- 1Departamento de Biología, Facultad de Ciencias, Universidad de La Serena, La Serena, Chile
- 2Departamento de Ingeniería Química, Facultad de Ingeniería y Ciencias Geológicas, Universidad Católica del Norte, Antofagasta, Chile
- 3Centro de Estudios Avanzados en Zonas Áridas (CEAZA), La Serena, Chile
- 4Instituto de Investigación Multidisciplinar en Ciencia y Tecnología, Universidad de la Serena, La Serena, Chile
- 5Nees Institute for Plant Biodiversity, Bonn University, Bonn, Germany
- 6Escuela de Ingeniería en Biotecnología Vegetal, Facultad de Ciencias Forestales, Universidad de Concepción, Concepción, Chile
- 7Centro de Investigación Tecnológica del Agua en el Desierto (CEITSAZA), Universidad Católica del Norte, Antofagasta, Chile
Salinity has extensive adverse effects on plant growth and the development of new agronomic strategies to improve crop salt tolerance is becoming necessary. Currently, the use of plant growth promoting rhizobacteria (PGPR) to mitigate abiotic stress in crops is of increasing interest. The most analyzed mechanism is based on ACC deaminase activity, an enzyme that decreases the ethylene synthesis, an important phytohormone in plant stress response. We aimed to identify other PGPR mediated mechanisms involved in the regulation of salt stress in plant. We used three PGPR strains (ESL001, ESL007, SH31), of which only ESL007 demonstrated ACC deaminase activity, to evaluate their effect on lettuce plants under salt stress (100 mM NaCl). We measured growth and biochemical parameters (e.g., proline content, lipid peroxidation and ROS degradation), as well as expression levels of genes involved in ethylene signaling (CTR1, EBF1) and transcription factors induced by ethylene (ERF5, ERF13). All bacterial strains enhanced growth on salt-stressed lettuce plants and modulated the proline levels. Strains ESL007 and SH31 triggered a higher catalase and ascorbate-peroxidase activity, compared to non-stressed plants. Differential expression of ethylene-related genes in inoculated plants subjected to salinity was observed. We gained consistent evidence for the existence of alternative mechanisms to ethylene modulation, which probably rely on bacterial IAA production and other chemical signals. These mechanisms modify the expression of genes associated with ethylene signaling and regulation, complementarily to the ACC deaminase model to diminish abiotic stress responses.
Introduction
In the last 25 years, soil salinity has increased faster due to inappropriate agricultural activities such as uncontrolled irrigation, low-quality irrigation water, urbanization, and climate change (Okur and Örçen, 2020). Thus, soil salinity has become a severe threat to agriculture, affecting up to 20 and 33% of the total cultivated and irrigated land worldwide, respectively (Goswami and Deka, 2020). Continuous exposure to salt stress conditions induces significant changes in physiological and morphological traits in plants. This finally leads to nutrient cytotoxicity and osmotic imbalances that negatively affect plant development, growth, and performance, resulting in a substantial reduction of crop productivity (Kaushal and Wani, 2016; Goswami and Deka, 2020).
Salt stress is composed of a rapid phase of osmotic stress (hours to days) followed by an ion toxicity stress (days to weeks) (Hasanuzzaman et al., 2021). During the osmotic stress, plants confront a trade-off between the acquisition of water and the risk of cell dehydration, which ultimately inhibits cell expansion and cell division (Munns and Tester, 2008). During the ion toxicity phase, the accumulation of reactive oxygen species (ROS) in the plant cell cytoplasm increases the risk of oxidative damage in cell and organelle membranes, and decreases the stability of proteins and nucleic acids (Vurukonda et al., 2016; Hasanuzzaman et al., 2021). Thus, different antioxidant defense systems are activated, which involve enzymes such as peroxidase (POX), glutathione reductase (GR), ascorbate peroxidase (APX), catalase (CAT), and superoxide dismutase (SOD). At the same time, the primary metabolism is modified and osmoprotectant as well as antioxidants [e.g., sugars (raffinose, sucrose and trehalose), sugar alcohols (sorbitol, mannitol), amino acids (proline) and amines (glycine, betaine and polyamines)] are accumulated to maintain the ionic balance in the cell (Taji et al., 2002; Ahn et al., 2007). The phytohormone ethylene plays a crucial role in stress response and tolerance in plants, increasing its synthesis in plants exposed to salinity and other stresses (Tao et al., 2015; Dubois et al., 2018). However, although ethylene could play a positive role in the early stage of self-adjustment or salt stress acclimation after this stage, an excessive ethylene concentration inhibits the plant development affecting its survival under salt conditions (Tao et al., 2015).
Research on plant growth-promoting rhizobacteria (PGPR) demonstrated that these beneficial microorganisms can enhance plant fitness and crop yield (Basu et al., 2021). Moreover, PGPRs are able to synthesize phytohormones (auxins, abscisic acid, gibberellins, cytokinin), and to stimulate plant endogenous hormone levels, activating the so-called induced systemic tolerance (IST) (Kumar et al., 2020; Hartmann et al., 2021). IST involves changes in physiological and biochemical processes of the plant, which mitigate the negative effects of environmental stresses, such as salinity and drought (Vurukonda et al., 2016; Kumar et al., 2020). The application of PGPR can also induce an enhancement of the antioxidant system to alleviate the deleterious effects of ROS (Vurukonda et al., 2016; Goswami and Deka, 2020). Nonetheless, the reduction of ethylene level of plants due to the hydrolysis of its immediate precursor ACC (1-aminocyclopropane-1-carboxylate) by the bacterial enzyme ACC deaminase is considered a key trait for stress mitigating PGPR (Glick et al., 1998; Gamalero and Glick, 2015). This so-called ACC deaminase mechanism leads to a downregulation of the ethylene-mediated signaling pathway, and thereby reduces the deleterious effect driven by an excess of the ethylene under severe stress conditions (Nascimento et al., 2018).
Up to now, bacterial-mediated hydrolysis of ACC is the only recognized mechanism by which PGPR can reduce ethylene levels in plants. It remains still unknown if other bacterial mechanisms could modulate plant ethylene synthesis, signaling and/or ethylene-induced gene expression, which would have a pivotal importance as knowledge for studies in stress tolerance in crops. Hence, analyzing the effect of bacterial inoculation in genes associated with ethylene signaling pathway such as Serine/threonine-protein kinase CTR1-like, Ethylene Insensitive protein 2/3, F-box protein 1/2-like (EBF1/2) or Ethylene Response genes (ERFs) (Zhang et al., 2016) could suggest a novel mechanism to decrease the plant stress using PGPRs. Our study hypothesized that PGPR can modulate the plant ethylene status not involving ACC degradation. To detect and elucidate these putative mechanisms, we use three PGPR strains, which differ in their ecological origin and in their ability to exert the ACC deaminase mechanism. We evaluated biochemical and molecular responses of plants subjected to saline conditions and bacterial inoculation, including the expression of genes involved in ethylene signaling and ethylene-induced genes. Finally, our results allow us to suggest a complementary strategy for ethylene signaling and regulation by PGPR, which do not possess the ACC deaminase mechanism.
Materials and Methods
Microorganisms
In this study, the bacterial strains ESL001, ESL007, and SH31 were used (Table 1). These strains were selected based on their origin (ecological context) and biochemical characterization; ESL001 and ESL007 were isolated from lettuce seeds, whereas SH31 was isolated from sediments of Salar de Huasco and displayed a natural resistance to the extreme salt environments (Remonsellez et al., 2018). To determine taxonomic classification, genomic DNA for each pure strain was isolated following the protocol of Yeates et al. (1998). Then, 16S RNA region was amplified using primer pair reported by Marchesi et al. (1998) and finally sequenced in Macrogen Inc. facilities, Seoul, Korea. Additionally, ESL007 possesses ACC deaminase activity, while ESL001 and SH31 do not (see section of PGPR traits and results).
Characterization of PGPR Traits
The three strains used in this study were characterized regarding their PGPR traits, following literature protocols as indicated: Quantification of solubilized inorganic phosphate expressed as the ratio of halo/colony on PVK agar plates (Vázquez et al., 2000; Zhu et al., 2011), IAA production (in μg/ml) in liquid culture (Glickmann and Dessaeux, 1995); Siderophores (CAS) expressed as the ratio of halo/colony on agar plates (Alexander and Zuberer, 1991); Nitrogen fixation capacity on agar plates (Baldani et al., 2014); Production of Exopolysaccharides (EPS, in μg CR/A600 nM) in liquid culture (Spiers et al., 2003).
Additionally, the tolerance to NaCl was determined for each selected strain by performing a bacterial growth curve according to Remonsellez et al. (2018) with modifications. Liquid LB medium supplemented with 0, 400, 800, and 1,200 mM NaCl, was used. The cultures were incubated at 25°C Erlenmeyer flasks on a shaker (160 rpm) for 72 h and optical density (OD) was measured at 600 nm every 12 h.
We assessed the presence and activity of ACC deaminase in the three strains. The presence of the ACC deaminase encoding gene was verified by employing the marker system and PCR conditions reported by Li et al. (2015). The quantification of the ACC deaminase activity followed the protocol of Gupta and Pandey (2019).
Finally, the capacity to promote plant growth was preliminarily evaluated in two in vitro assays with salt stress conditions: seedling growth promotion of Arabidopsis thaliana L. and germination of lettuce seeds. The methodological details are resumed in Supplement 1.
Salt Stress Assay With Lettuce
For this experiment, lettuce seedlings (20 days old; Lactuca sativa variety Corona) were obtained from the Servicio & Almácigos SA Nursery (Coquimbo Region, Chile). Seedling roots were carefully washed and inoculated with bacterial suspension with a final concentration of 107 CFU/ml [Optical Density (OD)600nm = 0.5] for 1 h. Inoculation treatments were: Control (without bacterial inoculation), ESL001, ESL007 and SH31. Two saline conditions were established in the assay: 0 and 100 mM NaCl. Inoculated and non-inoculated seedlings were transplanted to pots of 250 mL with a 2:1:1 substrate mixture (Cultivated vegetable field soil/ coarse sand/ peat) and grown for 24 days in the experimental greenhouse at Pan de Azúcar (Coquimbo Region, Chile). During the experiment, the mean temperature was 19°C and natural photoperiod was 12 h/12 h approx. Each treatment consisted in 15 independent replicates.
The pots were arranged as randomized blocks and first subjected to a 7-days adaptation period with regular irrigation. At day 8, a second application of each bacterial treatment was performed, consisting of 5 mL of bacterial suspension with a final concentration of 107 CFU/ml (OD600nm = 0.5), in Control treatments 5 mL water were added. On the 9th day post-transplant, the salt stress period was started by applying 4 accumulative saline irrigations until reaching a NaCl concentration of 100 mM. This gradual acclimation of seedlings to salinity conditions reduces the sudden death of plants at high salt concentrations (Xu and Mou, 2015). The electrical conductivity (EC) of each pot was measured using the ProCheck sensor Teros 12 (Decagon Devices Inc.), to assure homogeneity of saline conditions. Average electrical conductivity (EC) of the substrate of plants grown under salt conditions varied between 2.74 and 3.09 dS/m, whereas a control value of 1.36 dS/m was determined in the absence of salt treatment. Achieved soil salt concentration is equivalent to moderate salinity in lettuce agriculture production.
Plant Growth and Biochemical Parameters
After 24 days, the following growth parameters were measured in each lettuce plant: shoot growth (expressed as Δheight = final height–initial height), root length, fresh weight and, dry weight were evaluated for roots and shoots (aerial biomass). Dry weight values were used to calculate the root/shoot ratio (dry weight root/dry weight leaves) (Garcia et al., 2019). Additionally, plant tissue was collected and stored at −80°C until physiological, enzymatic and molecular analyses.
As biochemical parameters, the total chlorophyll content, chlorophyll a, chlorophyll b, and total carotenoids were determined according to the protocol of Porra et al. (1989). Total proline content was measured according to Bates et al. (1973) and Rivero et al. (2004). Lipid peroxidation in plant tissues was used as a physiological indicator of oxidative stress. Lipid peroxidation was assessed by the thiobarbituric acid method as described in Ortega-Villasante et al. (2005). This test determines the amount of malondialdehyde (MDA), a by-product of lipid peroxidation that reacts with thiobarbituric acid. Ground frozen tissue (0.1–0.2 g) was homogenized following addition of 1 mL of TCA–TBA–HCl reagent [15% (w/v) trichloroacetic acid (TCA), 0.37% (w/v) 2-thiobarbituric acid (TBA), 0.25 M HCl, and 0.01% butylated hydroxytoluene]. After homogenization, samples were incubated at 90°C for 30 min in a hot block, then chilled in ice and kept in darkness for 5 min, and then centrifuged at 12,000 g for 10 min. The resulting chromophore absorbs at 535 nm, which was evaluated using Infinite 200M NanoQuant Microplate Readers from Tecan®. Values corresponding to non-specific absorption at 600 nm were subtracted. MDA concentration was calculated directly from the extinction coefficient ε = 156 mM−1 cm−1.
Stress-Related Enzyme Activity
Under salt stress conditions, antioxidant systems keep the reactive oxygen species (ROS) at low levels. To identify if the application of PGPRs stimulates antioxidant enzymatic mechanisms, the activity of the antioxidant enzymes superoxide dismutase (SOD), catalase (CAT), and ascorbate peroxidase (APX) were evaluated. Plant tissues were prepared according to Zhu et al. (2004). Briefly, fresh leaves (1 g) were ground into a prechilled mortar with liquid nitrogen and homogenized with 2 mL of sodium phosphate buffer (2% PVP w/v, 0.05M Na2HPO4, 1 mM EDTA, pH 7.0). The homogenate was centrifuged (13,000 g, 4°C, 20 min), and the supernatant was used for spectrophotometric determinations of enzyme activity and total protein content.
Superoxide dismutase (SOD; EC 1.15.1.1) activity was determined by the method described by Giannopolitis and Ries (1977). The reaction mixture was composed of 1.3 mM riboflavin, 13 mM methionine, 63 μM NBT, 0.05M sodium carbonate (pH 10.2) and 100 μL of plant extract. Distilled H2O was added to bring to the final volume of 500 μL. One unit of enzyme activity was defined as the amount of enzyme required to result in a 50% inhibition of the rate of nitro blue tetrazolium reduction, measured at 560 nm.
Catalase (CAT; EC 1.11.1.6) activity was assayed by measuring the initial disappearance of H2O2 at 240 nm (Aebi, 1984) every 30 s at 25°C. The volume of the reaction mixture was 0.5 mL and included 175 μL of leaf extract, 200 μL of 25 mM H2O2, and 125 μL of 100 mM sodium phosphate buffer (pH 7.0).
Ascorbate peroxidase (APX; EC 1.11.1.11) activity was determined by monitoring the decrease of ascorbate absorbance at 290 nm (Nakano and Asada, 1981) at intervals of 30 s at 25°C. The reaction mix contained 250 μL of leaf extract, 0.25 mM ascorbate, 0.1 mM EDTA and 1 mM H2O2 in 25 mM sodium phosphate buffer (pH 7.0), and the final volume of the reaction was 500 μL.
Protein content was measured according to the method of Bradford, using bovine serum albumin as standard, at 595 nm (Bradford, 1976). All spectrophotometric measures were performed by using a NanoQuant Infinite m200 Pro Multimode Microplate Reader (Tecan).
RNA Isolation, cDNA Synthesis, and Real-Time PCR
Total RNA was isolated from leaves of L. sativa plants using Trizol® Reagent (Ambion, Life Technologies) according to manufacturer's instructions. The RNA obtained was treated with RNase-free DNase I (Qiagen) and quantified by absorbance using the NanoQuant Infinite m200 Pro (Tecan). RNA intactness was verified by visual inspection of the integrity of 28S and 18S rRNA bands in denaturing formaldehyde/agarose gel electrophoresis. RNA was stored at −80°C for further use.
RNA (1 μg) was reverse-transcribed into single-stranded cDNA templates using the PrimeScript™ RT reagent Kit (TAKARA) and oligo-p(dT)15 primer, according to the manufacturer's instructions. Each qPCR reaction consisted of 8.5 μL of the Takyon ™ Rox SYBR® MasterMix dTTP Blue kit (Eurogentec), 50 ng cDNA and 0.45 μM (final concentration) of each primer, in a final volume of 10 μL. To determine the effect of PGPR bacteria on the ethylene signaling pathway, the transcript levels of the following genes were evaluated by Real-Time qPCR: serine/threonine-protein kinase CTR1-like gene, EIN3-binding F-box protein 1-like (EBF1) gene and the ethylene-responsive transcription factor (ERF), ERF5 and ERF13. At the same time, the elongation Factor-1α (EF-1α) was used as an endogenous control in order to normalize experimental results. Gene-specific primer sequences and gene identification are described in Table 2. The following qPCR reaction protocol was employed, using the Agilent Mx3000P QPCR System (Agilent Technologies): initial denaturation time was 3 min at 95°C, followed by 40 PCR cycles (30 s at 95°C, 18 s at 60°C and 2 s at 60°C). After the PCR cycles, the purity of the PCR products was checked by analysis of the corresponding melting curve. The relative abundance of transcripts was quantified by applying the comparative CT method (also called the ΔΔCT method) or the standard curve method, depending on the efficiency of amplification of each gene with respect to the endogenous control (Livak and Schmittgen, 2001). Three biological replicates were used for the qPCR analysis, being used three technical replicates for each biological sample. The technical replicates were averaged for each biological replicate before the statistical analysis.

Table 2. Identification of L. sativa gene homologs related with ethylene signaling, and primers used in qPCR experiments.
Statistical Analysis
Fifteen independent replicates were analyzed for morphological parameters. From the obtained data, outliers values were removed. To identify the most relevant physiological and morphological parameters, an Analysis of Principal Component (PCA) was performed using R project software.
On the other hand, all spectrophotometric measures were performed by using three biological replicates, and two technical replicates, for each analysis. At the same time, three biological replicates were used for qPCR analysis, considering 3 technical replicates each one. Data from enzyme activities and gene expression were analyzed using One-way ANOVA test from R project software. Tukey post-hoc comparison was used when significant differences (p < 0.05) were found in each analysis.
Results
Characterization of PGPR Traits
The three strains in this study presented similar results for most of the evaluated PGPR traits (Table 3). All strains produced the phytohormone IAA in comparable range (60.9–65.9 μg/ml), were able to exude siderophores, fix nitrogen and to produce EPS. The strains ESL001 and ESL007 presented a measurable halo as an indicator of inorganic phosphate solubilization on agar plates. Their production of siderophores was also higher than for strain SH31. Growth in NaCl-enriched liquid LB medium was similar for the three strains at 0 and 400 mM, but only the extremophile SH31 grew at 1,200 mM.
Regarding to the ACC deaminase, the gene acdS was detected only in the ESL007 strain and the enzymatic activity was quantified only in this bacteria (Table 3).
During the in vitro assays, all strains promoted the plant growth compared to the Control treatment (Supplement 1 and Supplementary Figures 1–3). In the Arabidopsis experiment, ESL007 and SH31 without salt stress (0 mM NaCl) significantly (p < 0.001) increased rootw length and lateral root number compared to the Control (Supplementary Figures 1, 2). Whereas, under salt stress conditions, ESL001 and ESL007 improved significantly (p < 0.001) the root architecture vs. the Control, while SH31 did not enhance root growth.
The germination of lettuce seeds in the Control treatment decreased noticeably from >95% at 0 mM and 50 mM NaCl to <30% at 100 and 150 mM (Supplementary Figure 3). In contrast, all seeds with bacterial inoculation maintained high germination rates of >95% in the 100 mM salt treatment. Additionally, SH31 and ESL007 enabled a germination rate of >80% despite the salt concentration of 150 mM (Supplementary Figure 3). Seeds inoculated with ESL001 reached a germination rate of 50% at 150 mM salt concentration. This value is still five times higher than the germination rate of the Control seeds.
Salt Stress Assay With Lettuce
The Principal Component Analysis (PCA) showed differences in physiological and biochemical parameters were associated with the treatments of 0 and 100 mM of NaCl (Figure 1). Fresh and dry shoot weight, root and shoot length, total chlorophyll, and total carotenoids were related to growth promotion without salt stress (0 mM NaCl); chlorophyll b, dry weight root and proline content were related to the effect of the salt stress (100 mM NaCl). In root dry weight, statistical differences were observed in response to bacterial treatment (p < 0.01), and their interaction with salt treatment (p < 0.001), but not in response to the salt treatment alone (n.s.). No statistical differences were observed in root dry weight in response to the bacterial treatment (0 mM NaCl). However, under salt stress, significant differences were observed among Control vs. the strains SH31 and ESL007, where this parameter increased between 40 and 90% under stress conditions, respectively (Figure 2A). In addition, salt stress reduced the root dry weight in the stressed Control by 58%, whereas the loss of root biomass for all bacterial treatments was significantly less. The proline content changed significantly in response to bacterial treatment (p < 0.01) and salt stress (p < 0.001), as well as their interaction (p < 0.001) was significantly higher for salt stress Control plants than non-stressed Control (0 mM NaCl). Each bacterial treatment caused a different plant response: ESL001 significantly enhanced the proline content only without salt stress; ESL007 significantly increased the proline concentration only under salt stress; SH31 raised the proline concentration independently from the salt condition. The latter performed best, increasing this metabolite by 44 and 34%, without and with salt stress, respectively (Figure 2B).
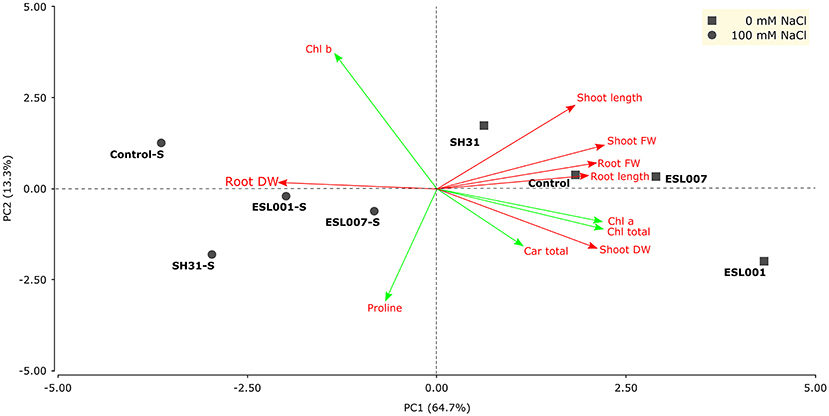
Figure 1. Principal component Analyses (PCA) of bacterial treatments based on growth and biochemical parameters evaluated in the lettuce pot experiment under salt conditions (100 mM NaCl in the soil). Vector Colors: red—growth parameters, green—biochemical parameters; Symbols: square—Control condition (0 mM NaCl), circle—salt condition (100 mM NaCl). Car, content of carotenoids; Chl, content of chlorophyll (a, b or total as indicated in the figure); DW, dry weight; FW, fresh weight.
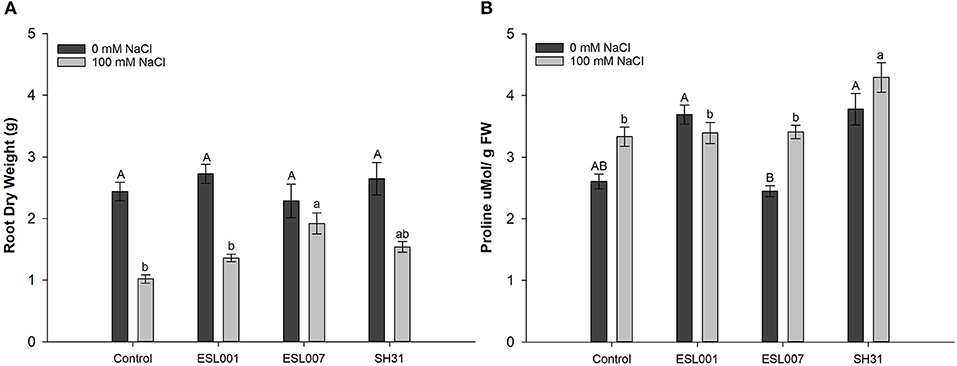
Figure 2. Effect of bacterial treatments on root dry weight (A) and proline content (B) in the lettuce pot experiment under salt stress (0 and 100 mM NaCl in soil). (a) Root dry weight, (b) Proline content. Data are presented as means of 15 individuals per treatment with standard errors. Letter(s) indicate statistical significance according to ANOVA at p < 0.05.
Antioxidant Responses to Salt Stress of Lettuce With and Without PGPRs
Lettuce plants treated with bacterial strains showed differential responses to oxidative stress and the antioxidant activity (Figure 3). Specifically, the levels of malondialdehyde (MDA), as a proxy of oxidative damage in lipid membranes, were significantly higher in salt stress treatments compared with the non-stressed Control (p < 0.01) (Figure 3A). Among the salt stress treatments, no statistical difference was detected. Nonetheless, a tendency is observed for plants inoculated with strains ESL001 and SH31, as their MDA level also demonstrated no statistical difference from the non-stress Control. On the other hand, no significant change of the SOD activity was detected under salt stress (Figure 3B). However, CAT and APX activities changed in response to stress and bacterial inoculation (p < 0.01 and p <0.001, respectively). Particularly, CAT activity increased 4- and 2-fold in ESL007 and SH31 inoculated plants, respectively, whereas APX activity increased 6- and 2-fold in ESL007 and SH31 inoculated plants, respectively, compared with non-stressed Control (Figures 3C,D).
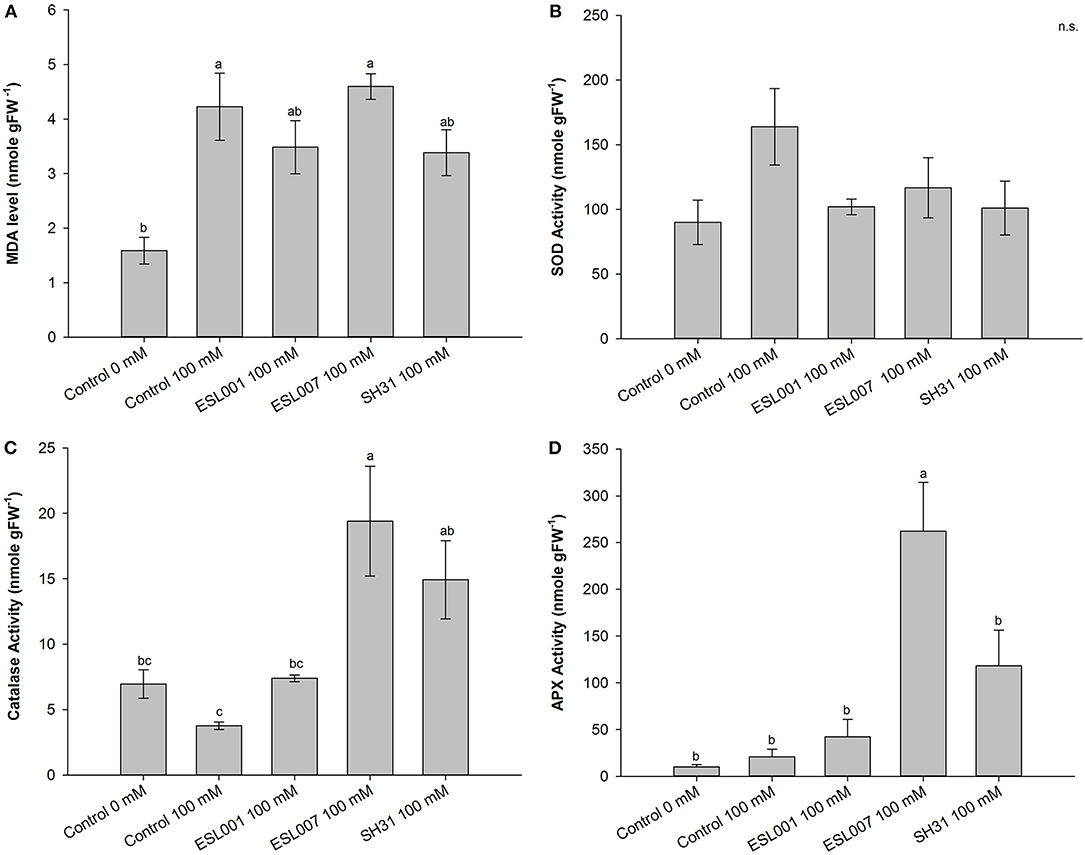
Figure 3. Effects of ESL007, SH31 and ESL001 strain inoculation on the level of (A) MDA and in the activities of the antioxidant enzymes (B) superoxide dismutase (SOD), (C) catalase (CAT), and (D) ascorbate peroxidase (APX) in lettuce. Different letters indicate statistical differences at p < 0.05.
Transcriptional Analysis of Genes Involved in Ethylene Signaling Pathway and Ethylene-Induced Gene Expression
Under salt stress (100 mM NaCl), a significant increase of CTR1 transcription was observed in the stressed Control. At the same time, all bacterial treatments showed a low expression profile, similar to the non-stressed Control (Figure 4A). On the other hand, EBF1 transcription levels differed between treatments (p < 0.001). The relative expression of EBF1 was significantly down-regulated in treatments inoculated with either ESL007 or SH31 under salt stress (Figure 4B).
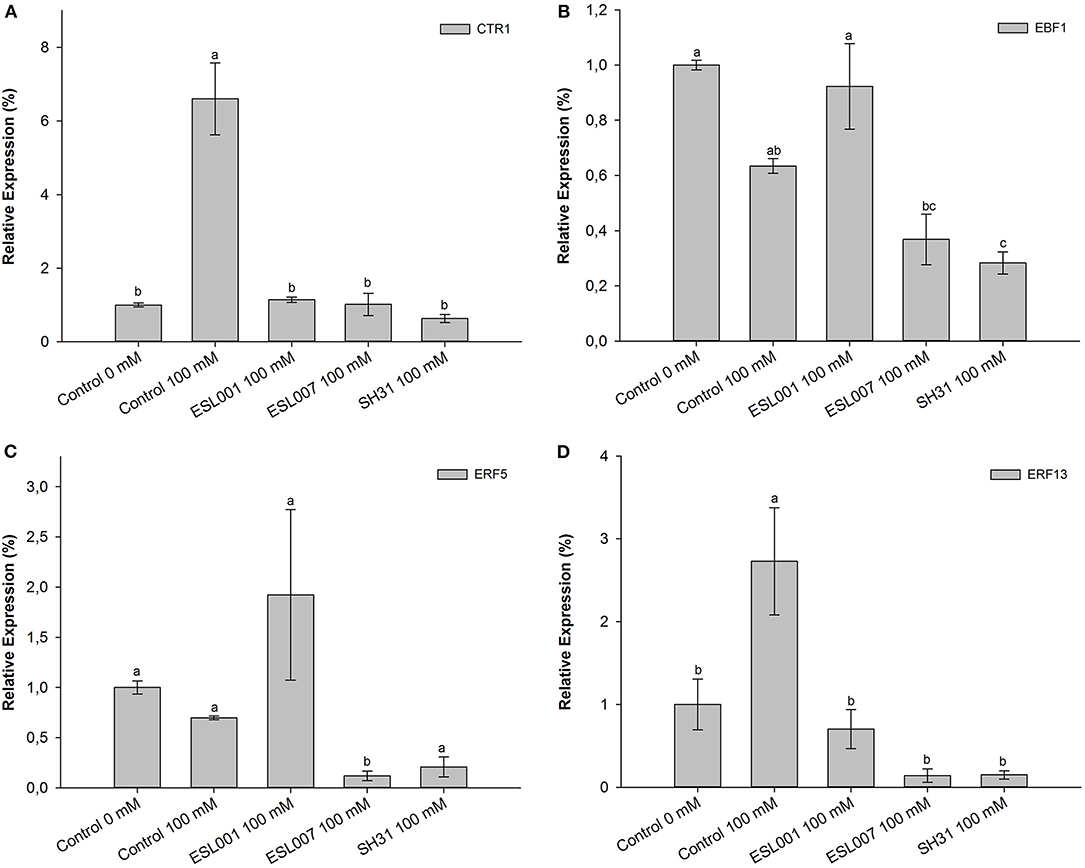
Figure 4. Comparative expression analysis of L. sativa gene homologs involved in ethylene signaling and ethylene-responsive genes. Samples were obtained from leaves of non-inoculated, non-stressed plants (Control 0 mM NaCl), non-inoculated plants subjected to 100 mM NaCl (Control 100 mM NaCl), and salt stressed plants previously inoculated by three different bacterial strains (ESL001 100 mM, ESL007 100 mM and SH31 100 mM NaCl). Relative mRNA levels of CTR1 (A), EBF1 (B), ERF5 (C), and ERF13 (D) gene homologs were quantified by real-time PCR. Elongation Factor 1α was used as endogenous gene. Results represent the mean value ± standard error (SE). Different letters denote significant statistical differences (p < 0.05). Data are the means of three biological replicates.
Finally, two ethylene response genes were evaluated. In the case of ERF5, no significant changes in gene expression in plants subjected to most treatments were observed, except ESL007. Under salt stress, the treatment with ESL007 displayed a significant down-regulation of ERF5 (p < 0.001) (Figure 4C). In the case of ERF13, transcripts were significantly upregulated in the salt-stressed Control compared to the non-stressed Control (p < 0.001) (Figure 4D). In contrast, such an up-regulation of ERF13 expression was not observed in the bacterial treatments (ESL001, ESL007, SH31) under salt stress. Meanwhile, ESL001 maintained an expression level comparable to the non-stressed Control, ESL007 and SH31 showed a tendency to down-regulate ERF13 expression (Figure 4D).
Discussion
The use of beneficial microorganisms to mitigate abiotic stress in crops is of increasing interest (Goswami and Deka, 2020; Kumar et al., 2020; Hartmann et al., 2021). In this context, ACC deaminase activity (acdS gene) is probably the most accepted and studied mechanism found in microorganisms to reduce the abiotic stress in plants. In those studies, the discussions are generally focused on ethylene synthesis and ACC degradation, while other microbial mechanisms to ameliorate stress are paid less attention. Our study aimed to elucidate biochemical and molecular responses of plants subjected to salt stress and bacterial inoculation, which are modulated independently from the ACC deaminase model (Glick et al., 1998). Therefore, we used three bacterial strains (ESL001, ESL007, SH31), of which only one possess ACC deaminase activity (ESL007).
The in vitro assay with A. thaliana adds a first important evidence, as the experimental design prevents physical contact between the seedlings and each strain. This setting excludes the colonization of the root surface by the PGPR strain, which is an initial condition for the ACC deaminase model (Glick et al., 1998). Thus, the improved A. thaliana growth under salt stress registered for all strains in this assay cannot be attributed to ACC deaminase activity and other mechanisms must act.
To guide our discussion, we summarized our findings in a schematic representation of the integrated regulation of the ethylene signaling pathway upon PGPR-plant interaction and its effects on plant homeostasis and growth (Figure 5).
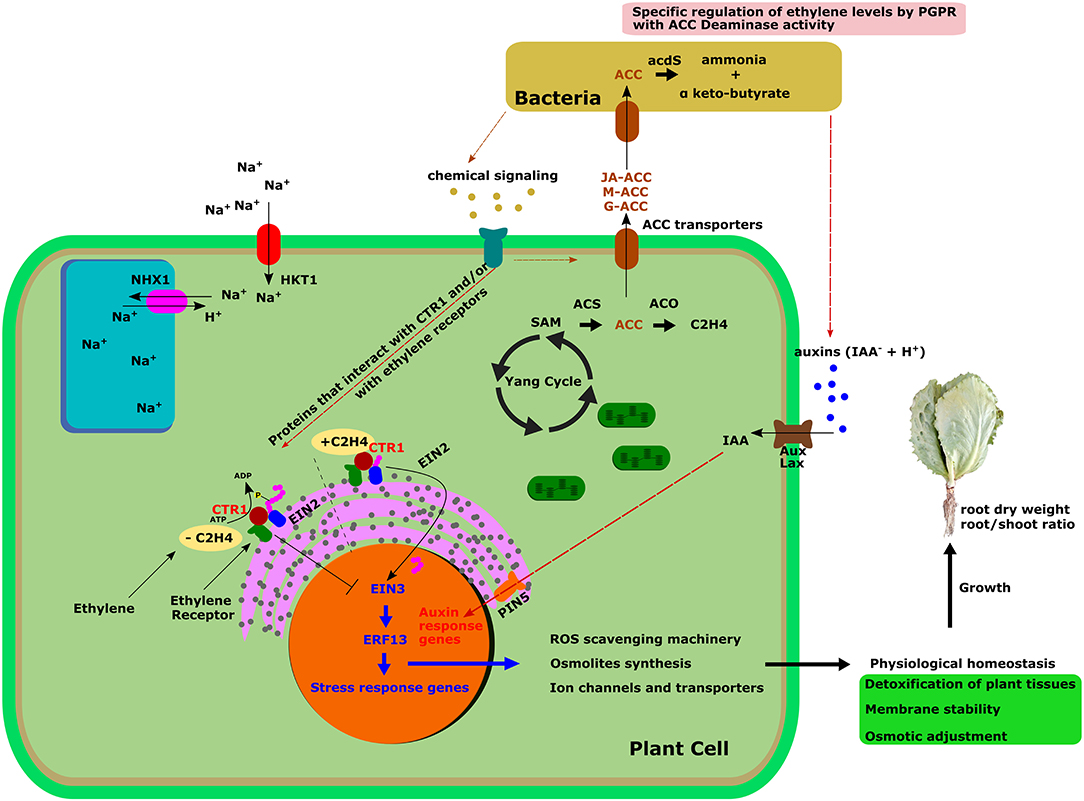
Figure 5. Graphical synthesis for integrated regulation of the ethylene signaling pathway upon PGPR-plant interaction, and its effects on plant homeostasis and growth. Ethylene signaling pathway is directly affected by inoculation with bacteria, which establishes a chemical communication with the plant cell. Specific molecules secreted by bacteria would bind plant receptors, inducing activation or synthesis of plant molecules which in turn would induce changes in the expression of genes involved in ethylene signaling. By this way, bacteria would up- and down-regulate key genes involved in the ethylene response, inducing a better response of the plant to salt stress, in comparison with Control plants, as reflected in an enhanced membrane stability (evaluated as lipid peroxidation), proline content and plant biomass. Brown colored pathway represents the established model of ethylene synthesis modulation by plant-bacteria interaction, postulated by Glick et al. (1998), based on degradation of ethylene precursor ACC by bacterial ACC deaminase activity. ACC, 1-aminocyclopropane-1-carboxylate; acdS, ACC deaminase; M-ACC, Malonyl-ACC; G-ACC, γ-glutamyl-ACC; JA-ACC, Jasmonoyl-ACC; NHX1, Vacuolar Na+/H+ antiporter; HKT1, High affinity K+ transporter; SAM, S-adenosyl-L-methionine; ACS, ACC-synthase; ACO, ACC-oxidase; C2H4, Ethylene; CTR1, Constitutive Triple Response 1; EIN2, Ethylene-Insensitive 2; EIN3, Ethylene-Insensitive 3; EBF1, EIN3-binding F-box protein 1-like; ERF13, Ethylene-Responsive transcription Factor 13; ROS, Reactive Oxygen Species; AUX/LAX, Influx carriers AUXIN RESISTANT and LIKE AUX; PIN5, Efflux carrier PIN-FORMED-5.
Molecular Regulation of Ethylene Signaling Pathway
Based on the ACC deaminase model, genes involved in the ethylene signaling pathway (CTR1, EBF1) and Ethylene Response Factors (ERFs) were evaluated in response to salt stress and bacterial inoculation. CTR1: In the absence of ethylene, CTR1 represses the Ethylene-insensitive protein 2 (EIN2), a positive regulator of ethylene responses that act downstream of CTR1. When ethylene targets ethylene receptors, CTR1 inhibition of EIN2 is relieved (Dubois et al., 2018). Similarly, to Peng et al. (2014), in our lettuce experiment, the salt stressed Control presented a significant increase in CTR1 transcription compared to the non-stressed Control, which might be related to the crosstalk with other hormones. Some authors reported that the levels of membrane-associated CTR1 protein vary in response to ethylene, doing so in a post-transcriptional manner that correlates with the ethylene-mediated changes in levels of the receptors (Shakeel et al., 2015). Interestingly, all bacterial treatments maintained the CTR1 transcript levels similar to that observed in the non-stressed Control. The plant physiological response to the bacterial treatments under salt stress is comparable to Vaseva et al. (2021a,b), despite of obtaining a CTR1 gene expression profile contrary to the ctr1-1 mutant. In addition, the evaluated PGPRs could be complementing other traits of the ctr1-1 mutant, such as auxin biosynthesis through bacterial IAA or other undescribed bacterial mechanisms, that would be involved in improve plant tolerance.
EBF1: EBF1 is a specific F-box proteins, which are components of the SCF ubiquitin E3 ligase complexes. EBF1 regulates EIN2 and EIN3, which are positive regulators of the ethylene signaling pathway, and both proteins accumulate in presence of ethylene (Stepanova and Alonso, 2009; Dubois et al., 2018). In the absence of ethylene, the F-box proteins EBF1 and EBF2 (for EIN3-Binding F-box protein 1 and 2) directly interact with EIN3, targeting this protein for degradation in the proteasome (Potuschak et al., 2003). It was also observed that EBF1 overexpression induced ethylene insensitivity and decreased levels of EIN3 protein (Potuschak et al., 2003). In our experiments, expression of an EBF1 homolog was down-regulated in salt-stressed lettuce inoculated with strains ESL007 or SH31, compared to non-stressed Control or with plants inoculated ESL001. These results suggest that inoculation with ESL007 or SH31 induced a modification of ethylene levels or signaling in salt-stressed plants, causing a decrease in EBF1 transcript levels and then, less degradation of EIN3. This could enhance ethylene signaling and expression of downstream ethylene-responsive genes to cope with salt stress by activating the response ethylene mechanisms without reaching detrimental response levels.
ERF5 and ERF13: Through its signaling pathway, ethylene induces the expression of numerous secondary transcription factors, as the Ethylene Responsive Factors (ERFs). Several ERFs are involved in abiotic stress response and tolerance (Licausi et al., 2013). In A. thaliana, ERF5 is induced by ethylene in an EIN2-dependent manner (Tao et al., 2015). This transcription factor has a role in the adaptation to drought and salt tolerance in tomato (Pan et al., 2012). Together with ERF6, it is involved in regulation of leaf growth under water-limiting conditions (Dubois et al., 2013). However, we observed that in lettuce ERF5 was not significantly upregulated neither in unstressed nor in salt-stressed plants. Probably, this transcription factor is not a salt stress-responsive gene in lettuce, but might have other functions, linked to e.g., the plant defense response to microbial pathogens (Moffat et al., 2012; Son et al., 2012).
On the other hand, our results showed a significant upregulation of ERF13 only in the salt stressed Control, suggesting that the inoculation with any of the three bacterial strains induced a change in the ethylene status, signaling, and in ethylene-mediated responses in salt-stressed plants. Despite the fact that the function of ERF13 has not been completely clarified, Chia (2013) suggested that ERF13 is an enhancer of ABA response, while Lee et al. (2010) reported a linkage between over-expression of ERF13 and COR15A (Cold-Related 15A gene).
Involvement of Exogenous Indole-3-Acetic Acid (IAA)
All three ewvaluated PGPRs produce Indole-3-acetic acid (IAA), which could contribute to ameliorate the salt stress through a different mechanism than ACC deaminase activity. According to Vaseva et al. (2021a), ctr1-1 Arabidopsis mutant can tolerate high salinity, which could be related to the altered ethylene/auxin regulatory loop. Due to the ctr1 mutation, ethylene signaling is constantly activated and also the auxin biosynthesis is increased. Under salt stress (100 mM NaCl), the ctr1-1 mutant significantly induced efflux (PIN1) and influx carriers (AUX1 and LAX3) in roots, suggesting a predisposition for their better performance on challenging substrates (Vaseva et al., 2021a). Also, the applications of exogenous IAA increased the salt tolerance in different commercial crops (Ribba et al., 2020). In strawberry (Fragaria × ananassa Duch. cv. Akihime) and cereals, auxin treatments enhanced the photosynthetic capacity, nutrient uptake and reduced the water loss under salt conditions (Zhang et al., 2021; Kosakivska et al., 2022), while in potato (Solanum tuberosum) promoted growth and increased the CAT activity (Khalid and Aftab, 2020). Similarly, Abdel-Latef et al. (2021) observed that exogenous auxins inhibited the excessive accumulation of Na+ in the roots of Vicia faba, enhanced the osmolyte accumulation and the antioxidant activity of SOD, CAT, and APX under salt stress. Thus, IAA produced by PGPR strains could activate plant antioxidant mechanisms and reduce salt stress without the participation of ACC deaminase.
Proline (Osmolyte) Synthesis
Proline accumulation under abiotic stress has often been described to be a compatible solute in osmotic adjustment, a free radical scavenger, stabilizer for membranes and activator of detoxification pathways (Hossain et al., 2014). Our results showed that the bacterial treatments enhanced proline accumulation in all treatments, even in Control conditions (0 mM NaCl). Therefore, proline accumulation could be a priming effect (Sher et al., 2019), where the proline synthesis and its accumulation could be a predisposition to increase tolerance by prior activation of the abiotic stress defense systems. However, another defense mechanism has been also described by Vaseva et al. (2021b). These authors identified an upregulation of the enzyme Delta-1-Pyrroline-5-Carboxylate Synthase (P5CS, a key step in the proline biosynthetic pathway) in the mutant ctr1-1. This overexpression increased the expression of the Na+/H+ antiporter, reducing the Na+ content and the ion toxicity in the plant tissues (Vaseva et al., 2021b). Thus, PGPR strains could induce salt stress tolerance through the induction of proline synthesis and accumulation, despite of the absence of ACC deaminase activity.
ROS Scavenging Machinery
In lettuce under salt stress, the application of Pseudomonas sp. (strain with ACC deaminase activity) influenced the Na+/K+ ratio and reduced the ion toxicity of salt stress, improving the membrane stability index (MSI) and plant growth (Azarmi-Atajan and Sayyari-Zohan, 2020). In our work, MDA analysis let us evaluate the negative effect of ROS on lipid membranes (Ortega-Villasante et al., 2005; Rawat et al., 2021). Treatment with the strains ESL001 and SH31, led to increased root dry weight and a tendency to reduce the negative effect of ROS production on lipid membranes despite of not possessing the ACC deaminase mechanism. The MDA results can be explained by the proline accumulation profiles identified on treated plants.
Along with osmotic and ionic stress, another detrimental effect of salinity for plant growth is the induction of a burst of reactive oxygen species (ROS), generating oxidative stress (Abdel-Latef et al., 2021; Vaseva et al., 2021b). In this work, all strains induced enzymes associated with the ROS detoxification system. Enzymes such as SOD and CAT catalyze ROS degradation, while enzymes of the ascorbate-glutathione cycle such as APX regenerate soluble antioxidants (Gill and Tuteja, 2010). We found that all treatments displayed similar SOD activity values, suggesting a non-significant O scavenging and dismutating capacity of lettuce under the salt stress conditions. Nevertheless, a significant induction of the antioxidant activity of CAT and APX was observed in plants inoculated with ESL007 (ACC deaminase activity) and strain SH31. Both enzymes are involved in scavenging hydrogen peroxide (H2O2), being a highly energy-efficient mechanism to remove H2O2. the activities of these two enzymes were similar in both non-stressed and salt-stressed Control, suggesting a signaling role of H2O2 when plants were interacting with SH31 and ESL007 strains, but not with ESL001. This may be explained by different mechanisms and pathways, such as ACC deaminase or induced systemic resistance, induced by different bacterial strains (Fan et al., 2020). Interestingly, other authors the current literature reported contrasting results about the effects of PGPR on the activity of these two enzymes (Han and Lee, 2005; Kohler et al., 2010; Jha and Subramanian, 2013), suggesting that this effect might be an interplay of several factors like the type, severity and duration of the abiotic stress, the traits of the applied PGPR strain (ACC deaminase activity, auxin production, among others) and its interactions with the plants.
Conclusions
Our results provide a new insight into plant-bacteria interactions, highlighting the involvement of ethylene-regulated mechanisms in the induction of salt stress tolerance (Figure 5), unlike most other PGPR-related studies performed in this context. The current model by which PGPR bacteria mediate stress response via a reduction in ethylene production is based on the bacterial ACC deaminase enzyme, which cleavage of ACC to ammonia and α- ketobutyrate. However, the results of our work suggest the existence of different strategies, according to which the plant could repress its ethylene signaling pathway in response to bacteria. However, more research is needed to identify the bacterial-induced mechanisms involved in the regulation of ethylene signaling and response during salt stress. A deeper understanding of the mechanisms underlying the ethylene response during plant-bacteria interactions would help to identify new strains which could have a novel and more efficient mechanisms than those described until now to reduce the negative effect of the salt stress, enhancing crop yield in the context of global change, such as those of arid and semi-arid zones, and would serve as an alternative tool for reduce the salt stress in the agriculture.
Data Availability Statement
The raw data supporting the conclusions of this article will be made available by the authors, without undue reservation.
Author Contributions
AS and FR designed the research. JF, PM, and NA sampled and performed laboratory work under the guidance of AS, MG, and EO-G. JF, MG, EO-G, and TC carried out data analysis. All authors wrote the manuscript, designed Tables and Figures, revised the manuscript, and approved the final version.
Conflict of Interest
The authors declare that the research was conducted in the absence of any commercial or financial relationships that could be construed as a potential conflict of interest.
Publisher's Note
All claims expressed in this article are solely those of the authors and do not necessarily represent those of their affiliated organizations, or those of the publisher, the editors and the reviewers. Any product that may be evaluated in this article, or claim that may be made by its manufacturer, is not guaranteed or endorsed by the publisher.
Acknowledgments
The authors thank Williams Arancibia, Jennifer Tapia, Carolayn Barraza, Carolina Nuñez, Eduardo Muñoz and Stefanie Maldonado. Authors acknowledge funding by Conicyt project R16A10003.
Supplementary Material
The Supplementary Material for this article can be found online at: https://www.frontiersin.org/articles/10.3389/fsufs.2022.768250/full#supplementary-material
References
Abdel-Latef, A. A. H., Tahjib-Ul-Arif, M., and Rhaman, M. S. (2021). Exogenous auxin-mediated salt stress alleviation in faba bean (Vicia faba L.). Agronomy 11, 547 doi: 10.3390/agronomy11030547
Aebi, H.. (1984). Catalase in vitro. Meth. Enzymol. 105, 121–126. doi: 10.1016/S0076-6879(84)05016-3
Ahn, T. S., Ka, J. O., Lee, G. H., and Song, H. G. (2007). Microcosm study for revegetation of barren land with wild plants by some plant growth-promoting rhizobacteria. J. Microbiol. Biotechnol. 17, 52–57. doi: 10.1080/07352680590910410
Alexander, D. B., and Zuberer, D. A. (1991). Use of chrome azurol S reagents to evaluate siderophore production by rhizosphere bacteria. Biol. Fertil. Soils 45, 12–39. doi: 10.1007/BF00369386
Azarmi-Atajan, F., and Sayyari-Zohan, M. H. (2020). Alleviation of salt stress in lettuce (Lactuca sativa L.) by plant growth-promoting rhizobacteria. J Horticulture Postharvest Res. 3, 67–78. doi: 10.22077/JHPR.2020.3013.1114
Baldani, J. I., Reis, V. M., Videira, S. S., Boddey, L. H., and Baldani, V. L. D. (2014). The art of isolating nitrogen-fixing bacteria from non-leguminous plants using N-free semi-solid media: a practical guide for microbiologists. Plant Soil 384, 413–431. doi: 10.1007/s11104-014-2186-6
Basu, A., Prasad, P., Das, S. N., Kalam, S., Sayyed, R. Z., Reddy, M. S., et al. (2021). Plant Growth Promoting Rhizobacteria (PGPR) as green bioinoculants: recent developments, constraints, and prospects. Sustainability 13, 1140 doi: 10.3390/su13031140
Bates, L., Waldren, S. R. P., and Teare, I. D. (1973). Rapid determination of free proline for water-stress studies. Plant Soil 39, 205–207. doi: 10.1007/BF00018060
Bradford, M. M.. (1976). A rapid and sensitive method for the quantitation of microgram quantities of protein utilizing the principle of protein dye binding. Anal. Biochem. 72, 248–254. doi: 10.1016/0003-2697(76)90527-3
Chia, K. F.. (2013). The Arabidopsis transcription factor ERF13 negatively regulates defense against Pseudomonas syringae. Ph.D. Thesis. University of California. California Digital Library.
Dubois, M., Skirycz, A., Claeys, H., Maleux, K., Dhondt, S., De Bodt, S., et al. (2013). Ethylene response factor 6 acts as a central regulator of leaf growth under water-limiting conditions in Arabidopsis. Plant Physiol. 162, 319–332. doi: 10.1104/pp.113.216341
Dubois, M., Van den Broeck, L., and Inzé, D. (2018). The pivotal role of ethylene in plant growth. Trends Plant Sci. 23, 311–323. doi: 10.1016/j.tplants.2018.01.003
Fan, D., Subramanian, S., and Smith, D. L. (2020). Plant endophytes promote growth and alleviate salt stress in Arabidopsis thaliana. Sci. Rep. 10, 12740 doi: 10.1038/s41598-020-69713-5
Gamalero, E., and Glick, B. R. (2015). Bacterial modulation of plant ethylene levels. Plant Physiol. 169, 13–22. doi: 10.1104/pp.15.00284
Garcia, C. L., Dattamudi, S., Chanda, S., and Jayachandran, K. (2019). Effect of salinity stress and microbial inoculations on glomalin production and plant growth parameters of snap bean (Phaseolus vulgaris). Agronomy 9, 545 doi: 10.3390/agronomy9090545
Giannopolitis, C. N., and Ries, S. K. (1977). Superoxide dismutases. Plant Physiol. 59, 309–314. doi: 10.1104/pp.59.2.309
Gill, S. S., and Tuteja, N. (2010). Reactive oxygen species and antioxidant machinery in abiotic stress tolerance in crop plants. Plant Physiol. Biochem. 48, 909–930. doi: 10.1016/j.plaphy.2010.08.016
Glick, B. R., Penrose, D. M., and Li, J. (1998). A model for the lowering of plant ethylene concentrations by plant growth-promoting bacteria. J. Theor. Biol. 190, 63–68. doi: 10.1006/jtbi.1997.0532
Glickmann, E., and Dessaeux, Y. (1995). A critical examination of the specificity of the Salkowski Reagent for indolic compounds produced by phytopathogenic bacteria. Appl. Environ. Microbiol. 61, 793–796. doi: 10.1128/aem.61.2.793-796.1995
Goswami, M., and Deka, S. (2020). Plant growth-promoting rhizobacteria alleviators of abiotic stresses in soil: a review. Pedosphere 30, 40–61. doi: 10.1016/S1002-0160(19)60839-8
Gupta, S., and Pandey, S. (2019). ACC deaminase producing bacteria with multifarious plant growth promoting traits alleviates salinity stress in French Bean (Phaseolus vulgaris) Plants. Front. Microbiol. 10, 1506 doi: 10.3389/fmicb.2019.01506
Han, H. S., and Lee, K. D. (2005). Plant growth promoting rhizobacteria effect on antioxidant status, photosynthesis, mineral uptake and growth of lettuce under soil salinity. J. Agri. Biol. Sci. 1, 210–215. Available online at: http://www.aensiweb.net/AENSIWEB/rjabs/rjabs/210-215.pdf
Hartmann, A., Klink, S., and Rothballer, M. (2021). Plant growth promotion and induction of systemic tolerance to drought and salt stress of plants by Quorum Sensing Auto-Inducers of the N-acyl-homoserine Lactone Type: recent developments. Front. Plant Sci. 12, 683546 doi: 10.3389/fpls.2021.683546
Hasanuzzaman, M., Raihan, M. R. H., Masud, A. A. C., Rahman, K., Nowroz, F., Rahman, M., et al. (2021). Regulation of reactive oxygen species and antioxidant defense in plants under salinity. Int. J. Mol. Sci. 22, 9326 doi: 10.3390/ijms22179326
Hossain, M. A., Hoque, M. A., Burritt, D. J., and Fujita, M. (2014). Chapter 16 - Proline protects plants against abiotic oxidative stress: biochemical and molecular mechanisms. In: Oxidative Damage to Plants (Academic press), 477–522. doi: 10.1016/B978-0-12-799963-0.00016-2
Jha, Y., and Subramanian, R. B. (2013). Root associated bacteria from the local variety of rice GJ-17 antagonized the growth of Magnaporthe grisea. Adv. Biores. 4, 70–77. Available online at: https://www.cabdirect.org/cabdirect/abstract/20133163180
Kaushal, M., and Wani, S. P. (2016). Rhizobacterial-plant interactions: strategies ensuring plant growth promotion under drought and salinity stress. Agric. Ecosyst. Environ. 231, 68–78. doi: 10.1016/j.agee.2016.06.031
Khalid, A., and Aftab, F. (2020). Effect of exogenous application of IAA and GA3 on growth, protein content, and antioxidant enzymes of Solanum tuberosum L. grown in vitro under salt stress. In Vitro Cell. Dev. Biol. Plant 56, 377–389. doi: 10.1007/s11627-019-10047-x
Kohler, J., Caravaca, F., and Roldán, A. (2010). An AM fungus and a PGPR intensify the adverse effects of salinity on the stability of rhizosphere soil aggregates of Lactuca sativa. Soil Biol. Biochem. 42, 429–434. doi: 10.1016/j.soilbio.2009.11.021
Kosakivska, I. V., Vedenicheva, N. P., Babenko, L. M., Voytenko, L. V., Romanenko, K. O., and Vasyuk, V. A. (2022). Exogenous phytohormones in the regulation of growth and development of cereals under abiotic stresses. Mol. Biol. Rep. 49, 617–628. doi: 10.1007/s11033-021-06802-2
Kumar, A., Singh, S., Gaurav, A. K., Srivastava, S., and Verma, J. P. (2020). Plant growth-promoting bacteria: biological tools for the mitigation of salinity stress in plants. Front. Microbiol. 11, e1216 doi: 10.3389/fmicb.2020.01216
Lee, S. J., Park, J. H., Lee, M. H., Yu, J. H., and Kim, S. Y. (2010). Isolation and functional characterization of CE1 binding proteins. BMC Plant Biol. 10, 277 doi: 10.1186/1471-2229-10-277
Li, Z., Chang, S., Ye, S., Chen, M., Lin, L., Li, Y., et al. (2015). Differentiation of 1-aminocyclopropane-1-carboxylate (ACC) deaminase from its homologs is the key for identifying bacteria containing ACC deaminase. FEMS Microbiol. Ecol. 91, fiv112 doi: 10.1093/femsec/fiv112
Licausi, F., Ohme-Takagi, M., and Perata, P. (2013). APETALA2/Ethylene Responsive Factor (AP2/ERF) transcription factors: mediators of stress responses and developmental programs. N. Phytol. 199, 639–649. doi: 10.1111/nph.12291
Livak, K. J., and Schmittgen, T. D. (2001). Analysis of relative gene expression data using real-time quantitative PCR and the 2– ΔΔCT method. Methods 25, 402–408. doi: 10.1006/meth.2001.1262
Marchesi, J. R., Sato, T., Weightman, A. J., Martin, T. A., Fry, J. C., Hiom, S. J., et al. (1998). Design and evaluation of useful bacterium-specific PCR primers that amplify genes coding for bacterial 16S rRNA. Appl. Environ. Microbiol. 64, 795–799. doi: 10.1128/AEM.64.2.795-799.1998
Moffat, C. S., Ingle, R. A., Wathugala, D. L., Saunders, N. J., Knight, H., and Knight, M. R. (2012). ERF5 and ERF6 play redundant roles as positive regulators of JA/Et-mediated defense against Botrytis cinerea in Arabidopsis. PLoS ONE 7, e35995 doi: 10.1371/journal.pone.0035995
Munns, R., and Tester, M. (2008). Mechanisms of salinity tolerance. Annu. Rev. Plant Biol. 59, 651–681. doi: 10.1146/annurev.arplant.59.032607.092911
Nakano, Y., and Asada, K. (1981). Hydrogen peroxide scanvenged by ascorbated specific peroxidase in spinach chloroplast. Plant Cell Physiol. 22, 867–880.
Nascimento, F. X., Rossi, M. J., and Glick, B. R. (2018). Ethylene and 1-aminocyclopropane-1-carboxylate (ACC) in plant-bacterial interactions. Front. Plant Sci. 9, 114 doi: 10.3389/fpls.2018.00114
Okur, B., and Örçen, N. (2020). “Chapter 12 - Soil salinization and climate change,” in Climate Change and Soil Interactions, 331–350. doi: 10.1016/B978-0-12-818032-7.00012-6
Ortega-Villasante, C., Rellán-Álvarez, R., Del Campo, F. F., Carpena-Ruiz, R. O., and Hernández, L. E. (2005). Cellular damage induced by cadmium and mercury in Medicago sativa. J. Exp. Bot. 56, 2239–2251. doi: 10.1093/jxb/eri223
Pan, Y., Seymour, G. B., Lu, C., Hu, Z., Chen, X., and Chen, G. (2012). An ethylene response factor (ERF5) promoting adaptation to drought and salt tolerance in tomato. Plant Cell Rep. 31, 349–360. doi: 10.1007/s00299-011-1170-3
Peng, Z., He, S., Gong, W., Sun, J., Pan, Z., Xu, F., et al. (2014). Comprehensive analysis of differentially expressed genes and transcriptional regulation induced by salt stress in two contrasting cotton genotypes. BMC Genomics 15, 760 doi: 10.1186/1471-2164-15-760
Porra, R. J., Thompson, W. A., and Kriedemann, P. E. (1989). Determination of accurate extinction coefficients and simultaneous equations for assaying chlorophylls a and b extracted with four different solvents: verification of the concentration of chlorophyll standards by atomic absorption spectroscopy. Biochim. Biophys. Acta 975, 384–394. doi: 10.1016/S0005-2728(89)80347-0
Potuschak, T., Lechner, E., Parmentier, Y., Yanagisawa, S., Grava, S., Koncz, C., et al. (2003). EIN3-dependent regulation of plant ethylene hormone signaling by two Arabidopsis F box proteins: EBF1 and EBF2. Cell 115, 679–689. doi: 10.1016/S0092-8674(03)00968-1
Rawat, N., Singla-Pareek, S. L., and Pareek, A. (2021). Membrane dynamics during individual and combined abiotic stresses in plants and tools to study the same. Physiol. Plant. 171, 653–676. doi: 10.1111/ppl.13217
Remonsellez, F., Castro-Severyn, J., Aguilar Espinosa, P. M., Fortt, J., Salinas, C., Barahona, S., et al. (2018). Characterization and salt response in recurrent halotolerant Exiguobacterium sp. SH31 isolated from sediments of Salar de Huasco, Chilean Altiplano. Front. Microbiol. 9, 2228 doi: 10.3389/fmicb.2018.02228
Ribba, T., Garrido-Vargas, F., and O'Brien, J. A. (2020). Auxin-mediated responses under salt stress: from developmental regulation to biotechnological applications. J. Exp. Bot. 71, 3843–3853. doi: 10.1093/jxb/eraa241
Rivero, R. M., Ruiz, J. M., and Romero, L. M. (2004). Importance of N source on heat stress tolerance due to the accumulation of proline and quaternary ammonium compounds in tomato plants. Plant Biol. 6, 702–707. doi: 10.1055/s-2004-821293
Shakeel, S. N., Gao, Z., Air, M., Chen, Y. F., Rai, M. I., Haq, U. N., et al. (2015). Ethylene regulates levels of ethylene receptor/CTR1 signaling complexes in Arabidopsis thaliana. J. Biol. Chem. 290, 12415–12424. doi: 10.1074/jbc.M115.652503
Sher, A., Sarwar, T., Nawaz, A., Ijaz, M., Sattar, A., and Ahmad, S. (2019). “Methods of seed priming,” in Priming and Pretreatment of Seeds and Seedlings (Singapore: Springer), 1–10.
Son, G. H., Wan, J., Kim, H. J., Nguyen, X. C., Chung, W. S., Hong, J. C., et al. (2012). Ethylene-responsive element-binding factor 5, ERF5, is involved in chitin-induced innate immunity response. Mol. Plant-Microbe Interact. 25, 48–60. doi: 10.1094/MPMI-06-11-0165
Spiers, A., Bohannon, J., Gehrig, S., and Rainey, P. (2003). Biofilm formation at the air-liquid interface by the Pseudomonas fluorescens SBW25 wrinkly spreader requires an acetylated form of cellulose. Mol. Microbiol. 50, 15–27. doi: 10.1046/j.1365-2958.2003.03670.x
Stepanova, A. N., and Alonso, J. M. (2009). Ethylene signalling and response: where different regulatory modules meet. Curr. Opin. Plant Biol. 12, 548–555. doi: 10.1016/j.pbi.2009.07.009
Taji, T., Ohsumi, C., Iuchi, S., Seki, M., Kasuga, M., Kobayashi, M., et al. (2002). Important roles of drought- and cold-inducible genes for galactinol synthase in stress tolerance in Arabidopsis thaliana. Plant J. 29, 417–426. doi: 10.1046/j.0960-7412.2001.01227.x
Tao, J. J., Chen, H. W., Ma, B., Zhang, W. K., Chen, S. Y., and Zhang, J. S. (2015). The role of ethylene in plants under salinity stress. Front. Plant Sci. 6, 1059 doi: 10.3389/fpls.2015.01059
Vaseva, I. I., Mishev, K., Depaepe, T., Vassileva, V., and Van Der Straeten, D. (2021a). The Diverse salt-stress response of Arabidopsis ctr1-1 and ein2-1Ethylene signaling mutants is linked to altered root auxin homeostasis. Plants 10, 452 doi: 10.3390/plants10030452
Vaseva, I. I., Simova-Stoilova, L., Kirova, E., Mishev, K., Depaepe, T., Van Der Straeten, D., et al. (2021b). Ethylene signaling in salt-stressed Arabidopsis thaliana ein2-1 and ctr1-1 mutants–a dissection of molecular mechanisms involved in acclimation. Plant Physiol. Biochem. 167, 999–1010. doi: 10.1016/j.plaphy.2021.09.029
Vázquez, P., Holguin, G., Puent, M. E., Lopez-Cortes, A., and Bashan, Y. (2000). Phosphate solubilizing microorganisms associated with the rhizosphere of mangroves in a semiarid coastal lagoon. Biol. Fertil. Soils 30, 460–468. doi: 10.1007/s003740050024
Vurukonda, S. S. K. P., Vardharajula, S., Shrivastava, M., and Sk, Z. A. (2016). Enhancement of drought stress tolerance in crops by plant growth promoting rhizobacteria. Microbiol. Res. 184, 13–24. doi: 10.1016/j.micres.2015.12.003
Xu, C., and Mou, B. (2015). Evaluation of Lettuce Genotypes for Salinity Tolerance. HortScience 50, 1441–1446. doi: 10.21273/HORTSCI.50.10.1441
Yeates, C., Gillings, M. R., Davison, A. D., Altavilla, N., and Veal, D. A. (1998). Methods for microbial DNA extraction from soil for PCR amplification. Biol. Proced. Online 14, 40–47. doi: 10.1251/bpo6
Zhang, M., Smith, J. A. C., Harberd, N. P., and Jiang, C. (2016). The regulatory roles of ethylene and reactive oxygen species (ROS) in plant salt stress responses. Plant Mol. Biol. 91, 651–659. doi: 10.1007/s11103-016-0488-1
Zhang, Y., Xu, F., Ding, Y., Du, H., Zhang, Q., Dang, X., et al. (2021). Abscisic acid mediates barley rhizosheath formation under mild soil drying by promoting root hair growth and auxin response. Plant Cell Environ. 44:1935–1945. doi: 10.1111/pce.14036
Zhu, F., Qu, L., Hong, X., and Sun, X. (2011). Isolation and Characterization of a Phosphate- Solubilizing Halophilic Bacterium Kushneria sp. YCWA18 from Daqiao Saltern on the Coast of Yellow Sea of China. Evidence-Based Complementary and Alternative Medicine, 1–6: ID 615032.
Keywords: PGPR, ethylene, ACC deaminase, salt stress tolerance, Latuca sativa L., ethylene signal pathway, antioxidant system
Citation: Fortt J, González M, Morales P, Araya N, Remonsellez F, Coba de la Peña T, Ostria-Gallardo E and Stoll A (2022) Bacterial Modulation of the Plant Ethylene Signaling Pathway Improves Tolerance to Salt Stress in Lettuce (Lactuca sativa L.). Front. Sustain. Food Syst. 6:768250. doi: 10.3389/fsufs.2022.768250
Received: 31 August 2021; Accepted: 11 February 2022;
Published: 22 March 2022.
Edited by:
Everlon Cid Rigobelo, São Paulo State University, BrazilReviewed by:
Himadri Bhusan Bal, Regional Medical Research Center (ICMR), IndiaShobhit Raj Vimal, University of Allahabad, India
Maarten Ryder, University of Adelaide, Australia
Copyright © 2022 Fortt, González, Morales, Araya, Remonsellez, Coba de la Peña, Ostria-Gallardo and Stoll. This is an open-access article distributed under the terms of the Creative Commons Attribution License (CC BY). The use, distribution or reproduction in other forums is permitted, provided the original author(s) and the copyright owner(s) are credited and that the original publication in this journal is cited, in accordance with accepted academic practice. No use, distribution or reproduction is permitted which does not comply with these terms.
*Correspondence: Alexandra Stoll, YWxleGFuZHJhLnN0b2xsJiN4MDAwNDA7Y2VhemEuY2w=; Máximo González, bWF4aW1vLmdvbnphbGV6JiN4MDAwNDA7Y2VhemEuY2w=