- Department of Plant Science, McGill University, Montreal, QC, Canada
The negative effects of more extreme pH conditions (soil acidity and alkalinity) are increasingly challenging crop production. Managing acidity and alkalinity in soils has been achieved through techniques such as the use of lime, afforestation, tillage, and addition of organic matter. The use of microbes to address this challenge is new and could increase agroecosystem sustainability while helping plants survive more extreme acidity and alkalinity, among other stresses. Use of plant growth promoting microbes (PGPM) has recently gained attention as these microbes afford plants several benefits, including nutrient acquisition and stress tolerance, both biotic and abiotic. Several methods of microbe application have been developed, all intended to maximize the benefits of plant-microbial interactions. The current study assessed the potential of changing microbial culture pH during production, followed by removal of cells to produce supernatant that enhances plant growth, specifically under acidity and alkalinity stresses. The study included L. helveticus. (EL2006H) and B. subtilis (EB2004S) which were cultured at three pH levels (5, 7, and 8) incubated for 24–48 h then centrifuged at 12 000 g to remove the cells. The cell-free supernatants obtained were used for seed germination and early seedling growth assays. The results indicated significant increase in seed germination rate, for both corn and tomato, compared to experimental controls. Supernatants produced at pH 5, for both strains, had greater effect than those produced at pHs 7 and 8. Similarly, the positive effect of these supernatants was observed in seedling growth as increased root length and volume. Their results indicate that there is potential in stressing microbes below or above optimum pH (~7) to induce production and excretion of favorable materials into the growth medium, as was evident in this study. To the best of our knowledge this would be the first attempt to look at this pH change to increase potential benefits related to plant growth promotion by microbes. It was interesting to learn that using the CFS of microbes cultured at pH 5 increased germination rate and seedling growth. These results provide an initial indication that support broadened research into PGPM under pH stressed conditions.
Introduction
Worldwide the effects of soil acidity and alkalinity are increasingly challenging crop production and the plant science community attempting to improve yields (Liu et al., 2019). Nearly 3% of the global geographic area is dominated by saline-sodic soils (Singh et al., 2016) and about 30% of ice free land in the world is acidic (Mehmood et al., 2017). In America alone acidic soils cover about 40% of potential arable land (Von Uexküll and Mutert, 1995; Ngoune Tandzi et al., 2018), putting pressure on crop production management, productivity and sustainability. Dealing with abiotic stresses requires short- and long-term interventions. Acidity and alkalinity impact crop production; various severe effects are seen in plant root system damage and the resulting imbalance of nutrient availability from soil (Sapre et al., 2018). Acidity and alkalinity can be corrected by deploying techniques such as the use of lime, afforestation, tillage and addition of organic matter (OM) in soil (Machado and Serralheiro, 2017).
The use of microbes has been reported to assist plant survival and robustness at various levels of alkalinity and acidity, among other stresses they face (Backer et al., 2018). The use of plant growth promoting microbes (PGPM) has gained scientific attention during the past decade, as these microbes afford plants a range of benefits. The major contributions of microbes to plants include their ability to assist in plant nutrient acquisition (Sashidhar and Podile, 2010; Kalayu, 2019), stress tolerance both abiotic (Pandey et al., 2012; Msimbira and Smith, 2020) and biotic (Takishita, 2018; Mahmood et al., 2019). Microbes achieve these benefits to plants through various mechanisms. To find these beneficial strains, one generally begins by screening for their performance in terms of a particular aspect of plant-microbe interaction. With knowledge improvement and development, combination of several microbial strains, to form a consortium is possible, and leads to broad-spectrum effects of these technologies when deployed. Furthermore, it has been shown that these microbes, whether applied singly or as a consortium, produce specific compounds which are directly or indirectly beneficial to plants (Antar et al., 2021).
Some of the already studied compounds released by PGPM include phytohormones, LCOs and bacteriocins (Gray et al., 2006; Smith et al., 2015). Given the current understanding, there is a need to identify specific compounds responsible for assisting plants confronted with specific stresses by taking a more holistic approach, one favoring production of the beneficial compounds from a particular microbe. The use of microbial cell-free supernatants (CFS) is another emerging and potentially important field, as reviewed by Pellegrini et al. (2020), that could optimize the PGPM harnessed benefits. CFSs contain a range of compounds and are obtained through a range of methods, including mechanical separation by centrifugation. Compounds released by microbes in CFSs reported to have plants growth promotion activity include but not limited to Indole-3-acetic acid (IAA) (Yahalom et al., 1990; El-Khawas and Adachi, 1999; Molla et al., 2001; Idris et al., 2004; Morel et al., 2015; Tallapragada et al., 2015; Posada et al., 2016), Extracellular Proteins (EP) (Buensanteai et al., 2008; Buensateai et al., 2013), Lipopeptides (LP) (Buensanteai et al., 2008), Lipo-Chitin oligosaccharides (LCO) (Kidaj et al., 2012; Meena et al., 2012; Moretti et al., 2020), Indole-3-lactic acid (ILA) and gibberellins (GA) (Molla et al., 2001), L-lactic acid (LLA) (Rodríguez-Morgado et al., 2017; Caballero et al., 2020), Indolic compounds (Rondina et al., 2020), Siderophores (Dimkpa et al., 2009; Posada et al., 2016), Flavonoids and tryptophan (Trp) (Berquó Marks et al., 2013; Morel et al., 2015), and Peptides and amino acids (AA) (Caballero et al., 2020).
Review of the published literature indicates clearly that most research has focused on culturing microbes under the most optimal conditions (pH included) then evaluating their efficacy on stressed plants. To broaden the so-far-acquired knowledge regarding plant-microbe interaction, the present study was conducted to delve into the individual microbial strains of a plant growth promoting consortium currently marketed by EVL company. This is the first report documenting the effects of cell-free supernatants from microbial strains, produced at a range of pHs, on seed germination and early seedling growth of tomato and corn.
Materials and Methods
Preparation of CFS
The individual two microbial strains out of five constituting the biostimulant consortium from EVL Inc., stored in glycerol stocks, were used for this study. The stocks were stored at −80°C; a loopful was added into 50 mL fresh sterile M13 or MRS medium broth, contained in 250 mL flasks. The cultures were then incubated for 24–48 h at 30 or 37°C in an orbital shaker at 120 rpm (except for the Lactobacillus stain which does not require agitation) after which suitable dilution was carried out to obtain ~108 CFU at the required turbidity, measured as optical density (OD) at 600 nm. The pH tolerance screening for growth was conducted prior to the start of plant effect experiments (Table 1). Based on initial screening three pHs (5, 7, & 8) were selected for testing on seed germination and seedling growth. Microbial strains were then grown at these pHs after which cells were removed by centrifugation at 12,000 g and the cell free aliquots were used at various concentration as treatments in seed germination and seedling growth bioassays.
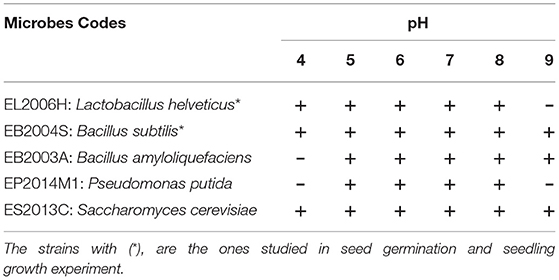
Table 1. Growth response at different pH levels for the studied microbial strains: +, growth and –, No growth.
Seed Germination Assay
Vigorous sorted seeds of corn (Zea mays L. var 25M75) and tomato (Solanum lycopersicum L. var. Beefsteak) were used in the study. The germination was 95 and 98% for corn and tomato, respectively. Seeds were surface sterilized to avoid fungal contamination using 70% ethyl alcohol for 2 min, then washed with 3.5% NaOCl for 5 min. Seeds were then thoroughly rinsed 5 times with deionized water. Surface sterilized seeds were germinated inside petri dishes (sterile 100 ×15 mm polystyrene Petri dishes) lined with filter paper (Fisherbrand™ - P8 Grade, Pittsburgh, US). Each petri dish received 10 seeds for corn and 20 for tomato. The treatment solutions were then prepared using deionized water and cell-free supernatant (CFS) with concentrations of 1, 0.4, 0.2, and 0.1% (v/v). The respective broth medium concentrations were used as a positive control while deionized water was used as a negative control. The filter paper of each Petri dish was then wetted with 5 and 4 mL of each concentration of the CFSs of corn and tomato, respectively.
Petri dishes were then sealed using parafilm to avoid water loss, which would hinder the normal process of germination. Petri dishes were arranged in a completely randomized design (CRD) with three replicates of each treatment in a germination cabinet set at 25°C with a relative humidity of 70% and 24 h darkness. Only two *microbial strains (Table 1) were selected as showed effect on seed germination assay with corn and tomato after screening (data not shown). In all experiments seeds were considered germinated when their radicle was about 2 mm long; data were collected at 24, 30, 42, and 54 h for corn and 48, 60, 72, and 84 h for tomato. The experiments were each repeated twice.
Early Seedling Growth Assay
Corn Seedling Growth Assay
Seeds were held in petri dishes until they had germinated (average radicle length of 2 cm, ~4 days after sowing) then transferred into magenta jars containing 50 mL of half strength Hoagland solution (Table 2). Magenta jars, containing deionized water adjusted to pH 7, were used as negative controls, while those which contained only culture medium without CFS were used as positive controls. At each pH level cell-free supernatant concentration was selected based on effect on seed germination and seedling growth effect during screening. The selected concentrations for B. subtilis EB2004S CFS was 1% (v/v) for all the pHs. The selected concentration for L. helveticus. were 0.4% for pH 5 and 1% for pH 7. One fully germinated seed was placed into each jar, ensuring the radicle was touching the solution after suspending it on mesh fixed in the jar.
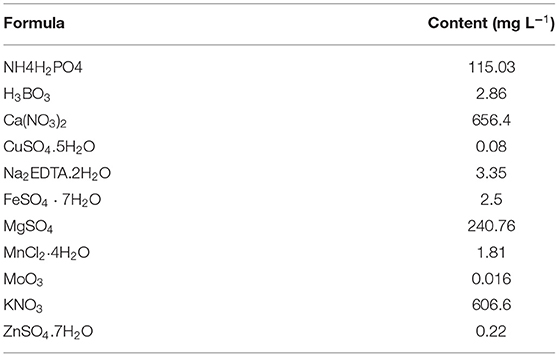
Table 2. Hoagland nutrient solution composition (macro and micronutrients) for seedling growth experiment, half of the concentration was applied during the experiment.
All magenta jars were then transferred to a growth chamber set at the maximum photosynthetically active radiation of 300 μmol m−2 s−1, for 14/10 h of light/darkness and temperatures of 25/20 ± 3°C, day/night. The experiment was organized following a CRD with four replicates of each treatment. Seedlings were allowed to grow in magenta jars for 14 days then destructively harvested. Variables measured after harvesting included seedling shoot height and whole seedling dry weight (WSDW), and individual seedling total roots scanned using an EPSON-Expression 11000XL scanner then analyzed for root length and volume, using WinRHIZO™ Pro software.
Tomato Seedling Growth Assay
Seeds were germinated in petri dishes until they had an average radicle length of 1.5 cm (~7 days after sowing) then they were transferred into seedling trays filled with 100 g of perlite. Seedling trays were then transferred to a growth chamber set at maximum photosynthetically active radiation (1,000 μmol m−2 s−1), for 14/10 h of light/darkness and temperatures of 25/20 ± 3°C, day/night. For the first 10 days the seedlings were watered using half strength Hoagland solution (10 mL per watering) at pH 7, alternating with water every other day. On the eleventh day seedlings were subjected to half strength hoagland solution at pH 5, 7 or 8, diluted to one of the concentrations of the CFSs while others remained as controls. Seedlings were left to grow in trays for another 11 days and then destructively harvested. Variables measured after harvesting included seedling shoot height and whole seedling dry weight (WSDW), and individual seedling total roots scanned using an EPSON-Expression 11000XL scanner then analyzed for root length and volume using WinRHIZO™ Pro software.
Data Analysis
To detect the differences between treatments for seedling growth experimental data were subjected to analysis of variance (ANOVA) using SAS® OnDemand for Academics. Where the program found treatment means to be different, they were separated using the Fisher least significant difference (LSD) at p ≤ 0.05.
Results and Discussion
Seed Germination
Effect of EB2004S and EL2006H CFS on Corn Seed Germination
There were no significant differences in germination of corn seeds across all treatments at the first 30 h after incubation (Figure 1). The tested CFS had significant effects on corn seed germination at 42 h (Figure 1). The effect of CFS from EB2004S at pH 5 showed greater influence on corn germination at the lowest concentration (0.1% v/v) as observed at pH 5 (Figure 1). Significant increases in cumulative corn seed germination were also detected for 0.4 and 1% (v/v) concentrations of CFS from pH7 for EB2004S (Figure 1). The un-inoculated (positive) control and those without CFS culture medium (negative) controls had the lowest germination rates, except for at pH 7 from EL2006H (Figure 1). Except for the CFS obtained from pH5 of EB2004S, positive controls had lower germination percentages at 42 h than the respective 1% CFS concentration. The performance of the CFSs at pH 8 at 1% (v/v) was significantly higher for corn seed germination at 42 h (Figure 1) than the control medium. While treating corn seeds with CFSs caused increased germination, it was still lower for CFSs from pH 8 than the effect obtained from those at pH 5 and 7 at 42 h. There was no statistically significant difference (p ≤ 0.05) in corn germination at 54 h among the treatments and controls of the experiment.
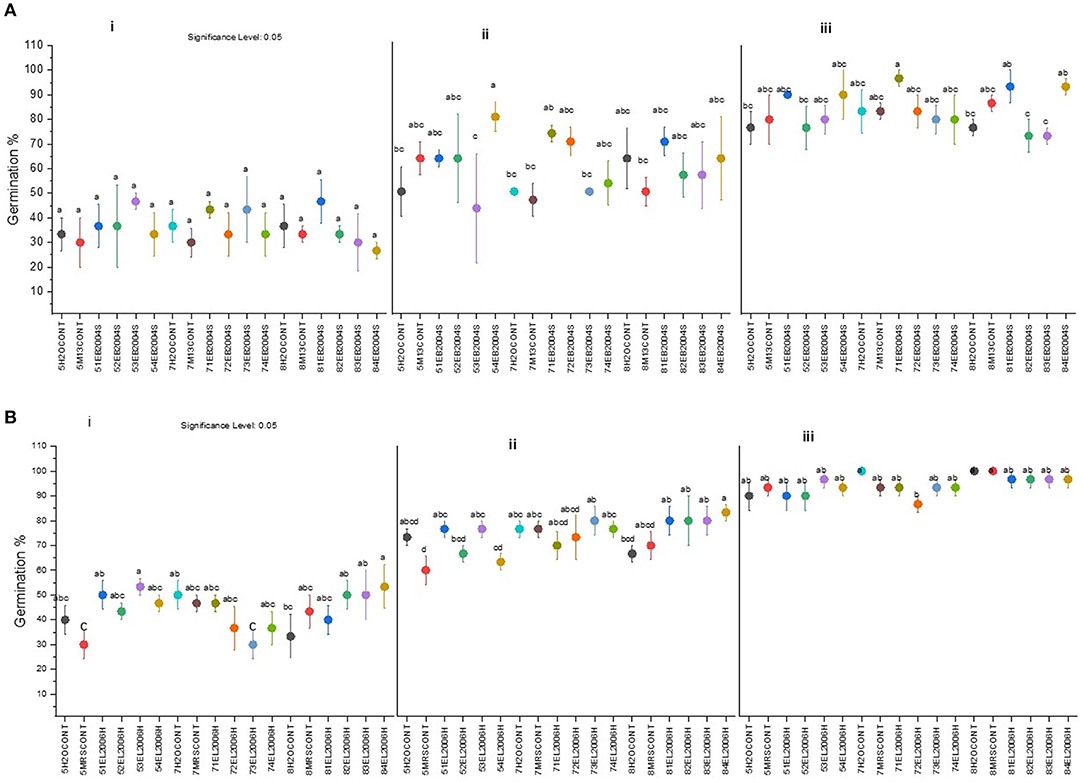
Figure 1. Cumulative seed germination for corn seeds treated with solutions of EB2004S CFS (A) obtained from pH 5 (5H2OCONT ~ Negative control, 5M13CONT ~ Positive control, 51EB2004S ~ 1%, 52EB2004S ~ 0.4%, 53EB2004S ~ 0.2% and 54EB2004S ~ 0.1%), pH7(7H2OCONT ~ Negative control, 7M13CONT ~ Positive control, 71EB2004S ~ 1%, 72EB2004S ~ 0.4%, 73EB2004S ~ 0.2% and 74EB2004S ~ 0.1%), and pH8 (8H2OCONT ~ Negative control, 8M13CONT ~ Positive control, 81EB2004S ~ 1%, 82EB2004S ~ 0.4%, 83EB2004S ~ 0.2% and 84EB2004S ~ 0.1%) and EL2006H CFS (B) obtained from pH 5 (5H2OCONT ~ Negative control, 5MRSCONT ~ Positive control, 51EL200H ~ 1%, 52EL2006H ~ 0.4%, 53EL2006H ~ 0.2% and 54EL2006H ~ 0.1%), pH7(7H2OCONT ~ Negative control, 7MRSCONT ~ Positive control, 71EL200H ~ 1%, 72EL2006H ~ 0.4%, 73EL200H ~ 0.2% and 74EL200H ~ 0.1%), and pH8 (8H2OCONT ~ Negative control, 8MRSCONT ~ Positive control, 81EL2006H~ 1%, 82EL2006H ~ 0.4%, 83EL2006H ~ 0.2% and 84EL2006H ~ 0.1%). All data for (A,B) were recorded at three time points 30 h (i), 42 h (ii), and 54 h (iii). Values are expressed as germination % means. Means, sharing the same letters are not significantly (p = 0.05, LSD) different among treatments.
Corn seed germination was greater for EL2006H CFS obtained at pH 5 than the medium control at the first 30 h after incubation (Figure 1). Treating tomato seeds with CFS obtained from pH 7 and 8 did not cause any significant cumulative increase in germination.
Effect of EB2004S and EL2006H CFS on Tomato Seed Germination
The tested CFS from pH 5, at all evaluated concentrations, slowed tomato seed germination both for EB2004S and EL2006H (Figures 2, 3B). The CFSs obtained from EB2004S had no effect on germination of tomato seeds. The same results were obtained for EL2006H CFS obtained from pH 7.
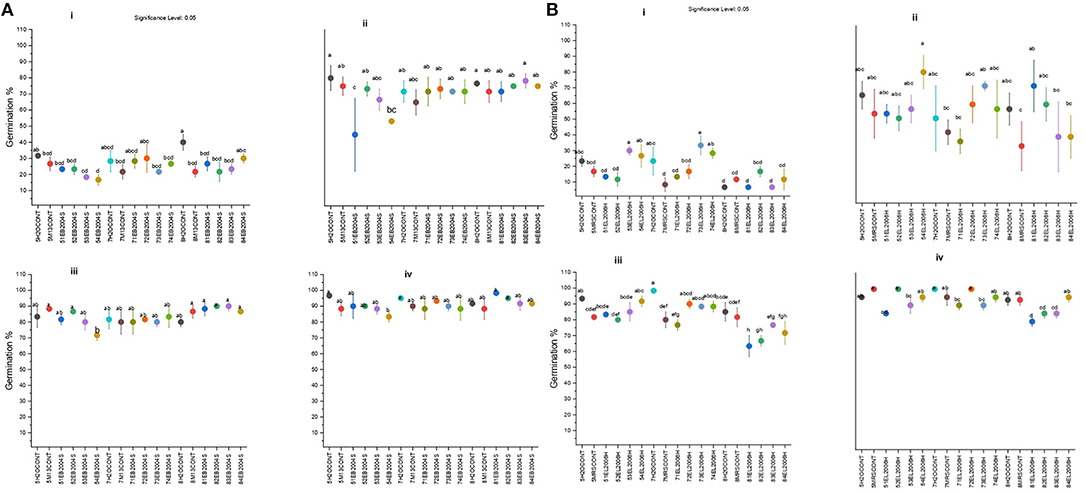
Figure 2. Cumulative seed germination for tomato seeds treated with solutions of EB2004S CFS (A) obtained from pH 5 (5H2OCONT ~ Negative control, 5M13CONT ~ Positive control, 51EB2004S ~ 1%, 52EB2004S ~ 0.4%, 53EB2004S ~ 0.2% and 54EB2004S ~ 0.1%), pH7(7H2OCONT ~ Negative control, 7M13CONT ~ Positive control, 71EB2004S ~ 1%, 72EB2004S ~ 0.4%, 73EB2004S ~ 0.2% and 74EB2004S ~ 0.1%), and pH8 (8H2OCONT ~ Negative control, 8M13CONT ~ Positive control, 81EB2004S ~ 1%, 82EB2004S ~ 0.4%, 83EB2004S ~ 0.2% and 84EB2004S ~ 0.1%) and EL2006H CFS (B) obtained from pH 5 (5H2OCONT ~ Negative control, 5MRSCONT ~ Positive control, 51EL200H ~ 1%, 52EL2006H ~ 0.4%, 53EL2006H ~ 0.2% and 54EL2006H ~ 0.1%), pH7(7H2OCONT ~ Negative control, 7MRSCONT ~ Positive control, 71EL200H ~ 1%, 72EL2006H ~ 0.4%, 73EL200H ~ 0.2% and 74EL200H ~ 0.1%), and pH8 (8H2OCONT ~ Negative control, 8MRSCONT ~ Positive control, 81EL2006H~ 1%, 82EL2006H ~ 0.4%, 83EL2006H ~ 0.2% and 84EL2006H ~ 0.1%). All data for (A,B) were recorded at four time points 48 h (i), 60 h (ii), 72 h (iii), and 84 h (iv). Values are expressed as germination % means. Means, sharing the same letters are not significantly (p = 0.05, LSD) different among treatments.
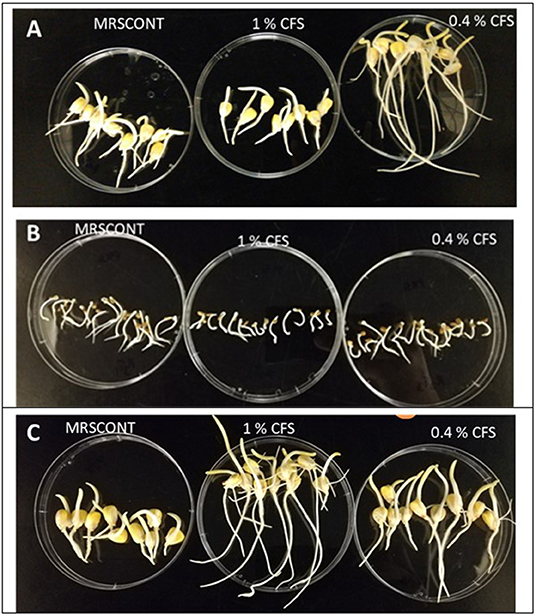
Figure 3. Some of the screening of CFS done before being selected as treatment concentrations for the seedling experiment. For Lactobacillus helveticus. CFS obtained from pH 5 tested as seen on (A,B) for corn and tomato, respectively, while CFS obtained from pH 7 is in (C).
Seedling Growth
Treatment Screening
The screening of the treatment concentrations was conducted so that the best was chosen, and other concentrations were not retained for further experimentation. To achieve this, data analysis of seed germination was used as the basis to further confirm the effect of the CFS on early seedling growth. Visual effects on roots development (number and length), seedling vigor and leaves appearance and size as seen in some of the selected concentrations used in the seedling growth experiments are provided in Figure 3.
Effect CFSs Obtained From Bacillus subtilis (EB2004S) on Corn and Tomato Seedling Growth
The ANOVA (Table 3) shows increased performance of seedlings treated with 1% (v/v) CFS obtained from EB2004S at pH 5 for root length (93.8 cm) and seedling height (9.1 cm) compared to un-inoculated controls. These results are congruent with those of corn seed germination when the same concentration was used. Similarly, root volume increased for corn seedlings treated with 1% (v/v) CFS from EB2004S at pH 5, compared to the controls (Table 3; Figure 4). Conversely, there was a statistically significant increase in seedling height (5.3 cm) at pH 8 when treated with CFS, compared to controls. Even though WSDW was not statistically different for corn seedlings at pH 5 and 8, it was numerically higher than the controls (Table 3) while a significant increase in WSWS (0.1598 g) occurred at pH 7 by EB2004S, over the controls.
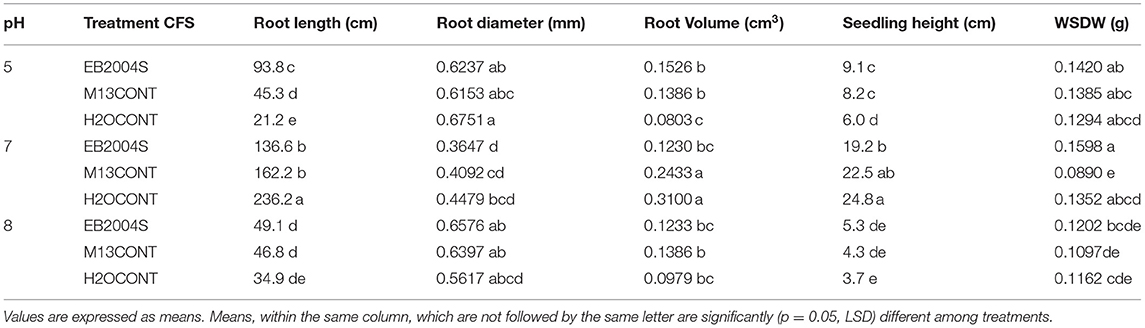
Table 3. Effect of Bacillus subtilis CFS on corn seedling growth variables at pH 5 and 8 grown in a growth chamber.
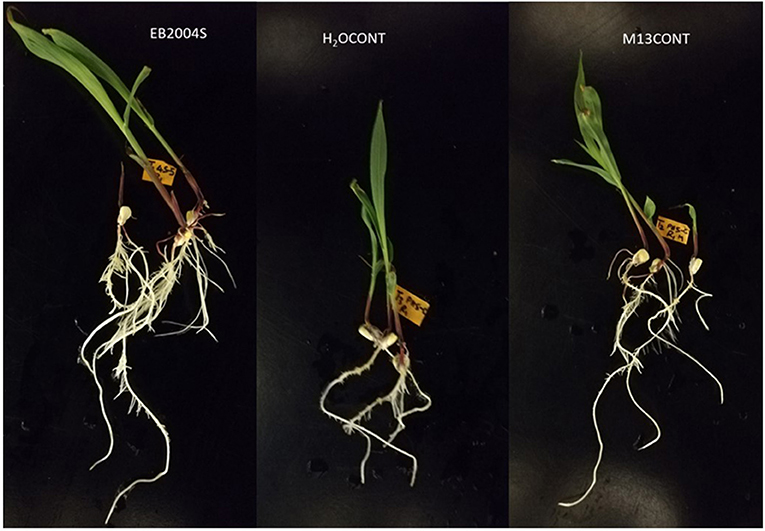
Figure 4. Visual effect of Bacillus subtilis. CFS on corn seedling growth variables at pH 5 grown in a growth chamber.
Except for seedling root diameter at pH 8, which was significantly increased (p = 0.05), the other variables did not respond to treatments across all pH levels tested (Table 4). Most of treated seedlings had lower variable values than the positive controls.
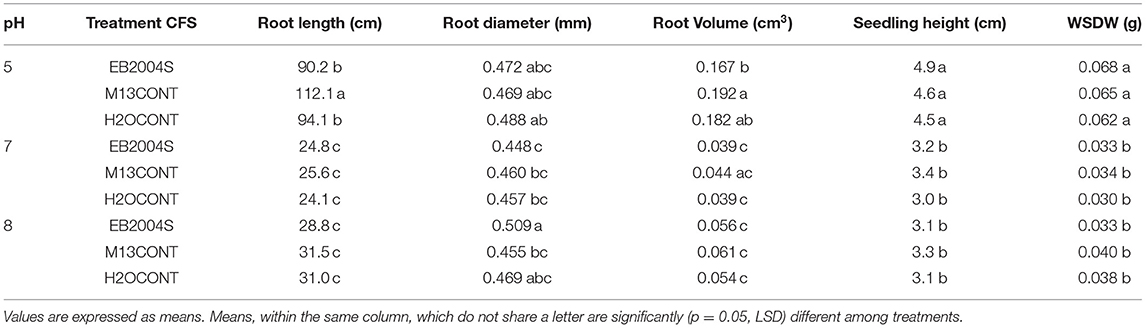
Table 4. Effect of Bacillus subtilis (EB2004S) CFS on tomato seedling growth variables at pH 5, 7 and 8 when grown in a growth chamber.
Effect of CFS Obtained From Lactobacillus helveticus (EL2006H) on Corn and Tomato Seedling Growth
Treatment of corn seedlings with CFS did not affect measured growth variables (Table 5), except for root volume, which was slightly increased over the positive control (Table 5).
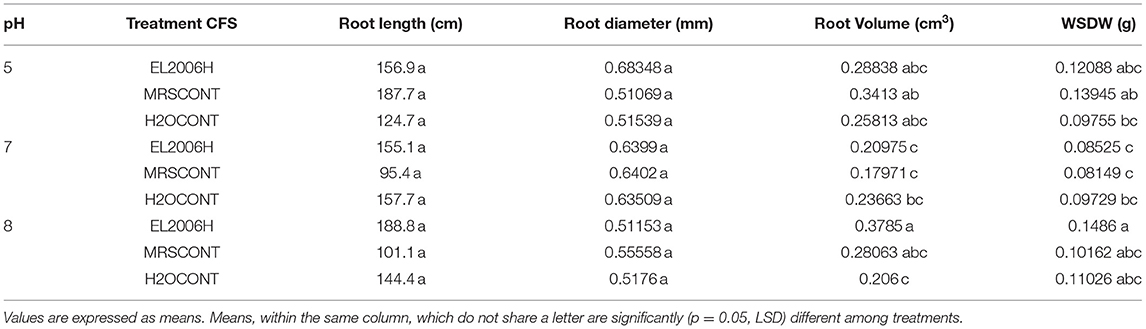
Table 5. Effect of Lactobacillus helveticus (EL2006H) CFS on corn seedling growth variables at pH 5, 7, and 8 when grown in a growth chamber.
Treatment with CFS did not affect tomato seedling growth for the measured variables, when compared to positive controls (Table 5; Figure 5). Only root volume was increased slightly, compared to positive controls, while causing small relative increases when compared to the negative control (Table 5).
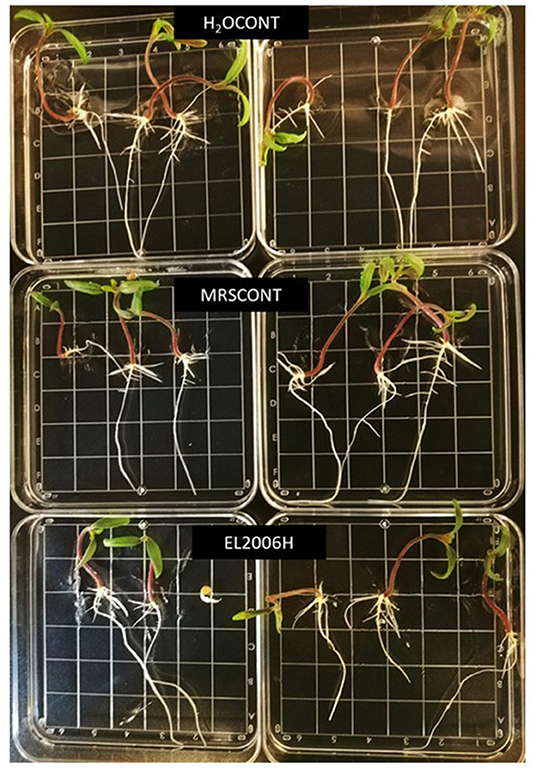
Figure 5. Visual effect of Lactobacillus helveticus. CFS on tomato seedling growth variables at pH 5 grown in a growth chamber.
Discussion
Seed Germination
Seed germination is a critical step in the progression of plant development, and particularly so for any plant that solely depends on seeds for propagation. Seeds that germinate robustly lead to early establishment of seedlings; as a result final production/yield is heavily impacted by these early plant growth stages (Tian et al., 2014). The present study has demonstrated that the use of CFS from EB2004S and EL2006H increased corn and tomato seed germination. Prior to evaluation of the CFSs, seeds were first germinated under normal conditions, to get the average germination percentages, to confirm the quality of the seed used (corn 95% and tomato 98%). Our study is one of several efforts to increase early plant establishment, particularly at high levels of abiotic stresses such as more extreme soil pHs. Previous studies have used a range of methods to improve seed germination including physical, physiological and biologicals (Afzal et al., 2016); the current study focused on the use of CFSs a derivative of biological seed enhancement applied at various concentrations obtained from pH 5, 7, and 8 to explore the potential of microbes in improving germination.
PGPM suspension are known to enhance tomato seed germination (Widnyana, 2018). Similar studies have been reported for corn such enhancement of seed germination in most plant species. Results from this study (Figures 1, 2) show that CFS increases the rate of seed germination, congruent with a study on CFSs to increase seed germination which was reported for Burkholderia seminalis on tomato (Tallapragada et al., 2015).
The CFS from EB2004S cultured at pH 5 positively effected corn seed germination at 42 h (Figure 1). The CFS obtained at pH 5 meaningfully enhanced corn germination, even at the lowest concentration of 0.1% (v/v) (Figure 1). The lowest concentration of CFS applied to corn seeds enhanced germination significantly, in contrast to an 8% (v/v) CFS solution used to affect rice seed germination (El-Khawas and Adachi, 1999).
The study also observed effects, although smaller of the CFSs obtained from pH 7 and 8 on corn and tomato seed germination. This was not expected for CFS from pH 7, but provided insight regarding culturing microbes below or above their optimum pH, or perhaps at stressful levels of some other abiotic stress, to increase potential for benefiting plants, as was the case for seed germination. The most probable explanation for this situation could be that when a microbe is dealing with stress it excretes more material related to adaptation mechanisms (Decho and Gutierrez, 2017), perhaps in the form of beneficial microbe-to-plant signal compounds that increase seed germination rate.
Seedling Growth
Seedling growth, the earliest stage of actual plant establishment, needs to withstand the initial stresses the plant confronts as it grows. The use of products that enhance seedling growth are of great importance as they exert an ultimate effect on final crop performance/production. PGPM have shown to promote seedling growth from both their suspensions (Almaghrabi et al., 2014; Widnyana, 2018) and CFSs (Pellegrini et al., 2020) for both controlled and open field conditions. In the reported work we scanned seedling roots to determine their total length, volume, and average diameter (Tables 3–6) among seedling growth variables. This is the first report of the L. helveticus enhancing seedling growth directly, as opposed to the report by Rodríguez-Morgado et al. (2017), which stimulation of microbial activity in the soil and hence increased soluble phosphates, which increased plant root development. Furthermore, Lactobacillus rhamnosus CFS reported was analyzed whereby LLA was a metabolite responsible for improved soil properties (Rodríguez-Morgado et al., 2017) and LLA, peptides, and AA were responsible for increased microbial growth in the soil (Caballero et al., 2020). Whether the same metabolites are responsible for reported results in this study or not remains to be found out and further test under field conditions.
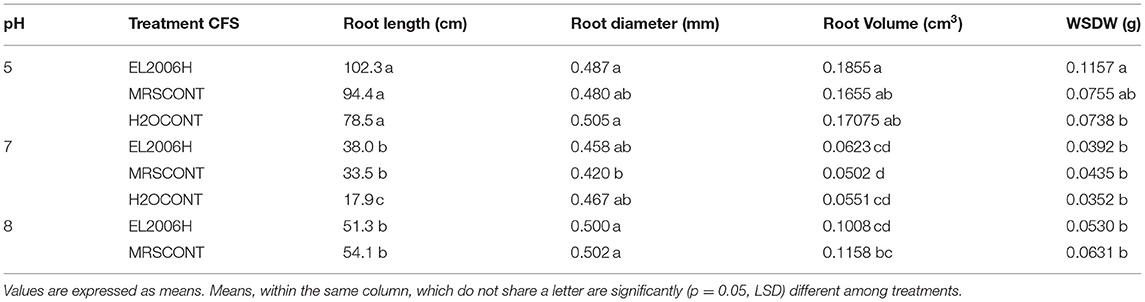
Table 6. Effect of Lactobacillus helveticus (EL2006H) CFS on tomato seedling growth variables at pH 5, 7, and 8 when grown in a growth chamber.
Visual inspection of the corn seedlings (Figure 4) indicated that 1% (v/v) CFS from B.s subtilis significantly increased root volume; these findings are consistent with a study on rice seedlings (El-Khawas and Adachi, 1999) which observed increased root length and volume following treatment with PGPM CFS. Moreover, Azospirillum brasilense Ab-V5 and Ab-V6 CFSs application yielded positive results in improving Glycine max root morphology and nodulation (Rondina et al., 2020).
Despite, the positive effects of the CFS on treated corn seedlings (Table 3), there was no statistically significant effect on WSDW, as compared to the positive controls. For tomato, the same treatment provided little to no effect. Most of the B. subtilis CFS seedling treatments did not significantly improve seedling variables regardless of pH level, the exception being root diameter which was increased at pH 8.
These interesting and in some cases apparently contradictory results provide a reason for investigating this hypothesis further under greenhouse conditions and even further under open field conditions. Currently, studies of CFS as biostimulants remain scarce for both greenhouse and open-field condition. Grain yield from Z. mays L. and G. max L. was enhanced by rhizobial CFS metabolites (LCOs, phytohormone and exopolysaccharides) by combined inoculation with Azospirillum sp. and Bradyrhizobium sp. (Marks et al., 2013). Similarly, one recent study by Tewari et al. (2020) has shown more interesting results under field condition that a combined formulation of Bradyrhizobium sp., its CFS and exopolysaccharides, which resulted in increased productivity and nodulation of pigeon peas as opposed to CFS or bacterium inoculum applied alone.
Conclusions
This study has provided new information on the use of CFSs from PGPM which have been cultured at a range of pHs: 5, 7, and 8. The results indicated both positive and negative effects. Specifically at higher pH the effect of CFSs were not greater than the positive controls for the pH for both germination and seedling growth variables. These findings, due to pH variation, which would affect the properties of these CFSs, or possibly others, requires further research to validate and expand on the discoveries reported here. It would also be of interest to study the chemical composition of the CFSs which caused positive effects. Microbial strains such as the L. helveticus used here are not well-characterized among the PGPM; expanding beyond commonly considered microbes would provide results allowing a broader understanding of bio-stimulation for plant growth associated with PGPM.
Data Availability Statement
The raw data supporting the conclusions of this article will be made available by the authors, without undue reservation.
Author Contributions
LM set up the experiment and wrote the manuscript. JN and MA helped with data collection and experimental set up. SS advised on scientific approach and provided background knowledge. DS provided funding, guided in scientific knowledge, provided the intellectual context, and extensive editorial input. All authors contributed to the article and approved the submitted version.
Funding
This work was funded through a grant from Consortium de recherche et innovations en bioprocédés industriels au Québec, number CRIBIQ 2017-034-C30.
Conflict of Interest
The authors declare that the research was conducted in the absence of any commercial or financial relationships that could be construed as a potential conflict of interest.
Publisher's Note
All claims expressed in this article are solely those of the authors and do not necessarily represent those of their affiliated organizations, or those of the publisher, the editors and the reviewers. Any product that may be evaluated in this article, or claim that may be made by its manufacturer, is not guaranteed or endorsed by the publisher.
References
Afzal, I., Rehman, H. U., Naveed, M., and Basra, S. M. A. (2016). Recent advances in Seed Enhancements. New Challenges in Seed Biology-Basic and Translational Research Driving Seed Technology, eds S. Araujo and A. Balestrazzi (London: Intechopen), 47–74.
Almaghrabi, O. A., Abdelmoneim, T., Albishri, H. M., and Moussa, T. (2014). Enhancement of maize growth using some plant growth promoting rhizobacteria (PGPR) under laboratory conditions. Life Sci. J. 11, 764–772. doi: 10.7537/marslsj111114.139
Antar, M., Gopal, P., Msimbira, L. A., Naamala, J., Nazari, M., Overbeek, W., et al. (2021). Inter-organismal Signaling in the Rhizosphere. Rhizosphere Biology: Interactions Between Microbes and Plants. Singapore: Springer.
Backer, R., Rokem, J. S., Ilangumaran, G., Lamont, J., Praslickova, D., Ricci, E., et al. (2018). Plant growth-promoting rhizobacteria: context, mechanisms of action, and roadmap to commercialization of biostimulants for sustainable agriculture. Front. Plant Sci. 9, 1473. doi: 10.3389/fpls.2018.01473
Buensanteai, N., Yuen, G. Y., and Prathuangwong, S. (2008). The biocontrol bacterium Bacillus amyloliquefaciens KPS46 produces auxin, surfactin and extracellular proteins for enhanced growth of soybean plant. Thai. J. Agric. Sci. 41, 101–116. Available online at: https://www.thaiscience.info/journals/Article/TJAS/10469537.pdf
Buensateai, N., Sompong, M., Thamnu, K., Athinuwat, D., Brauman, A., and Plassard, C. (2013). The plant growth promoting bacterium Bacillus sp. CaSUT007 produces phytohormone and extracellular proteins for enhanced growth of cassava. Afr. J. Microbiol. Res. 7, 4949–4954. doi: 10.5897/AJMR12.1839
Caballero, P., Rodríguez-Morgado, B., Macías, S., Tejada, M., and Parrado, J. (2020). Obtaining plant and soil biostimulants by waste whey fermentation. Waste Biomass Valoriz. 11, 3281–3292. doi: 10.1007/s12649-019-00660-7
Decho, A. W., and Gutierrez, T. (2017). Microbial extracellular polymeric substances (EPSs) in ocean systems. Front. Microbiol. 8, 922. doi: 10.3389/fmicb.2017.00922
Dimkpa, C. O., Merten, D., Svatos, A., Büchel, G., and Kothe, E. (2009). Metal-induced oxidative stress impacting plant growth in contaminated soil is alleviated by microbial siderophores. Soil Biol. Biochem. 41, 154–162. doi: 10.1016/j.soilbio.2008.10.010
El-Khawas, H., and Adachi, K. (1999). Identification and quantification of auxins in culture media of Azospirillum and Klebsiella and their effect on rice roots. Biol. Fertil. Soils 28, 377–381. doi: 10.1007/s003740050507
Gray, E., Lee, K., Souleimanov, A., Di Falco, M., Zhou, X., Ly, A., et al. (2006). A novel bacteriocin, thuricin 17, produced by plant growth promoting rhizobacteria strain Bacillus thuringiensis NEB17: isolation and classification. J. Appl. Microbiol. 100, 545–554. doi: 10.1111/j.1365-2672.2006.02822.x
Idris, E. E., Bochow, H., Ross, H., and Borriss, R. (2004). Use of Bacillus subtilis as biocontrol agent. VI. Phytohormonelike action of culture filtrates prepared from plant growth-promoting Bacillus amyloliquefaciens FZB24, FZB42, FZB45 and Bacillus subtilis FZB37/Nutzung von Bacillus subtilis als Mittel f?r den biologischen Pflanzenschutz. VI. Phytohormonartige Wirkung von Kulturfiltraten von pflanzenwachstumsfördernden Bacillus amyloliquefaciens FZB24, FZB42, FZB45 und Bacillus subtilis FZB37. Zeitschrift für Pflanzenkrankheiten und Pflanzenschutz/J. Plant Dise. Prot. 111, 583–597. Available online at: https://www.jstor.org/stable/43215615
Kalayu, G (2019). Phosphate solubilizing microorganisms: promising approach as biofertilizers. Int. J. Agron. 2019, 7. doi: 10.1155/2019/4917256
Kidaj, D., Wielbo, J., and Skorupska, A. (2012). Nod factors stimulate seed germination and promote growth and nodulation of pea and vetch under competitive conditions. Microbiol. Res. 167, 144–150. doi: 10.1016/j.micres.2011.06.001
Liu, J., Tang, L., Gao, H., Zhang, M., and Guo, C. (2019). Enhancement of alfalfa yield and quality by plant growth-promoting rhizobacteria under saline-alkali conditions. J. Sci. Food Agric. 99, 281–289. doi: 10.1002/jsfa.9185
Machado, R. M. A., and Serralheiro, R. P. (2017). Soil salinity: effect on vegetable crop growth. Management practices to prevent and mitigate soil salinization. Horticulturae 3, 30. doi: 10.3390/horticulturae3020030
Mahmood, I., Rizvi, R., Sumbul, A., and Ansari, R. A. (2019). Potential Role of Plant Growth Promoting Rhizobacteria in Alleviation of Biotic Stress. Plant Health Under Biotic Stress. Singapore: Springer.
Marks, B. B., Megías, M., Nogueira, M. A., and Hungria, M. (2013). Biotechnological potential of rhizobial metabolites to enhance the performance of Bradyrhizobium spp. and Azospirillum brasilense inoculants with soybean and maize. AMB Exp. 3, 1–10. doi: 10.1186/2191-0855-3-21
Meena, K. K., Kumar, M., Kalyuzhnaya, M. G., Yandigeri, M. S., Singh, D. P., Saxena, A. K., et al. (2012). Epiphytic pink-pigmented methylotrophic bacteria enhance germination and seedling growth of wheat (Triticum aestivum) by producing phytohormone. Antonie Van Leeuwenhoek. 101, 777–786. doi: 10.1007/s10482-011-9692-9
Mehmood, K., Li, J.-Y., Jiang, J., Masud, M., and Xu, R.-K. (2017). Effect of low energy-consuming biochars in combination with nitrate fertilizer on soil acidity amelioration and maize growth. J. Soils Sediments 17, 790–799. doi: 10.1007/s11368-015-1219-y
Molla, A. H., Shamsuddin, Z. H., and Saud, H. M. (2001). Mechanism of root growth and promotion of nodulation in vegetable soybean by Azospirillum brasilense. Commun. Soil Sci. Plant Anal. 32, 2177–2187. doi: 10.1081/CSS-120000276
Morel, M. A., Cagide, C., Minteguiaga, M. A., Dardanelli, M. S., and Castro-Sowinski, S. (2015). The pattern of secreted molecules during the co-inoculation of alfalfa plants with Sinorhizobium meliloti and Delftia sp. strain JD2: an interaction that improves plant yield. Mol. Plant-Microbe Interact. 28, 134–142. doi: 10.1094/MPMI-08-14-0229-R
Moretti, L. G., Crusciol, C. A., Kuramae, E. E., Bossolani, J. W., Moreira, A., Costa, N. R., et al. (2020). Effects of growth-promoting bacteria on soybean root activity, plant development, and yield. Agron. J. 112, 418–428. doi: 10.1002/agj2.20010
Msimbira, L. A., and Smith, D. L. (2020). The roles of plant growth promoting microbes in enhancing plant tolerance to acidity and alkalinity stresses. Front. Sustain. Food Syst. 4, 106. doi: 10.3389/fsufs.2020.00106
Ngoune Tandzi, L., Mutengwa, C. S., Ngonkeu, E. L. M., and Gracen, V. (2018). Breeding maize for tolerance to acidic soils: a review. Agronomy 8, 84. doi: 10.3390/agronomy8060084
Pandey, P., Bisht, S., Sood, A., Aeron, A., Sharma, G., and Maheshwari, D. (2012). Consortium of plant-growth-promoting bacteria: future perspective in agriculture. Bacteria Agrobiol. Plant Probiot. 185–200. doi: 10.1007/978-3-642-27515-9_10
Pellegrini, M., Pagnani, G., Bernardi, M., Mattedi, A., Spera, D. M., and Gallo, M. D. (2020). Cell-free supernatants of plant growth-promoting bacteria: a review of their use as biostimulant and microbial biocontrol agents in sustainable agriculture. Sustainability 12, 9917. doi: 10.3390/su12239917
Posada, L. F., Ramírez, M., Ochoa-Gómez, N., Cuellar-Gaviria, T. Z., Argel-Roldan, L. E., Ramírez, C. A., and Villegas-Escobar, V. (2016). Bioprospecting of aerobic endospore-forming bacteria with biotechnological potential for growth promotion of banana plants. Sci. Horticult. 212, 81–90. doi: 10.1016/j.scienta.2016.09.040
Rodríguez-Morgado, B., Jiménez, P., Moral, M., and Rubio, J. (2017). Effect of l-lactic acid from whey wastes on enzyme activities and bacterial diversity of soil. Biol. Fertility Soils 53, 389–396. doi: 10.1007/s00374-017-1187-z
Rondina, A. B. L., Dos Santos Sanzovo, A. W., Guimarães, G. S., Wendling, J. R., Nogueira, M. A., and Hungria, M. (2020). Changes in root morphological traits in soybean co-inoculated with Bradyrhizobium spp. and Azospirillum brasilense or treated with A. brasilense exudates. Biol. Fertility Soils 56, 537–549. doi: 10.1007/s00374-020-01453-0
Sapre, S., Gontia-Mishra, I., and Tiwari, S. (2018). Klebsiella sp. confers enhanced tolerance to salinity and plant growth promotion in oat seedlings (Avena sativa). Microbiol. Res. 206, 25–32. doi: 10.1016/j.micres.2017.09.009
Sashidhar, B., and Podile, A. R. (2010). Mineral phosphate solubilization by rhizosphere bacteria and scope for manipulation of the direct oxidation pathway involving glucose dehydrogenase. J. Appl. Microbiol. 109, 1–12. doi: 10.1111/j.1365-2672.2009.04654.x
Singh, K., Trivedi, P., Singh, G., Singh, B., and Patra, D. D. (2016). Effect of different leaf litters on carbon, nitrogen and microbial activities of sodic soils. Land Degrad. Dev. 27, 1215–1226. doi: 10.1002/ldr.2313
Smith, D. L., Subramanian, S., Lamont, J. R., and Bywater-Ekegärd, M. (2015). Signaling in the phytomicrobiome: breadth and potential. Front. Plant Sci. 6, 709. doi: 10.3389/fpls.2015.00709
Takishita, Y (2018). Pseudomonas entomophila 23S, a PGPR With Potential for Control of Bacterial Canker Disease in Tomato (Solanum lycopersicum L.) Against Clavibacter michiganensis subsp. Michiganensis. Montreal: McGill University.
Tallapragada, P., Dikshit, R., and Seshagiri, S. (2015). Isolation and optimization of IAA producing Burkholderia seminalis and its effect on seedlings of tomato. Songklanakar. J. Sci. Technol. 37, 553–59.
Tewari, S., Pooniya, V., and Sharma, S. (2020). Next generation bioformulation prepared by amalgamating Bradyrhizobium, cell free culture supernatant, and exopolysaccharides enhances the indigenous rhizospheric rhizobial population, nodulation, and productivity of pigeon pea. Appl. Soil Ecol. 147, 103363. doi: 10.1016/j.apsoil.2019.103363
Tian, Y., Guan, B., Zhou, D., Yu, J., Li, G., and Lou, Y. (2014). Responses of seed germination, seedling growth, and seed yield traits to seed pretreatment in maize (Zea mays L.). Sci. World J. 2014, 8. doi: 10.1155/2014/834630
Von Uexküll, H., and Mutert, E. (1995). Global extent, development and economic impact of acid soils. Plant Soil 171, 1–15. doi: 10.1007/BF00009558
Widnyana, I. K (2018). PGPR (Plant Growth Promoting Rizobacteria) Benefits in Spurring Germination, Growth and Increase the Yield of Tomato Plants. Recent Advances in Tomato Breeding and Production. London: IntechOpen.
Keywords: cell free supernatant, pH, germination, growth enhancement, plant growth promoting microbes
Citation: Msimbira LA, Naamala J, Antar M, Subramanian S and Smith DL (2022) Effect of Microbial Cell-Free Supernatants Extracted From a Range of pH Levels on Corn (Zea mays L.) and Tomato (Solanum lycopersicum L.) Seed Germination and Seedling Growth. Front. Sustain. Food Syst. 6:789335. doi: 10.3389/fsufs.2022.789335
Received: 04 October 2021; Accepted: 11 January 2022;
Published: 11 February 2022.
Edited by:
Pushp Sheel Shukla, Dalhousie University, CanadaReviewed by:
Durgesh Kumar Jaiswal, Savitribai Phule Pune University, IndiaBirinchi Kumar Sarma, Banaras Hindu University, India
Arup Ghosh, Council of Scientific and Industrial Research (CSIR), India
Copyright © 2022 Msimbira, Naamala, Antar, Subramanian and Smith. This is an open-access article distributed under the terms of the Creative Commons Attribution License (CC BY). The use, distribution or reproduction in other forums is permitted, provided the original author(s) and the copyright owner(s) are credited and that the original publication in this journal is cited, in accordance with accepted academic practice. No use, distribution or reproduction is permitted which does not comply with these terms.
*Correspondence: Donald L. Smith, ZG9uYWxkLnNtaXRoJiN4MDAwNDA7bWNnaWxsLmNh