.-H., Joo, G.-J., Lee, I.-J., et al. (2015). Enterococcus faecium LKE12 cell-free extract accelerates host plant growth via gibberellin and indole-3-acetic acid secretion. J. Microbiol. Biotechnol. 25, 1467–1475. doi: 10.4014/jmb.1502.02011
Leontidou, K., Genitsaris, S., Papadopoulou, A., Kamou, N., Bosmali, I., Matsi, T., et al. (2020). Plant growth promoting rhizobacteria isolated from halophytes and drought-tolerant plants: genomic characterisation and exploration of phyto-beneficial traits. Sci. Rep. 10, 1–15. doi: 10.1038/s41598-020-71652-0
Li, H., Lei, P., Pang, X., Li, S., Xu, H., Xu, Z., and Feng, X. (2017). Enhanced tolerance to salt stress in canola (Brassica napus L.) seedlings inoculated with the halotolerant Enterobacter cloacae HSNJ4. Appl. Soil Ecol. 119, 26–34. doi: 10.1016/j.apsoil.2017.05.033
Lucas, T. M. R., Carlos, H. C., Fabio, L. C. M., and Gustavo, V. M. (2018). Azospirillum spp. potential for maize growth and yield. Afr. J. Biotechnol. 17, 574–585. doi: 10.5897/AJB2017.16333
Matthews, K. R., and Cuchiara, M. L. (2014). Gene patents, patenting life and the impact of court rulings on US stem cell patents and research. Regen. Med. 9, 191–200. doi: 10.2217/rme.13.93
Mayak, S., Tirosh, T., and Glick, B. R. (2004). Plant growth-promoting bacteria that confer resistance to water stress in tomatoes and peppers. Plant Sci. 166, 525–530. doi: 10.1016/j.plantsci.2003.10.025
Mellidou, I., Ainalidou, A., Papadopoulou, A., Leontidou, K., Genitsaris, S., Karagiannis, E., et al. (2021). Comparative transcriptomics and metabolomics reveal an intricate priming mechanism involved in PGPR-mediated salt tolerance in tomato. Front. Plant Sci. 12, 1–22. doi: 10.3389/fpls.2021.713984
Mishra, J., Singh, R., and Arora, N. K. (2017). Alleviation of heavy metal stress in plants and remediation of soil by rhizosphere microorganisms. Front. Microbiol. 8, 1706. doi: 10.3389/fmicb.2017.01706
Mitra, D., Mondal, R., Khoshru, B., Shadangi, S., Das Mohapatra, P. K., and Panneerselvam, P. (2021). Rhizobacteria mediated seed bio-priming triggers the resistance and plant growth for sustainable crop production. Curr. Res. Microb. Sci. 2, 100071. doi: 10.1016/j.crmicr.2021.100071
Mohanty, P., Singh, P. K., Chakraborty, D., Mishra, S., and Pattnaik, R. (2021). Insight into the role of PGPR in sustainable agriculture and environment. Front. Sustain. Food Syst. 5, 1–12. doi: 10.3389/fsufs.2021.667150
Müller, M.. (2021). Foes or friends: ABA and ethylene interaction under abiotic stress. Plants (Basel, Switzerland) 10, 448. doi: 10.3390/plants10030448
Namwongsa, J., Jogloy, S., Vorasoot, N., Boonlue, S., Riddech, N., and Mongkolthanaruk, W. (2019). Endophytic bacteria improve root traits, biomass and yield of Helianthus tuberosus L. Under normal and deficit water conditions. J. Microbiol. Biotechnol. 29, 1777–1789. doi: 10.4014/jmb.1903.03062
Nautiyal, C. S., Srivastava, S., Chauhan, P. S., Seem, K., Mishra, A., and Sopory, S. K. (2013). Plant growth-promoting bacteria Bacillus amyloliquefaciens NBRISN13 modulates gene expression profile of leaf and rhizosphere community in rice during salt stress. Plant Physiol. Biochem. 66, 1–9. doi: 10.1016/j.plaphy.2013.01.020
Nogales, A., Nobre, T., Valadas, V., Ragonezi, C., Döring, M., Polidoros, A., et al. (2016). Can functional hologenomics aid tackling current challenges in plant breeding? Brief. Funct. Genomics 15, 288–297. doi: 10.1093/bfgp/elv030
Oleńska, E., Małek, W., Wójcik, M., Swiecicka, I., Thijs, S., and Vangronsveld, J. (2020). Beneficial features of plant growth-promoting rhizobacteria for improving plant growth and health in challenging conditions: a methodical review. Sci. Total Environ. 743, e14068. doi: 10.1016/j.scitotenv.2020.140682
Orozco-Mosqueda, M., del, C., Glick, B. R., and Santoyo, G. (2020). ACC deaminase in plant growth-promoting bacteria (PGPB): An efficient mechanism to counter salt stress in crops. Microbiol. Res. 235, 126439. doi: 10.1016/j.micres.2020.126439
Paez-Espino, D., Eloe-Fadrosh, E. A., Pavlopoulos, G. A., Thomas, A. D., Huntemann, M., Mikhailova, N., et al. (2016). Uncovering earth's virome. Nature 536, 425–430. doi: 10.1038/nature19094
Pérez-Jaramillo, J. E., Carrión, V. J., de Hollander, M., and Raaijmakers, J. M. (2018). The wild side of plant microbiomes. Microbiome 6, 4–9. doi: 10.1186/s40168-018-0519-z
Ravanbakhsh, M., Kowalchuk, G. A., and Jousset, A. (2021). Targeted plant hologenome editing for plant trait enhancement. New Phytol. 229, 1067–1077. doi: 10.1111/nph.16867
Ravanbakhsh, M., Sasidharan, R., Voesenek, L. A. C. J., Kowalchuk, G. A., and Jousset, A. (2017). ACC deaminase-producing rhizosphere bacteria modulate plant responses to flooding. J. Ecol. 105, 979–986. doi: 10.1111/1365-2745.12721
Riaz, U., Murtaza, G., Anum, W., Samreen, T., Sarfraz, M., and Nazir, M. Z. (2021). “Plant Growth-Promoting Rhizobacteria (PGPR) as biofertilizers and biopesticides,” in Microbiota and Biofertilizers: A Sustainable Continuum for Plant and Soil Health, eds K. R. Hakeem, G. H. Dar, M. A. Mehmood, and R. A. Bhat (Cham: Springer International Publishing), 181–196.
Salomon, M. V., Bottini, R., de Souza Filho, G. A., Cohen, A. C., Moreno, D., Gil, M., et al. (2014). Bacteria isolated from roots and rhizosphere of Vitis vinifera retard water losses, induce abscisic acid accumulation and synthesis of defense-related terpenes in in vitro cultured grapevine. Physiol. Plant. 151, 359–374. doi: 10.1111/ppl.12117
Sarkar, A., Pramanik, K., Mitra, S., Soren, T., and Maiti, T. K. (2018). Enhancement of growth and salt tolerance of rice seedlings by ACC deaminase-producing Burkholderia sp. MTCC 12259. J. Plant Physiol. 231, 434–442. doi: 10.1016/j.jplph.2018.10.010
Sessitsch, A., Pfaffenbichler, N., and Mitter, B. (2019). Microbiome applications from lab to field: facing complexity. Trends Plant Sci. 24, 194–198. doi: 10.1016/j.tplants.2018.12.004
Shah, A., Nazari, M., Antar, M., Msimbira, L. A., Naamala, J., Lyu, D., et al. (2021). PGPR in agriculture: a sustainable approach to increasing climate change resilience. Front. Sustain. Food Syst. 5, 1–22. doi: 10.3389/fsufs.2021.667546
Singh, R. P., and Jha, P. N. (2017). The PGPR stenotrophomonas maltophilia SBP-9 augments resistance against biotic and abiotic stress in wheat plants. Front. Microbiol. 8, 1945. doi: 10.3389/fmicb.2017.01945
Singh, V. K., Singh, A. K., Singh, P. P., and Kumar, A. (2018). Interaction of plant growth promoting bacteria with tomato under abiotic stress: a review. Agricult. Ecosyst. Environ. 267, 129–140. doi: 10.1016/j.agee.2018.08.020
Srivastava, A. K., Suresh Kumar, J., and Suprasanna, P. (2021). Seed ‘primeomics': plants memorize their germination under stress. Biol. Rev. 96, 1723–1743. doi: 10.1111/brv.12722
Swain, H., Adak, T., Mukherjee, A. K., Sarangi, S., Samal, P., Khandual, A., et al. (2021). Seed biopriming with trichoderma strains isolated from tree bark improves plant growth, antioxidative defense system in rice and enhance straw degradation capacity. Front. Microbiol. 12, 633881. doi: 10.3389/fmicb.2021.633881
Ullah, A., Bano, A., and Khan, N. (2021). Climate change and salinity effects on crops and chemical communication between plants and plant growth-promoting microorganisms under stress. Front. Sustain. Food Syst. 5, 1–16. doi: 10.3389/fsufs.2021.618092
Vacheron, J., Desbrosses, G., Bouffaud, M. L., Touraine, B., Moënne-Loccoz, Y., Muller, D., et al. (2013). Plant growth-promoting rhizobacteria and root system functioning. Front. Plant Sci. 4, 1–19. doi: 10.3389/fpls.2013.00356
Zhou, C., Ma, Z., Zhu, L., Xiao, X., Xie, Y., Zhu, J., et al. (2016). Rhizobacterial strain Bacillus megaterium BOFC15 induces cellular polyamine changes that improve plant growth and drought resistance. Int. J. Mol. Sci. 17, 976. doi: 10.3390/ijms17060976
Zubair, M., Hanif, A., Farzand, A., Sheikh, T. M., Khan, A. R., Suleman, M., et al. (2019). Genetic screening and expression analysis of psychrophilic Bacillus spp. reveal their potential to alleviate cold stress and modulate phytohormones in wheat. Microorganisms. 7, 337. doi: 10.3390/microorganisms7090337
Keywords: beneficial microbes, biostimulants, holobiont, hormonal reprogramming, rhizobacteria, seed biopriming, sustainability
Citation: Mellidou I and Karamanoli K (2022) Unlocking PGPR-Mediated Abiotic Stress Tolerance: What Lies Beneath. Front. Sustain. Food Syst. 6:832896. doi: 10.3389/fsufs.2022.832896
Received: 10 December 2021; Accepted: 25 January 2022;
Published: 18 February 2022.
Edited by:
Autar Krishen Mattoo, Agricultural Research Service (USDA), United StatesReviewed by:
Senthilkumar Murugaiyan, Tamil Nadu Agricultural University, IndiaCopyright © 2022 Mellidou and Karamanoli. This is an open-access article distributed under the terms of the Creative Commons Attribution License (CC BY). The use, distribution or reproduction in other forums is permitted, provided the original author(s) and the copyright owner(s) are credited and that the original publication in this journal is cited, in accordance with accepted academic practice. No use, distribution or reproduction is permitted which does not comply with these terms.
*Correspondence: Katerina Karamanoli, a2F0a2FyQGFncm8uYXV0aC5ncg==