- 1Soil Research Centre, Department of Geography and Environmental Science, University of Reading, Reading, United Kingdom
- 2Department of Plant Biology and Soil Science, University of Vigo, Vigo, Spain
- 3Department of Horticulture, Kwame Nkrumah University of Science and Technology, Kumasi, Ghana
- 4Department of Horticulture, University for Development Studies, Tamale, Ghana
- 5Soil Research Centre, School of Agriculture, Policy, and Development, University of Reading, Reading, United Kingdom
Biochars have been proposed as a novel biotechnology to increase crop yields in acidic soils due to a liming effect. However, the application of biochar to soils with a neutral soil pH is less likely to improve yield. A rise in pH typically increases the availability of macronutrients (e.g., , ) but biochar is known to immobilize some elements due to a pH increase and adsorption on the biochar surface. Therefore, biochar application may reduce the uptake of important micronutrients (e.g., Cu, Fe, and Zn) into the edible portions of food crops. Before recommending indiscriminate biochar application to tropical soils, an understanding of the potentially negative impacts of biochar application to contrasting soil types should be fully appreciated to prevent unintended consequences. Our aim was to determine the impact of biochar amendment to an acidic soil and a neutral soil on micronutrient availability and uptake into leafy greens. We produced biochars from 3 different organic feedstock materials (corn cobs, rice husk and teak sawdust) and applied these in pot experiments to an acidic tropical soil (pH 4.5) and a neutral tropical soil (pH 6.9) collected from urban farms in Tamale and Kumasi, respectively, in Ghana. We grew leafy greens (Amaranthus, Corchorus, and Lettuce) and measured their growth and the uptake of Cu, Fe, and Zn, alongside supporting measurements of soil pH and micronutrient availability in the soil. We also measured water soluble Cu, Fe, and Zn in the soils amended with biochars pyrolyzed at different temperatures. The corn cobs biochar increased soil pH and considerably increased plant growth in the acidic soil from Tamale. In the neutral soil from Kumasi we found that, while corn cob biochar increased soil pH, rice husk biochar decreased soil pH. Furthermore, corn cob biochar considerably reduced plant growth in the neutral soil. The concentration of micronutrients in the edible portions of leafy greens was not greatly affected by biochar application, but the total uptake (i.e., concentration multiplied by biomass) of micronutrients into leaves was generally increased by biochar application in the acidic (Tamale) soil and application of the corn cob biochar generally decreased total uptake of micronutrients in the neutral (Kumasi) soil. Our results highlight the need for site-specific information on biochar feedstock and soil pH prior to recommending biochar application to tropical urban soils so that the benefits can be optimized and unintended consequences can be prevented.
Introduction
Yield gaps are large for food crops in Sub-Saharan Africa and primarily limited by poor soil fertility and nutrient availability (Van Ittersum et al., 2016). The intensification of agricultural practices alongside lack of investment in inputs (e.g., lime and fertilizers) has resulted in negative nutrient balances, acidification, and declining soil organic matter levels. Even if organic matter is available, a large quantity is required over a long period of time before soils respond to fertilizer additions, trapping smallholders in a “poverty trap” (Tittonell and Giller, 2013). Compared to other countries in Sub-Saharan Africa, Ghana has an above average proportion of the population in urban areas and an above average rate of rural-to-urban migration (De Brauw et al., 2014). The population density of Ghana has increased from 29 persons/km2 in 1960 to 103 persons/km2 in 2010, and a doubling of the proportion of the population living in urban areas from 23% in 1960 to 51% in 2010 [Ghana Statistical Service (GSS), Ghana Health Service (GHS), and ICF International, 2015]. The region is also a global hotspot of micronutrient deficiency (often referred to as the “hidden hunger”), largely due to shifting diets toward fast foods with naturally low micronutrient availability because these crops store more easily. Micronutrient deficiencies are known causes of anemia and stunting in Ghana (Anin et al., 2020; Lemoine and Tounian, 2020). Locally grown traditional leafy greens (e.g., Amaranthus and Corchorus) and exotic crops introduced from Europe (e.g., Lettuce) are therefore an important component of urban diets because they are a rich source of Vitamin A and C and micronutrients such as Cu, Fe, and Zn (Kamga et al., 2013; Achigan-Dako et al., 2014; Grubben et al., 2014; Aworh, 2018), but cannot be stored, so must be grown locally.
Biochar is a carbon-rich material produced from the pyrolysis of biomass. The pyrolysis temperature and the composition of feedstock material significantly influence the properties of biochar (Tan et al., 2015). A recent meta-analysis indicated that, while biochar has been shown to have no overall effect on crop yield in temperate soils, application to tropical soils results in an overall increase in crop yields, due to the liming effect resulting from an increase in soil pH (Jeffery et al., 2017). Greater cation exchange capacity and water retention also play a role. Unlike the long term commitment to organic amendments that is required to restore soil organic matter levels, the benefits of biochar application are typically observed within the first year of application and (due to its recalcitrant nature) are likely to persist for several years and sequester atmospheric carbon (Liu et al., 2014). Therefore, the application of biochar to soils offers a considerable “climate-smart” opportunity to improve soil fertility and the resilience of smallholder farming systems in the tropics, and increase food security (Schmidt et al., 2021). Due to their small scale, urban farms provide an ideal setting to deploy biochar to improve soil fertility, water retention, and crop yield and quality (Gwenzi et al., 2015). Therefore, experimentation with biochar made in kilns that farmers can use and with crops grown by smallholder urban farmers is necessary.
There is considerable evidence to show that biochar is capable of adsorbing high concentrations of metal cations such as Cu and Zn (Rodríguez-Vila et al., 2018) and reducing metal availability and uptake by plants from soil (Sizmur et al., 2016). This reduction in availability is largely due to an increase in soil pH and is a function of the biochar pyrolysis temperature (Gomez-Eyles et al., 2013). However, while the addition of biochar to uncontaminated temperate soils has resulted in no change in the uptake of micronutrients (Cu, Fe, and Zn) by grasses (Schimmelpfennig et al., 2015), the influence of biochar addition to acidic tropical soil on the availability and plant uptake of micronutrients into leafy greens grown on urban farms has not been investigated. It is critical that biochar applications do not exasperate the “hidden hunger” by reducing mobility and uptake of micronutrients by food crops that are vital for urban food security. We hypothesized that the increase in soil pH resulting from biochar additions to urban soils may reduce the mobility and plant uptake of key micronutrients, such as Cu, Fe and Zn, into the edible portions of leafy greens.
This study aimed to evaluate the effect of biochar application on micronutrient availability in two contrasting tropical soils collected from urban farms in Tamale and Kumasi, respectively, in Ghana. We produced biochars from corn cob, rice husk, and teak sawdust using a laboratory furnace under defined temperatures and also using kilns that are available to farmers (ELSA barrel and Japanese Retort Stove). We used the biochar made in kilns that are available to farmers to conduct pot experiments to identify the influence of biochar on the availability and uptake of Cu, Fe, and Zn. We grew leafy greens (Amaranthus, Corchorus, and Lettuce) in an acidic tropical soil (pH 4.5) from Tamale and a neutral tropical soil (pH 6.9) from Kumasi and measured their growth and the concentration of micronutrients in their leaves, alongside supporting measurements of soil pH and micronutrient availability in the soil.
Materials and Methods
Soils and Biochars
Soils were collected from small urban vegetable farms within the two major Ghanaian cities of Kumasi and Tamale. The Kumasi soil was collected from within the grounds of Kwame Nkrumah University of Science and Technology (6°40′13.1″N 1°34′05.8″W) and the Tamale soil was collected from a large open space adjacent to the city parks and gardens (9°24′47.2″N 0°51′07.3″W). Each soil sample was air-dried and sieved to <2 mm prior to analysis in triplicate for pH, electrical conductivity (EC), loss on Ignition (LOI), texture, water holding capacity (WHC), total C and N, total (aqua regia digestible) P, K, Mg, S, Ca, Mn, Cu, Fe, and Zn and EDTA extractable Mn, Cu, Fe, and Zn (Table 1). Analytical methods are described below in Section Analytical Methods.
Biochars were produced from three different feedstock materials: corn cobs (Zea mays), rice husks (Oryza sativa) and teak sawdust (Tectona grandis) obtained from farms and sawmills in Ghana. Feedstock underwent no pre-treatment prior to pyrolysis. The feedstocks were used to make biochar in a laboratory environment at 300, 500, and 700°C. The feedstock was loaded into a steel container (diameter 10 cm, height 18.2 cm) and covered with a lid with a small hole in the top to prevent over-pressure. The container was heated in a Carbolite furnace to the desired temperature and then held for 1 h and allowed to cool overnight before removing the biochar. The same feedstocks were pyrolyzed using methods by which farmers have been trained (Steiner et al., 2018), where temperature cannot be specified or precisely controlled. Corn cobs were pyrolyzed using an ELSA barrel since this is the best locally available kiln for this feedstock. Whole corn cobs were loaded into an inner steel container (diameter 43 cm, height 78 cm), lowered into an oil drum (diameter 60 cm, height 97 cm), and covered with a lid with a chimney (height 74 cm, opening width 20 cm) The feedstock was packed around the outside with “starter material” that was burned, heating the feedstock until the fire subsided and then the resulting biochar was quenched with water. Teak sawdust and rice husk were pyrolyzed using a Japanese Retort Stove (also referred to as a Kun-tan) because this is the best locally available kiln for this feedstock since feedstocks with small particle sizes result in an uneven pyrolysis in an ELSA barrel. A small fire was started and covered with an inverted steel cone (60 × 40 cm) with a chimney (60 cm high) attached. Holes measuring 2 cm were punched 5 × 5 cm apart. The feedstock was piled up around the hot cone and continuously rotated until all the feedstock was black in color before quenching with water. Prior to analysis, all biochars were ground to a fine powder using a TEMA mill (Laboratory Disc Mill T100ACH). The biochar samples were analyzed in triplicate for pH, electrical conductivity (EC), total C and N, and total P, K, Mg, S, Ca, Mn, Cu, Fe, and Zn (Table 2). Analytical methods are described below in Section Analytical Methods.
Water Extractable Micronutrients
To measure the water solubility of micronutrients in soil-biochar mixtures, 3.8 g of either Kumasi or Tamale soil (air dried and 2 mm sieved) was weighed into a polypropylene centrifuge tube along with 0.2 g of finely ground biochar (apart from the unamended control treatment, which received only the 3.8 g of soil). Each of the 12 biochars was combined with both soils in triplicate, resulting in 26 experimental treatments and 78 experimental units. Based on the method described by Sizmur et al. (2011), 40 ml of ultrapure water was added to each centrifuge tube. Samples were placed on an end-over-end shaker at 30 rpm for 16 h at 20°C and then pH of the biochar/soil slurry was analyzed with a Jenway 3310 pH meter. The tubes were immediately centrifuged at 3,600 rpm for 10 min and the supernatant filtered through 0.45 μm syringe filters. Dissolved Organic Carbon (DOC) was analyzed in the filtered supernatant using a Shimadzu TOC-L analyzer. A 10 mL sub-sample of the filtered supernatant was acidified with 5% HNO3 and analyzed for Fe, Cu, and Zn on a ThermoFisher Scientific iCAP Q Inductively Coupled Plasma Mass Spectrometer (ICP-MS).
Plant Pot Experiment
Seeds of Amaranthus, Corchorus, and Lettuce (var Eden F1) were germinated in a coco coir medium for 1 week, after which plants for which three true leaves were observed were transplanted into experimental 3 liter pots (n = 9). One kilogram of air-dried, 2 mm sieved soil from either Kumasi or Tamale was added to each plot, and amended with 50 g of either corn cobs biochar made using the ELSA barrel, rice husk biochar made using the Japanese retort stove, or sawdust biochar made using the Japanese retort stove at a rate equivalent to 5% (w/w). Plants were transplanted shortly after the biochar was mixed with the soil. The experiment with the Kumasi soil was undertaken prior to the experiment undertaken with the Tamale soil, and so direct statistical comparisons should not be made between the soil types. The rice husk and sawdust biochars were not ground prior to addition to the pots since they already had a small enough particle size to ensure homogenous mixing. The corn cobs biochar (where feedstock was much more bulky) was milled with a hammer mill to a size of 0.3–0.5 mm prior to addition to the Kumasi soil, but was crushed with a wooden mallet to a size of 1–3 mm (which better reflected the particle size of the rice husks and sawdust) prior to addition to the Tamale soil. Each experiment included 10 treatments, each with 9 replicate pots, resulting in a total of 90 pots per experiment, and 180 pots in total.
Plants were reared in a polytunnel in the Department of Horticulture on the campus of Kwame Nkrumah University of Science and Technology, Kumasi, Ghana. They were watered daily with 200 ml of tap water per pot, regardless of soil type, crop size, or air temperature. No fertilizer was applied. Weekly assessments were made of plant growth. The number of leaves on each plant was counted and the height measured using a ruler. The girth of the stems of Amaranthus and Corchorus plants was measured using a Vernier caliper at 5 cm from the surface of the soil (data not reported in the paper, but included in the online dataset).
After 4 weeks the plants were harvested by cutting the stem at ground level. The leaves were removed from the stem and the fresh weight of both the leaves and the stem were taken. Leaves were dried, at 60°C overnight in paper envelopes, re-weighed, and then milled prior to analysis for Fe, Cu, and Zn using Inductively Coupled Plasma Optical Emission Spectroscopy (ICP-OES) after microwave digestion. Three pots per treatment were randomly selected and the soils remaining in each pot were homogenized and one ~50 g sub-sample per pot air dried prior to analysis of EDTA extractable Fe, Cu, and Zn using ICP-OES.
Analytical Methods
Soil moisture content was determined by mass lost after drying soil samples in an oven for ~24 h at 105°C. Loss on ignition was determined by mass lost after oven-dry samples were combusted in a muffle furnace for ~24 h at 500°C. Texture and particle size distribution were determined using a Malvern Mastersizer 3000 laser granulometer. Soil water holding capacity was determined after completely saturated soils were drained gravimetrically for 24 h and then mass lost was quantified after drying in an oven for ~24 h at 105°C.
Soil and biochar pH was determined with a Jenway 3310 pH meter, calibrated with pH 4 and 7 buffers. 2.5 g of sample was shaken with 25 ml of ultra-pure water on a rotary shaker for 15 min. Electrical conductivity was analyzed using a Jenway 4310 conductivity meter. Total C and N content of soils and biochars were analyzed using a Thermo Scientific Flash 2000 Carbon Nitrogen analyzer, calibrated with 1 and 3 mg samples of an aspartic acid standard.
EDTA extractable concentrations in soils were analyzed by ICP-OES. 2.5 g of sample was shaken on a rotary shaker for 1 h at 20°C, with 25 ml of 0.05M EDTA. Suspensions were then centrifuged for 10 min at 3,600 rpm and the supernatants were filtered through a Whatman no. 540 filter paper. Total concentrations of elements in soils were determined by digesting soil samples in reverse aqua regia using the MARS 6 microwave digestion system. Our method was based on US-EPA Method 3,051A—Microwave assisted acid digestion of sediment, sludges, soils and oils. 0.5 g of sample was weighed into MARSXpress digestion tubes, and 9 ml of trace element grade concentrated nitric acid (HNO3) and 3 ml of trace element grade concentrated hydrochloric acid (HCl) were added to each tube. Samples were digested using the MARS 6 microwave digestion system (the method ramped the tubes to 175°C for 5 min 30 s and then held them at 175°C for 4 min 30 s). Digestates were filtered through Whatman no. 540 filter paper and analyzed by ICP-OES.
Total concentrations of elements in plants were determined by digesting plant samples in nitric acid using the MARS 6 microwave digestion system. 0.5 g of sample was weighed into MARSXpress digestion tubes, and 2 ml of ultra-pure water and 8 ml of Trace Element Grade concentrated nitric acid (HNO3) were added to each tube. Samples were digested using the MARS 6 microwave digestion system (the method ramped the tubes to 200°C for 20–25 min and then held them at 200°C for 10 min). Digestates were filtered through Whatman no. 540 filter paper and analyzed by ICP-OES.
Total concentrations of elements in biochar were determined by digesting biochar samples in nitric acid using a digestion block. 0.5 g of sample was weighed into 100 ml Kjeldahl digestion tubes and 10 ml of Trace Element Grade concentrated nitric acid (HNO3) were added to each tube. Samples were heated to 60°C and held for 3 h before raising to 110°C and digested for 6 h. Digestates were filtered through Whatman no. 540 filter paper and analyzed by ICP-OES.
Quality Control
For total C and N content, soils and biochars were analyzed alongside one blank every 20 samples and 10 mg internal reference material (105 ± 0.2% recovery for C and 101 ± 2.5% recovery for N), traceable to certified reference materials AR4016, Alpha Resources (certified for C) and GBW07412, State Bureau of Technical Supervision, China (certified for N). For EDTA extractions, reverse aqua regia digestions of soils, and nitric acid digestion of plant material, samples were analyzed alongside internal reference materials which are traceable to certified reference materials. Blanks were included in all extractions and digestions and values were subtracted from sample results if they exceeded the detection limit.
Statistical Analysis
Statistical analyses were carried out using SPSS, including Analysis of Variance (ANOVA) to calculate the difference in means between samples. Dunnett T3 and Tukey's post-hoc tests were used for mean comparisons. Statistical significance was determined at 95% confidence interval with the significance level at p < 0.05.
Results
Soil pH and DOC in Biochar-Amended Soils
The pH of Kumasi and Tamale soil samples were found to generally increase with the addition of biochar and increase with increasing biochar pyrolysis temperature (Figures 1A,B). A 5% (w/w) amendment of Tamale soil with corn cob, rice husk and teak sawdust biochar significantly (p < 0.05) increased the soil pH from 4.89 to 6.92, 5.47, and 6.13, respectively (Figure 1B). Conversely, only the corn cob biochar made using the ELSA barrel significantly (p < 0.05) increased the soil pH of the Kumasi soil from 7.24 to 8.47. While the amendment of soils with biochars produced in the laboratory at 300°C significantly (p < 0.05) increased the DOC, there was a general trend that DOC in water extracts decreased with increasing biochar pyrolysis temperature (Figures 1C,D).
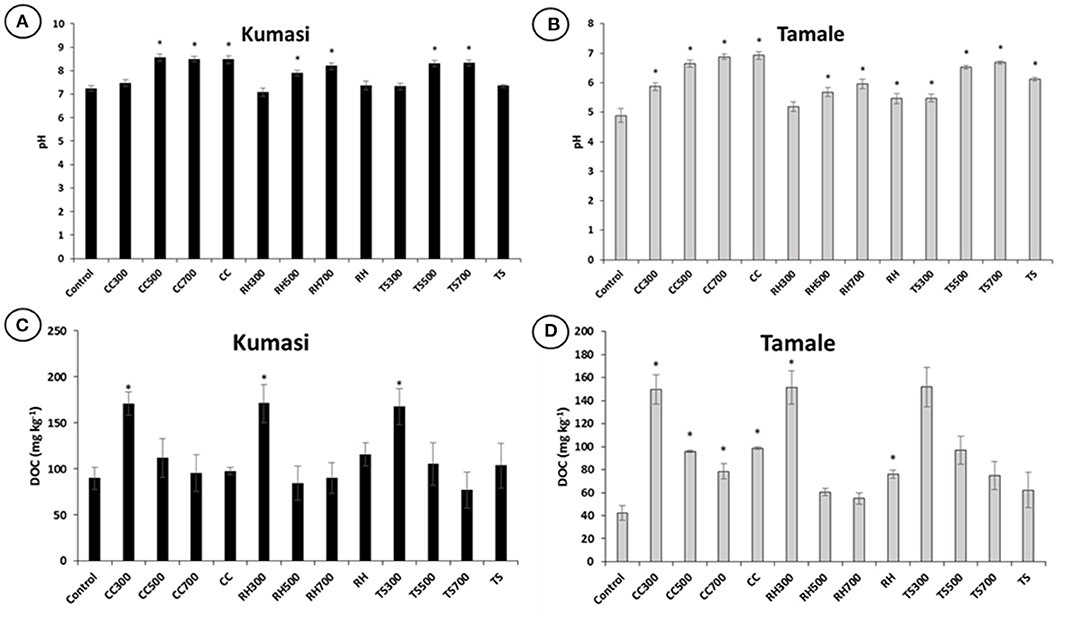
Figure 1. Biochar influence on soil pH and DOC in water extracts in Kumasi (A,C) and Tamale (B,D) soil. The control treatment comprised no amendment. CC, RH and TS represent treatments amended with 5% (w/w) corn cob biochar, rice husk biochar, and teak sawdust biochar, respectively, made in the laboratory at 300, 500, or 700°C, or made in the field using an ELSA barrel (CC) or a Japanese retort stove (RH and TS). Data with asterisks are statistically significantly different from control (p < 0.05). Error bars represent standard deviation (n = 3).
Water Extractable Fe, Cu, and Zn Concentrations in Biochar-Amended Soils
While there are few statistically significant differences between biochar-amended soils and unamended control soils in terms of water extractable Cu and Zn, biochars tended to decrease water extractable Fe in the Kumasi soil (Figure 2A) and increase water extractable Fe in the Tamale soil (Figure 2B). Some results for Zn concentrations in teak sawdust amended soils were below detection limit (0.08 mg kg−1) (Figures 2E,F). Whereas the Fe concentrations of the soils (Table 1) are much higher than the Fe concentrations of the biochars (Table 2), the Zn concentrations of the corn cob biochars (Table 2) are greater than the Zn concentrations of the soils (Table 1), and thus may contribute directly to the water extractable Zn (Figures 2E,F).
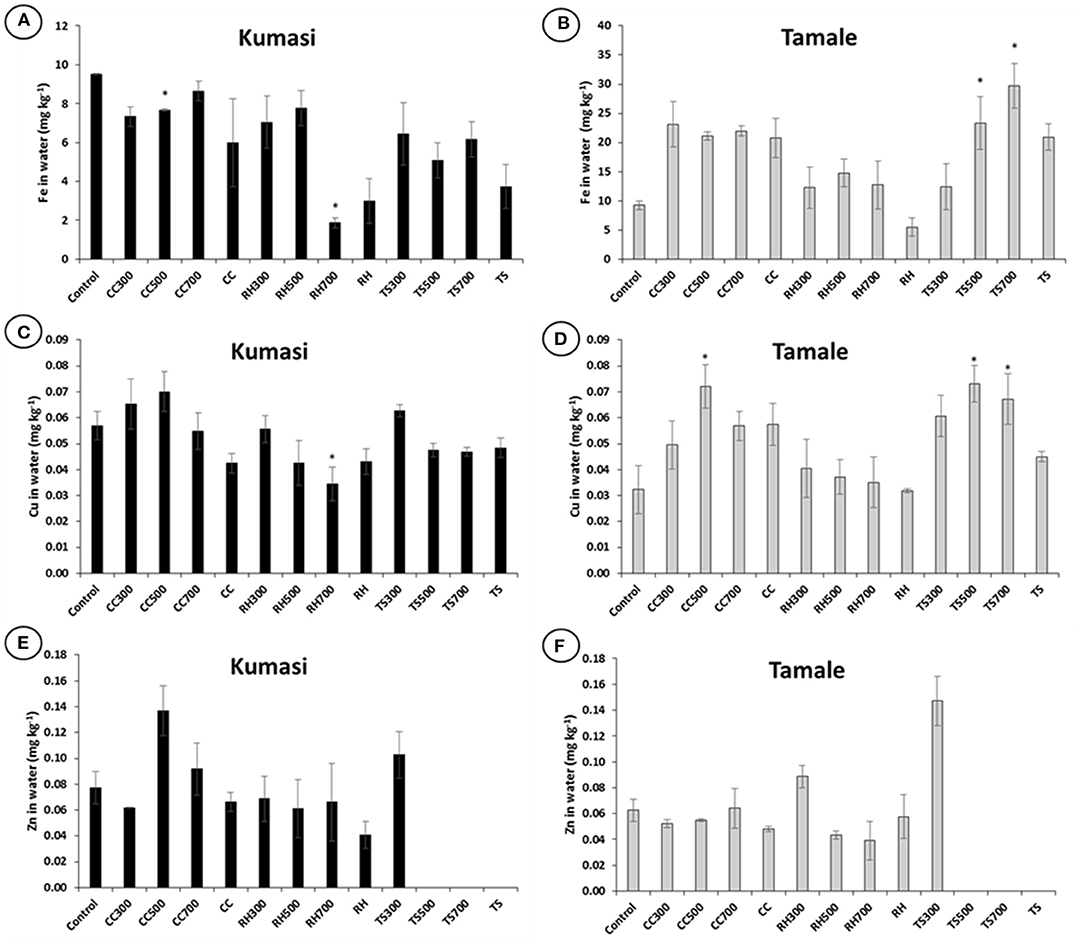
Figure 2. Biochar influence on Fe, Cu, and Zn concentrations in water extracts in Kumasi (A,C,E) and Tamale (B,D,F) soil. The control treatment comprised no amendment. CC, RH and TS represent treatments amended with 5% (w/w) corn cob biochar, rice husk biochar, and teak sawdust biochar, respectively made in the laboratory at 300, 500, or 700°C, or made in the field using an ELSA barrel (CC) or a Japanese retort stove (RH and TS). Some measurements Zn concentrations in water extracts of teak sawdust biochar amended soils were below detection limit (0.08 mg kg−1). Data with asterisks are statistically significantly different from control (p < 0.05). Error bars represent standard deviation n = 3.
EDTA Extractable Fe, Cu, and Zn Concentrations in Biochar-Amended Soils
All three biochars significantly (p < 0.05) reduced EDTA-extractable Fe in Kumasi soil grown with Amaranthus and Lettuce (Figure 3A), and EDTA-extractable Cu in Kumasi soil grown with Amaranthus, Corchorus and Lettuce (Figure 3C). Corn cob biochar significantly (p < 0.05) increased EDTA-extractable Zn in Kumasi soil grown with Amaranthus (Figure 3E). The teak sawdust biochar significantly (p < 0.05) reduced EDTA-extractable Fe in Tamale soil grown with Amaranthus, Corchorus and Lettuce but corn cob and rice husk biochars significantly (p < 0.05) increased EDTA-extractable Fe in Tamale soil grown with Lettuce (Figure 3B). Rice husk biochar significantly (p < 0.05) reduced EDTA-extractable Cu in Tamale soil grown with Amaranthus, and corn cob and teak sawdust biochars significantly (p < 0.05) increased EDTA-extractable Cu in Tamale soil grown with Lettuce (Figure 3D). Corn cob biochar also significantly (p < 0.05) increased EDTA-extractable Zn in Tamale soil grown with Lettuce (Figure 3F).
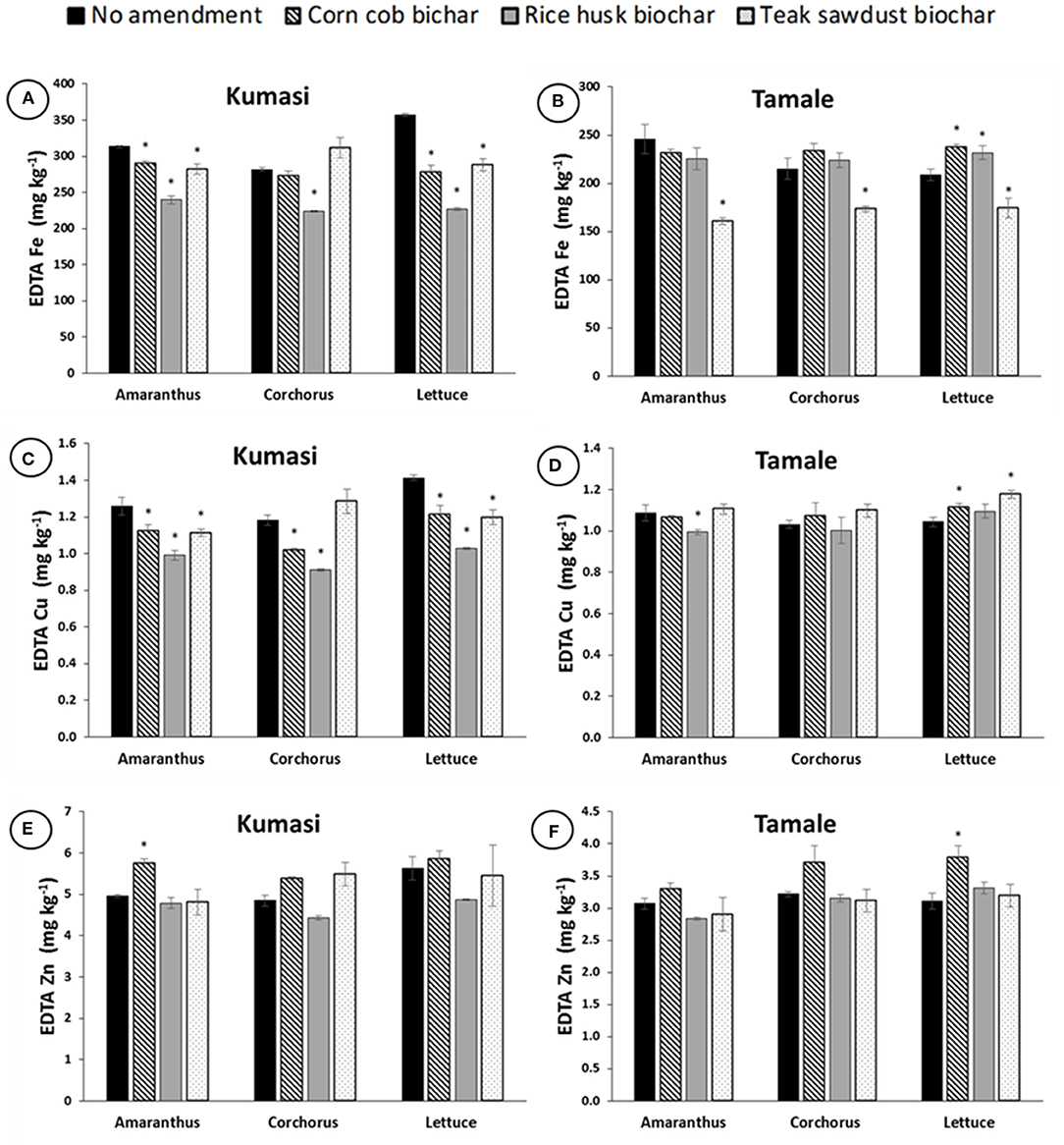
Figure 3. Fe, Cu, and Zn concentrations in EDTA extracts of Kumasi (A,C,E) and Tamale (B,D,F) soils planted with Amaranth, Corchorus and Lettuce and amended with 5% (w/w) corn cob biochar, rice husk biochar, or teak sawdust biochar. Data with asterisks are statistically significantly different from control for each crop (p < 0.05). Error bars represent standard deviation n = 3.
Soil pH Impacts on Leafy Green Growth in Biochar-Amended Soils
The corn cob biochar significantly (p < 0.05) increased the pH of both the Kumasi and Tamale soils used in the polytunnel experiment (Figures 4A,B), but the rice husk and teak sawdust biochars increased the pH of the Tamale soil and decreased the pH of the Kumasi soil. Corn cob biochar amended soil had the highest pH (pH >8) in Kumasi soil (Figure 4A), and teak sawdust biochar amended soil had the highest pH (pH ~6.5) in Tamale soil (Figure 4B). Plant biomass and the number of leaves for biochar amended soil was similar to, or lower than, the unamended control in Kumasi soil (Figure 4C), with considerable and significant (p < 0.05) reductions in biomass due to the addition of corn cob biochar. Amendment with all three biochars increased plant biomass and the number of leaves of Amaranthus, Corchorus and Lettuce grown in Tamale soil (Figure 4D), where significant (p < 0.05) increases in soil pH were also found (Figure 4B). Considerable significant (p < 0.05) increases in both the number of leaves (Figure 4F) and biomass (Figure 4D) of Amaranthus were observed with a 5% (w/w) amendment of Tamale soil with either corn cob, rice husk, or teak sawdust biochar.
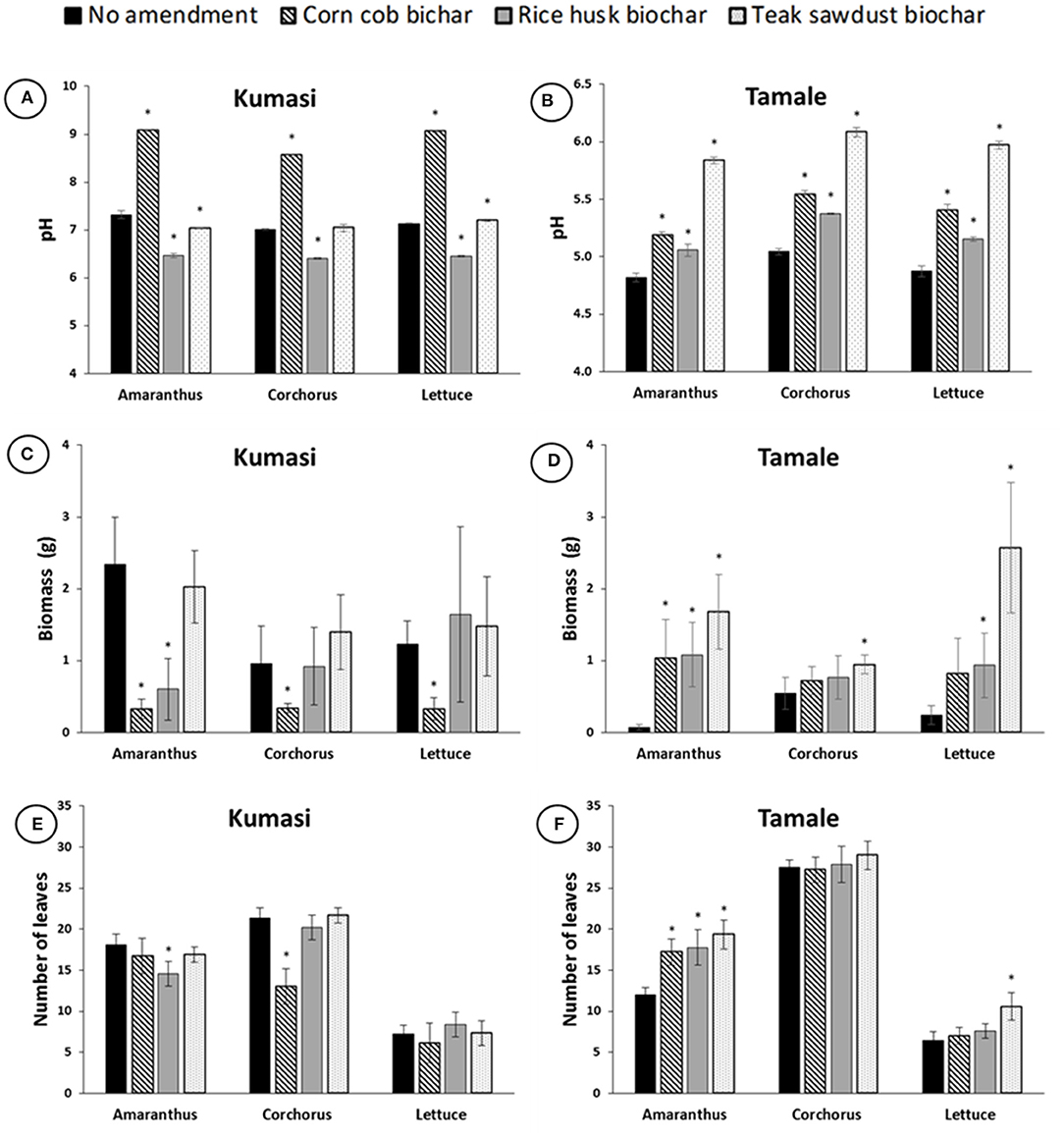
Figure 4. Soil pH, leaf biomass per pot, and number of leaves per pot 4 weeks after planting, for Amaranth, Corchorus, and Lettuce grown in Kumasi (A,C,E) and Tamale (B,D,F) soils amended with 5% (w/w) corn cob biochar, rice husk biochar, or teak sawdust biochar. There was one plant per pot in the greenhouse experiments (n = 9). Data with asterisks are statistically significantly different from control for each crop (p < 0.05). Error bars represent standard deviation n = 3.
Micronutrient Uptake by Leafy Greens in Biochar-Amended Soils
Although a direct statistical comparison cannot be made, plants growing in Tamale soil tended to have greater concentrations of Fe, Cu, and Zn in their edible portions than the plants grown in Kumasi soil and the differences in micronutrient content of plants grown in the different soils were generally larger than the differences observed due to the amendment of the soils with biochar (Figure 5). For Kumasi soil, corn cob biochar significantly (p < 0.05) increased the concentration Cu and Zn in Amaranthus leaves and Zn in Corchorus leaves (Figures 5C,E), but significantly (p < 0.05) reduced Fe and Cu concentration in Corchorus leaves and Zn concentration in Lettuce leaves (Figures 5A,C,E). Teak sawdust biochar significantly (p < 0.05) reduced Fe in Amaranthus and Cu in Corchorus leaves in Kumasi soil (Figures 5A,C). Amendment of the Kumasi soil with rice husk biochar significantly (p < 0.05) reduced Fe, Cu, and Zn concentration in Amaranthus and Corchorus leaves, and Zn in Lettuce leaves (Figures 5A,C,E). Teak sawdust biochar significantly (p < 0.05) increased Fe concentration in Amaranthus and Lettuce leaves and Cu concentration in the leaves of Lettuce grown in Tamale soil (Figures 5B,D), but significantly (p < 0.05) reduced Cu concentration in Amaranthus leaves, and Zn concentration in Amaranthus, Corchorus and Lettuce leaves grown in Tamale soil (Figures 5D,F). Corn cob and rice husk biochars increased Cu concentrations in Lettuce leaves grown in Tamale soil (Figure 5D).
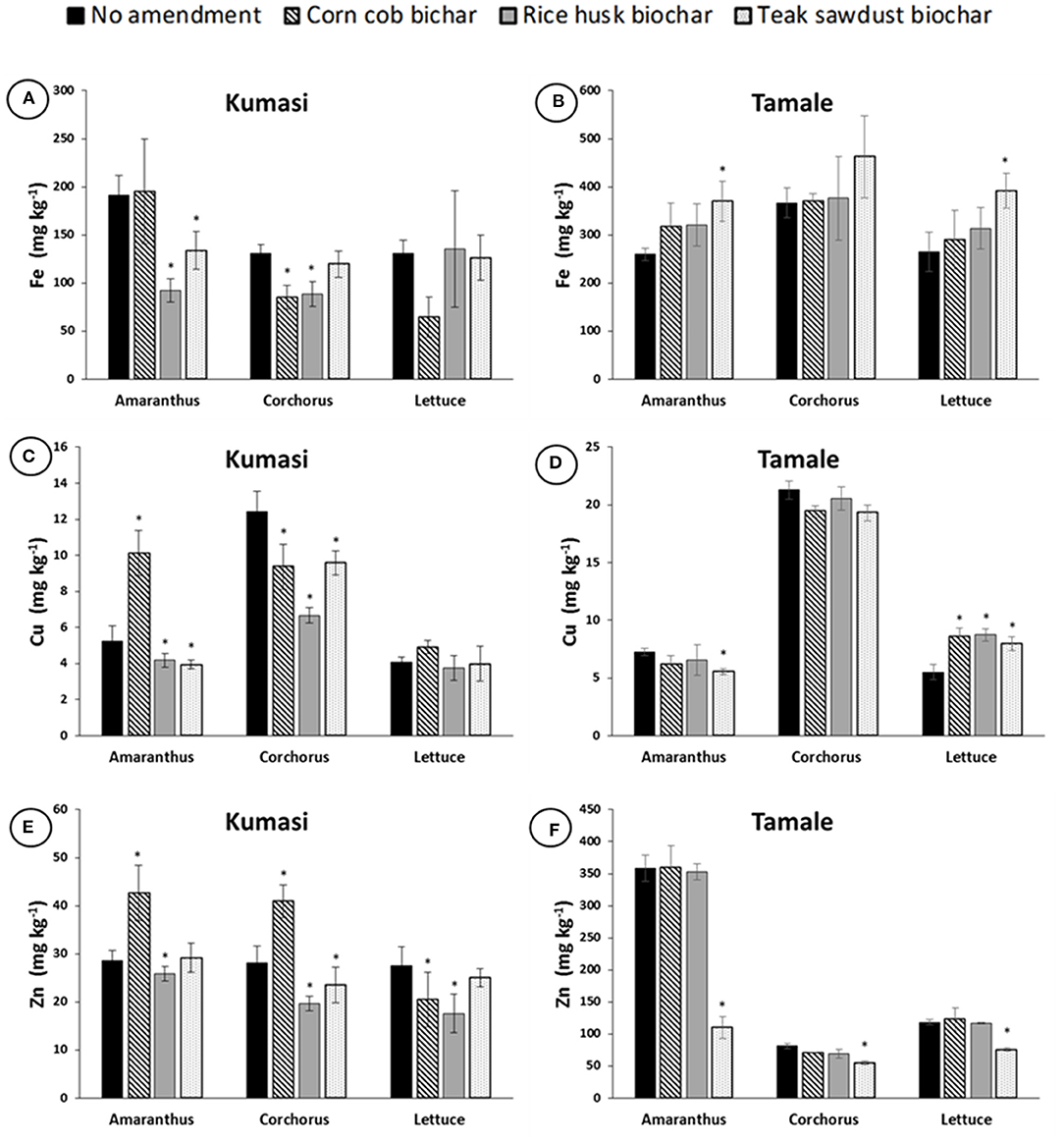
Figure 5. Fe, Cu, and Zn concentrations in Amaranth, Corchorus and Lettuce grown in Kumasi (A,C,E) and Tamale (B,D,F) soils amended with 5% (w/w) corn cob biochar, rice husk biochar, or teak sawdust biochar. Data with asterisks are statistically significantly different from control for each crop (p < 0.05). Error bars represent standard deviation n = 3.
When micronutrient concentration was multiplied by leaf biomass, the total plant uptake of Fe, Cu, and Zn into Amaranthus, Corchorus, and Lettuce leaves can be calculated and the effect of biochar amendment on total plant uptake of micronutrients is shown in Figure 6. Generally, plant uptake of Fe, Cu, and Zn from biochar amended Kumasi soil was similar or lower than from the unamended control (Figures 6A,C,E). However, biochars generally increased total plant uptake of Fe, Cu, and Zn into Amaranthus, Corchorus, and Lettuce leaves grown in Tamale soil (Figures 6B,D,F). These differences are driven more by the impacts of biochar on plant biomass (Figure 4) than the impacts of biochar on Fe, Cu, and Zn concentrations in the leaves of the plants (Figure 5).
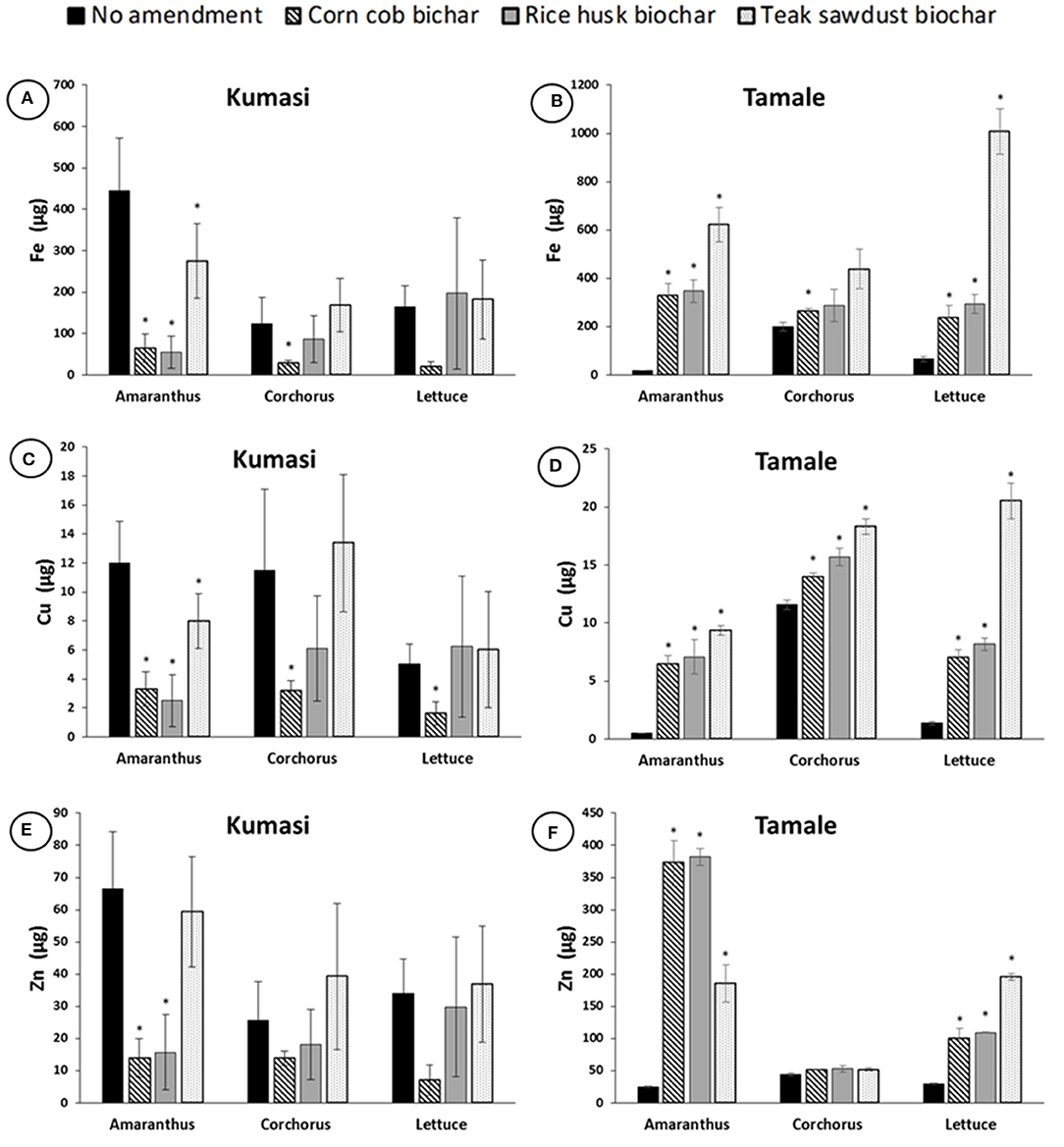
Figure 6. Plant uptake of Fe, Cu, and Zn into Amaranth, Corchorus, and Lettuce in Kumasi (A,C,E) and Tamale (B,D,F) soils amended with 5% (w/w) corn cob biochar, rice husk biochar, or teak sawdust biochar. Data with asterisks are statistically significantly different from control for each crop (p < 0.05). Error bars represent standard deviation n = 3.
Discussion
Soil pH and Micronutrient Availability in Biochar-Amended Soils
The soil pH was found to generally increase with increasing biochar pyrolysis temperature in water extracts from both Kumasi and Tamale soils amended with biochar (Figures 1A,B) in agreement with Khanmohammadi et al. (2015) and Pariyar et al. (2020). If we compare the pH of Kumasi soil amended with the three biochars produced using the ELSA barrel (corn cobs) and Japanese retort stove (rice husk and teak sawdust), we observe that corn cob biochar significantly increased soil pH, but rice husk and teak sawdust did not (Figure 1A). It is therefore possible that this increase in pH observed can be due to the type of feedstock or the pyrolysis method used to produce the corn cob biochar. The corncob biochar resulted in significantly (p < 0.05) greater pH in both the Kumasi and Tamale soils remaining in pots at the end of the plant pot experiment (Figure 4). Although a direct statistical comparison cannot be made between the two pot experiments, we observed that the corn cob biochar produced the highest increase of pH in neutral Kumasi soil compared to the unamended control (Figure 4A), but not in acidic Tamale soil (Figure 4B). For the first plant pot experiment, corn cob biochar applied to Kumasi soil was milled, but in the second experiment corn cob biochar applied to Tamale soil was crushed so that the particle size was more similar to that or rice husks and sawdust. This may explain the differences in pH between Kumasi and Tamale soils amended with corn cob biochar in the plant pot experiment, since milling increases biochar surface area and can influence biochar pH (Lyu et al., 2018; Leng et al., 2020). The different influence of different biochars on the soil pH supports the possibility that individual feedstocks could be used to manipulate different soils to target a specific pH range that is suitable for growing specific crops and a decision support tool could be used to recommend biochar type and rates (Phillips et al., 2020).
The addition of biochar to soils had a relatively minor impact on the water extractable (Figure 2) or EDTA extractable (Figure 3) Fe, Cu, and Zn concentrations. However, some of the treatments with the highest water extractable Cu concentrations in Kumasi soil (CC300, RH300, and TS300; Figure 2C) also had the highest DOC concentrations (Figure 1C). The water extractable Cu concentrations could therefore partially be explained by DOC concentrations since Cu binds strongly with DOC in solution, resulting in more Cu desorbing from soil surfaces and establishing a new equilibrium with a greater amount of Cu in solution (Zhao et al., 2007). There is also a general trend that DOC in water extracts decreased with increasing biochar pyrolysis temperature (Figure 1), in agreement with Gui et al. (2020) and Wang et al. (2021). The only significant impact of biochar on water soluble Cu in Kumasi soil was the significant reduction observed in treatment RH700. It is possible that the biochars contribute with Zn to the soils due to the biochar composition. It has been suggested that that some biochars may release heavy metals (including Zn, which is both a heavy metal and a micronutrient) to the extent that it causes toxicity (Kookana et al., 2011). However, the concentrations of Zn for all the biochars were generally an order of magnitude lower than the limits set by the European Biochar Certificate (Schmidt et al., 2016). Other authors have also observed a decrease in exchangeable Fe after biochar amendment, alongside an increase in pH (Ch'ng et al., 2016). However, while chemical extractions (including EDTA extracts and water soluble extractions) are useful for determining how amendments influence micronutrient solubility, they are not necessarily representative of plant uptake and this is particularly the case with Fe. Most Fe in soil is present as Fe(III), mostly precipitated as oxides, hydroxides, and oxyhydroxides, and is generally not very soluble and this is insufficient to satisfy plant demand. Therefore, plants regulate Fe availability and achieve Fe uptake through rhizosphere processes that include acidification, chelation and reduction of Fe(III) in the soil and then uptake of Fe(II) from the soil solution (Robin et al., 2008). It is therefore unlikely that the Fe concentrations in the water extractable or EDTA extractable fractions represent the pool of Fe truly accessible to the plants. This may explain why EDTA extractable Fe and water extractable Fe do not reflect plant uptake.
Plant Growth and Micronutrient Uptake in Biochar-Amended Soils
The application of biochar considerably increased the leaf biomass in the pH 4.5 Tamale soil, but decreased the leaf biomass in the pH 6.9 Kumasi soil (Figure 4). This effect can largely be explained by the starting pH of the soil and the pH of the soil after biochar amendment, although other differences between the soils cannot be ruled out. The application of corn cob biochar to the Kumasi soil raised the pH to >9 and this was reflected by significantly lower Amaranthus, Corchorus, and Lettuce leaf biomass. While it has been well demonstrated that there is a greater potential to increase crop yields in acidic soils than neutral soils due to a liming effect (Jeffery et al., 2011), the idea that biochar increases crop yields in tropical soils (Jeffery et al., 2017) may be a little too simplistic. The negative impact of inappropriate biochar application on pH neutral tropical soils is less well publicized than its beneficial impacts in acidic soils (Xu et al., 2012; Manso et al., 2019). A single application of biochar can influence soil properties and plant performance for several years afterwards (Frimpong et al., 2021). Therefore, advice to farmers on the application of biochar to soils must be site-specific and effectively predict the influence that the biochar application will have on the pH of the soil.
While there has been considerable focus on the impact of biochar on crop yields, the nutritional quality of the crops has not been so well studied, particularly with regard to micronutrients. We found that the concentration of Fe, Cu, and Zn in the edible portions of leafy greens was not greatly affected by biochar application (Figure 5), but (after multiplying concentration by biomass) the total uptake of Fe, Cu, and Zn was, generally speaking, increased by all three biochars in the acidic Tamale soil and, generally speaking, decreased by the corn cobs biochar in the neutral Kumasi soil (Figure 6). Since biochars are known to have a high surface area, which increases with pyrolysis temperature (Tomczyk et al., 2020), we hypothesized that the surface of the biochar may adsorb metal ions and prevent their uptake into plants, as has been observed in soils contaminated with Cu and Zn (Namgay et al., 2010; Kim et al., 2015; Puga et al., 2015). However, as we discuss above, the biochar had relatively little impact on micronutrient solubility and it is clear that the leafy greens are able to regulate their internal micronutrient concentrations and acquire sufficient Cu, Fe, and Zn. The concentrations required for good nutritional status vary considerably between different varieties, but there were no indications that any of the plants grown in this experiment were nutrient deficient. Therefore, the biomass of the plant was the primary driver of the total micronutrient extraction from the soil.
Conclusions
The results of this study revealed that biochar increased soil pH and considerably increased plant growth in the acidic (pH 4.5) urban soil from Tamale. It is therefore appropriate to recommend the application of biochars to this soil and the 5% (w/w) amendment with teak sawdust biochar is particularly recommended since it increased Amaranth, Corchorus and Lettuce biomass by 2,194, 173, and 1,032%, respectively. However, in the neutral (pH 6.9) urban soil from Kumasi soil pH was increased to >9 after amendment with corn cob biochar and this resulted in a decrease in the leaf biomass of Amaranthus, Corchorus, and Lettuce to 15, 36, and 25% of the unamended biomass, respectively. The concentrations of micronutrients (Cu, Fe, Zn) in the soil solution and the edible portions of leafy greens were not greatly affected by biochar application, but the total uptake of micronutrients from the soil (multiplying leaf micronutrient concentration by leaf biomass) was increased by biochar application to the acidic soil and decreased in the neutral soil, proportional to the changes in biomass. We therefore do not identify concerns that biochar application reduces the nutritional quality of food crops. However, our results highlight the need for site-specific information on soil pH prior to recommendations on biochar application to tropical soils. Soil pH was found to generally increase with increasing biochar pyrolysis temperature and the three biochars increased pH to a different extent. Therefore, recommendations on the appropriate application rates of particular biochars pyrolyzed at particular temperatures for specific soils would optimize benefits and prevent unintended and potentially irreversible consequences of raising the pH above optimum levels.
Data Availability Statement
The datasets presented in this study can be found online: Rodríguez-Vila et al. (2022), https://doi.org/10.17632/pkys2f8288.2.
Author Contributions
TS, LA, and AA conceived the research. TS, LA, AA, JH, SR, and AR-V designed the experiments and interpreted the data. AR-V, DA, AA-K, and SC conducted the experiments. AR-V analyzed the data and created the figures and tables. AR-V and TS wrote the paper. All authors were given the opportunity to comment on and approved the final version of the paper.
Funding
This work was funded by the Global Challenges Research Fund allocation of the Quality-Related funding allocated to the University of Reading by Research England and awarded to Tom Sizmur in the form of a Developing Equitable Partnerships Grant. AR-V's postdoctoral fellowship at the University of Reading was sponsored by the Government of Galicia.
Conflict of Interest
The authors declare that the research was conducted in the absence of any commercial or financial relationships that could be construed as a potential conflict of interest.
Publisher's Note
All claims expressed in this article are solely those of the authors and do not necessarily represent those of their affiliated organizations, or those of the publisher, the editors and the reviewers. Any product that may be evaluated in this article, or claim that may be made by its manufacturer, is not guaranteed or endorsed by the publisher.
Acknowledgments
We acknowledge the contributions of Anne Dudley, Andrew Dodson, and Yiran Zou for assisting with laboratory analysis.
References
Achigan-Dako, E. G., Sogbohossou, O. E. D., and Maundu, P. (2014). Current knowledge on Amaranthus spp.: research avenues for improved nutritional value and yield in leafy amaranths in sub-Saharan Africa. Euphytica 197, 303–317. doi: 10.1007/s10681-014-1081-9
Anin, S. K., Saaka, M., Fischer, F., and Kraemer, A. (2020). Association between Infant and Young Child Feeding (IYCF) Indicators and the Nutritional Status of Children (6–23 Months) in Northern Ghana. Nutrients 12, 2565. doi: 10.3390/nu12092565
Aworh, O. C. (2018). From lesser-known to super vegetables: the growing profile of African traditional leafy vegetables in promoting food security and wellness. J. Sci. Food Agric. 98, 3609–3613. doi: 10.1002/jsfa.8902
Ch'ng, H. Y., Ahmed, O. H., and Majid, N. M. A. (2016). Improving phosphorus availability, nutrient uptake and dry matter production of Zea mays L. on a tropical acid soil using poultry manure biochar and pineapple leaves compost. Exp. Agric. 52, 447–465. doi: 10.1017/S0014479715000204
De Brauw, A., Mueller, V., and Lee, H. L. (2014). The role of rural–urban migration in the structural transformation of Sub-Saharan Africa. World Dev. 63, 33–42. doi: 10.1016/j.worlddev.2013.10.013
Frimpong, K. A., Phares, C. A., Boateng, I., Abban-Baidoo, E., and Apuri, L. (2021). One-time application of biochar influenced crop yield across three cropping cycles on tropical sandy loam soil in Ghana. Heliyon 7, e06267. doi: 10.1016/j.heliyon.2021.e06267
Ghana Statistical Service (GSS) Ghana Health Service (GHS), and ICF International. (2015). Ghana Demographic and Health Survey 2014. Rockville, MD: GSS, GHS, and ICF International.
Gomez-Eyles, J. L., Beesley, L., Moreno-Jimenez, E., Ghosh, U., and Sizmur, T. (2013). The potential of biochar amendments to remediate contaminated soils. Biochar Soil Biota. 4, 100–133. doi: 10.1201/b14585
Grubben, G., Klaver, W., Nono-Womdim, R., Everaarts, A., Fondio, L., Nugteren, J. A., et al. (2014). Vegetables to combat the hidden hunger in Africa. Chronica Horticulturae 54, 24–32. Available online at: https://www.ishs.org/system/files/chronica-documents/ch5401.pdf
Gui, X., Liu, C., Li, F., and Wang, J. (2020). Effect of pyrolysis temperature on the composition of DOM in manure-derived biochar. Ecotoxicol. Environ. Safety 197, 110597. doi: 10.1016/j.ecoenv.2020.110597
Gwenzi, W., Chaukura, N., Mukome, F. N. D., Machado, S., and Nyamasoka, B. (2015). Biochar production and applications in sub-Saharan Africa: opportunities, constraints, risks and uncertainties. J. Environ. Manage. 150, 250–261. doi: 10.1016/j.jenvman.2014.11.027
Jeffery, S., Abalos, D., Prodana, M., Bastos, A. C., Van Groenigen, J. W., Hungate, B. A., et al. (2017). Biochar boosts tropical but not temperate crop yields. Environ. Res. Lett. 12, 053001. doi: 10.1088/1748-9326/aa67bd
Jeffery, S., Verheijen, F. G., van der Velde, M., and Bastos, A. C. (2011). A quantitative review of the effects of biochar application to soils on crop productivity using meta-analysis. Agric. Ecosyst. Environ. 144, 175–187. doi: 10.1016/j.agee.2011.08.015
Kamga, R. T., Kouamé, C., Atangana, A., Chagomoka, T., and Ndango, R. (2013). Nutritional evaluation of five African indigenous vegetables. J. Horticult. Res. 21, 99–106. doi: 10.2478/johr-2013-0014
Khanmohammadi, Z., Afyuni, M., and Mosaddeghi, M. R. (2015). Effect of pyrolysis temperature on chemical and physical properties of sewage sludge biochar. Waste Manag. Res. 33, 275–283. doi: 10.1177/0734242X14565210
Kim, H.-S., Kim, K.-R., Kim, H.-J., Yoon, J.-H., Yang, J. E., et al. (2015). Effect of biochar on heavy metal immobilization and uptake by lettuce (Lactuca sativa L.) in agricultural soil. Environ. Earth Sci. 74, 1249–1259. doi: 10.1007/s12665-015-4116-1
Kookana, R. S., Sarmah, A. K., Van Zwieten, L., Krull, E., and Singh, B. (2011). Biochar application to soil: agronomic and environmental benefits and unintended consequences. Adv. Agron. 112, 103–143. doi: 10.1016/B978-0-12-385538-1.00003-2
Lemoine, A., and Tounian, P. (2020). Childhood anemia and iron deficiency in sub-Saharan Africa–risk factors and prevention: a review. Arch. Pédiatrie. 27, 490–496. doi: 10.1016/j.arcped.2020.08.004
Leng, L., Xiong, Q., Yang, L., Li, H., Zhou, Y., Zhang, W., et al. (2020). An overview on engineering the surface area and porosity of biochar. Sci. Total Environ. 763, 144204. doi: 10.1016/j.scitotenv.2020.144204
Liu, X., Ye, Y., Liu, Y., Zhang, A., Zhang, X., Pan, G., et al. (2014). Sustainable biochar effects for low carbon crop production: a 5-crop season field experiment on a low fertility soil from Central China. Agric. Syst. 129, 22–29. doi: 10.1016/j.agsy.2014.05.008
Lyu, H., Gao, B., He, F., Zimmerman, A. R., Ding, C., Huang, H., et al. (2018). Effects of ball milling on the physicochemical and sorptive properties of biochar: experimental observations and governing mechanisms. Environ. Pollut. 233, 54–63. doi: 10.1016/j.envpol.2017.10.037
Manso, E. F., Nartey, E., Adjadeh, T., Darko, D., Lawson, I., and Amoatey, C. (2019). Use of corn cob and rice husk biochar as liming materials in acid soils. West Afr. J. Appl. Ecol. 27, 32–50. Available online at: https://www.ajol.info/index.php/wajae/article/view/192376
Namgay, T., Singh, B., and Singh, B. P. (2010). Influence of biochar application to soil on the availability of As, Cd, Cu, Pb, and Zn to maize (Zea mays L.). Soil Res. 48, 638–647. doi: 10.1071/SR10049
Pariyar, P., Kumari, K., Jain, M. K., and Jadhao, P. S. (2020). Evaluation of change in biochar properties derived from different feedstock and pyrolysis temperature for environmental and agricultural application. Sci. Total Environ. 713, 136433. doi: 10.1016/j.scitotenv.2019.136433
Phillips, C. L., Light, S. E., Lindsley, A., Wanzek, T. A., Meyer, K. M., and Trippe, K. M. (2020). Preliminary evaluation of a decision support tool for biochar amendment. Biochar 2, 93–105. doi: 10.1007/s42773-020-00037-3
Puga, A., Abreu, C., Melo, L., and Beesley, L. (2015). Biochar application to a contaminated soil reduces the availability and plant uptake of zinc, lead and cadmium. J. Environ. Manag. 159, 86–93. doi: 10.1016/j.jenvman.2015.05.036
Robin, A., Vansuyt, G., Hinsinger, P., Meyer, J. M., Briat, J.-F., et al. (2008). Iron dynamics in the rhizosphere: consequences for plant health and nutrition. Adv. Agron. 99, 183–225. doi: 10.1016/S0065-2113(08)00404-5
Rodríguez-Vila, A., Atuah, L., Abubakari, A. H., Worlanyo, A. D., Alhassan, A.-K., Coole, S., et al. (2022). Data for: Effect of biochar on micronutrient availability and uptake into leafy greens in two urban tropical soils with contrasting soil pH. Front. Sustain. Food Syst. doi: 10.17632/pkys2f8288.2
Rodríguez-Vila, A., Selwyn-Smith, H., Enunwa, L., Smail, I., Covelo, E. F., and Sizmur, T. (2018). Predicting Cu and Zn sorption capacity of biochar from feedstock C/N ratio and pyrolysis temperature. Environ. Sci. Pollut. Res. 25, 7730–7739. doi: 10.1007/s11356-017-1047-2
Schimmelpfennig, S., Kammann, C., Moser, G., Grünhage, L., and Müller, C. (2015). Changes in macro- and micronutrient contents of grasses and forbs following Miscanthus x giganteus feedstock, hydrochar and biochar application to temperate grassland. Grass Forage Sci. 70, 582–599. doi: 10.1111/gfs.12158
Schmidt, H. P., Bucheli, T., Kammann, C., Glaser, B., Abiven, S., and Leifeld, J. (2016). European Biochar Certificate-Guidelines for a Sustainable Production of Biochar. Arbaz: European Biochar Foundation (EBC).
Schmidt, H. P., Kammann, C., Hagemann, N., Leifeld, J., Bucheli, T. D., Sánchez Monedero, M. A., et al. (2021). Biochar in agriculture–a systematic review of 26 global meta-analyses. GCB Bioenergy 13, 1708–1730. doi: 10.1111/gcbb.12889
Sizmur, T., Palumbo-Roe, B., and Hodson, M. E. (2011). Impact of earthworms on trace element solubility in contaminated mine soils amended with green waste compost. Environ. Pollut. 159, 1852–1860. doi: 10.1016/j.envpol.2011.03.024
Sizmur, T., Quilliam, R., Puga, A. P., Moreno-Jiménez, E., Beesley, L., and Gomez-Eyles, J. L. (2016). Application of biochar for soil remediation. Agric. Environ. Appl. Biochar Adv. Barriers 63, 295–324. doi: 10.2136/sssaspecpub63.2014.0046.5
Steiner, C., Bellwood-Howard, I., Häring, V., Tonkudor, K., Addai, F., Atiah, K., et al. (2018). Participatory trials of on-farm biochar production and use in Tamale, Ghana. Agron. Sustain. Dev. 38, 1–10. doi: 10.1007/s13593-017-0486-y
Tan, X., Liu, Y., Zeng, G., Wang, X., Hu, X., Gu, Y., et al. (2015). Application of biochar for the removal of pollutants from aqueous solutions. Chemosphere 125, 70–85. doi: 10.1016/j.chemosphere.2014.12.058
Tittonell, P., and Giller, K. E. (2013). When yield gaps are poverty traps: the paradigm of ecological intensification in African smallholder agriculture. Field Crops Res. 143, 76–90. doi: 10.1016/j.fcr.2012.10.007
Tomczyk, A., Sokołowska, Z., and Boguta, P. (2020). Biochar physicochemical properties: pyrolysis temperature and feedstock kind effects. Rev. Environ. Sci. Biotechnol. 19, 191–215. doi: 10.1007/s11157-020-09523-3
Van Ittersum, M. K., Van Bussel, L. G., Wolf, J., Grassini, P., Van Wart, J., Guilpart, N., et al. (2016). Can sub-Saharan Africa feed itself? Proc. Natl. Acad. Sci. 113, 14964–14969. doi: 10.1073/pnas.1610359113
Wang, W., Bai, J., Lu, Q., Zhang, G., Wang, D., Jia, J., et al. (2021). Pyrolysis temperature and feedstock alter the functional groups and carbon sequestration potential of Phragmites australis-and Spartina alterniflora-derived biochars. GCB Bioenergy 13, 493–506. doi: 10.1111/gcbb.12795
Xu, R.-k., Zhao, A.-z., Yuan, J.-h., and Jiang, J. (2012). pH buffering capacity of acid soils from tropical and subtropical regions of China as influenced by incorporation of crop straw biochars. J. Soils Sediments 12, 494–502. doi: 10.1007/s11368-012-0483-3
Keywords: micronutrients, urban farming, crops, soil fertility, food security, Amaranthus, Corchorus, Lettuce
Citation: Rodríguez-Vila A, Atuah L, Abubakari AH, Atorqui DW, Abdul-Karim A, Coole S, Hammond J, Robinson S and Sizmur T (2022) Effect of Biochar on Micronutrient Availability and Uptake Into Leafy Greens in Two Urban Tropical Soils With Contrasting Soil pH. Front. Sustain. Food Syst. 6:821397. doi: 10.3389/fsufs.2022.821397
Received: 24 November 2021; Accepted: 31 March 2022;
Published: 10 May 2022.
Edited by:
Teresa Fresno, Autonomous University of Madrid, SpainReviewed by:
Aline Puga, Embrapa Meio Ambiente, BrazilAritz Burges, Groupe Institut supérieur d'agriculture de Lille, France
Copyright © 2022 Rodríguez-Vila, Atuah, Abubakari, Atorqui, Abdul-Karim, Coole, Hammond, Robinson and Sizmur. This is an open-access article distributed under the terms of the Creative Commons Attribution License (CC BY). The use, distribution or reproduction in other forums is permitted, provided the original author(s) and the copyright owner(s) are credited and that the original publication in this journal is cited, in accordance with accepted academic practice. No use, distribution or reproduction is permitted which does not comply with these terms.
*Correspondence: Tom Sizmur, dC5zaXptdXImI3gwMDA0MDtyZWFkaW5nLmFjLnVr