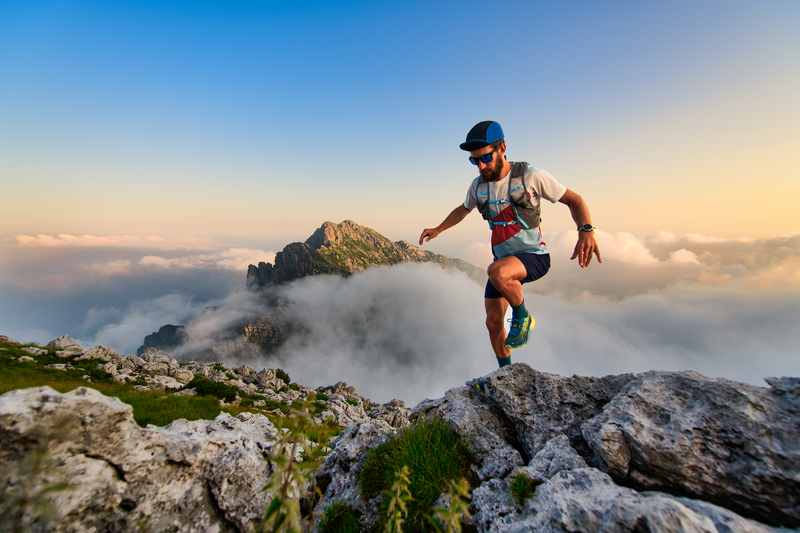
94% of researchers rate our articles as excellent or good
Learn more about the work of our research integrity team to safeguard the quality of each article we publish.
Find out more
ORIGINAL RESEARCH article
Front. Sustain. Food Syst. , 09 January 2023
Sec. Crop Biology and Sustainability
Volume 6 - 2022 | https://doi.org/10.3389/fsufs.2022.1111573
This article is part of the Research Topic Biostimulants for Climate-Smart and Sustainable Agriculture View all 12 articles
The production of pepper plants for industrial use is not enough to satisfy the demand of consumers and agrochemicals are frequently used to increase production. In this study four native plant growth promoting rhizobacteria (PGPR) was tested as an alternative to select the most effective to enhance growth, development, and productivity of pepper plants. Seedlings were inoculated with Pseudomonas 42P4, Cellulosimicrobium 60I1, Ochrobactrum 53F, Enterobacter 64S1 and cultivated on pots in the greenhouse and the morphological, biochemical, and physiological parameters were determined. In addition, the phenolic compound profiles were evaluated. All four strains increased the different parameters evaluated but Pseudomonas 42P4 and Cellulosimicrobium 60I1 were the most effective strains, improving leaf and root dry weight, stem diameter, nitrogen level, stomatal conductance, chlorophyll quantum efficiency, chlorophyll SPAD index, total chlorophyll and carotenoid levels, number of flowers and fruits per plant, and the length, diameter and dry weight of the fruit. Also, these strains modified the phenolic compound profiles, and 18 compounds were quantified. Pseudomonas 42P4 inoculation modified the phenolic compound profile similarly to the Fertilized treatment and induced the synthesis of different endogenous compounds in the flavonoid family, also increasing catechin, naringin, naringenin, myricetin, procyanidin B1, epigallocatechin-gallate, cinnamic, and ferulic acids related to antioxidant activity and catechin, cinnamic, and ferulic acids related to the induced systemic response. Pseudomonas 42P4 can be used as a bioinoculant in pepper plants to enable better agronomic management, decreasing the use of chemical fertilizer to contribute to sustainable agriculture.
- Bioinoculants formulated with PGPR are a sustainable alternative to increase crop production.
- Pseudomonas 42P4 and Cellulosimicrobium 60I1 are two promising strains for preparing a bioinoculant.
- The strains used have the ability to increase growth, development, and production of pepper plants.
- The phenolic compound profiles (eighteen compounds identified and quantified) are modified in plants inoculated with the strains under study.
- Pseudomonas 42P4 mainly increases compounds grouped in the flavonoid and phenolic acid families.
Pepper (Capsicum annuum) is one of the most important horticultural crops worldwide. It is an essential food ingredient and the fourth major crop produced globally. Approximately 400 varieties of pepper are cultivated worldwide (Saxena et al., 2016). In the province of Mendoza, Argentina, it is calculated that around 1246 ha of peppers are grown for the packaging industry (FAOSTAT, 2021). Pepper plants need a sufficient amount of nutrients to grow and develop fruit and so the farmers apply fertilizer and agrochemicals to improve the production. However, the overuse of some chemical products affects human health and deteriorates the quality of the environment.
Bioinoculants formulated with microorganisms are currently considered as a promising alternative to inorganic fertilizers, constituting a powerful tool in organic agriculture and for the restoration of degraded soils (De-Bashan et al., 2012; Gouda et al., 2018). Some bioinoculants are formulated with plant growth promoting rhizobacteria (PGPR). These strains have beneficial properties that improve plant growth and development through different mechanisms, such as atmospheric nitrogen fixation, siderophore production, phosphate solubilization, and production of plant growth regulators (Basu et al., 2021; Mohanty et al., 2021). In addition, native PGPR can alleviate the environmental stresses to which plants are often exposed, since they tend to adapt easily to the local conditions of the soils from which they were isolated, withstanding environmental stress caused by extreme gradients of temperature, high concentrations of salts in the soil and hydric stress (Grover et al., 2011; Cordero et al., 2018).
In the rhizosphere there are a series of organic molecules that regulate chemotaxis between plant roots and PGPR. The phenolic compounds stand out within this group of molecules, which are part of the secondary metabolism in plants, and their levels in plants may be regulated by interactions that occur in the rhizosphere (Feng et al., 2018). Simple phenolic alcohols, flavonoids, phenolic acids, and stilbenoids represent the main families of phenolic compounds. In each family the number of phenolic compounds and their concentrations are different depending on the plant tissue studied (Vuolo et al., 2019; Alara et al., 2021). Phenolic compounds have a key role as defense compounds under environmental stress conditions, such as inadequate light (excess or deficiency), saline stress or heavy metals, low temperatures, unfavorable pH, pathogen infection, herbivory, and nutrient deficiency, which can induce a higher production of oxidative species in plants (Lattanzio, 2013; Tanase et al., 2019).
Some studies based on colorimetric techniques have reported an increase in phenolic compounds in plants inoculated with PGPR (Del Rosario Cappellari et al., 2017; Pagnani et al., 2018; Rahimi et al., 2020). However, the mechanism of modification of phenolic compound profiles in the plant-PGPR interaction is limited and the specific role of the different families is unknown (Sarma et al., 2002). For this purpose, it is necessary to use liquid chromatography to individualize each compound.
In Mendoza province, Argentina, our research group isolated and characterized native PGPR strains from roots and the rhizosphere of a tomato crop (Pérez-Rodriguez et al., 2020). We showed that inoculation with these native strains was effective in promoting the germination percentage, the vigor index and modifying the profile of phenolic compounds, suggesting an elicitation of phenylpropanoid pathways related to induced systemic response (IRS) in pepper seeds (Lobato-Ureche et al., 2021). We also reported that these native PGPR promote the growth and development of tomato plants and reduce the negative effects of salt stress by NaCl in tomato plants cultivated in the greenhouse (Pérez-Rodriguez et al., 2020, 2022).
In the present study, we were interested in the impact of these native strains, known as Enterobacter 64S1, Pseudomonas 42P4, Cellulosimicrobium 60I1, and Ochrobactrum 53F, on the morphological, biochemical, physiological, and on the phenolic compounds profile changes of pepper plants. Also we evaluated the effect of inoculation on the fruit production. Our hypothesis was that inoculation of pepper plants with native PGPR modifies the profile of phenolic compounds, favoring growth, development, and yield, similar to a chemical fertilizer.
Seeds of Capsicum annuum cv Calafyuco INTA were kindly supplied by Dr. C. Galmarini (National Institute of Agricultural Technology, INTA-EEA, La Consulta, Mendoza, Argentina).
The strains used were Cellulosimicrobium 60I1 (60I1), Ochrobactrum 53F (53F), Enterobacter 64S1 (64S1), and Pseudomonas 42P4 (42P4). These strains belong to the Microbiology and Agricultural Physiology Lab (IBAM-FCA, CONICET-UNCuyo, Mendoza, Argentina) and have been deposited in the GenBank (NCBI) under accession numbers MT047266, MT047264, MT047267, MT045593. These strains were isolated from the rhizosphere and roots of tomato plants from productive farms in Mendoza, Argentina and were characterized as PGPR (Pérez-Rodriguez et al., 2020).
The pre-inoculum was prepared in 50 mL of a rich medium of LB (Luria Broth, Sigma Chem. Co.) from one colony of the Cellulosimicrobium 60I1, Ochrobactrum 53F, Enterobacter 64S1, and Pseudomonas 42P4 strains. Every culture was grown for 24 h at 28°C and 120 rpm until reaching an OD530 = 1.2. To prepare the inoculum, 500 μL of pre-inoculum were grown in 50 mL of LB for 24 h at 28°C and 120 rpm until reaching 108 CFU mL−1. The seedlings were inoculated with 1,000 μL of each culture as detailed below.
The seeds were surface disinfected with 20% sodium hypochlorite for 1 min, then washed three times with sterile distilled water to remove the rest of the disinfectant. The seeds were sown in sterile trays containing sterilized substrate Kekkilä DSM 1 W (Kekkilä professional, Vantaa, Finland). The substrate contained 70% brown and 30% dark Sphagnum fuscum dominant peat, N–P2O5-K2O 15–12–29 and microelements 0.6 kg m−3, pH 5.9, electrical conductivity 0.2 dS m−1. The trays were placed in a greenhouse at a temperature of (24–26°C average daily temperature, 10–14 light and dark periods, and 700–750 μmol m−2 s−1 of light intensity at solar noon). The seedlings with two fully expanded leaves were inoculated with 1,000 μL of PGPR containing 108 CFU mL−1 of the corresponding bacterial culture (inoculum). Thus, the treatments were: (1) seedlings inoculated with Cellulosimicrobium 60I1, (2) seedlings inoculated with 53F, (3) seedlings inoculated with Enterobacter 64S1, (4) seedlings inoculated with Pseudomonas 42P4, (5) seedlings treated with fertilizer Hakaphos® 18-18-18 (N-P-K), (6) Control: seedlings without bacterial inoculum (inoculated with LB medium). All treatments were applied on the soil surface near the root collar. The seedlings were then transplanted into plastic pots (30 L) containing a mixture of Kekkila DSM 1 W substrate:sand (50:50) and placed in a greenhouse with the same condition described previously. The Fertilized treatment was prepared at the rate of 0.7 g L −1 with Hakaphos® (a completely water soluble fertilizer for fruit and vegetable crops, free of chlorine and urea with EDTA-chelated trace elements). The treatment was applied at the rate of 1 mL per seedlings, and followed by subsequent treatments at 10 mL on 45 days-old and 20 mL on 70 days-old plants. A completely randomized design of six treatments (1–6) was established, with three repetitions of 10 seeds in each one (with a total of 60 seedlings). The experiment was repeated three times.
The growth parameters were measured in 75 day-old plants and data were collected to evaluate the morphological aspects including: plant height and stem diameter, while leaf area was measured using the Micrometrics SE premium software. Physiological parameters were also measured at 90 day-old plants: stomatal conductance was determined with a porometer (Decagon Devices, Model SC-1, USA), maximum quantum yield of PSII (Fv/Fm) was determined with a fluorometer (Hansatech Instruments LTD). Fv/Fm is the maximum efficiency at which light is absorbed by PSII and is used as a sensitive indicator of plant photosynthetic performance (Asghari et al., 2020), where Fm: maximum fluorescence and Fv: the variable fluorescence. The Chlorophyll index (SPAD), was determined using a portable chlorophyll meter (SPAD-502, Konica Minolta Sensing). At the time of flowering, the number of flowers per plant and the days of flowering were counted. Yield parameters were measured at the end of the assay after 110 days (number of fruits per plant, weight, length and diameter of the fruits). Finally, the aerial and root dry weights were determined.
Leaves of 55 day-old plants were dried in an oven at 70°C for 72 h until reaching a constant dry weight. Later, they were ground and the nitrogen content was determined by Micro Kjeldahl, as described by Guebel et al. (1991).
Determinations were carried out spectrophotometrically in a UV–Vis spectrophotometer Cary 50 (Varian Inc.) as described by Chappelle et al. (1992), with modifications of Cohen et al. (2015), using leaf samples (55 days old). Total chlorophyll (Chl; Chl a + Chl b), carotenoid and anthocyanin levels were measured from 1 cm diameter disc samples and expressed in mg−1 of leaves.
The phenolic compounds were extracted using a liquid-solid extraction according to a previously reported procedure (Moussi et al., 2015), which can be briefly described as follows: 0.5 g of lyophilized material (leaves) were weighed in a conical centrifuge tube and mixed with 5 mL of ethanol. Then, the tube was left in an ultrasonic bath for 10 min and the supernatant obtained by centrifugation (2,500 g for 10 min) was evaporated to dryness using a rotary evaporator at 40°C. The residue was redissolved in 1 mL of 0.1% (v/v) formic acid.
Each individual phenolic compound in the pepper leaf extracts was separated using high-performance liquid chromatography, coupled to diode array and fluorescence detectors (HPLC-DAD-FLD). Dionex UltiMate 3000 HPLC system (California, USA). Chromatographic separations were carried out in reversed-phase Kinetex C18 column (3.0 mm × 100 mm, 2.6 μm). Phenomenex (Torrance, CA, USA) at 35°C. The mobile phases were ultrapure water with 0.1% (v/v) formic acid (phase A) and acetonitrile (phase B). Separation of the analytes was performed using the following gradient: 0–1.7 min, 5% B; 1.7–10 min, 30% B; 10–13.5 min, 95% B; 13.5–15 min, 95% B; 15–16 min, 5% B; 16–19, 5% B. The flow rate was set constant at 0.8 mL min−1 during the whole process, and the injection volume was 5 μL.
The identification and quantification of the target phenolic compounds in the extracts were based on the comparison of the retention times (tR) and the maximum absorbance value of detected peaks in samples of interest with those obtained by the injection of pure standards. The working wavelengths for DAD of the different families of analytes were 254, 280, 320, and 370 nm, while an excitation wavelength (Ex) of 290 nm and monitored emission (Em) responses of 315 and 400 nm were used depending on the targeted analytes for FLD. The Chromeleon 7.1 software was used to control all the acquisition parameters of the HPLC-DAD-FLD system and also to process the data obtained.
Data were processed by analysis of variance followed by a Duncan test to discriminate between the averages by the minimum difference with a significance level of P ≤ 0.01. The InfoStat statistical software (InfoStat version 2018v. Grupo InfoStat, Argentina) was used.
Inoculation with PGPR increased the leaf and aerial (leaves plus stems) dry weight of the plants, as shown in Figure 1. The Fertilized, Pseudomonas 42P4, and Cellulosimicrobium 60I1 treatments increased the dry weight of the leaves (34, 21, and 15%, respectively), with respect to the Control. A similar trend was observed in the dry weight of the aerial part.
Figure 1. (A) Dry weight of the leaves and (B) dry weight of the aerial parts (leaves and stems) of pepper plants of 110 day-old grown in pots and treated with: Fert: Fertilized; 42P4: Pseudomonas 42P4; 60I1: Cellulosimicrobium 60I1; 64S1: Enterobacter 64S1; 53F: Ochrobactrum 53F and Control (without bacteria). Data are presented as mean ± SEM of seven independent biological replicates. Different letters indicate significant differences according to one-way ANOVA with Duncan's multiple range test. P (A) = 0.0034 and P (B) = 0.0005.
Inoculation with PGPR increased root dry weight and stem diameter, as shown in Figures 2A, B. The increase in the root dry weight was the same between the inoculated treatments with respect to the Control. The Fertilized, Enterobacter 64S1, Cellulosimicrobium 60I1, and Pseudomonas 42P4 treatments increased stem diameter by 16%; 13%; 11 and 6%, respectively.
Figure 2. (A) Root dry weight and (B) stem diameter, of pepper plants of 110 and 75 day-old (respectively), grown in pots and treated with: Fert: Fertilized; 42P4: Pseudomonas 42P4; 60I1: Cellulosimicrobium 60I1; 64S1: Enterobacter 64S1; 53F: Ochrobactrum 53F and Control (without bacteria). Data are presented as mean ± SEM of 10 independent biological replicates. Different letters indicate significant differences according to one-way ANOVA with Duncan's multiple range test. P (A) = 0.0001 and P (B) = 0.0028.
The highest nitrogen content was found in the inoculated and fertilized treatments with respect to the Control, as shown in Figure 3. The Pseudomonas 42P4 and Cellulosimicrobium 60I1 treatments increased the nitrogen content (48 and 41%, respectively), with respect to the Control, showing similar behavior to the Fertilized treatment, while Enterobacter 64S1 and Ochrobactrum 53F exceeded the control (29 and 18%, respectively).
Figure 3. Nitrogen content in leaves of pepper plants of 55 day-old after treatment with Fert: Fertilized; 42P4: Pseudomonas 42P4; 60I1: Cellulosimicrobium 60I1; 64S1: Enterobacter 64S1; 53F: Ochrobactrum 53F and Control (without bacteria). Data are presented as mean ± SEM of three independent biological replicates. Different letters indicate significant differences (P < 0.0085) according to one-way ANOVA with Duncan's multiple range test.
Inoculation with PGPR modifies the physiological parameters in pepper plants. The Enterobacter 64S1, Pseudomonas 42P4, fertilized, Ochrobactrum 53F and Cellulosimicrobium 60I1 treatments increased stomatal conductance by 24%; 21, 21%; 18 and 15%, respectively, with respect to the Control (Figure 4A). Similarly, the Fv/Fm increased in the inoculated and Fertilized treatments, exceeding the control by as much as 11% (Figure 4B).
Figure 4. (A) Stomatal conductance and (B) Chlorophyll quantum efficiency (Fv/Fm) of pepper plants of 90 day-old grown in pots and treated with: Fert: Fertilized; 42P4: Pseudomonas 42P4; 60I1: Cellulosimicrobium 60I1; 64S1: Enterobacter 64S1; 53F: Ochrobactrum 53F and Control (without bacteria). Data are presented as mean ± SEM of 10 independent biological replicates. Different letters indicate significant differences according to one-way ANOVA with Duncan's multiple range test. P (A) = 0.0025 and P (B) = 0.0009.
The contents of photosynthetic pigments (Chl a and total), as well as carotenoids, were higher in the inoculated and Fertilized treatments, with respect to the Control, as shown in Table 1 and Figure 5.
Figure 5. Chlorophyll index (SPAD) of pepper plants of 90 day-old grown in pots and treated with: Fert: Fertilized; 42P4: Pseudomonas 42P4; 60I1: Cellulosimicrobium 60I1; 64S1: Enterobacter 64S1; 53F: Ochrobactrum 53F and Control (without bacteria). Data are presented as mean ± SEM of 10 independent biological replicates. Different letters indicate significant differences (P < 0.0099) according to one-way ANOVA with Duncan's multiple range test.
Inoculation did not modify the days to flowering. However, the number of flowers per plant increased, and all treatments differed from the Control except the 64S1 treatment, as shown in Table 2.
The Fertilized, Pseudomonas 42P4, Cellulosimicrobium 60I1, and Enterobacter 64S1 treatments had the highest fruit production with respect to the Control. The Fertilized treatments, 42P4 and 60I1 had the longest fruits (9; 8 and 8%, respectively), with respect to the Control. The Enterobacter 64S1, Pseudomonas 42P4 and Ochrobactrum 53F treatments increased the fruit diameter and were different to the Fertilized and Control treatments. The Fertilized, Pseudomonas 42P4, Cellulosimicrobium 60I1, and Ochrobactrum 53F treatments increased the fruit dry weight (67; 45; 42 and 38%, respectively) with respect to the Control (Table 2).
Figure 6 shows the biplot graph for 16 variables (morphological, physiological, and yield component) of the pepper plants cultivated in pots. PC1 explained 76% of the variance and the variables associated with this component were fruit dry weight, total chlorophyll, carotenoids, Fv/Fm, total Chl, Chl a, aerial dry weight, fruit length, root dry weight, leaf dry weight, number of fruits and SPAD index. PC2 explained 12% of the variance and the variables associated with this component were fruit diameter, stomatal conductance, and number of flowers. Principal components analysis separated the Pseudomonas 42P4, Cellulosimicrobium 60I1 and Fertilized treatments in a first group and the second group consisted of the Ochrobactrum 53F3 and Enterobacter 64S1 treatments, and the third group, the Control treatment.
Figure 6. Biplot of principal components analysis of the effect of PGPR strains on the parameters evaluated in pepper plants grown in pots under greenhouse conditions.
The constitution of the first group shows that inoculation with Pseudomonas 42P4 and Cellulosimicrobium 60I1 obtained results similar to those found with the chemical fertilizer treatment. Based on these results, these treatments were selected for evaluating the phenolic compound profiles and to compare with the Control.
The phenolic compounds present in the leaves of uninoculated (Control), inoculated with Pseudomonas 42P4 and Cellulosimicrobium 60I1 strains, and Fertilized pepper plants are shown in Table 3. A total of 18 phenolic compounds, grouped in four families based on their chemical structure, were identified and quantified: phenolic alcohols, flavonoids, phenolic acids, and stilbenoids. This study revealed that the sum of the phenolic compounds was higher in plants inoculated with the Pseudomonas 42P4 strain and Fertilized treatments, whereas plants inoculated with the Cellulosimicrobium 60I1 strain were similar to the Control treatment.
Table 3. Phenolic compounds quantified in pepper leaves of plants subjected to different treatments.
As phenolic alcohols we identified hydroxytyrosol and tyrosol. The concentration of both compounds was highest in the Fertilized treatment (4.92 mg g−1), followed by the Control (2.17 mg g−1), but the inoculated treatments had lower concentration.
In the flavonoid family, the following compounds were identified and quantified: (+)-catechin, rutin, (-)-gallocatechin gallate, (-)-epigallocatechin gallate, quercetin, astilbin, naringin, naringenin, myricetin, and procyanidin B1. The Fertilized treatment had the highest concentration of total flavonoids (152.71 mg g−1), followed by Pseudomonas 42P4 (140.55 mg g−1), Cellulosimicrobium 60I1 (52.38 mg g−1), and the Control (45.58 mg g−1). The (+)-catechin and procyanidin B1 were only detected in the Pseudomonas 42P4 treatment. The 42P4 had the highest concentration of (-)-gallocatechin gallate (35.98 mg g−1), followed by Fertilized and Cellulosimicrobium 60I1 (30.95 and 15.61 mg g−1, respectively) and they differed from the Control (12.15 mg g−1). The Fertilized had the highest concentration of naringin (68.68 mg g−1), followed by Pseudomonas 42P4 and Cellulosimicrobium 60I1 (54.93 and 32.91 mg g−1, respectively) and they differed from the Control (24.66 mg g−1).
As phenolic acids, cinnamic, p-coumaric, and ferulic acids were quantified. The 42P4 had high concentrations of total phenolic acids (39.63 mg g−1), followed by Fertilized, Control, and 60I1 (35.96, 9.14, and 5.50 mg g−1, respectively). The Pseudomonas 42P4 and Fertilized treatments had high concentrations of cinnamic acid (32.07 and 28.92 mg g−1, respectively). The Pseudomonas 42P4 had the highest concentration of ferulic acid (7.24 mg g−1), followed by Fertilized and Cellulosimicrobium 60I1 (6.60 and 1.95 mg g−1), but it was not detected in the Control. The Control had high concentrations of p-coumaric acid.
The stilbenoid group includes polydatin, trans-resveratrol and pterostilbene. The Control had high concentrations of total stilbenoid (16.16 mg g−1) followed by Pseudomonas 42P4, Fertilized and Cellulosimicrobium 60I1 (11.97; 9.24 and 6.06 mg g−1). The Control had the highest concentration of polydatin, triple the content of the other treatments; while Pseudomonas 42P4 had higher concentrations of trans-resveratrol.
Capsicum annuum is an important commercial horticultural crop that has high nutritional value (Shiragaki et al., 2020). Bioinoculants formulated with PGPR are an alternative for increasing production while reducing adverse effects on the environment. In our study, the inoculation with PGPR stimulated the growth and nitrogen uptake of pepper plants. The greater absorption of nitrogen in the inoculated plants favored an increase in the physiological parameters, such as the content of total Chl, Chl index, and maximum quantum yield of PSII (Fv/Fm). The increase in Chl and the stability of the photosynthetic rate allow us to explain the increase in morphological variables, such as the stem diameter and the aerial and root dry weights. Pérez-Rodriguez et al. (2020) showed that the strains used in this study produce indole acetic acid and siderophores, fix nitrogen and solubilize phosphate. In the present study, we suggest that these mechanisms could be involved in the higher dry matter of the inoculated plants. Similar results have been reported in other plant species inoculated with PGPR (Samaniego-Gámez et al., 2016; Lopes et al., 2018; Hafez et al., 2019; Anbi et al., 2020).
The greater vegetative development in the inoculated plants improved the parameters associated with the yield components. The inoculated plants had a greater number of flowers associated with a greater probability of fruit set, which correlated with an increase in yield. The higher fruit dry weight of the inoculated plants could be a consequence of the higher photosynthetic rate, which induced a higher fixation of photo-assimilates that favor the filling of the fruits.
The principal component analysis showed that the treatments inoculated with Cellulosimicrobium 60I1 and Pseudomonas 42P4 behaved similarly to the Fertilized treatment and these strains were the most effective inoculation treatments. We suggest that the strains have displayed various growth promotion mechanisms, inducing an improvement in the growth and yield parameters with respect to the Control treatment. Similar results have been reported after inoculation with different PGPR in chrysanthemum (Kumari et al., 2016), blackberry (Robledo-Buriticá et al., 2018), strawberry (Ipek et al., 2014; Kurokura et al., 2017), and cherry tomato plants (Aini et al., 2019).
An increase in the total phenolic compounds has frequently been reported in different plant species inoculated with PGPR. However, to deepen the topic, we studied four families of phenolic compounds. In this way, the individualization of each compound helped us to clarify their possible role in the inoculated pepper plants. So, the abundance of flavonoids in plants inoculated with the Pseudomonas 42P4 strain may be related to the effective colonization of the root system by bacteria. The role of flavonoids in the signaling that mediates root colonization by PGPR is well-documented (Mierziak et al., 2014).
The compound (+)- catechin was only detected in the treatment inoculated with Pseudomonas 42P4. The role of catechin has not been clearly defined in plant physiology (Bais et al., 2010). Rani et al. (2011) indicated that the exogenous application of this compound in Arabidopsis thaliana plants improved the net photosynthetic rate, stomatal conductance, and indole acetic acid (IAA), also enhancing biomass accumulation, leaf area and leaf thickness. Other authors reported that catechin is associated with tolerance to oxidative stress and cold acclimatization in plants (Yiu et al., 2011; Ding et al., 2019). These antecedents led us to suggest that inoculation with strain Pseudomonas 42P4 stimulates the synthesis endogenous of (+)-catechin, which might induce a positive response in growth, nitrogen accumulation, enhanced Chl and photosynthetic rate, which leads to a greater accumulation of biomass and an increased yield. Similar results were reported by Chakraborty et al. (2015) in tea plants inoculated with Bacillus megaterium.
The inoculated and Fertilized treatments had the highest concentration of endogenous naringin and myricetin. These compounds are considered as a non-enzymatic antioxidant mechanism effective in the elimination of reactive oxygen species (Csepregi and Hideg, 2018; Liu et al., 2021). According to our results, we suggest that the metabolic balance observed in the photosynthetic rates and physiological parameters in inoculated plants pepper could be related to the antioxidant capacity conferred by antioxidant compounds, such as naringin and myricetin.
Naringenin was only detected in the Pseudomonas 42P4 and Fertilized treatments. Naringenin is a precursor for the synthesis of other flavonoids (Liu et al., 2021). It is feasible to suggest that both treatments induce the production of naringenin, stimulating the production of other flavonoids in pepper plants. This hypothesis is confirmed by the fact that procyanidin B1 concentrations were also higher in the Pseudomonas 42P4 treatment. However, the results allow us to suggest an alternative hypothesis based on the accumulation of (+)-catechin for the production of procyanidin B1 in plants inoculated with Pseudomonas 42P4. A recent study indicated that (+)-catechin is closely linked to the synthesis of procyanidin B1 in Camellia sinensis (Wang et al., 2020).
The flavonoid (-)-epigallocatechin gallate was only quantified in the Pseudomonas 42P4 treatment. Therefore, it can be suggested that the 42P4 strain acts by modifying or modulating the levels of endogenous (-)-epigallocatechin gallate, favoring the accumulation of biomass and increasing the contents of photosynthetic pigments of plants. This compound increased seed germination and the growth of tomato seedlings. In addition, (-)-epigallocatechin gallate is a known protective agent against different types of stress such as salt stress, heat, cold, and drought. The beneficial effect of (-)-epigallocatechin gallate is based on the protection of the photosynthetic apparatus through the reduction of ROS (Ahammed et al., 2018; Li et al., 2019). Hong et al. (2015) reported that exogenous (-)-epigallocatechin gallate induces antifungal defense in Arabidopsis thaliana.
The Pseudomonas 42P4 treatment had the highest concentration of phenolic acids. The most abundant compounds quantified in this treatment were cinnamic and ferulic acids that have antioxidant activity and are effective in controlling pathogens. Therefore, Pseudomonas 42P4 may promote induced systemic resistance by stimulating the synthesis of these compounds. These family compounds can act as powerful antifungals, improving the response of pepper plants against the attack of phytopathogens. These results agree with studies carried out under in vitro conditions where an increase in endogenous phenolic acids was demonstrated when confronting strains of P. aeruginosa and P. fluorescens in the presence of Sclerotium rolfsii, which is an important phytopathogen in pepper crops (Singh et al., 2012). In this sense, other authors reported that inoculation with P. fluorescens and Microccucuce yunnanensis increased the endogenous levels of cinnamic acid (Sarma et al., 2002; Sarma and Singh, 2003; Singh et al., 2012; Rahimi et al., 2020).
The higher concentration of trans-resveratrol was quantified in the Pseudomonas 42P4 treatment. This compound may benefit the health of pepper plants because it intervenes in the response of plants against fungal attacks. Similar results were reported in another study where grapevine plants inoculated with Paraburkholderia phytofirmans modulated the levels of stilbenoids (Miotto-Vilanova et al., 2019).
In summary, this study demonstrated that Pseudomonas 42P4 and Cellulosimicrobium 60I1 are two promising native strains that can be used to improve the growth, development, and production of pepper plants. However, the response of Pseudomonas 42P4 was more effective than Cellulosimicrobium 60I1, and Pseudomonas 42P4 inoculation modified the phenolic compound profile similarly to the Fertilized treatment. Figure 7 shows a schematic representation of the main changes produced by the different treatments. Pseudomonas 42P4 induced the synthesis of different endogenous polyphenolic compounds mainly related to chemotaxis (flavonoid family), antioxidant capacity (catechin, naringin, naringenin, myricetin, procyanidin B1, and epigallocatechin-gallate), and the induction of resistance to pathogens because trans-cinnamic acid and benzoic acid are precursor of salicylic acid, a hormone that mediates host response upon pathogen infection. Also cinnamic and ferulic acid participate in the response to pathogen attack. These results contribute to understanding the changes in the endogenous levels of the phenolic compound profile. However, studies with exogenous application of these compounds are necessary to corroborate the proposed hypothesis. Finally, Pseudomonas 42P4 can be used as a bioinoculant in pepper plants to allow better agronomic management, decreasing the use of chemical fertilizers to contribute to climate-smart and sustainable agriculture, improving productivity and contributing efficiently to the country's economy and the conservation of natural resources.
Figure 7. Summary diagram of the main phenolic compounds in pepper plants grown in pots and treated with: Fert: Fertilized; 42P4: Pseudomonas 42P4; 60I1: Cellulosimicrobium 60I1 and Control (without bacteria).
The datasets presented in this study can be found in online repositories. The names of the repository/repositories and accession number(s) can be found at: https://www.ncbi.nlm.nih.gov/ MT047266, MT047264, MT047267, and MT045593.
Conception and design of the experiments: RM, AC, and ML-U. Performance of the experiments and data analysis: ML-U with collaboration of MP-R (bacteria and morphological determination) and DS (nitrogen determination). Contribution of reagents/materials/analysis tools: AC and RM. Writing the initial draft: ML-U. All authors critically reviewed and modified the paper.
This study was supported by funding from Fondo para la Investigación Científica y Tecnológica (FONCYT, PICT 2017-2571 and PICT-2020-SERIEA-03618 to AC) and Universidad Nacional de Cuyo (SIIP-UNCUYO to AC).
AC and RM are career members of CONICET. MP-R and ML-U are recipients of a scholarship from CONICET. DS is a Professional Technician of CONICET. The authors are grateful to Rosemary Scoffield for the English editing of the manuscript.
The authors declare that the research was conducted in the absence of any commercial or financial relationships that could be construed as a potential conflict of interest.
All claims expressed in this article are solely those of the authors and do not necessarily represent those of their affiliated organizations, or those of the publisher, the editors and the reviewers. Any product that may be evaluated in this article, or claim that may be made by its manufacturer, is not guaranteed or endorsed by the publisher.
Ahammed, G. J., Li, Y., Li, X., Han, W. Y., and Chen, S. (2018). Epigallocatechin-3-gallate alleviates salinity-retarded seed germination and oxidative stress in tomato. J. Plant Growth Regul. 37, 1349–1356. doi: 10.1007/s00344-018-9849-0
Aini, N., Dwi Yamika, W. S., and Pahlevi, R. W. (2019). The effect of nutrient concentration and inoculation of PGPR and AMF on the yield and fruit quality of hydroponic cherry tomatoes (Lycopersicon esculentum Mill. var. cerasiforme). J. Appl. Hortic. 21, 2–25. doi: 10.37855/jah.2019.v21i02.20
Alara, O. R., Abdurahman, N. H., and Ukaegbu, C. I. (2021). Extraction of phenolic compounds: a review. CRFS 4, 200–214. doi: 10.1016/j.crfs.2021.03.011
Anbi, A. A., Mirshekari, B., Eivazi, A., Yarnia, M., and Behrouzyar, E. K. (2020). PGPRs affected photosynthetic capacity and nutrient uptake in different Salvia species. J. Plant Nutr. 43, 108–121. doi: 10.1080/01904167.2019.1659342
Asghari, B., Khademian, R., and Sedaghati, B. (2020). Plant growth promoting rhizobacteria (PGPR) confer drought resistance and stimulate biosynthesis of secondary metabolites in pennyroyal (Mentha pulegium L.) under water shortage condition. Sci. Hortic. 263, 109132. doi: 10.1016/j.scienta.2019.109132
Bais, H. P., Venkatachalam, L., and Biedrzycki, M. L. (2010). Stimulation or inhibition: conflicting evidence for (±)-catechin's role as a chemical facilitator and disease protecting agent. Plant Signal. Behav. 5, 239–246. doi: 10.4161/psb.5.3.10573
Basu, A., Prasad, P., Das, S. N., Kalam, S., Sayyed, R. Z., Reddy, M. S., et al. (2021). Plant growth promoting rhizobacteria (PGPR) as green bioinoculants: recent developments, constraints, and prospects. Sustainability 13, 3–1140. doi: 10.3390/su13031140
Chakraborty, A. P., Chakraborty, B. N., and Chakraborty, U. (2015). Bacillus megaterium from tea rhizosphere promotes growth and induces systemic resistance in tea against Sclerotium rolfsii. Indian Phytopathol. 68, 237–247. Available online at: https://epubs.icar.org.in/index.php/IPPJ/article/view/49833
Chappelle, E. W., Kim, M. S., and McMurtrey, I. I. I. J. E. (1992). Ratio analysis of reflectance spectra (RARS): an algorithm for the remote estimation of the concentrations of chlorophyll a, chlorophyll b, and carotenoids in soybean leaves. Remote Sens. Environ. 39, 239–247. doi: 10.1016/0034-4257(92)90089-3
Cohen, A. C., Bottini, R., Pontin, M., Berli, F. J., Moreno, D., Boccanlandro, H., et al. (2015). Azospirillum brasilense ameliorates the response of Arabidopsis thaliana to drought mainly via enhancement of ABA levels. Physiol. Plant. 153, 79–90. doi: 10.1111/ppl.12221
Cordero, I., Balaguer, L., Rincón, A., and Pueyo, J. J. (2018). Inoculation of tomato plants with selected PGPR represents a feasible alternative to chemical fertilization under salt stress. JPNSS 181, 694–703. doi: 10.1002/jpln.201700480
Csepregi, K., and Hideg, É. (2018). Phenolic compound diversity explored in the context of photo-oxidative stress protection. PCA 29, 129–136. doi: 10.1002/pca.2720
De-Bashan, L. E., Hernandez, J. P., and Bashan, Y. (2012). The potential contribution of plant growth-promoting bacteria to reduce environmental degradation-a comprehensive evaluation. Agric. Ecosyst. Environ. Appl. Soil Ecol. 61, 171–189. doi: 10.1016/j.apsoil.2011.09.003
Del Rosario Cappellari, L., Chiappero, J., Santoro, M. V., Giordano, W., and Banchio, E. (2017). Inducing phenolic production and volatile organic compounds emission by inoculating Mentha piperita with plant growth-promoting rhizobacteria. Sci. Hortic. 220, 193–198. doi: 10.1016/j.scienta.2017.04.002
Ding, C., Lei, L., Yao, L., Wang, L., Hao, X., Li, N., et al. (2019). The involvements of calcium-dependent protein kinases and catechins in tea plant [Camellia sinensis (L.) O. Kuntze] cold responses. Plant Physiol. Biochem. 143, 190–202. doi: 10.1016/j.plaphy.2019.09.005
FAOSTAT (2021). Available online at: http://www.fao.org/faostat/en/#data/QC
Feng, H., Zhang, N., Du, W., Zhang, H., Liu, Y., Fu, R., et al. (2018). Identification of chemotaxis compounds in root exudates and their sensing chemoreceptors in plant-growth-promoting rhizobacteria Bacillus amyloliquefaciens SQR9. MPMI 31, 995–1005. doi: 10.1094/MPMI-01-18-0003-R
Gouda, S., Kerry, R. G., Das, G., Paramithiotis, S., Shin, H. S., and Patra, J. K. (2018). Revitalization of plant growth promoting rhizobacteria for sustainable development in agriculture. Microbiol. Res. 206, 131–140. doi: 10.1016/j.micres.2017.08.016
Grover, M., Ali, S. Z., Sandhya, V., Rasul, A., and Venkateswarlu, B. (2011). Role of microorganisms in adaptation of agriculture crops to abiotic stresses. World J. Microbiol. Biotechnol. 27, 1231–1240. doi: 10.1007/s11274-010-0572-7
Guebel, D. V., Nudel, B. C., and Giulietti, A. M. (1991). A simple and rapid micro-Kjeldahl method for total nitrogen analysis. Biotechnol. Tech. 5, 427–430. doi: 10.1007/BF00155487
Hafez, E. M., Alsohim, A. S., Farig, M., Omara, A. E. D., Rashwan, E., and Kamara, M. M. (2019). Synergistic effect of biochar and plant growth promoting rhizobacteria on alleviation of water deficit in rice plants under salt-affected soil. Agronomy 9, 12–847. doi: 10.3390/agronomy9120847
Hong, G., Wang, J., Hochstetter, D., Gao, Y., Xu, P., and Wang, Y. (2015). Epigallocatechin-3-gallate functions as a physiological regulator by modulating the jasmonic acid pathway. Physiol. Plant. 153, 432–439. doi: 10.1111/ppl.12256
Ipek, M., Pirlak, L., Esitken, A., Figen Dönmez, M., Turan, M., and Sahin, F. (2014). Plant growth-promoting rhizobacteria (PGPR) increase yield, growth and nutrition of strawberry under high-calcareous soil conditions. J. Plant Nutr. 37, 990–1001. doi: 10.1080/01904167.2014.881857
Kumari, A., Goyal, R. K., Choudhary, M., and Sindhu, S. S. (2016). Effects of some plant growth promoting rhizobacteria (PGPR) strains on growth and flowering of chrysanthemum. J. Crop Weed 12, 7–15.
Kurokura, T., Hiraide, S., Shimamura, Y., and Yamane, K. (2017). PGPR improves yield of strawberry species under less-fertilized conditions. Environ. Control Biol. 55, 121–128. doi: 10.2525/ecb.55.121
Lattanzio, V. (2013). “Phenolic compounds: Introduction,” in Natural Products, eds K. Ramawat and J. M. Mérillon (Berlin; Heidelberg: Springer). doi: 10.1007/978-3-642-22144-6_57
Li, X., Li, Y., Ahammed, G. J., Zhang, X. N., Ying, L., Zhang, L., et al. (2019). RBOH1-dependent apoplastic H2O2 mediates epigallocatechin-3-gallateinduced abiotic stress tolerance in Solanum lycopersicum L. Environ. Exp. Bot. 161, 357–366. doi: 10.1016/j.envexpbot.2018.11.013
Liu, Y., Wu, L., Deng, Z., and Yu, Y. (2021). Two putative parallel pathways for naringenin biosynthesis in Epimedium wushanense. RSC Adv. 11, 13919–13927. doi: 10.1039/D1RA00866H
Lobato-Ureche, M. A., Pérez-Rodriguez, M. M., Ortiz, R., Monasterio, R. P., and Cohen, A. C. (2021). Rhizobacteria improve the germination and modify the phenolic compound profile of pepper (Capsicum annuum L.). Rhizosphere 18, 100334. doi: 10.1016/j.rhisph.2021.100334
Lopes, M. J. S., Dias-Filho, M. B., Castro, T. H. R., and Silva, G. B. (2018). Light and plant growth-promoting rhizobacteria effects on Brachiaria brizantha growth and phenotypic plasticity to shade. Grass Forage Sci. 73, 493–499. doi: 10.1111/gfs.12336
Mierziak, J., Kostyn, K., and Kulma, A. (2014). Flavonoids as important molecules of plant interactions with the environment. Molecules 19, 16240–16265. doi: 10.3390/molecules191016240
Miotto-Vilanova, L., Courteaux, B., Padilla, R., Rabenoelina, F., Jacquard, C., Clément, C., et al. (2019). Impact of Paraburkholderia phytofirmans PsJN on grapevine phenolic metabolism. Int. J. Mol. Sci. 20, 57–75. doi: 10.3390/ijms20225775
Mohanty, P., Singh, P. K., Chakraborty, D., Mishra, S., and Pattnaik, R. (2021). Insight into the role of PGPR in sustainable agriculture and environment. Front. Sustain. Food Syst. 5, 667150. doi: 10.3389/fsufs.2021.667150
Moussi, K., Nayak, B., Perkins, L. B., Dahmoune, F., Madani, K., and Chibane, M. (2015). HPLC-DAD profile of phenolic compounds and antioxidant activity of leaves extract of Rhamnus alaternus L. Ind. Crops Prod. 74, 858–866. doi: 10.1016/j.indcrop.2015.06.015
Pagnani, G., Pellegrini, M., Galieni, A., D'Egidio, S., Matteucci, F., Ricci, A., et al. (2018). Plant growth-promoting rhizobacteria (PGPR) in Cannabis sativa 'Finola' cultivation: an alternative fertilization strategy to improve plant growth and quality characteristics. Ind. Crops Prod. 123, 75–83. doi: 10.1016/j.indcrop.2018.06.033
Pérez-Rodriguez, M. M., Piccoli, P., Anzuay, M. S., Baraldi, R., Neri, L., Taurian, T., et al. (2020). Native bacteria isolated from roots and rhizosphere of Solanum lycopersicum L. increase tomato seedling growth under a reduced fertilization regime. Sci. Rep. 10, 1–14. doi: 10.1038/s41598-020-72507-4
Pérez-Rodriguez, M. M., Pontin, M., Piccoli, P., Lobato Ureche, M. A., Gordillo, M. G., Funes-Pinter, I., et al. (2022). Halotolerant native bacteria Enterobacter 64S1 and Pseudomonas 42P4 alleviate saline stress in tomato plants. Physiol. Plant. 174, e13742. doi: 10.1111/ppl.13742
Rahimi, S., Talebi, M., Baninasab, B., Gholami, M., Zarei, M., and Shariatmadari, H. (2020). The role of plant growth-promoting rhizobacteria (PGPR) in improving iron acquisition by altering physiological and molecular responses in quince seedlings. Plant Physiol. Biochem. 155, 406–415. doi: 10.1016/j.plaphy.2020.07.045
Rani, A., Kumar Vats, S., Sharma, M., and Kumar, S. (2011). Catechin promotes growth of Arabidopsis thaliana with concomitant changes in vascular system, photosynthesis and hormone content. Biol. Plant. 55, 779–782. doi: 10.1007/s10535-011-0187-3
Robledo-Buriticá, J., Aristizábal-Loaiza, J. C., Ceballos-Aguirre, N., and Cabra-Cendales, T. (2018). Influence of plant growth-promoting rhizobacteria (PGPR) on blackberry (Rubus glaucus Benth. cv. thornless) growth under semi-cover and field conditions. Acta Agron. 67, 258–263. doi: 10.15446/acag.v67n2.62572
Samaniego-Gámez, B. Y., Garruña, R., Tun-Suárez, J. M., Kantun-Can, J., Reyes-Ramírez, A., and Cervantes-Díaz, L. (2016). Bacillus spp. inoculation improves photosystem II efficiency and enhances photosynthesis in pepper plants. Chil. J. Agric. Res. 76, 409–416. doi: 10.4067/S0718-58392016000400003
Sarma, B. K., Singh, D. P., Mehta, S., Singh, H. B., and Singh, U. P. (2002). Plant growth-promoting rhizobacteria-elicited alterations in phenolic profile of chickpea (Cicer arietinum) infected by Sclerotium rolfsii. J. Phytopathol. 150, 277–282. doi: 10.1046/j.1439-0434.2002.00755.x
Sarma, B. K., and Singh, U. P. (2003). Ferulic acid may prevent infection by Sclerotium rolfsii in Cicer arietinum. J. Microbiol. Biotechnol. 19, 123–127. doi: 10.1023/A:1023205522032
Saxena, A., Raghuwanshi, R., Gupta, V. K., and Singh, H. B. (2016). Chilli anthracnose: the epidemiology and management. Front. Microbiol. 7, 1527. doi: 10.3389/fmicb.2016.01527
Shiragaki, K., Yokoi, S., and Tezuka, T. (2020). Phylogenetic analysis and molecular diversity of Capsicum based on rDNA-ITS region. Hortic 6, 87. doi: 10.3390/horticulturae6040087
Singh, A., Maurya, S., Singh, R., and Singh, U. P. (2012). Antibiotic potential of plant growth promoting rhizobacteria (PGPR) against Sclerotium rolfsii. Arch. Phytopathol. Plant Prot. 45, 1655–1662. doi: 10.1080/03235408.2012.702460
Tanase, C., Coşarcă, S., and Muntean, D. L. (2019). A critical review of phenolic compounds extracted from the bark of woody vascular plants and their potential biological activity. Molecules 24, 1182. doi: 10.3390/molecules24061182
Vuolo, M. M., Lima, V. S., and Junior, M. R. M. (2019). “Phenolic compounds: structure, classification, and antioxidant power,” in Bioactive Compounds, ed M. R. Segura Campos (Amsterdam: Woodhead Publishing), 33–50.
Wang, P., Liu, Y., Zhang, L., Wang, W., Hou, H., Zhao, Y., et al. (2020). Functional demonstration of plant flavonoid carbocations proposed to be involved in the biosynthesis of proanthocyanidins. Plant J. 101, 18–36. doi: 10.1111/tpj.14515
Keywords: pepper plants, sustainable agriculture, PGPR, Pseudomonas, Cellulosimicrobium, bioinoculants, phenolic compounds
Citation: Lobato-Ureche MA, Pérez-Rodriguez MM, Segura D, Monasterio R and Cohen AC (2023) Pseudomonas 42P4 and Cellulosimicrobium 60I1 as a sustainable approach to increase growth, development, and productivity in pepper plants. Front. Sustain. Food Syst. 6:1111573. doi: 10.3389/fsufs.2022.1111573
Received: 29 November 2022; Accepted: 14 December 2022;
Published: 09 January 2023.
Edited by:
Mohamed Ait-El-Mokhtar, University of Hassan II Casablanca, MoroccoReviewed by:
Sowmyalakshmi Subramanian, McGill University, CanadaCopyright © 2023 Lobato-Ureche, Pérez-Rodriguez, Segura, Monasterio and Cohen. This is an open-access article distributed under the terms of the Creative Commons Attribution License (CC BY). The use, distribution or reproduction in other forums is permitted, provided the original author(s) and the copyright owner(s) are credited and that the original publication in this journal is cited, in accordance with accepted academic practice. No use, distribution or reproduction is permitted which does not comply with these terms.
*Correspondence: Ana Carmen Cohen, YWNvaGVuQGZjYS51bmN1LmVkdS5hcg==
Disclaimer: All claims expressed in this article are solely those of the authors and do not necessarily represent those of their affiliated organizations, or those of the publisher, the editors and the reviewers. Any product that may be evaluated in this article or claim that may be made by its manufacturer is not guaranteed or endorsed by the publisher.
Research integrity at Frontiers
Learn more about the work of our research integrity team to safeguard the quality of each article we publish.