- 1Department of Biosciences, University of Wah, Rawalpindi, Pakistan
- 2Department of Biology, The Peace Group of Schools and Colleges, Charsadda, KP, Pakistan
The consistent use of synthetic fertilizers and chemicals in traditional agriculture has not only compromised the fragile agroecosystems but has also adversely affected human, aquatic, and terrestrial life. The use of phytostimulants is an alternative eco-friendly approach that eliminates ecosystem disruption while maintaining agricultural productivity. Phytostimulants include living entities and materials, such as microorganisms and nanomaterials, which when applied to plants or to the rhizosphere, stimulate plant growth and induce tolerance to plants against biotic and abiotic stresses. In this review, we focus on plant growth-promoting rhizobacteria (PGPR), beneficial fungi, such as arbuscular mycorrhizal fungi (AMF) and plant growth-promoting fungi (PGPF), actinomycetes, cyanobacteria, azolla, and lichens, and their potential benefits in the crop improvement, and mitigation of abiotic and biotic stresses either alone or in combination. PGPR, AMF, and PGPF are plant beneficial microbes that can release phytohormones, such as indole acetic acid (IAA), gibberellic acid (GA), and cytokinins, promoting plant growth and improving soil health, and in addition, they also produce many secondary metabolites, antibiotics, and antioxidant compounds and help to combat biotic and abiotic stresses. Their ability to act as phytostimulator and a supplement of inorganic fertilizers is considered promising in practicing sustainable agriculture and organic farming. Glomalin is a proteinaceous product, produced by AMF, involved in soil aggregation and elevation of soil water holding capacity under stressed and unstressed conditions. The negative effects of continuous cropping can be mitigated by AMF biofertilization. The synergistic effects of PGPR and PGPF may be more effective. The mechanisms of control exercised by PGPF either direct or indirect to suppress plant diseases viz. by competing for space and nutrients, mycoparasitism, antibiosis, mycovirus-mediated cross-protection, and induced systemic resistance (ISR) have been discussed. The emerging role of cyanobacterial metabolites and the implication of nanofertilizers have been highlighted in sustainable agriculture.
Introduction
The term “phytostimulant” was aptly defined by Du Jardin (2012) stating “substances and materials, with the exception of nutrients and pesticides, when applied to plants, seeds or growing substrates in specific formulations, have the capacity to modify physiological processes of plants in a way that provides potential benefits to growth, development, and/or stress response.” Phytostimulants play a substantial role in agro-environmental sustainability as their use in crop production model seeks to protect the environment by reducing detrimental amounts of chemical inputs to crop fields, while maintaining crop yield and quality.
Plant growth-promoting rhizobacteria are plant beneficial bacteria that produce metabolites to protect plants from getting infected and also assist in the healthier growth of plant against environmental stresses. Induced systematic resistance, antibiosis, and production of bioactive metabolites are the indirect action of PGPR (Meena et al., 2020). PGPF and AMF can coexist with other microorganisms, present in the rhizosphere soil, and can form a beneficial relationship with the plant roots, improve plant growth, and provide assistance to plants to adapt adverse environmental conditions, such as drought, salinity, heavy metal toxicity, hydrocarbon toxicity, and diseases (El-Maraghy et al., 2021; Murali et al., 2021). Cyanobacteria are a major component of biogeochemical cycles, as they are exploited for biofertilizers and produce plant growth-promoting hormones and bioactive compounds (Gupta et al., 2021). Lichen is a stable symbiotic association between algae and fungi and contains a massive reservoir of secondary metabolites (Calcott et al., 2018; Kanivebagilu and Mesta, 2020). In addition, it is a good indicator of atmospheric pollution. Azolla is a fern and serves as a valuable nitrogen (N) source, particularly in rice field, and exhibits high bioremediation potential for Cd, Cr, Cu, and Zn (Yang et al., 2021). It can work alone and more so in synergism with cyanobacteria.
Fungal phytostimulants
Fungi are considered integral components of the soil, existing in different forms and shapes (Yan et al., 2019). Some fungal species are pathogenic and are catastrophic to plant health, while some benefit the plants in numerous ways. Beneficial fungi act as a phytostimulant and play an essential role in the functioning of ecosystem as they progressively intervene various soil nutrient cycles and enhance water retention properties, making a positive impact not only on plant performance but also enhance abiotic and biotic stress tolerance in plants (Begum et al., 2019).
Classification of beneficial fungi
Beneficial fungi can be broadly classified into two main categories: plant growth-promoting fungi (PGPF) and mycorrhizal fungi (MF). MF establishes an intimate association with the roots of majority of land plants through the network of hyphae (arbuscules) and in turn consumes plant products like lipids and carbon substrates to accomplish their life cycle (Jiang et al., 2017). Based on the hyphal arrangement of MF within cortical tissues of plants, MF can be classified into three main types: (1) Endomycorrhiza forms intracellular arbuscules, for example, arbuscular mycorrhizal fungi (AMF), (2) ectomycorrhiza develops intercellular network of hyphae, and (3) ectendomycorrhiza is a combination of both intracellular and intercellular network of arbuscules (He et al., 2020; Tedersoo et al., 2020).
On the contrary, PGPF is a heterogeneous group of nonpathogenic fungi that form a non-symbiotic, non-obligate mutualism with a broader range of host plants. PGPF can include species of the genera Aspergillus, Fusarium, Trichoderma, Penicillium, Piriformospora, Phoma, and Rhizoctonia. Based on the type of plant tissues they inhabit, PGPF can either be (1) endophytic {found inside both the aerial (stems and leaves) and belowground tissues of plants [seeds and roots (Guerreiro et al., 2018)]}, (2) epiphytic [found on the phyllosphere (aerial parts-leaves) of plants and survive by consuming carbohydrates and amino acids exuded by the leaves (Bacon and White, 2016)], and (3) free-living PGPF/rhizosphere fungi [found in close proximity of plant roots (Busby et al., 2017)]. Francioli et al. (2021) found plant functional groups as the main factor driving root-associated fungal saprophytic community richness. The details are given in Figure 1.
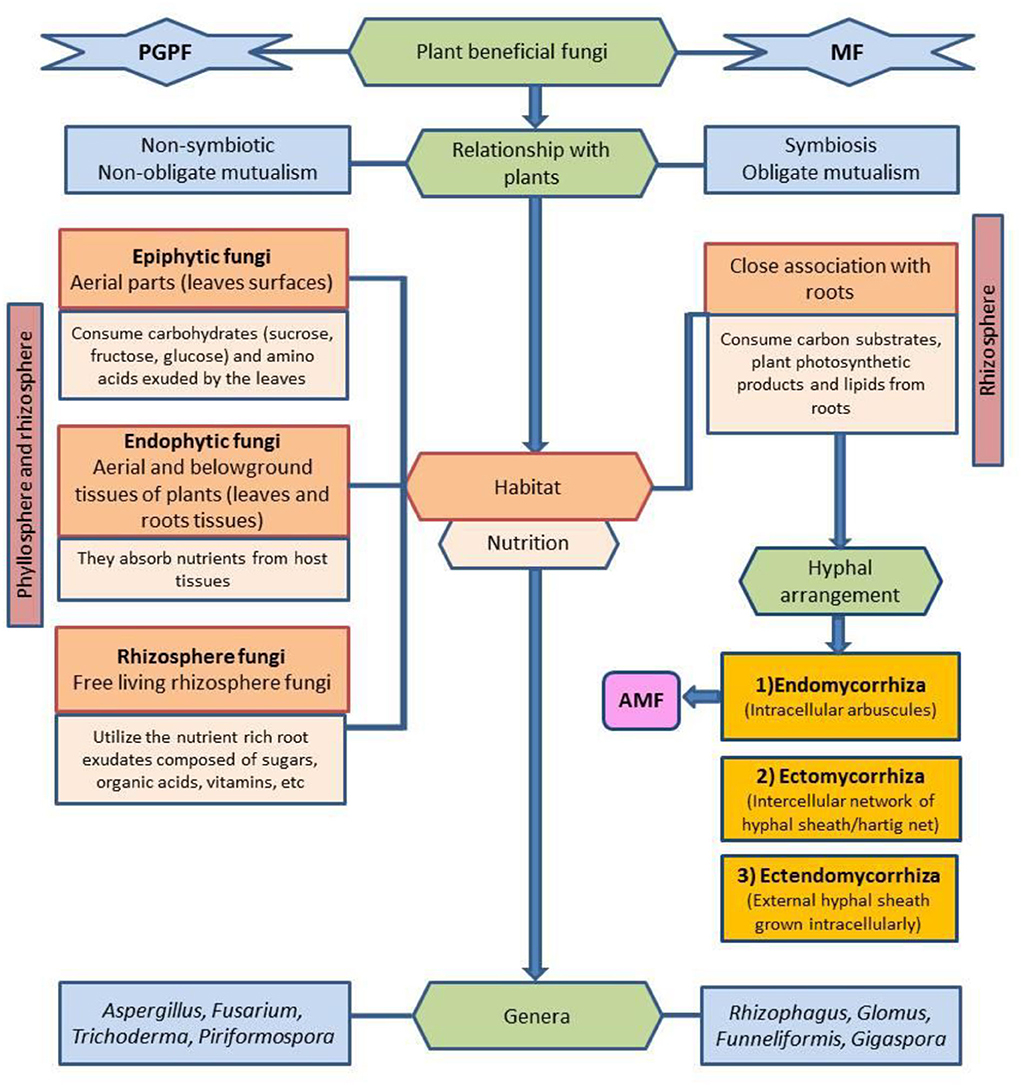
Figure 1. Schematic illustration of classification of beneficial fungi on the basis of their relationship with plants, habitat, nutrition, and hyphal arrangement. PGPF, plant growth-promoting fungi; MF, mycorrhizal fungi; AMF, arbuscular mycorrhizal fungi.
Arbuscular mycorrhizal fungi
AMF are obligate biotrophs and form an excellent symbiotic relationship with the plant roots by penetrating the cell walls and cell membranes via extraradical and intraradical hyphae, thus radically improving the nutrient transport system of plants (Bowles et al., 2016; Jiang et al., 2017). Moreover, AMF improve the soil texture, nutrient cycling, and nutrient uptake (N, P, and K) of plants and also improve plant tolerance to biotic/abiotic stresses (Oliveira et al., 2017a,b). Glomalin is a proteinaceous product, produced by AMF, involved in soil aggregation and elevation of soil water holding capacity under stressed and unstressed conditions (Sharma et al., 2017).
Positive impact of beneficial fungi on plant growth responses
AMF affect growth-related functions in plants, such as stomatal conductance and leaf gas exchange, leaf relative water content (LRWC), photosynthetic capacity (photosystem II efficiency), plant biomass, and CO2 assimilation (He et al., 2017; Chandrasekaran et al., 2019). AMF not only improve plant yield but also play a significant role in increasing bioactive components of essential oils in medicinal plants and their nutritional status as indicated by Khalediyan et al. (2021). Continuous cropping can cause scarcity of nutrients and an imbalance in the microbiome of the rhizosphere resulting in reduced yield and poor plant quality. A study by Liu et al. (2020) reported that the negative effects of continuous cropping of American ginseng, such as decreased seedling emergence rate, shoot and root dry weight, soil pH, ammonium contents, and available P and K contents in the rhizosphere, were relieved by the use of endomycorrhizal fungi biofertilizer (Rhizoglomus irregulare, Funneliformis mosseae, and Funneliformis caledonium). Moreover, it increased the relative abundances of Acidobacteria and Ascomycota in the rhizosphere microbiota. A recent report by Xu et al. (2021) indicated that fungal epiphytes (PGPF) namely Fusarium fujikuroi and Fusarium oxysporum produced elevated levels of endogenous IAA, resulting in increased aboveground and belowground biomass of Ipomoea cairica cuttings.
Plant growth responses to AMF when in combination with PGPR
Plant growth-promoting rhizobacteria are plant beneficial bacteria that can improve plant growth through mineral solubilization and mobilization, nitrogen fixation, production of phytohormone and siderophore, and increased soil water holding capacity (Santoyo et al., 2021). PGPR-induced beneficial changes in the root morphology include increased root surface area and absorption of nutrients mainly solubilized by plant microbiome, including PGPR (Grover et al., 2021). Pseudomonas fluorescens and Bacillus subtilis are examples of mycorrhiza helper bacteria that facilitate AMF symbiosis (Wilkes et al., 2021; Yadav et al., 2021). The magnitude of stimulation offered by AMF is significantly augmented when combined with PGPR, such as plant nutrient availability and nutrient use efficiency and yield (Kamali and Mehraban, 2020). Table 1 shows responses of plant species treated with AMF and PGPR. Research has also been conducted on assessing the beneficial effects of using more than two biofertilizers. One such study by El Amerany et al. (2020) reported that the application of AMF (Glomus sp. Sclerocystis sp. Acaulospora sp.), chitosan, and compost increased chlorophyll fluorescence, sugar, and protein content of tomato plants, as well as improved root and shoot growth and stem tissues. Chitosan and compost when applied together could be a continuous source of nutrition (Xu and Mou, 2018).
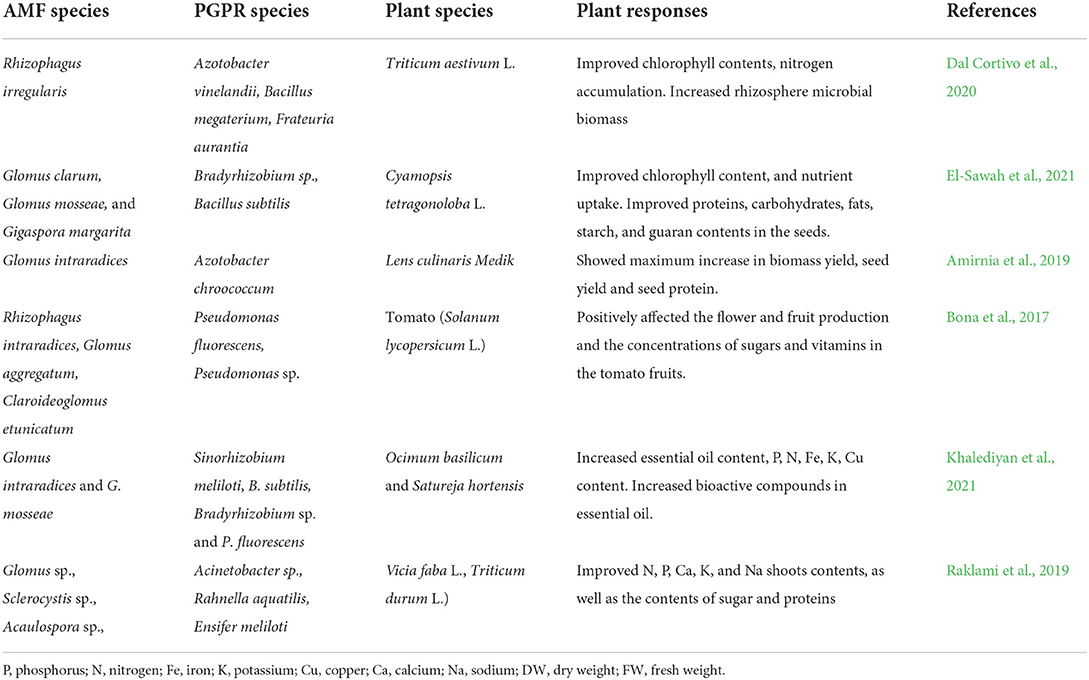
Table 1. Plant responses to the combined application of arbuscular mycorrhizal fungi (AMF) and plant growth-promoting fungi (PGPR).
Plant–microbe interactions involve complex mechanisms and the extent to which a host plant benefits depend on numerous variables, including environmental conditions, soil microbiota, host plant species, and the species of microbes inoculated as biofertilizers. Some combinations of inoculants performed better than others (Desai et al., 2016).
Biotic stress management using fungi
Plant disease
Beneficial fungi employ various biocontrol mechanisms, either direct or indirect, to suppress plant diseases. These mechanisms include outpacing the pathogens by competing for space and nutrients, mycoparasitism, antibiosis, mycovirus-mediated cross-protection, and induced systemic resistance (ISR) (Ghorbanpour et al., 2018). Liu et al. (2020) reported less incidence of root disease after the use of AMF biofertilizer (Rhizoglomus irregulare, Funneliformis mosseae, and Funneliformis caledonium). Furthermore, the treatment was able to recruit beneficial soil microbes in soil subjected to continuous cropping that suppressed pathogenic microbes like Fusarium oxysporum, Fusarium solani, and the harmful bacteria Candidatus solibacter. Yeast is a promising biocontrol agent that can colonize pathogenic fungal hyphae and retard its growth, while producing vitamin B12 that promotes mycorrhizal growth in the rhizosphere (Mukherjee et al., 2020). AMF can be combined with non-filamentous fungi, such as yeast to combat fungal-borne diseases. One such study was reported by Nafady et al. (2019) in which co-inoculation of sunflower plants with yeast (Brettanomyces naardensis) and AMF not only reduced the root rot and charcoal rot disease incidence by 11 and 5%, caused by Macrophomina phaseolina, but also improved plant growth parameters (plant height, dry weight, and leaf number) in both healthy and infected plants. A decrease in malondialdehyde (MDA) was reflective of induced immune system that translated into mitigation of disease symptoms. Similarly, enhancement of plant growth was indicative of enhanced plant nutrient availability. Another study reported by Sarabia et al. (2018) concluded that rhizosphere yeasts (Cryptococcus flavus and Candida railenensis) improve P uptake of maize via hyphae of AMF (Rhizophagus irregularis) and improved root length. The ability of PGPR to synthesize pathogen-antagonizing compounds and triggering induced systemic resistance (ISR) assist AMF in improving plant disease resistance (Jiao et al., 2021). Co-inoculation of AMF (Rhizobium leguminosarum and Glomus spp) and PGPR (Bacillus subtilis and Pseudomonas fluorescens) also proved to be an effective biocontrol against Sclerotium rolfsii (White Rot fungi) as significant increase in nutrient uptake (P and Fe) and plant defense enzymes, for example, chitinase, peroxidase, and polyphenol oxidase were observed in common beans. Stress mitigation was evident through increased straw and green pod yields of plants infected by Sclerotium rolfsii (Mohamed et al., 2019). Naziya et al. (2019) reported that PGPF (Talaromyces sp. NBP-61, Penicillium sp. NBP-45, and Trichoderma sp. NBP-67) offered protection in chili (Capsicum annuum L.) plants against anthracnose disease caused by Colletotrichum capsici, as evidenced by the accumulation of lignin and callose (indicative of induced ISR) and enhanced antioxidant enzyme activities in PGPF-treated plants.
Abiotic stress management using fungi
Drought and salinity
Various mechanisms have been proposed by which AMF reduce the negative effects of drought and salinity stress. AMF maintain ionic homeostasis and offer osmoprotection. It facilitates expedites nutrient uptake and augments photosynthetic efficiency, water use efficiency, stomatal conductance, and leaf gas exchange. In addition, production of metabolites (proline content and alpha-tocopherol content) and antioxidant enzymes (SOD, CAT, and POX) by plant assists in the amelioration of the negative effects of drought and salinity stress (Evelin et al., 2019). Malondialdehyde (MDA) is an indicator of plant membrane damage caused by lipid peroxidation and can be reduced by AMF (Li et al., 2019). It was reported by Zhang et al. (2020) that AMF (Rhizophagus intraradices) ameliorated the drought and salinity stress of castor bean plants as evidenced by increment in protein and proline contents and decrease in MDA content. Photosynthesis, stomatal conductance, and transpiration rate of castor bean were also increased concomitant with the decrease in the intercellular CO2 concentration. Zhang et al. (2019) monitored endogenous hormonal changes in mycorrhizal inoculated (Funneliformis mosseae) trifoliate orange under drought stress and found significant increase in root abscisic acid (ABA), indole-3-acetic acid (IAA), methyl jasmonate, and brassinosteroids concentrations. These AMF-treated plants under drought stress exhibited significantly higher root-hair density, length, and diameter in taproot and lateral roots. Klinsukon et al. (2021) reported colonization by AMF (Glomus sp., Gigaspora albida, and Gigaspora decipiens) improved salinity tolerance of eucalyptus plants by increasing photosynthetic pigments and K/Na ratio, while reducing proline accumulation. Another study by Li et al. (2020) reported alleviation of moderate salinity stress (100 and 150 mM) in Euonymus maackii (an important ecological restoration tree) by AMF (Rhizophagus intraradices) as improved photosynthesis, plant nutrient absorption, and antioxidant enzyme activities were observed. Studies have reported drought and salinity stress mitigation via the use of dual combination of AMF and PGPR. Maximum increase in biomass, ion, and nutrient content in maize was exhibited by plants under salinity stress, treated with combination of AMF (Rhizoglomus irregulare) and PGPR (Pseudomonas reactans and Pantoea alli) (Moreira et al., 2021). In another study by Kamali and Mehraban (2020), Azotobacter and Azospirillum (PGPR) in combination with Glomus mosseae (AMF) resulted in significant amelioration of drought stress in sorghum as indicated by enhanced antioxidant enzymes (CAT, POD, and APX), total carotenoid, anthocyanin, and flavonoid contents. Improved auxin (IAA) content, root colonization, grain yield (27%), and grain protein yield (19%) were also reported. The performance of Casuarina obesa under saline stress conditions was improved by PGPR (Pantoea agglomerans and Bacillus sp.) and AMF (Rhizophagus fasciculatus and Rhizophagus aggregatum) as high survival rate, accumulation of proline, improved photosynthetic contents, and plant biomass were observed (Diagne et al., 2020). Halophytes are salt-tolerant plants and naturally survive in saline soils. In a study reported by Pan et al. (2020), a typical halophyte (Elaeagnus angustifolia L.) was found more responsive to AMF (Glomus mosseae) over single treatment of PGPR (Bacillus amyloliquefaciens) or combined treatment of both PGPR and AMF. Glomus mosseae improved soil enzymes and root surface area, in addition to biomass accumulation in aboveground parts that favored light absorption and maximized photosynthetic capacity and gas exchanges of Elaeagnus angustifolia L. seedlings in saline-sodic soil. Combined AMF (Funneliformis mosseae and Rhizophagus irregularis) and PGPR (Pseudomonas fluorescens and P. putida) inoculation improved drought resistance of Myrtus communis as indicated by improved essential oil yield (non-enzymatic antioxidant), survival rate, leaf physiology, reduced electrolyte leakage, MDA, and proline concentrations (Azizi et al., 2022).
Heavy metal contamination
Exposure to heavy metals could result in disturbed plant metabolic activities which negatively influence plant growth and physiology (Sharma et al., 2017). Agricultural crops such as rice and maize act as hyper-accumulators of chromium and copper, respectively, posing highest cancer risk in people consuming these grains (Sharma P. et al., 2021). Oxidative stress induced by heavy metal accumulation triggers defense mechanisms in plants. Non-enzymatic antioxidants like proline, carotenoids, glutathione, and ascorbic acid and enzymatic antioxidants, such as SOD, CAT, glutathione peroxidase, and guaiacol peroxidase (GPX), scavenge reactive oxygen species and assist the plant in tolerating oxidative stress (Dhir, 2021). Fungi especially AMF elevate the production of these phytohormones under heavy metal contaminated soils, produce siderophores and ACC deaminase, regulate root morphology, and improve plant nutrient absorption. Rhizoglomus intraradices is reported to mitigate arsenic toxicity by participating in the antioxidant defense system and thiol metabolism of wheat (Sharma et al., 2017). Zhang et al. (2020) also reported beneficial effects (root morphology, plant biomass, and growth hormone regulation) of Rhizoglomus intraradices on plants (black locust) grown in arsenic-contaminated soil. Rostami et al. (2021) reported that the dual inoculation of PGPR (Pseudomonas putida) and AMF (Funneliformis mosseae and Rhizophagus intraradices) ameliorated cadmium stress in corn through improved plant nutrient status, biomass, and photosynthetic capacity. As AMF maintain strong affiliation with host roots, it reduces the mobility of heavy metals in plants, a term known as phytostabilization, and increases efficiency of metal degradation. Ikram et al. (2018) treated wheat plants grown on heavy metal contaminated soils (Ni, Cd, Cu, Zn, and Pb) with IAA-producing fungal endophyte Penicillium roqueforti Thom. and reported restricted transfer of heavy metals from soil to the plants due to IAA-producing ability of P. ruqueforti. Furthermore, in comparison with control, plants grown under heavy metal concentrations had higher plant growth, nutrient uptake, and low concentrations of heavy metals in shoot and roots. Another study showed that AMF Funneliformis mosseae reduced effects of lead (Pb) toxicity in R. pseudoacacia seedlings, by improving the plant biomass, root activity, antioxidant enzymes, and photosynthetic activities (Huang et al., 2019). A flowchart (Figure 2) highlights the role of PGPF in sustainable agriculture.
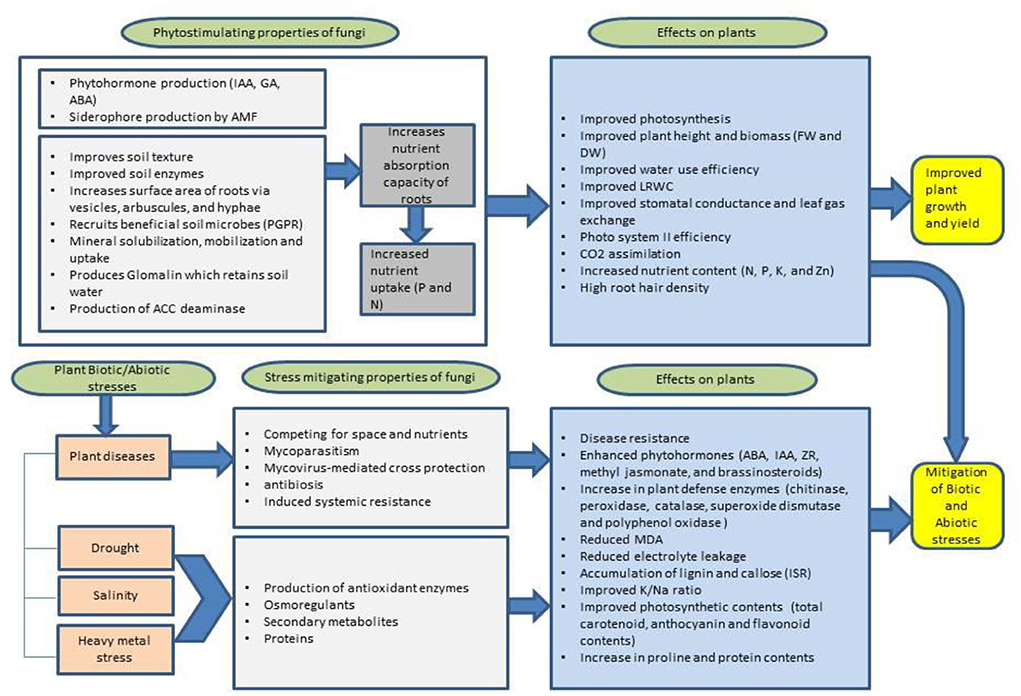
Figure 2. Schematic illustration of phytostimulation and stress mitigating properties of fungi and their effects on plant responses. IAA, indole acetic acid; GA, gibberellic acid; ABA, abscisic acid; ZR, zeatin riboside; PGPR, plant growth-promoting rhizobacteria; AMF, arbuscular mycorrhizal fungi; ACC, amino cyclopropane carboxylate; FW, fresh weight; DW, dry weight; LRWC, leaf relative water content; MDA, malondialdehyde; ISR, induced systemic resistance; N, nitrogen; P, phosphorus; K, potassium; Zn, zinc.
PGPR phytostimulants in sustainable agriculture
Plant growth-promoting rhizobacteria comprise a group of bacteria found in rhizosphere of plants and also form association with plants (Meena et al., 2020). They promote plant growth through direct or indirect mechanism. Direct mechanism includes phytohormone production and solubilization of soil nutrients through acid secretion to make them available to plants root system (Kumar and Meena, 2019). Indirectly, they suppress plant pathogens by providing defense to plants through secretion of secondary metabolites or induce plant immunity through activation of induced systemic resistance or systemic acquired resistance pathways.
Notable genera of bacteria acting as PGPR include Pseudomonas, Enterobacter, Bacillus, Variovorax, Klebsiella, Burkholderia, Azospirillum, Serratia, Planomicrobium, and Azotobacter.
PGPR biofertilizer as phytostimulants
Biofertilizer, defined as formulation containing live or latent cell of efficient strains of bacteria (PGPR) mixed with carrier material, can be applied to seeds, plant surfaces, or soil, colonize the rhizosphere or the interior of the plant, and promote growth by increasing the availability of primary nutrients to the host plant (Mohammadi and Sohrabi, 2012). PGPR significantly increased soil microbial biomass, total nitrogen, available phosphorus, and soil organic matter contents in the form of biofertilizers (Ju et al., 2019). The role of PGPR biofertilizer is summarized in Table 2.
PGPR produces secondary metabolites
Phytohormones
Plant growth regulators (auxins, gibberellins, and cytokinins) are the organic compounds produced in extremely low concentration but greatly influence the biochemical, morphological, and physiological metabolic activities of plants. PGPR produce indole acetic acid (Sharma S. et al., 2021), gibberellin (Baghel et al., 2019), and abscisic acid (Kour et al., 2020). The signaling between rhizobia and legumes is a model for hormone-type signaling between PGPR and plants. Plants respond to compounds which are produced by PGPR through systemic acquired resistance (SAR) in which a cascade of defense mechanisms are involved (Kamle et al., 2020). For instance, SAR involves signal transduction, generation of phytoalexins, protection from oxidative stress, and lignification (Kallamadi et al., 2022; Yu et al., 2022). In all of these defense mechanisms, salicylic acid (SA), jasmonic acid (JA), and ethylene play vital roles. These hormones may also impart in plant growth stimulation.
Rhizobacteria activate salicylic acid (SA)-dependent pathways and defense-related genes (pathogenesis-related proteins) in inoculated plants against phytopathogens (Huang et al., 2021). Pseudomonas aeruginosa has the ability to induce systematic resistance via SA-dependent pathway against Rhizoctonia solani and M. phaseolina (Tewari and Arora, 2018; Rahman et al., 2022).
PGPR produce signaling molecules
The bioactive compounds secreted by phytostimulants activate signaling pathways in plants (Yakhin et al., 2017). Peptide signaling is important in various aspects of plant development, growth, and defense responses to biotic and abiotic stresses (Vaahtera et al., 2019). Specific signaling peptides affect defense response, callus growth, meristem organization, root growth, leaf-shape, and nodule development (Cataldo et al., 2022). Flavonoids, isoflavonoids, and phenolic signaling molecules excreted by the plant root induce expression of rhizobial nod genes. In response to these compounds, rhizobia produce a series of host-specific signaling molecules, for example, LCOs also referred to as nod factors (NFs). For instance, genistein, a phenolic compound along with other potential signal compounds secreted include daidzein, O-acetyldaidzein, 6′-O-malonylgenistin, 6′-O-malonyldiadzin, glycitin, and 6′-O-malonylglycin secreted by soybean and other plants, activates nod gene expression in B. japonicum (Gray and Smith, 2005). The nod genes common to all rhizobia include nods A, B, and C which are responsible for the biosynthesis of the basic LCO. The structure-specific nod genes, required for the “decorative” features found on the chitin backbone of LCOs, play a role in regulating the specificity of the symbiosis; these species-specific features of LCOs contribute to host specificity of bacterium-to-plant signaling and response mechanisms (Persson et al., 2015). Hence in future, plant-to-bacteria signals will be shown to enhance the production of growth stimulating materials produced by non-rhizobial PGPR.
Antimicrobial substances
Plant growth-promoting rhizobacteria produce a wide range of antimicrobial substances, for example, broad-spectrum non-ribosomal antibiotics, organic acids, lysozymes, and bacteriocins (Nazari and Smith, 2020). Bacteriocins are ribosomally synthesized cationic peptides which inhibit the growth of target organisms by binding the phospholipid membrane of target strain. For instance, bacteriocin produced by P. fluorescens SF39a (isolated from wheat rhizosphere) inhibited the growth of phytopathogenic Pseudomonas and Xanthomonas strains (Godino et al., 2016).
Until now, 18 different bacteriocins extracted and purified from B. thuringiensis have been reported. These include thuricin, thuricin7, entomocin110, morricin269, and tochicin (Cherif et al., 2008; Nazari and Smith, 2020). These compounds promote plant growth via activation of antioxidant cascade, modulating protein activities, synthesis of enzymes related to plant defense systems, increase in photosynthetic rate, stimulate the production of IAA, SA, and ABA, and modify the root system in host plants for better uptake of water and nutrients from soil (Subramanian and Smith, 2015; Nazari and Smith, 2020).
Antibiotics
Rhizospheric PGPR are excellent biocontrol agents suppressing soilborne diseases in host plants caused by fungi, bacteria, viruses, and nematodes. Nowadays, PGPR are used in integrated pest management. In association with planta, they secrete antibiotics which are oligopeptides of varying specificity and mode of action. Antibiotics inhibit the protein synthesis in pathogen cell as it retards the formation of initiation complex on small ribosome subunit, thus inhibiting the growth of pathogenic bacteria (Watcharin, 2015). Antibiotics also act as inhibitory factor to some important enzymes like aldose reductase which isomerase the glucose to fructose (Barbier et al., 2017).
Antibiotics of PGPR origin are phloroglucinols, D-gluconic acid, 2-hydroxymethyl-chroman-4-one, oomycin A, phenazine, pyoluteorin, pyrrolnitrin, tensin, tropolone, cyclic lipopeptide oligomycin A, kanosamine, zwittermicin A, and xanthobaccin (Maheshwari et al., 2013). Various Pseudomonas spp. produces 2,4-diacetylphloroglucinols that arrested the growth of R. solanacearum and fungal pathogens, such as Rhizoctonia solani, Sclerotium rolfsii, Macrophomina phaseolina, and Fusarium oxysporum, compared to control (Suresh et al., 2021).
PGPR as phytoremediator of heavy metals and trace metals
PGPR assist in phytoremediation of heavy metals in various ways, that is, (i) chelate heavy metals via secretion of siderophore (Patel et al., 2018), (ii) lower ethylene levels of plants to combat heavy metal stress through secretion of ACC deaminase (Ghosh et al., 2018), produce exopolysaccharides and osmoprotectants (Kumar and Verma, 2018). PGPR produce rhamnolipid biosurfactants which remediate soil from heavy metals (Chellaiah, 2018). Cadmium-contaminated soil has inhibitory effect on plant growth due to the formation of ethylene, whereas ACC deaminase exhibiting P. putida alleviated the production of ethylene and reduced the inhibitory effects of heavy metal stress on plant (Hassan et al., 2017; Pramanik et al., 2018).
Siderophore production
Siderophores are low molecular weight proteins produced by PGPR to chelate the insoluble form of iron (Fe+3) into soluble form (Fe+2) and make it available to the host plant (Figure 3). Siderophore can be of different types, for example, hydroxamate siderophores, catecholate siderophores, and mixed ligands. Siderophore binds with iron tightly, reduces the availability of iron to phytopathogens, and inhibits their growth (Rajkumar et al., 2010). Inoculation of P. fluorescens and S. acidiscabies to cowpea in nickel-contaminated soil exhibited dual role.
Induced systematic resistance
In plants, Induced systematic resistance (ISR) is triggered upon colonization with biological agent like herbivore / other pathogens attack or chemical inducers (Pieterse et al., 2014). Plant defense system has the ability to recognize specialized microbial compounds (i.e., flagellin and fungal chitin) commonly called microbe-associated molecular patterns (MAMPs) or pathogen-associated molecular patterns (PAMPs) through specialized pattern recognition receptors (PRRs) which activate PAMP-trigger immunity (PTI) (Pel and Pieterse, 2013). Pathogens have the ability to suppress the first line of defense, the PTI, or suppress pathogen-detection system of host. Subsequently, plants induce effector trigger immunity (ETI) and cause cell death at infected area to prevent further invasion of pathogen (Chuberre et al., 2018). After PTI and ETI, host cells trigger signals to uninfected parts to induce immunity in distal parts remote from site of infection, and this kind of immunity is termed as systemic acquired resistance (SAR) (Pusztahelyi, 2018; Teixeira et al., 2019).
Plants inoculated with PGPR have developed ISR against foliar and root-borne pathogens (Beneduzi et al., 2012) (Table 3). Induced resistance involves the activation of pathogen-related proteins parallel to salicylic acid synthesis, which induces defense response in plants. Many secondary metabolites secreted by PGPR phytostimulants are involved in providing defense to plants against pathogen attack. It was reported that tomato, inoculated with ACC deaminase secreting Paenibacillus lentimorbus B-30488, provided tolerance against southern blight disease caused by Sclerotium rolfsii, upon activation of antioxidant enzymes, and pathogen-related gene expression analysis (Backer et al., 2018). Cotton plants, inoculated with Bacillus spp., exhibited increased gossypol and jasmonic acid secretion reducing larval feeding by Spodoptera exigua (Zebelo et al., 2016). Enterobacter asburiae BQ9 induced resistance in tomato against yellow leaf curl virus by increasing the expression of defense-related genes and antioxidant enzymes, including phenylalanine ammonia lyase, peroxidase, catalase, and superoxide dismutase (Li et al., 2016).
Role of cyanobacteria in agricultural sustainability
Cyanobacteria are emerging candidates for efficient conversion of radiant energy into chemical energy. Their biomass can be used for large-scale production of food, biofertilizers, secondary metabolites, and medicines. This bacterium increases the atmospheric O2 level which provided a platform for biota to move from simple to more complex form. Their N-fixing ability enables them to maintain the N cycle in ecosystem. It became a potential candidate to be exploited on agriculture and industrial level due to lower production of CO2. In climate change scenario, this organism has the potential to release methane into atmosphere, which is 20 times more potent than CO2 (Bižić et al., 2020). An in-depth knowledge of research is needed to bioengineer some new physiological mechanism in this organism that could switch off the CH4-producing properties to make them 100 % efficient, effective, and resilient in sustaining future agriculture practices. A flowchart related to the role of cyanobacteria in soil, environment, and plant is summarized in Figure 4. Some important roles are discussed below.
Nitrogen fixation
Heterocyst is a specialized cell found in cyanobacteria; it contains cyanophycin which acts as storage house of nitrogen. The fixed nitrogen species can easily be assimilated by plants. It could be used to supplement chemical nitrogen fertilizers to reduce the cost of crop production by 25 to 40%. The formation of soil crust in cultivated cyanobacteria field can avoid the runoff and therefore reduces the risk of water contamination with nitrogen. Anabaena species has the ability to fix nitrogen even under nitrogen deprived environment (He et al., 2021). In modern agriculture practices, cyanobacteria could be used as biofertilizer as they provide 70–75% fixed N to agriculture system (Berthelot et al., 2015; Klawonn et al., 2016).
Nutrient availability and increased organic carbon level
Cyanobacterial biomass is reservoir of N, P, K, Mg, S, and Fe. These nutrients play an important role in plant metabolism. Cyanobacteria fix atmospheric CO2 and convert it into organic biomass (Pathak et al., 2017). After death and decay by other organisms and grazers, the biomass assimilates into soil resulting in increased organic carbon in soil. Noteworthy is the secretion of extracellular polymeric substances by cyanobacteria imparting enhanced carbon content of soil. They improve soil quality and make the macro- and micronutrients available resulting in enhanced yield and quality.
Soil physico-chemical properties
Several studies have shown that the application of cyanobacterial biomass to soil helped to maintain pH, removed heavy metals from affected soil, and improved water retention property of soil. Improvement in soil characteristics, such as soil aggregation, porosity, permeability, aeration, and humidity level, has been reported in soil inoculated with cyanobacteria (Costa et al., 2018; Abinandan et al., 2019).
Production of secondary metabolites
Phenolic compounds provide protection against pathogens due to their antimicrobial, fungicidal, and antioxidant properties. Terpenoid compounds play an important role during preliminary growth and development of plants, as well as on the attraction of pollinators (Lin and Pakrasi, 2019). Cyanobacteria provide protection to crops against bacteria, insects, and other organisms due to their antimicrobial, allelochemical, and antioxidant properties (Gonçalves, 2021). Free fatty acids (FFAs) derived from cyanobacteria are extensively explored for biodiesel production (Madusanka and Manage, 2018). Polysaccharides have a preponderant role in the improvement of soil quality, with major properties like soil conditioner, nutrient carrier, and a safe release of agrochemicals. Carotenoids have been applied in soil bioremediatory, as an antioxidant, fertilizers, and biopesticides with major impact on soil improvement and crop protection. They also increase the availability of provitamin A in biofortified crops (Gonçalves, 2021).
Apart from its importance, there are some constraints to the use of cyanobacteria, as they secrete some toxins like cylindrospermopsin, anatoxin, and saxitoxin in fresh water with harmful effects on humans, animals, and aquatic biota (Moreira et al., 2021). Therefore, some mechanical, that is, artificial mixing, hypolimnetic aeration, dredging, and sonication and biological (biomanipulation, macrophytes, and straws), methods should be employed to manage cyanobacterial bloom (Kibuye et al., 2021).
Azolla—The green manure for sustainable agriculture
Azolla is a macrophyte fern (pteridophyte) commonly known as duckweed fern or mosquito fern. The frond of this genus harbors in their dorsal leaf lobe ellipsoid cavities which act as nest of Anabaena (cyanobacterium) association referred to as Azolla-anabaena symbiosis. The nitrogen-fixing Anabaena allow Azolla to grow rapidly in nitrogen-free medium (Carrapiço, 2017). Eily et al. (2019) reported that glutamine synthetase (GS) and glutamate synthase (GOGAT) genes regulate the machinery used by Azolla-Nostoc symbiosis for nitrogen assimilation. Azolla is an ideal candidate in intercropping as it improves N use efficiency and quality of water (Yang et al., 2021). The content of N reported in standing crop treated with Azolla ranged from 2 to 4 Kg N/ha/day. It has been grown as intercrop cover in paddy field to reduce ammonia volatilization and improved nitrogen use efficiency of rice (Yang et al., 2021), and also used as top dressing over water surface to control weeds, mosquitoes, and improved water quality by removing nitrates and phosphorous from water.
Lichen in sustainable agriculture
A stable symbiotic association between photobiont (algae) and mycobiont (fungi) leads to evolution of lichen (Calcott et al., 2018). About 95% thalli of lichen is composed of fungi (ascomycota and sometime basidiomycota), and only 5% is comprised of cyanobacteria or green algae. Lichens produce metabolites which induce protection against damaging effect of light, herbicides, act as effective source of solubilizing macro- and micronutrient, and also act as indicator of the atmospheric pollutants (Abas, 2021). Lichen is a natural reservoir of 6,000–8,000 secondary metabolites, which include allelopathic compounds, such as depsides, depsidones, depones, and dibenzofurans, that provide protection against damaging effect of light, acts as weathering compounds also exhibit antiherbicidal property. Among other metabolites, barbatic acid, barbatolic acids, diffractaic acid, and evernic acids are well-known biological control agents for pests and insects (Kanivebagilu and Mesta, 2020). GC-MS analysis of Teloschistes flavicans showed vicanicin, methyl oleate, methyl palmitate, and patchouli alcohol were the main compounds showing anti-termite activity (Avidlyandi et al., 2021). Dermatocarpon miniatum, a species of lichen, had high amount of amino acids (histidine, alanine, cysteine, glutamate, aspartate, asparagine, glutamine, and glycine), organic acid (butyric, propanoic, malic, malonic, citric, maleic, and succinic acid), and hormones (GA, SA, IAA, and ABA) (Gunes et al., 2016). Along with Parmelia saxatilis, both species had sufficient macro-(N, P, K Ca, Mg, and S) and micro-(Fe, Cu, Mn, Zn, and B) nutrient contents. Both had significant potential to be used as an organic fertilizer for plant growth in organic farming.
Nanobiofertilizers as phytostimulants
Nanobiofertilizers are synthesized by using the desired microorganism grown over particular nutrient media; after complete growth phase, the biomass of microbes is separated, and the nanoparticles are synthesized using the extracellular secreted enzymes of bacteria which have the ability to penetrate into plant cells via stomatal or vascular system of plants and enhance the metabolic activities of plant cell and affect the productivity (El-Ghamry et al., 2018). Micronutrients and macronutrients are essential for the growth of plants, urea-coated zeolite chips, and urea-modified hydroxyapatite, and Ca and P hydroxyapatite nanoparticles increase the availability of N, Ca, and P resulting in 20–33% increment in plant yield (Liu and Lal, 2014). Nanobiofertilizers have the ability to make N available to plants and prevent the denitrification, volatilization, leaching, and fixation in soil by controlling the release of nutrients from nanoparticles. The zeolite increases the crop yield (Preetha and Balakrishnan, 2017; Singh, 2017). Nano-formulations using TiO2 increase root vigor because they have the ability to trap moisture content more than their size and make more water available to plant for vigorous growth. Nanoparticles form a strong interaction with genes of plant cells and enhance the working of metabolic machinery of cell, convert the leaves into biochemical sensors, and modify the expression system (Khodakovskaya et al., 2009; Nair et al., 2010). Nanoparticles assist the phytoremediation system by removing pollutants, stimulate plant growth, and promote the phytoavailability of pollutant. Plants under abiotic stress (heavy metal stress) increase the ROS which increase and trigger the expression of various genes when plants lag behind the destructive capacity of abiotic stress. Nanoparticles enhance the activities of antioxidant enzymes and stimulate accumulation of osmolytes and amino acids. Nanoparticles assist the phytoremediation by alleviating metal-induced toxicity in plants (Khan et al., 2017). For example, magnetite nanotubes act as a strong magnet and adsorb the metals from water and soil whereas zerovalent ion NPs act as reductant and reduced Cr (VI) to Cr (III) and reduced the bioavailability of Cr(VI) to Brassica juncea plants (Madhavi et al., 2013). Cu-NPs reduced the abiotic stress in tomato by increasing the activity of phenylalanine ammonia lyase (PAL), ascorbate peroxidase (APX), glutathione peroxidase (GPX), superoxide dismutase (SOD), and catalase (CAT) (Pérez-Labrada et al., 2019).
Conclusion
Plant growth-promoting fungi and PGPR are promising tools for sustainable agriculture. Their combined effort also mitigates climate-induced abiotic and biotic stresses experienced in plants and in bioremediation of pollutants. The mycelial web of PGPF increases the absorptive area of root enhancing water and nutrient uptake, solubilizes soil nutrients, and produces plant hormones (IAA, GA, and ABA), antibiotics, osmoregulants, and antioxidant enzymes which allow them to alleviate abiotic and biotic stresses. The mechanisms of PGPR include regulating hormonal and nutritional balance and induction of systemic resistance. In addition, PGPR show synergistic and antagonistic interactions with microorganisms within the rhizosphere and in bulk soil may be more beneficial for agricultural sustainability and healthier soil and ecosystem. The intricate relationship and cross talk between PGPR with PGPF/AMF and rhizobia may be investigated in more detail for better understanding signaling behavior and cross talk particularly under biotic and abiotic stresses. The PGPR from stressed rhizosphere and stressed plants impart tolerance to plants against stresses when used as bioinoculant. To function as phytostimulator, PGPR inoculants used in seed dressing or applied to rhizosphere should proliferate in the rhizosphere, make use of the organic/inorganic compounds exuded by host plant roots, and able to compete with indigenous microbes. The establishment of PGPR and PGPF depends on soil characteristics, soil health biotic and abiotic stresses, and environmental conditions. Their cross talk among each other and production of bioactive metabolites modulating the physiology of plants needs further investigation. Various antibiotics synthesized by PGPR which include phloroglucinols, D-gluconic acid, 2-hydroxymethyl-chroman-4-one, oomycin A, phenazine, pyoluteorin, pyrrolnitrin, tensin, tropolone, cyclic lipopeptide oligomycin A, kanosamine, zwittermicin A, and xanthobaccin inhibit the growth of zoospore to prevent proliferation of plant pathogens. Nanobiofertilizers assist the phytoremediation by alleviating metal-induced toxicity and bioavailability to plants. Azolla is an ideal candidate for intercropping. Lichens produce metabolites which induce protection against damaging effect of light, herbicides, act as effective source of macro- and micronutrient, and also act as indicator of the atmospheric pollutants. Cyanobacterial biomass is a pool of phenolic compounds, terpenoids, free fatty acids, polysaccharides, and carotenoids. These metabolites are active against phytopathogens, as resource for biodiesel production, and act as soil conditioner. Attention may also be given to cyanobacteria, Azolla, and lichen for their role in agroecosystem and their bioactive metabolites and the use of nanotechnology in biofertilizer formulation with beneficial microbes. A cumulative effect of the abovementioned phytostimulants may be applied to boost productivity, revegetate barren lands, and may also be implicated for sustainable healthier ecosystem.
Author contributions
AW, AK, and HT contributed to writing the review of three main domains of the fungi PGPR, cyanobacteria azolla, and lichens which was guided by AB. All authors contributed to the article and approved the submitted version.
Conflict of interest
The authors declare that the research was conducted in the absence of any commercial or financial relationships that could be construed as a potential conflict of interest.
Publisher's note
All claims expressed in this article are solely those of the authors and do not necessarily represent those of their affiliated organizations, or those of the publisher, the editors and the reviewers. Any product that may be evaluated in this article, or claim that may be made by its manufacturer, is not guaranteed or endorsed by the publisher.
References
Abas, A. (2021). A systematic review on biomonitoring using lichen as the biological indicator: A decade of practices, progress and challenges. Ecol. Indic. 121, 107197. doi: 10.1016/j.ecolind.2020.107197
Abinandan, S., Subashchandrabose, S. R., Venkateswarlu, K., and Megharaj, M. (2019). Soil microalgae and cyanobacteria: the biotechnological potential in the maintenance of soil fertility and health. Crit. Rev. Biotechnol. 39, 981–998. doi: 10.1080/07388551.2019.1654972
Altinok, H. H., Dikilitas, M., and Yildiz, H. N. (2013). Potential of Pseudomonas and Bacillus isolates as biocontrol agents against fusarium wilt of eggplant. Biotechnol. Biotechnol. Equip. 27, 3952–3958. doi: 10.5504/BBEQ.2013.0047
Amirnia, R., Ghiyasi, M., Siavash Moghaddam, S., Rahimi, A., Damalas, C. A., and Heydarzadeh, S. (2019). Nitrogen-fixing soil bacteria plus mycorrhizal fungi improve seed yield and quality traits of lentil (Lens culinaris Medik). J. Soil Sci. Plant Nutr. 19, 592–602. doi: 10.1007/s42729-019-00058-3
Avidlyandi, A., Adfa, M., and Yudha, S. S. (2021). Antitermite activity of methanol extract of lichen Teloschistes flavicans. (Sw) Norman against Coptotermes curvignathus. J. Phys. Conf. Ser. 1731, 012022. doi: 10.1088/1742-6596/1731/1/012022
Azizi, S., Tabari, M., Abad, A. R. F. N., Ammer, C., Guidi, L., and Bader, M. K. F. (2022). Soil inoculation with beneficial microbes buffers negative drought effects on biomass, nutrients, and water relations of common myrtle. Front. Plant Sci. 13, 892826. doi: 10.3389/fpls.2022.892826
Backer, R., Rokem, J. S., Ilangumaran, G., Lamont, J., Praslickova, D., Ricci, E., et al. (2018). Plant growth-promoting rhizobacteria: context, mechanisms of action, and roadmap to commercialization of biostimulants for sustainable agriculture. Front. Plant Sci. 9, 1473. doi: 10.3389/fpls.2018.01473
Bacon, C. W., and White, J. F. (2016). Functions, mechanisms and regulation of endophytic and epiphytic microbial communities of plants. Symbiosis 68, 87–98. doi: 10.1007/s13199-015-0350-2
Baghel, M., Nagaraja, A., Srivastav, M., Meena, N. K., Kumar, M. S., Kumar, A., et al. (2019). Pleiotropic influences of brassinosteroids on fruit crops: a review. Plant Growth Regul. 87, 375–388. doi: 10.1007/s10725-018-0471-8
Barbier, T., Machelart, A., Zúñiga-Ripa, A., Plovier, H., Hougardy, C., Lobet, E., et al. (2017). Erythritol availability in bovine, murine and human models highlights a potential role for the host aldose reductase during Brucella infection. Front. Microbiol. 8, 1088. doi: 10.3389/fmicb.2017.01088
Begum, N., Qin, C., Ahanger, M. A., Raza, S., Khan, M. I., Ashraf, M., et al. (2019). Role of arbuscular mycorrhizal fungi in plant growth regulation: implications in abiotic stress tolerance. Front. Plant Sci. 10, 1068. doi: 10.3389/fpls.2019.01068
Beneduzi, A., Ambrosini, A., and Passaglia, L. M. (2012). Plant growth-promoting rhizobacteria (PGPR): their potential as antagonists and biocontrol agents. Genet. Mol. Biol. 35, 1044–1051. doi: 10.1590/S1415-47572012000600020
Berthelot, H., Bonnet, S., Camps, M., Grosso, O., and Moutin, T. (2015). Assessment of the dinitrogen released as ammonium and dissolved organic nitrogen by unicellular and filamentous marine diazotrophic cyanobacteria grown in culture. Front. Mar. Sci. 2, 80. doi: 10.3389/fmars.2015.00080
Bižić, M., Klintzsch, T., Ionescu, D., Hindiyeh, M. Y., Günthel, M., Muro-Pastor, A. M., et al. (2020). Aquatic and terrestrial cyanobacteria produce methane. Sci. Adv. 6, eaax5343. doi: 10.1126/sciadv.aax5343
Bona, E., Cantamessa, S., Massa, N., Manassero, P., Marsano, F., Copetta, A., et al. (2017). Arbuscular mycorrhizal fungi and plant growth-promoting pseudomonads improve yield, quality and nutritional value of tomato: a field study. Mycorrhiza 27, 1–11. doi: 10.1007/s00572-016-0727-y
Bowles, T. M., Barrios-Masias, F. H., Carlisle, E. A., Cavagnaro, T. R., and Jackson, L. E. (2016). Effects of arbuscular mycorrhizae on tomato yield, nutrient uptake, water relations, and soil carbon dynamics under deficit irrigation in field conditions. Sci. Total Environ. 566, 1223–1234. doi: 10.1016/j.scitotenv.2016.05.178
Busby, P. E., Soman, C., Wagner, M. R., Friesen, M. L., Kremer, J., Bennett, A., et al. (2017). Research priorities for harnessing plant microbiomes in sustainable agriculture. PLoS Biol. 15, e2001793. doi: 10.1371/journal.pbio.2001793
Calcott, M. J., Ackerley, D. F., Knight, A., Keyzers, R. A., and Owen, J. G. (2018). Secondary metabolism in the lichen symbiosis. Chem. Soc. Rev. 47, 1730–1760. doi: 10.1039/C.7C.S.00431A
Carrapiço (2017). “The Azolla-Anabaena-Bacteria association: a case of symbiotic abduction?,” in Algal and Cyanobacteria Symbioses (pp. 329–345). doi: 10.1142/9781786340580_0010
Cataldo, E., Fucile, M., and Mattii, G. B. (2022). Biostimulants in viticulture: A sustainable approach against biotic and abiotic stresses. Plants 11, 162. doi: 10.3390/plants11020162
Chandrasekaran, M., Chanratana, M., Kim, K., Seshadri, S., and Sa, T. (2019). Impact of arbuscular mycorrhizal fungi on photosynthesis, water status, and gas exchange of plants under salt stress-a meta-analysis. Front. Plant Sci. 10, 457. doi: 10.3389/fpls.2019.00457
Chellaiah, E. R. (2018). Cadmium (heavy metals) bioremediation by Pseudomonas aeruginosa: a minireview. Appl. Water Sci. 8, 154. doi: 10.1007/s13201-018-0796-5
Cherif, A., Rezgui, W., Raddadi, N., Daffonchio, D., and Boudabous, A. (2008). Characterization and partial purification of entomocin 110, a newly identified bacteriocin from Bacillus thuringiensis subsp. Entomocidus HD110. Microbiol. Res. 163, 684–692. doi: 10.1016/j.micres.2006.10.005
Chuberre, C., Plancot, B., Driouich, A., Moore, J. P., Bardor, M., Gügi, B., et al. (2018). Plant immunity is compartmentalized and specialized in roots. Front. Plant Sci. 9, 1692. doi: 10.3389/fpls.2018.01692
Costa, O. Y., Raaijmakers, J. M., and Kuramae, E. E. (2018). Microbial extracellular polymeric substances: ecological function and impact on soil aggregation. Front. Microbiol. 9, 1636. doi: 10.3389/fmicb.2018.01636
Dal Cortivo, C., Ferrari, M., Visioli, G., Lauro, M., Fornasier, F., Barion, G., et al. (2020). Effects of seed-applied biofertilizers on rhizosphere biodiversity and growth of common wheat (Triticum aestivum L.) in the field. Front. Plant Sci. 11, 72. doi: 10.3389/fpls.2020.00072
Défago, G., and Haas, D. (2017). Pseudomonads as antagonists of soilborne plant pathogens: modes of action and genetic analysis. In Soil biochemistry (pp. 249-292). Routledge. doi: 10.1201/9780203739389-5
Desai, M. S., Seekatz, A. M., Koropatkin, N. M., Kamada, N., Hickey, C. A., Wolter, M., et al. (2016). A dietary fiber-deprived gut microbiota degrades the colonic mucus barrier and enhances pathogen susceptibility. Cell 167, 1339–1353. doi: 10.1016/j.cell.2016.10.043
Dhir, B. (2021). “Plant responses to heavy metals during cultivation in mining dump sites,” in Phytorestoration of Abandoned Mining and Oil Drilling Sites (Amsterdam: Elsevier), 359–372. doi: 10.1016/B978-0-12-821200-4.00010-8
Diagne, N., Ndour, M., Djighaly, P. I., Ngom, D., Ngom, M. C. N., Ndong, G., et al. (2020). Effect of plant growth promoting rhizobacteria (PGPR) and arbuscular mycorrhizal fungi (AMF) on salt stress tolerance of Casuarina obesa (Miq.). Front. Sustain. Food Syst. 4, 601004. doi: 10.3389/fsufs.2020.601004
Du Jardin, P. (2012). The Science of Plant Biostimulants-A Bibliographic Analysis, Ad Hoc Study Report.
Eily, A. N., Pryer, K. M., and Li, F. W. (2019). A first glimpse at genes important to the Azolla-Nostoc symbiosis. Symbiosis 78, 149–162. doi: 10.1007/s13199-019-00599-2
El Amerany, F., Meddich, A., Wahbi, S., Porzel, A., Taourirte, M., Rhazi, M., et al. (2020). Foliar application of chitosan increases tomato growth and influences mycorrhization and expression of endochitinase-encoding genes. Int. J. Mol. Sci. 21, 535. doi: 10.3390/ijms21020535
El-Ghamry, A., Mosa, A. A., Alshaal, T., and El-Ramady, H. (2018). Nanofertilizers vs. biofertilizers: new insights. Environ. Biodivers. Soil Secur. 2, 51–72. doi: 10.21608/jenvbs.2019.6775.1043
El-Maraghy, S. S., Tohamy, A. T., and Hussein, K. A. (2021). Plant protection properties of the plant growth-promoting fungi (PGPF): mechanisms and potentiality. Curr. Res. Environ. Appl. Mycol. 11, 391–415. doi: 10.5943/cream/11/1/29
El-Sawah, A. M., El-Keblawy, A., Ali, D. F. I., Ibrahim, H. M., El-Sheikh, M. A., Sharma, A., et al. (2021). Arbuscular mycorrhizal fungi and plant growth-promoting rhizobacteria enhance soil key enzymes, plant growth, seed yield, and qualitative attributes of guar. Agriculture 11, 194. doi: 10.3390/agriculture11030194
Evelin, H., Devi, T. S., Gupta, S., and Kapoor, R. (2019). Mitigation of salinity stress in plants by arbuscular mycorrhizal symbiosis: current understanding and new challenges. Front. Plant Sci. 10, 470. doi: 10.3389/fpls.2019.00470
Francioli, D., van Rijssel, S. Q., van Ruijven, J., Termorshuizen, A. J., Cotton, T. E., Dumbrell, A. J., et al. (2021). Plant functional group drives the community structure of saprophytic fungi in a grassland biodiversity experiment. Plant Soil 461, 91–105. doi: 10.1007/s11104-020-04454-y
Ghorbanpour, M., Omidvari, M., Abbaszadeh-Dahaji, P., Omidvar, R., and Kariman, K. (2018). Mechanisms underlying the protective effects of beneficial fungi against plant diseases. Biol. Control 117, 147–157. doi: 10.1016/j.biocontrol.2017.11.006
Ghosh, P. K., De, T. K., and Maiti, T. K. (2018). Role of ACC deaminase as a stress ameliorating enzyme of plant growth-promoting rhizobacteria useful in stress agriculture: a review. Role Rhizospheric Microb. Soil 2018, 57–106. doi: 10.1007/978-981-10-8402-7_3
Godino, A., Príncipe, A., and Fischer, S. (2016). A ptsP deficiency in PGPR Pseudomonas fluorescens SF39a affects bacteriocin production and bacterial fitness in the wheat rhizosphere. Res. Microbiol. 167, 178–189. doi: 10.1016/j.resmic.2015.12.003
Gonçalves, A. L. (2021). The use of microalgae and cyanobacteria in the improvement of agricultural practices: a review on their biofertilising, biostimulating and biopesticide roles. Appl. Sci. 11, 871. doi: 10.3390/app11020871
Gray, E. J., and Smith, D. L. (2005). Intracellular and extracellular PGPR: commonalities and distinctions in the plant-bacterium signaling processes. Soil Biol. Biochem. 37, 395–412. doi: 10.1016/j.soilbio.2004.08.030
Grover, M., Bodhankar, S., Sharma, A., Sharma, P., Singh, J., and Nain, L. (2021). PGPR mediated alterations in root traits: way toward sustainable crop production. Front. Sustain. Food Syst. 4, 618230. doi: 10.3389/fsufs.2020.618230
Guerreiro, M. A., Brachmann, A., Begerow, D., and Peršoh, D. (2018). Transient leaf endophytes are the most active fungi in 1-year-old beech leaf litter. Fungal Divers. 89, 237–251.s. doi: 10.1007/s13225-017-0390-4
Gunes, A., Aslan, A., and Turan, M. (2016). Biochemical properties of some lichen species as a source of organic fertilizer. Comp. Acad. Bulgare Sci. 69, 539–549.
Gupta, C., Rabani, M. S., Gupta, M. K., Habib, A., Pathak, A., Tripathi, S., et al. (2021). “Cyanobacteria as sustainable microbiome for agricultural industries,” in: Microbiota and Biofertilizers, Vol 2, eds Dar, G. H., Bhat, R. A., Mehmood, M. A., Hakeem, K. R. (Cham: Springer). doi: 10.1007/978-3-030-61010-4_13
Hassan, T. U., Bano, A., and Naz, I. (2017). Alleviation of heavy metals toxicity by the application of plant growth promoting rhizobacteria and effects on wheat grown in saline sodic field. Int. J. Phytoremed. 19, 522–529. doi: 10.1080/15226514.2016.1267696
He, H., Miao, R., Huang, L., Jiang, H., and Cheng, Y. (2021). Vegetative cells may perform nitrogen fixation function under nitrogen deprivation in Anabaena sp. strain PCC 7120 based on genome-wide differential expression analysis. PLoS ONE 16, e0248155. doi: 10.1371/journal.pone.0248155
He, L., Li, C., and Liu, R. (2017). Indirect interactions between arbuscular mycorrhizal fungi and Spodoptera exigua alter photosynthesis and plant endogenous hormones. Mycorrhiza. 27, 525–535. doi: 10.1007/s00572-017-0771-2
He, L., Rodrigues, J. L. M., Soudzilovskaia, N. A., Barceló, M., Olsson, P. A., Song, C., et al. (2020). Global biogeography of fungal and bacterial biomass carbon in topsoil. Soil Biol. Biochem. 151, 108024. doi: 10.1016/j.soilbio.2020.108024
Huang, L., Chen, D., Zhang, H., Song, Y., Chen, H., and Tang, M. (2019). Funneliformis mosseae enhances root development and Pb phytostabilization in Robinia pseudoacacia in Pb-contaminated soil. Front. Microbiol. 10, 2591. doi: 10.3389/fmicb.2019.02591
Huang, Y. T., Cai, S. Y., Ruan, X. L., Chen, S. Y., Mei, G. F., Ruan, G. H., et al. (2021). Salicylic acid enhances sunflower seed germination under Zn2+ stress via involvement in Zn2+ metabolic balance and phytohormone interactions. Sci. Hortic. 275, 109702. doi: 10.1016/j.scienta.2020.109702
Ikram, M., Ali, N., Jan, G., Jan, F. G., Rahman, I. U., Iqbal, A., et al. (2018). IAA producing fungal endophyte Penicillium roqueforti Thom., enhances stress tolerance and nutrients uptake in wheat plants grown on heavy metal contaminated soils. PLoS ONE 13, e0208150. doi: 10.1371/journal.pone.0208150
Islam, M. A., Nain, Z., Alam, M. K., Banu, N. A., and Islam, M. R. (2018). In vitro study of biocontrol potential of rhizospheric Pseudomonas aeruginosa against Fusarium oxysporum f. sp. cucumerinum. Egypt. J. Biol. Pest Control 28, 1–11. doi: 10.1186/s41938-018-0097-1
Jiang, Y. N., Wang, W. X., Xie, Q. J., Liu, N., Liu, L. X., Wang, D. P., et al. (2017). Plants transfer lipids to sustain colonization by mutualistic mycorrhizal and parasitic fungi. Science. 356, 1172–1175. doi: 10.1126/science.aam9970
Jiao, X., Takishita, Y., Zhou, G., and Smith, D. L. (2021). Plant associated rhizobacteria for biocontrol and plant growth enhancement. Front. Plant Sci. 12, 634796. doi: 10.3389/fpls.2021.634796
Ju, W., Liu, L., Fang, L., Cui, Y., Duan, C., and Wu, H. (2019). Impact of co-inoculation with plant-growth-promoting rhizobacteria and rhizobium on the biochemical responses of alfalfa-soil system in copper contaminated soil. Ecotoxicol. Environ. Saf. 167, 218–226. doi: 10.1016/j.ecoenv.2018.10.016
Kallamadi, P. R., Yadav, P., Dandu, K., Soni, P. K., Sankaraneni, C. R., Bharadwaja, K. P., et al. (2022). Host defense responses during powdery mildew (Golovinomyces latisporus comb. nov.) infection in sunflower (Helianthus annuus L.). Trop. Plant Pathol. 2021, 1–14. doi: 10.1007/s40858-022-00501-4
Kamali, S., and Mehraban, A. (2020). Nitroxin and arbuscular mycorrhizal fungi alleviate negative effects of drought stress on Sorghum bicolor yield through improving physiological and biochemical characteristics. Plant Signal. Behav. 15, 1813998. doi: 10.1080/15592324.2020.1813998
Kamle, M., Borah, R., Bora, H., Jaiswal, A. K., Singh, R. K., and Kumar, P. (2020). “Systemic acquired resistance (SAR) and induced systemic resistance (ISR): role and mechanism of action against phytopathogens,” in Fungal Biotechnology and Bioengineering (Cham: Springer), 457–470. doi: 10.1007/978-3-030-41870-0_20
Kanivebagilu, V. S., and Mesta, A. R. (2020). “Lichens: a novel group of natural biopesticidal sources,” in Plant Pathogens. (New York, NY: Apple Academic Press), 231–240.
Kannan, C. (2019). “Chapter-8 biological management of plant diseases,” in Current Research and Innovations in Plant Pathology. p. 167.
Kazerooni, E. A., Maharachchikumbura, S. S., Adhikari, A., Al-Sadi, A. M., Kang, S. M., Kim, L. R., et al. (2021). Rhizospheric Bacillus amyloliquefaciens protects Capsicum annuum cv. Geumsugangsan from multiple abiotic stresses via multifarious plant growth-promoting attributes. Front. Plant Sci. 12, 669693. doi: 10.3389/fpls.2021.669693
Khalediyan, N., Weisany, W., and Schenk, P. M. (2021). Arbuscular mycorrhizae and rhizobacteria improve growth, nutritional status and essential oil production in Ocimum basilicum and Satureja hortensis. Ind. Crops Prod. 160, 113163. doi: 10.1016/j.indcrop.2020.113163
Khan, M. N., Mobin, M., Abbas, Z. K., AlMutairi, K. A., and Siddiqui, Z. H. (2017). Role of nanomaterials in plants under challenging environments. Plant Physiol. Biochem. 110, 194–209. doi: 10.1016/j.plaphy.2016.05.038
Khodakovskaya, M., Dervishi, E., Mahmood, M., Xu, Y., Li, Z., Watanabe, F., et al. (2009). Carbon nanotubes are able to penetrate plant seed coat and dramatically affect seed germination and plant growth. ACS Nano 3, 3221–3227. doi: 10.1021/nn900887m
Kibuye, F. A., Zamyadi, A., and Wert, E. C. (2021). A critical review on operation and performance of source water control strategies for cyanobacterial blooms: Part II-mechanical and biological control methods. Harmful Algae 109, 102119. doi: 10.1016/j.hal.2021.102119
Kim, H. S., Sang, M. K., Jung, H. W., Jeun, Y. C., Myung, I. S., and Kim, K. D. (2012). Identification and characterization of Chryseobacterium wanjuense strain KJ9C8 as a biocontrol agent of Phytophthora blight of pepper. Crop Prot. 32, 129–137. doi: 10.1016/j.cropro.2011.10.018
Kishore, G. K., Pande, S., Rao, J. N., and Podile, A. R. (2005). Pseudomonas aeruginosa inhibits the plant cell wall degrading enzymes of Sclerotium rolfsii and reduces the severity of groundnut stem rot. Eur. J. Plant Pathol. 113, 315–320. doi: 10.1007/s10658-005-0295-z
Klawonn, I., Nahar, N., Walve, J., Andersson, B., Olofsson, M., and Svedén, J. (2016). Cell-specific nitrogen-and carbon-fixation of cyanobacteria in a temperate marine system (Baltic Sea). Environ. Microbiol. 18, 4596–4609. doi: 10.1111/1462-2920.13557
Klinsukon, C., Lumyong, S., Kuyper, T. W., and Boonlue, S. (2021). Colonization by arbuscular mycorrhizal fungi improves salinity tolerance of eucalyptus (Eucalyptus camaldulensis) seedlings. Sci. Rep. 11, 1–10. doi: 10.1038/s41598-021-84002-5
Kour, D., Rana, K. L., Kaur, T., Sheikh, I., Yadav, A. N., Kumar, V., et al. (2020). Microbe-mediated alleviation of drought stress and acquisition of phosphorus in great millet (Sorghum bicolor L.) by drought-adaptive and phosphorus-solubilizing microbes. Biocatal. Agric. Biotechnol. 23, 101501. doi: 10.1016/j.bcab.2020.101501
Kumar, A., and Meena, V. S. (2019). Plant Growth Promoting Rhizobacteria for Agricultural Sustainability, Vol. 10. Berlin: Springer, 978–981. doi: 10.1007/978-981-13-7553-8
Kumar, A., and Verma, J. P. (2018). Does plant-microbe interaction confer stress tolerance in plants: a review? Microbiol. Res. 207, 41–52. doi: 10.1016/j.micres.2017.11.004
Li, H., Ding, X., Wang, C., Ke, H., Wu, Z., Wang, Y., et al. (2016). Control of tomato yellow leaf curl virus disease by Enterobacter asburiae BQ9 as a result of priming plant resistance in tomatoes. Turk. J. Biol. 40, 150–159. doi: 10.3906/biy-1502-12
Li, H. P., Gan, Y. N., Yue, L. J., Han, Q. Q., Chen, J., Liu, Q. M., et al. (2022). Newly Isolated Paenibacillus monticola sp. nov., a novel plant growth-promoting rhizobacteria strain from high-altitude spruce forests in the Qilian Mountains, China. Front. Microbiol. 13, 833313. doi: 10.3389/fmicb.2022.833313
Li, J., Meng, B., Chai, H., Yang, X., Song, W., Li, S., et al. (2019). Arbuscular mycorrhizal fungi alleviate drought stress in C3 (Leymus chinensis) and C4 (Hemarthria altissima) grasses via altering antioxidant enzyme activities and photosynthesis. Front. Plant Sci. 10, 499. doi: 10.3389/fpls.2019.00499
Li, Z., Wu, N., Meng, S., Wu, F., and Liu, T. (2020). Arbuscular mycorrhizal fungi (AMF) enhance the tolerance of Euonymus maackii Rupr. at a moderate level of salinity. PLoS ONE 15, e0231497. doi: 10.1371/journal.pone.0231497
Lin, P. C., and Pakrasi, H. B. (2019). Engineering cyanobacteria for production of terpenoids. Planta 249, 145–154. doi: 10.1007/s00425-018-3047-y
Liu, N., Shao, C., Sun, H., Liu, Z., Guan, Y., Wu, L., et al. (2020). Arbuscular mycorrhizal fungi biofertilizer improves American ginseng (Panax quinquefolius L.) growth under the continuous cropping regime. Geoderma 363, 114155. doi: 10.1016/j.geoderma.2019.114155
Liu, R., and Lal, R. (2014). Synthetic apatite nanoparticles as a phosphorus fertilizer for soybean (Glycine max). Sci. Rep. 4, 5686. doi: 10.1038/srep05686
Ludueña, L. M., Anzuay, M. S., Angelini, J. G., McIntosh, M., Becker, A., Rupp, O., et al. (2018). Strain Serratia sp. S119: a potential biofertilizer for peanut and maize and a model bacterium to study phosphate solubilization mechanisms. Appl. Soil Ecol. 126, 107–112. doi: 10.1016/j.apsoil.2017.12.024
Lyu, D., Backer, R., and Smith, D. L. (2022). Three plant growth-promoting rhizobacteria alter morphological development, physiology, and flower yield of Cannabis sativa L. Ind. Crops Prod. 178, 114583. doi: 10.1016/j.indcrop.2022.114583
Madhavi, V. E. M. U. L. A., Prasad, T. N. V. K. V., Reddy, A. V. B., and Madhavi, G. (2013). Plant growth promoting potential of nano-bioremediation under Cr (vi) stress. Int. J. Nano Technol. Appl. 3, 1–10.
Madusanka, D. A. T., and Manage, P. M. (2018). Optimising a solvent system for lipid extraction from cyanobacterium Microcystis spp. future perspective for biodiesel production. Nat. Sci. Foundation Sri Lanka 46, 217–225. doi: 10.4038/jnsfsr.v46i2.8422
Maheshwari, D. K., Saraf, M., and Aeron, A. (2013). Bacteria in agrobiology. Dis. Manag. 2013, 473–485. doi: 10.1007/978-3-642-33639-3
Meena, M., Swapnil, P., Divyanshu, K., Kumar, S., Tripathi, Y. N., Zehra, A., et al. (2020). PGPR-mediated induction of systemic resistance and physiochemical alterations in plants against the pathogens: Current perspectives. J. Basic Microbiol. 60, 828–861. doi: 10.1002/jobm.202000370
Mohamed, I., Eid, K. E., Abbas, M. H., Salem, A. A., Ahmed, N., Ali, M., et al. (2019). Use of plant growth promoting Rhizobacteria (PGPR) and mycorrhizae to improve the growth and nutrient utilization of common bean in a soil infected with white rot fungi. Ecotoxicol. Environ. Saf. 171, 539–548. doi: 10.1016/j.ecoenv.2018.12.100
Mohammadi, K., and Sohrabi, Y. (2012). Bacterial biofertilizers for sustainable crop production: a review. ARPN J. Agric. Biol. Sci. 7, 307–316.
Moreira, C., Campos, A., Martins, J. C., Vasconcelos, V., and Antunes, A. (2021). Review on cyanobacterial studies in Portugal: current impacts and research needs. Appl. Sci. 11, 4355. doi: 10.3390/app11104355
Mukherjee, A., Verma, J. P., Gaurav, A. K., Chouhan, G. K., Patel, J. S., and Hesham, A. E. L. (2020). Yeast a potential bio-agent: future for plant growth and postharvest disease management for sustainable agriculture. Appl. Microbiol. Biotechnol. 104, 1497–1510. doi: 10.1007/s00253-019-10321-3
Murali, M., Naziya, B., Ansari, M. A., Alomary, M. N., AlYahya, S., Almatroudi, A., et al. (2021). Bioprospecting of rhizosphere-resident fungi: their role and importance in sustainable agriculture. J. Fungi 7, 314. doi: 10.3390/jof7040314
Nafady, N. A., Hashem, M., Hassan, E. A., Ahmed, H. A., and Alamri, S. A. (2019). The combined effect of arbuscular mycorrhizae and plant-growth-promoting yeast improves sunflower defense against Macrophomina phaseolina diseases. Biol. Control 138, 104049. doi: 10.1016/j.biocontrol.2019.104049
Nair, R., Varghese, S. H., Nair, B. G., Maekawa, T., Yoshida, Y., and Kumar, D. S. (2010). Nanoparticulate material delivery to plants. Plant Sci. 179, 154–163. doi: 10.1016/j.plantsci.2010.04.012
Nazari, M., and Smith, D. L. (2020). A PGPR-produced bacteriocin for sustainable agriculture: a review of thuricin 17 characteristics and applications. Front. Plant Sci. 11, 916. doi: 10.3389/fpls.2020.00916
Naziya, B., Murali, M., and Amruthesh, K. N. (2019). Plant growth-promoting fungi (PGPF) instigate plant growth and induce disease resistance in Capsicum annuum L. upon infection with Colletotrichum capsici (Syd.) Butler and Bisby. Biomolecules 10, 41. doi: 10.3390/biom10010041
Oliveira, R. S., Carvalho, P., Marques, G., Ferreira, L., Nunes, M., Rocha, I., et al. (2017b). Increased protein content of chickpea (Cicer arietinum L.) inoculated with arbuscular mycorrhizal fungi and nitrogen-fixing bacteria under water deficit conditions. J. Sci. Food Agric. 97, 4379–4385. doi: 10.1002/jsfa.8201
Oliveira, R. S., Carvalho, P., Marques, G., Ferreira, L., Pereira, S., Nunes, M., et al. (2017a). Improved grain yield of cowpea (Vigna unguiculata) under water deficit after inoculation with Bradyrhizobium elkanii and Rhizophagus irregularis. Crop Pasture Sci. 68, 1052–1059. doi: 10.1071/CP17087
Pan, J., Huang, C., Peng, F., Zhang, W., Luo, J., Ma, S., et al. (2020). Effect of arbuscular mycorrhizal fungi (AMF) and plant growth-promoting bacteria (PGPR) inoculations on Elaeagnus angustifolia L. in saline soil. Appl. Sci. 10, 945. doi: 10.3390/app10030945
Patel, P. R., Shaikh, S. S., and Sayyed, R. Z. (2018). Modified chrome azurol S method for detection and estimation of siderophores having affinity for metal ions other than iron. Environ. Sustain. 1, 81–87. doi: 10.1007/s42398-018-0005-3
Pathak, R., Shrestha, A., Lamichhane, J., and Gauchan, D. P. (2017). PGPR in biocontrol: mechanisms and roles in disease suppression. Int. J. Agron. Agric. 11, 69–80.
Pel, M. J., and Pieterse, C. M. (2013). Microbial recognition and evasion of host immunity. J. Exp. Bot. 64, 1237–1248. doi: 10.1093/jxb/ers262
Pérez-Labrada, F., López-Vargas, E. R., Ortega-Ortiz, H., Cadenas-Pliego, G., Benavides-Mendoza, A., and Juárez-Maldonado, A. (2019). Responses of tomato plants under saline stress to foliar application of copper nanoparticles. Plants 8, 151. doi: 10.3390/plants8060151
Persson, T., Battenberg, K., Demina, I. V., Vigil-Stenman, T., Vanden Heuvel, B., Pujic, P., et al. (2015). Candidatus Frankia datiscae Dg1, the actinobacterial microsymbiont of Datisca glomerata, expresses the canonical nod genes nodABC in symbiosis with its host plant. PLoS ONE 10, e0127630. doi: 10.1371/journal.pone.0127630
Pieterse, C. M., Zamioudis, C., Berendsen, R. L., Weller, D. M., Van Wees, S. C., and Bakker, P. A. (2014). Induced systemic resistance by beneficial microbes. Annu. Rev. Phytopathol. 52. doi: 10.1146/annurev-phyto-082712-102340
Pramanik, K., Mitra, S., Sarkar, A., and Maiti, T. K. (2018). Alleviation of phytotoxic effects of cadmium on rice seedlings by cadmium resistant PGPR strain Enterobacter aerogenes MCC 3092. J. Hazard. Mater. 351, 317–329. doi: 10.1016/j.jhazmat.2018.03.009
Prathap, S., Thiyageshwari, S., Krishnamoorthy, R., Prabhaharan, J., Vimalan, B., Gopal, N. O., et al. (2022). Role of zinc solubilizing bacteria in enhancing growth and nutrient accumulation in rice plants (Oryza sativa) grown on zinc (Zn) deficient submerged soil. J. Soil Sci. Plant Nutr. 22, 971–984. doi: 10.1007/s42729-021-00706-7
Preetha, P. S., and Balakrishnan, N. (2017). A review of nano fertilizers and their use and functions in soil. Int. J. Curr. Microbiol. App. Sci 6, 3117–3133. doi: 10.20546/ijcmas.2017.612.364
Pusztahelyi, T. (2018). Chitin and chitin-related compounds in plant-fungal interactions. Mycology 9, 189–201. doi: 10.1080/21501203.2018.1473299
Rahman, M. U., Fleming, D. F., Wang, L., Rumbaugh, K. P., Gordon, V. D., and Christopher, G. F. (2022). Microrheology of Pseudomonas aeruginosa biofilms grown in wound beds. NPJ Biofilms Microbiom. 8, 1–9. doi: 10.1038/s41522-022-00311-1
Rajkumar, M., Ae, N., Prasad, M. N. V., and Freitas, H. (2010). Potential of siderophore-producing bacteria for improving heavy metal phytoextraction. Trends Biotechnol. 28, 142–149. doi: 10.1016/j.tibtech.2009.12.002
Raklami, A., Bechtaoui, N., Tahiri, A. I., Anli, M., Meddich, A., and Oufdou, K. (2019). Use of rhizobacteria and mycorrhizae consortium in the open field as a strategy for improving crop nutrition, productivity and soil fertility. Front. Microbiol. 10, 1106. doi: 10.3389/fmicb.2019.01106
Rostami, F., Heydari, M., Golchin, A., and Igdelou, N. K. M. (2021). Effect of bio-fertilizers on corn (Zea mays L.) growth characteristics in Cd-spiked soils. Int. J. Environ. Sci.Technol. 18, 2651–2660. doi: 10.1007/s13762-020-03000-8
Santoyo, G., Gamalero, E., and Glick, B. R. (2021). Mycorrhizal-bacterial amelioration of plant abiotic and biotic stress. Front. Sustain. Food Syst. 5, 672881. doi: 10.3389/fsufs.2021.672881
Sarabia, M., Cazares, S., González-Rodríguez, A., Mora, F., Carreón-Abud, Y., and Larsen, J. (2018). Plant growth promotion traits of rhizosphere yeasts and their response to soil characteristics and crop cycle in maize agroecosystems. Rhizosphere 6, 67–73. doi: 10.1016/j.rhisph.2018.04.002
Sharma, P., Tripathi, S., Purchase, D., and Chandra, R. (2021). Integrating phytoremediation into treatment of pulp and paper industry wastewater: field observations of native plants for the detoxification of metals and their potential as part of a multidisciplinary strategy. J. Environ. Chem. Eng. 9, 105547. doi: 10.1016/j.jece.2021.105547
Sharma, S., Chandra, D., and Sharma, A. K. (2021). “Rhizosphere Plant-Microbe Interactions Under Abiotic Stress,” in Rhizosphere Biology: Interactions Between Microbes and Plants (Singapore: Springer), 195–216. doi: 10.1007/978-981-15-6125-2_10
Sharma, S., Prasad, R., Varma, A., and Sharma, A. K. (2017). Glycoprotein associated with Funneliformis coronatum, Gigaspora margarita and Acaulospora scrobiculata suppress the plant pathogens in vitro. Asian J. Plant Pathol. 11, 192–202. doi: 10.3923/ajppaj.2017.199.202
Singh, M. D. (2017). Nano-fertilizers is a new way to increase nutrients use efficiency in crop production. Int. J. Agric. Sci. 2017, 975–3710.
Subramanian, S., and Smith, D. L. (2015). Bacteriocins from the rhizosphere microbiome-from an agriculture perspective. Front. Plant Sci. 6, 909. doi: 10.3389/fpls.2015.00909
Suresh, P., Varathraju, G., Shanmugaiah, V., Almaary, K. S., Elbadawi, Y. B., and Mubarak, A. (2021). Partial purification and characterization of 2, 4-diacetylphloroglucinol producing Pseudomonas fluorescens VSMKU3054 against bacterial wilt disease of tomato. Saudi J. Biol. Sci. 28, 2155–2167. doi: 10.1016/j.sjbs.2021.02.073
Tedersoo, L., Anslan, S., Bahram, M., Drenkhan, R., Pritsch, K., Buegger, F., et al. (2020). Regional-scale in-depth analysis of soil fungal diversity reveals strong pH and plant species effects in Northern Europe. Front. Microbiol. 11, 1953. doi: 10.3389/fmicb.2020.01953
Teixeira, P. J. P., Colaianni, N. R., Fitzpatrick, C. R., and Dangl, J. L. (2019). Beyond pathogens: microbiota interactions with the plant immune system. Curr. Opin. Microbiol. 49, 7–17. doi: 10.1016/j.mib.2019.08.003
Tewari, R. S., and Arora, N. K. (2018). Role of salicylic acid from Pseudomonas aeruginosa PF23 EPS+ in growth promotion of sunflower in saline soils infested with phytopathogen Macrophomina phaseolina. Environ. Sustain. 1, 49–59. doi: 10.1007/s42398-018-0002-6
Vaahtera, L., Schulz, J., and Hamann, T. (2019). Cell wall integrity maintenance during plant development and interaction with the environment. Nat. Plants 5, 924–932. doi: 10.1038/s41477-019-0502-0
Watcharin, Y. (2015). Enhancement of Arbuscular Mycorrhizal Inoculant Production Using Plant Growth Promoting Rhizobacteria (PGPR). Doctoral dissertation, School of Biotechnology Institute of Agricultural Technology Suranaree University of Technology.
Weller, D. M., Landa, B. B., Mavrodi, O. V., Schroeder, K. L., De La Fuente, L., Blouin Bankhead, S., et al. (2007). Role of 2, 4-diacetylphloroglucinol-producing fluorescent Pseudomonas spp. in the defense of plant roots. Plant Biol. 9, 4–20. doi: 10.1055/s-2006-924473
Wilkes, R. A., Waldbauer, J., and Aristilde, L. (2021). Analogous metabolic decoupling in Pseudomonas putida and Comamonas testosteroni implies energetic bypass to facilitate gluconeogenic growth. MBio 12, e03259–e03221. doi: 10.1128/mbio.03259-21
Wu, Y., Zhao, C., Farmer, J., and Sun, J. (2015). Effects of bio-organic fertilizer on pepper growth and Fusarium wilt biocontrol. Sci. Hortic. 193, 114–120. doi: 10.1016/j.scienta.2015.06.039
Xu, C., and Mou, B. (2018). Chitosan as soil amendment affects lettuce growth, photochemical efficiency, and gas exchange. HortTechnology 28, 476–480. doi: 10.21273/HORTTECH04032-18
Xu, H., Cao, Y., Yu, D., Cao, M., He, Y., Gill, M., et al. (2021). Ensuring effective implementation of the post-2020 global biodiversity targets. Nat. Ecol. Evol. 5, 411–418. doi: 10.1038/s41559-020-01375-y
Yadav, R., Ror, P., Rathore, P., Kumar, S., and Ramakrishna, W. (2021). Bacillus subtilis CP4, isolated from native soil in combination with arbuscular mycorrhizal fungi promotes biofortification, yield and metabolite production in wheat under field conditions. J. Appl. Microbiol. 131, 339–359. doi: 10.1111/jam.14951
Yakhin, O. I., Lubyanov, A. A., Yakhin, I. A., and Brown, P. H. (2017). Biostimulants in plant science: a global perspective. Front. Plant Sci. 7, 2049. doi: 10.3389/fpls.2016.02049
Yan, L., Zhu, J., Zhao, X., Shi, J., Jiang, C., and Shao, D. (2019). Beneficial effects of endophytic fungi colonization on plants. Appl. Microbiol. Biotechnol. 103, 3327–3340. doi: 10.1007/s00253-019-09713-2
Yang, G., Ji, H., Liu, H., Feng, Y., Zhang, Y., Chen, L., et al. (2021). Nitrogen fertilizer reduction in combination with Azolla cover for reducing ammonia volatilization and improving nitrogen use efficiency of rice. PeerJ 9, e11077. doi: 10.7717/peerj.11077
Yang, M., Mavrodi, D. V., Mavrodi, O. V., Thomashow, L. S., and Weller, D. M. (2017). Construction of a recombinant strain of Pseudomonas fluorescens producing both phenazine-1-carboxylic acid and cyclic lipopeptide for the biocontrol of take-all disease of wheat. Eur. J. Plant Pathol. 149, 683–694. doi: 10.1007/s10658-017-1217-6
Yu, Y., Gui, Y., Li, Z., Jiang, C., Guo, J., and Niu, D. (2022). Induced systemic resistance for improving plant immunity by beneficial microbes. Plants 11, 386. doi: 10.3390/plants11030386
Zebelo, S., Song, Y., Kloepper, J. W., and Fadamiro, H. (2016). Rhizobacteria activates (+)-delta-cadinene synthase genes and induces systemic resistance in cotton against beet armyworm (Spodoptera exigua). Plant Cell Environ. 39, 935–943. doi: 10.1111/pce.12704
Zhang, F., Wang, P., Zou, Y. N., Wu, Q. S., and Kuca, K. (2019). Effects of mycorrhizal fungi on root-hair growth and hormone levels of taproot and lateral roots in trifoliate orange under drought stress. Arch. Agronom. Soil Sci. 65, 1316–1330. doi: 10.1080/03650340.2018.1563780
Zhang, Q., Gong, M., Liu, K., Chen, Y., Yuan, J., and Chang, Q. (2020). Rhizoglomus intraradices improves plant growth, root morphology and Phytohormone balance of Robinia pseudoacacia in arsenic-contaminated soils. Front. Microbiol. 11, 1428. doi: 10.3389/fmicb.2020.01428
Keywords: phytostimulant, PGPR, PGPF, cyanobacteria, lichen, sustainable agriculture, secondary metabolites, azolla
Citation: Bano A, Waqar A, Khan A and Tariq H (2022) Phytostimulants in sustainable agriculture. Front. Sustain. Food Syst. 6:801788. doi: 10.3389/fsufs.2022.801788
Received: 25 October 2021; Accepted: 27 June 2022;
Published: 15 August 2022.
Edited by:
Nicolas Desoignies, HEPH Condorcet, BelgiumReviewed by:
Jai Prakash, Babasaheb Bhimrao Ambedkar University, IndiaAjar Nath Yadav, Eternal University, India
Copyright © 2022 Bano, Waqar, Khan and Tariq. This is an open-access article distributed under the terms of the Creative Commons Attribution License (CC BY). The use, distribution or reproduction in other forums is permitted, provided the original author(s) and the copyright owner(s) are credited and that the original publication in this journal is cited, in accordance with accepted academic practice. No use, distribution or reproduction is permitted which does not comply with these terms.
*Correspondence: Asghari Bano, YmFuby5hc2doYXJpQGdtYWlsLmNvbQ==