- 1Plant Biology and Conservation, Northwestern University, Evanston, IL, United States
- 2Chicago Botanic Garden, Negaunee Institute for Plant Conservation Science and Action, Glencoe, IL, United States
- 3Department of Biology, Missouri State University, Springfield, MO, United States
- 4School of Environmental Sustainability, Loyola University Chicago, Chicago, IL, United States
Incorporating perennial crops into agroecosystems has been shown to mitigate soil degradation and improve soil health by enhancing soil aggregation and soil organic carbon (SOC) accrual. However, our understanding of the ability and timeframe for perennial crop systems to build soil health within the context of conversion from abandoned crop land remains limited. Here, we examined changes in soil health in the first year following the conversion of an abandoned crop field into an agroecosystem planted with various treatments, including: novel perennial grain (intermediate wheatgrass, IWG; Thinopyrum intermedium), IWG/ alfalfa biculture, forage grass, tallgrass prairie, or annual wheat. We analyzed factors considered central to the concept of mitigating soil degradation to improve soil health (soil aggregation, aggregate organic carbon (OC), bulk SOC) and their soil biological and physicochemical correlates throughout the first growing season. Comparisons between treatments showed that both annual and perennial treatments rapidly and significantly improved soil health metrics including aggregation, aggregate stability, and OC levels compared to pre-conversion conditions. Such increases were positively correlated with the abundance of arbuscular mycorrhizal fungi (AMF hyphae, root colonization), labile SOC and microbial activity. Notably, IWG/ alfalfa biculture resulted in significantly higher levels of macroaggregate OC in comparison to other treatments, including tallgrass prairie, supporting the potential of perennial grasses to contribute to soil carbon gains. Overall, the conversion of this abandoned land to an agroecosystem produced rapid and substantial increases in soil health in the first year after planting.
Introduction
Agricultural practices have contributed to widespread soil degradation by contributing to nutrient loss, erosion, salinity, and compaction and by reducing carbon storage (Lal, 2015). In response, there has been a push to adopt management practices that minimize soil physical disturbances (e.g., no-till), maximize surface coverage (e.g., cover crops), stimulate biological activity (e.g., organic amendments), and use polycultures or perennial crops to regenerate ecosystem services (Sprunger and Robertson, 2018). Of these, planting perennial crops or polycultures is expected to produce soil health benefits comparable to native ecosystems such as enhanced soil stability and increased water and nutrient cycling (Glover et al., 2010; Syswerda et al., 2012; Pugliese et al., 2019; Ledo et al., 2020), but this is not always the case (Johnson et al., 2021). These benefits are largely driven by soil organic carbon (SOC), a factor considered central to the concept of soil health (Bünemann et al., 2018). Establishing perennial crops on agricultural lands can rebuild SOC stocks by 19–39%, especially in the upper soil layers (Post and Kwon, 2010; Ledo et al., 2020), and on short time-scales (within two years; Sprunger and Robertson, 2018; Peixoto et al., 2020). The deep, extensive root systems of perennial species may also create significant subsoil C pools (>2 m depth). Peixoto et al. (2020) reported that perennial crops allocated substantial amounts of fresh root residues and exudates to subsoils (3.6 m deep). In turn, the substrates were metabolized by soil microbial communities and transformed into microbial necromass, a contributor to C stabilization.
Besides C inputs, soil aggregate dynamics influence SOC accrual and thus soil health (Totsche et al., 2018). For example, macroaggregates (>250 μm diameter) are formed as transient organic binding agents (e.g., fine roots, microbial mucilage, arbuscular mycorrhizal fungal (AMF) hyphae) enmesh and bind soil particles and organic matter (Jastrow et al., 2007). Over time, the plant and microbial residues within macroaggregates become encrusted onto mineral surfaces, humify, and condense to form stable organic C (OC)-rich microaggregates (20–250 μm diameter) that contribute to decadal—to century—scale SOC sequestration (Jastrow et al., 2007). Perennialization is thus expected to increase the abundance of macroaggregates and macroaggregate OC in the short-term (Chivenge et al., 2011), and microaggregate OC and SOC pools in the longer term (Virto et al., 2012; Novelli et al., 2017).
At the same time, there is increasing recognition that above- and below-ground components of ecosystems are closely linked (Kardol and Wardle, 2010). For example, plant species may differentially influence soil physicochemical properties including pH, organic matter content, soil structure and microclimate as well as the quality and quantity of root litter and the supply of C to root symbioses (e.g., mycorrhizas, and root exudates that support rhizosphere microbes) (De Deyn et al., 2008). In addition, root growth differs among species (Bergmann et al., 2016) and may physically alter the soil structure to create various physical and metabolic microhabitats (Freschet et al., 2021). Plant species identity may thus be an important consideration in regenerating soil health.
Perennial grasses show promise in land regeneration efforts. One emerging perennial grass species, Thinopyrum intermedium (Host) Barkworth and D.R. Dewey [intermediate wheatgrass (IWG), Kernza®], has the potential to rapidly enhance soil health. In crop fields, studies have reported that IWG may rapidly improve soil quality (Culman et al., 2010, 2013), SOC gain (Sprunger et al., 2017, 2019; Sprunger and Robertson, 2018), and water quality (Culman et al., 2013) in comparison to annual wheat (Triticum aestivum L.), but not always (Syswerda et al., 2011; Sprunger et al., 2018). IWG may have a similarly large potential for regenerating abandoned and/or degraded lands. However, the relative importance of IWG and its interaction with factors at local scales is poorly understood, thereby limiting quantitative predictions of how perennial crops could reverse land degradation.
To address this knowledge gap, we converted an abandoned old field to replicated plots containing IWG (monoculture or biculture with alfalfa—Medicago sativa L.), annual wheat, forage grasses, and tallgrass prairie. Over the first growing season, we measured the abundance of water-stable aggregates (WSA), aggregate and bulk SOC content, and soil biological and physicochemical variables that are expected to contribute to variations in WSA and SOC. Our goals were to: (1) investigate the role of crop type in soil aggregate stability and OC accrual in a newly-established agroecosystem on abandoned land, (2) identify the abiotic or biotic factors that enhanced the formation of water-stable (macro) aggregates, and (3) use these data to test the hypothesis that planting IWG in abandoned fields produces (a) increases in soil health factors that are comparable to those of a perennial tallgrass prairie restoration and (b) more rapid and substantial increases in soil health than those produced by annual crops (wheat, forage grasses).
Materials and methods
Study site and experimental design
The study was conducted in Mettawa, IL (42°14' N, 87°55' W; 191 m a.s.l.) in a field that had last been used for agriculture and pasture ~30 years ago and then abandoned (W. Kurtis; personal correspondence). Since then, the site was mowed several times each year and occasionally used to park cars. The area has a temperate, humid mid-continental climate with average annual minimum and maximum daily temperatures of 4.61°C and 15°C respectively (1991–2020, NOAA), and average annual precipitation is 1003.55 mm, the majority of which is deposited throughout the growing season (April–October). The soils belong to the Nappanee and Montgomery series and are described as deep, somewhat poorly drained silty clay loams with moderate shrink-swell potential (USDA-NRCS, 2019). Prior to conversion, the plant community comprised a mix of native and non-native grasses and forbs, including some aggressive weeds (Supplementary Table S1).
In 2018, the field was treated with two applications of herbicide (Roundup®, active ingredient glyphosate, C3H6NO5P−2). The first application (July) cleared the field of existing plants, while the second (August) reduced emergent weeds from the seedbank. Both applications were applied at a rate of 0.95 liter per acre. During the first week of September prior to planting, the field was rototilled to bare soil to a depth of ~30 cm. The experimental area is ~100 × 30 m and is oriented North-South (Supplementary Figure S1). The relief of the field varies slightly (4 m) and is higher in the south than the north. We initially established 30 plots, each 9 × 9 m, with a 1 m buffer strip around each plot. However, six plots within the northern section of the experimental area were regularly inundated by flooding and therefore removed from the experiment.
We used a randomized block design to account for stochastic effects of slope and spatial heterogeneity across the site (Supplementary Figure S1). We created four blocks, each comprising six plots, that were assigned to one of the following treatments: Thinopyrum intermedium (IWG-TLI 801) provided by The Land Institute, IWG- alfalfa biculture (cv “Kansas Common”; The Land Institute), wheat (Organic Soft Red Winter, LCS 3334; Albert Lea Seed, Albert Lea MN), forage grass (Organic Hay Mix; Albert Lea Seed, MN), or tallgrass prairie (detention basin seed mix; Prairie Moon Nursery, Winona MN). Species' lists for the forage grass and tallgrass prairie treatments are listed in Supplementary Table S2. One plot in each block was left as fallow (5 treatments + 1 fallow = 6 plots per block). For IWG and alfalfa, seeding rates followed The Land Institute's recommendations. For forage grass, annual wheat, and the prairie mix, seeding rates followed the suppliers' recommendations. The seeding rates were 16.82 kg/ha for IWG and alfalfa; 28 kg/ha for the forage grass; 22.40 kg/ha annual wheat; and 10.52 kg/ha for the prairie mix.
Seeding of row-crop treatments (IWG, IWG biculture, annual wheat) was initiated in September 2018 using an EarthWay Precision Garden Seeder (EarthWay Products, Bristol IN) at 1.5–2 cm depth. Each plot contained 29 rows with 30-cm of inter-row spacing; rows were oriented North-South. Plots containing non-row-crop treatments (forage, prairie) were hand-broadcast atop snow in November 2018. Grass seed (“Sunny Mix”; Main St. Seed and Supply, Bay City MI) was sown within buffer strips in April 2019 to limit weed emergence. Weeds were managed periodically in row-crop plots using a wheel-hoe and hand weeding, buffer strips were mowed as needed, and none of the plots received supplemental watering, fertilization, or pesticides from the time of seed sowing until harvest.
Soil sampling
In our site, sampling with a soil corer resulted in compressed soil plugs, and the destructive removal of plugs from the corer altered aggregate abundances. To avoid these problems, samples were collected using a modification of the spade method (Fernández-Ugalde et al., 2020). A V-shaped hole was dug to a depth of 15 cm using a clean trowel and a slice of soil (~3-cm thick) was taken parallel to one of the sides of the hole with the trowel. Samples were collected to 15 cm depth to detect the most rapid transitions in soil aggregation and SOC accrual (Matamala et al., 2008).
Pre-treatment soil samples were collected in Spring 2018 from 20 points distributed across the experimental area; these points roughly corresponded to all blocks and most treatment plots. At each point, three soil samples separated by at least 50 cm were collected, pooled and gently mixed in a 4.5-liter Ziploc™ bag to create one composite bulk sample per point.
In the 2019 growing season, samples were collected three times to coincide with the early plant growth (June), vegetative growth (July), and seed-set in IWG (August). Within each plot, we marked out a central 5 × 5 m area for sampling to reduce edge effects. We collected eight soil samples across two transects within the marked-out area, i.e., one sample every 1.2 m. The eight samples were then pooled in a 4.5-liter Ziploc™ bag to create one composite sample per plot. After collection, the samples were stored in coolers and transported back to the laboratory. Each soil sample was passed through an 8-mm sieve to remove coarse debris and gently homogenized. A sub-sample of fresh soil was removed from each bag for analysis of labile SOC and microbial activity (see Soil analyses) and gravimetric soil moisture, expressed as percent difference in weight between field moist and oven dried soils (90°C, 48 h). The remaining soil was air-dried and stored at room temperature (23°C) before analysis for soil physical properties (texture, WSA), SOC, pH, nutrient levels (inorganic N, P), and AMF hyphal length and AMF colonized of fine roots.
Soil analyses
Soil texture was determined using the micropipette method (Miller and Miller, 1987). Overall, soils across the experimental site comprised 25% sand, 62% silt, 13% clay, which is texturally-classed as a silt loam (USDA-NRCS, 2019). Soil aggregates were extracted from each sample using slaking and wet sieving on a set of nested sieves: 2000 μm (large macroaggregates; >2000 μm diameter), 250 μm (small macroaggregates; 250–2000 μm), and 53 μm (microaggregates, 53–250 μm; Tisdall and Oades, 1982). Aggregate fractions on each sieve were dried (80°C) for 48 h and weighed, and aggregate stability calculated as the mean weight diameter (MWD; Kemper and Rosenau, 1986).
Inorganic N and P were extracted in deionized water (1: 10 w/v, soil: water; pH 6.8) by vigorous shaking for 30 min. Extracts were filtered and analyzed colorimetrically on a microplate spectrophotometer (Biotek Epoch, Winooski, VT) using the vanadium reduction method for NO3 (Doane and Horwáth, 2003), phenol-hypochlorite method for NH4 (Weatherburn, 1967), and the malachite green method for PO4 (Baykov et al., 1988). Soil pH was measured in 1:5 soil: water (v/v) using a pH probe (Fisher Scientific). SOC was measured on finely ground bulk soil samples and aggregate fractions by combustion using a Leco TruSpec™ CN Elemental Analyzer (Leco Corp., St. Joseph, MI). Bulk SOC values are expressed as % soil dry weight. Aggregate fractional OC was corrected for sand content and expressed a grams OC per kg soil.
Two methods were used to quantify AMF abundance. First, AMF external hyphae were extracted in 5% (w/v) sodium hexametaphosphate and filtered onto gridded membrane (Jakobsen et al., 1992), and viewed and scored using a Leica DMLB LB30T microscope (400 × mag). Glomeromycotan hyphae were quantified over 50 fields of view for each sample, and AMF hyphal length was calculated using the method of Newman (1966). We defined AMF hyphae as non-septate or irregularly septate hyphae with characteristic unilateral elbow-like projections; all other hyphae were categorized as non-AMF hyphae. Second, we quantified AMF colonization in fine roots. Fine roots were manually picked from each soil sample, washed to remove adhering soil and then stained using methods described by Koske and Gemma (1989). Stained root samples were mounted in polyvinyl alcohol-lactic acid-glycerol (PVLG), and viewed and scored on a Leica DMLB LB30T florescence microscope (400 × magnification) for AMF root colonization using the line intersect method (Tennant, 1975). Fifty fields of view were examined in each sample for the presence or absence of fungal structures unique to Glomeromycota (hyphae, vesicles, arbuscules, and coils) as well as saprophytic fungi. Counts were converted to percentage of root length colonized by AMF structures.
Microbial activity was determined from the flush of CO2 following the addition of labile C source (sucrose) to field moist soils (i.e., substrate induced respiration, SIR; Degens and Harris, 1997) and analyzed by the NaOH trap-titration method (Franzluebbers, 2016). Readily soluble (labile) SOC pools were extracted from moist field soils with 0.5 M K2SO4 by shaking for 30 min. Filtered extracts were analyzed using the phenol-sulfuric method in microplate format (Masuko et al., 2005). Soil MBC was measured using the fumigation–extraction method and calculated as the difference between fumigated and non-fumigated samples divided by kc, the extraction efficiency coefficient (kc = 0.45; Vance et al., 1987).
Plant analyses
Plant tissue was analyzed at two time points: pre-treatment (oldfield) and July 2019, during crop vegetative growth. For the oldfield samples, leaves were clipped from plants adjacent to the soil sampling point. In July 2019, we collected and pooled the four uppermost leaves from >20 individual plants in each pasture grass, IWG, IWG biculture, and annual wheat plot. Leaf samples were dried (60°C, 72 h), finely ground, and analyzed by combustion for C and N content as described for soil and aggregate samples.
Statistical analyses
All statistical analyses were performed using R version 4.1.3 (R Core Team, 2017) assuming an alpha = 0.05 level of statistical significance. Assumptions of normality were assessed via Shapiro-Wilks normality test and residual diagnostic plots; no data transformations were required prior to statistical analysis. First, we used repeated-measures analysis of variance (ANOVA; lme4 package) to compare aggregate abundance, aggregate OC levels, and abiotic and biotic soil factors between pre-conversion (2018) and first year (2019) samplings.
Next, we used a mixed effect model to test the effect of individual treatments and sampling date (June–August) on the abundance of each aggregate size fraction, bulk SOC, aggregate OC levels, and abiotic and biotic soil factors (2019). Treatment type and sampling date were treated as fixed effects and block was a random effect. We also analyzed the data set by comparing the effects of mono- vs. polyculture crops, and perennial (IWG, IWG biculture, prairie) vs. annual (wheat) or mixed crops (forage) on soil properties. For analyses with significant outcomes, Tukey's Honestly Significant Differences (HSD) for multiple comparisons test was used to determine differences among crop treatments or sampling times (Hmisc package).
Finally, we identified the soil abiotic and biotic correlates of aggregate abundance and OC levels in 2019, especially those that had positive effects. Preliminary analyses showed that soil moisture was significantly correlated (Spearman rank, rs) with many factors including aggregate abundance, AMF hyphal length, and SIR (Supplementary Table S3). As a result, we used a mixed effect model with soil moisture and sampling date as random effects and individual soil factors as fixed effects. A marginal r2 value was calculated for individual soil factors as a way to quantify their effect(s) on aggregation and aggregate OC that were not explained by soil moisture (MuMIN package, rsquared.glmm function).
To summarize and visualize the overall effect of crop treatment or sampling time on soil properties, we analyzed data using non-metric multidimensional scaling (NMDS) on a Bray-Curtis dissimilarity matrix and used k-means clustering to assign samples to clusters. Permutational multivariate analysis of variance (PERMANOVA, 999 permutations) was used to determine significance differences between clusters, while pairwise differences between clusters were tested using pairwise PERMANOVA (RVAideMemoire package). Results were visualized using corrplot, ggpubr, and ggplot2 packages.
Results
Effects of land conversion on soil properties
Site conversion resulted in significant shifts in most soil properties (Figure 1; Supplementary Table S4), including WSA (p < 0.001), aggregate OC (p < 0.003), microbial factors (p < 0.03, except AMF root vesicles), and soil nutrient levels (p < 0.001). We found large and significant increases in the abundance and OC content in each aggregate fraction (p < 0.001; Figures 1A,B), as well as microbial activity including AMF hyphal length (p < 0.001), microbial biomass (MBC; p = 0.03) and respiration (SIR; p < 0.001; Figure 1C). Conversely, crop establishment resulted in significant reductions in soil moisture (39 ± 3–18 ± 0.4%, mean ± se; p < 0.001; Supplementary Table S4) and plant-available N and P (p < 0.001; Figure 1D), consistent with the uptake of soil resources necessary for plant productivity. Bulk SOC declined significantly following conversion (p < 0.001) whereas bulk %N increased significantly (p < 0.001; Figure 1E). There were also significant shifts in bulk soil and aggregate C:N (p < 0.001; Figure 1F). Pre-treatment bulk soil and aggregate C:N levels were within the range of oldfield leaf C:N levels (mean 19, range 14–41). Crop establishment resulted in a decline in both bulk soil and aggregate C:N (C:N 4–7), especially in comparison to leaf C:N in IWG, wheat, and pasture grass leaves (C:N 17.9 ± 1.4) and alfalfa (C:N 12 ± 0.5).
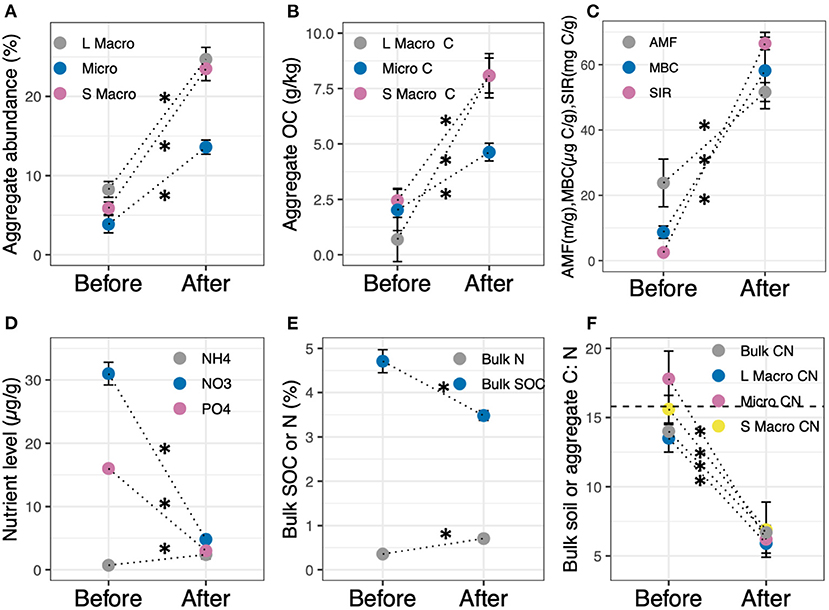
Figure 1. Levels of (A) WSA, (B) aggregate OC, (C) microbial factors, (D) plant-available N and P, (E) bulk soil C and N, and (F) bulk soil and aggregate C:N before and after site conversion. Horizontal line in (F) denotes plant C:N. Vertical bars indicate the standard error of the mean. For each soil property, means denoted * differ significantly before and after conversion at p < 0.05.
Effects of crop systems on soil properties
While land conversion significantly affected soil properties across the site, the only soil health properties that were shown to be significantly affected by cropping systems were small macroaggregate OC content (Figure 2) and AMF root colonization (Supplementary Table S5). Small macroaggregate OC levels were higher under biculture plots than other crop systems in July and August (p < 0.05), including the prairie treatment. These increases were significantly and positively correlated with soil pH and AMF root colonization (p < 0.05; Figure 3). AMF root colonization was highest in IWG (93.6 ± 2% root colonized) and forage plots (93.2 ± 2%) and lowest in the prairie plots (49.8 ± 2%; p < 0.002). There was no significant difference in other soil factors by cropping system alone (Supplementary Table S5) or cropping system over time (p > 0.05). The NMDS analyses also supported similar levels of soil health under the different crop systems (PERMANOVA, p = 0.897). All crop treatments were distributed across NMDS space and detected in every cluster (Figure 4A). This pattern persisted even when crops were classed and analyzed as mono- vs. polycultures, or annual vs. perennial crops (Supplementary Figure S2; Supplementary Table S5).
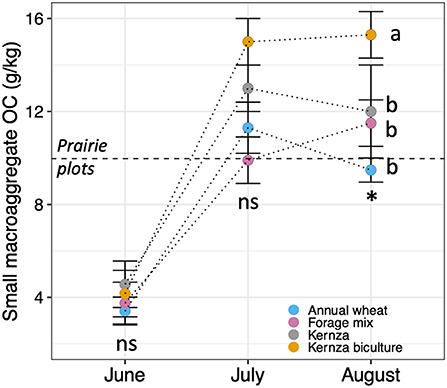
Figure 2. Temporal variation in small macroaggregate OC under IWG (Kernza), IWG biculture, annual wheat or forage mix in the first year after site conversion. Horizontal line in denotes prairie plot small macroaggregate OC. Vertical bars indicate the standard error of the mean. For each month, ns, means do not differ significantly (p > 0.05), * means differ at p < 0.05. Within each month, crops with same letter do not differ significantly at p < 0.05.
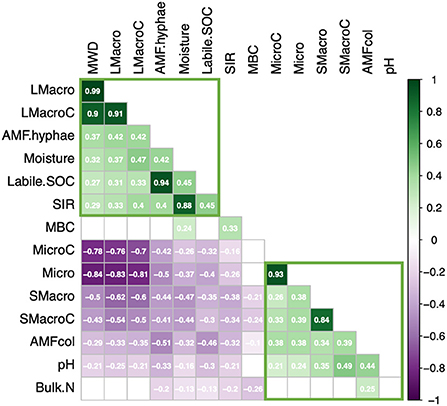
Figure 3. Summary of Spearman rank (rs) correlation analyses between WSA and aggregate OC and significant edaphic correlates (p > 0.05). Values within each square denote the value of rs with positive correlations in green and negative correlations in purple. Color intensity is proportional to the correlation coefficient. Non-significant correlations are left blank. LMacro, large macroaggregate abundance; LMacroC, large macroaggregate OC; AMF.hyphae, AMF hyphal length; SIR, substrate-induced respiration; MBC, microbial biomass C; MicroC, microaggregate OC; Micro, microaggregate abundance; Smacro, small macroaggregate abundance; SMacroC, small macroaggregate abundance; AMFcol, AMF root length colonized.
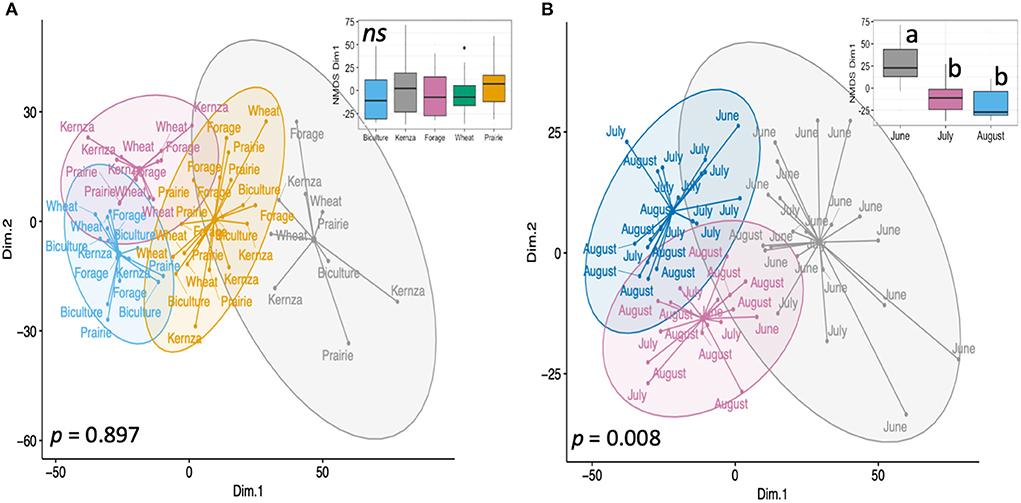
Figure 4. Non-metric Multidimensional Scaling (NMDS) ordination of soil properties with fitted k-means cluster ellipses to illustrate relationships between (A) crop treatment, and (B) sampling month. p-value by PERMANOVA.
Temporal patterns of WSA abundance and OC
Temporal patterns in the abundance and OC level differed by aggregate size (Figures 5A,B; Supplementary Table S6). Site conversion initially promoted the development of macroaggregates, with the highest abundance of large macroaggregates in June (p < 0.001; Figure 5A). In turn, the increased mass of soil within this fraction drove a significant increase in MWD from 0.46 ± 0.08 mm (Pre-conversion) to 2.34 ± 0.19 mm, indicating a substantial gain in soil stability (p < 0.001; Supplementary Tables S4, S6). However, these increases were short-lived. In July and August, large macroaggregate abundance declined significantly whereas the abundances of small macro- and microaggregates increased significantly (p < 0.001; Figure 5A). The mass loss from the large macroaggregates was equivalent to the mass gain in the smaller aggregates (Supplementary Table S6), meaning that large macroaggregates were disrupted into their constituent small macro- and micro-aggregates.
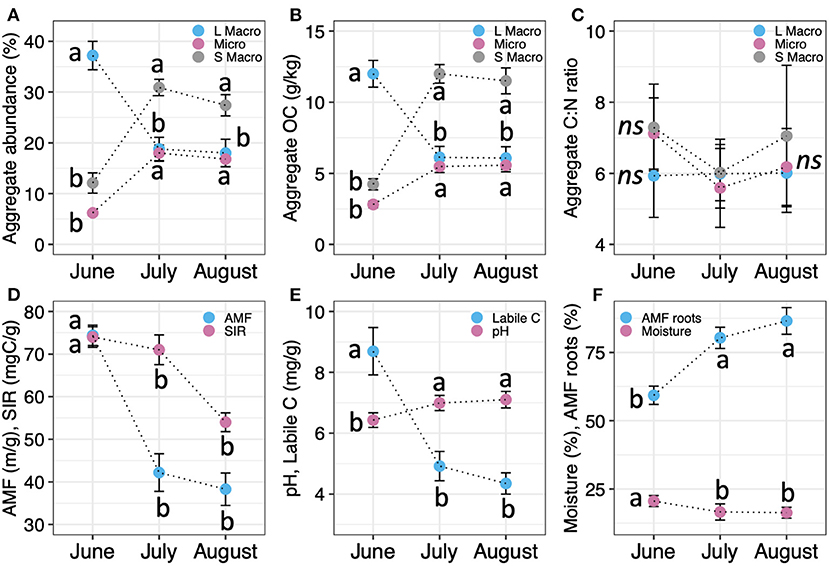
Figure 5. Temporal variation in (A) WSA abundance, (B) aggregate OC, (C) aggregate C:N; (D) AMF hyphal length and SIR, (E) pH and labile SOC levels; and (F) moisture and AMF root colonization after site conversion. Vertical bars indicate the standard error of the mean. For each soil factor, points with the same letter do not differ significantly at p < 0.05.
Similar patterns were detected in aggregate OC levels. Although site conversion resulted in significant increases in aggregate OC across all WSA fractions (Figure 5B), the largest increase was detected within the large macroaggregate fraction in June (p < 0.001). Disruption of large macroaggregates in July resulted in the release of OC-rich small macro- and micro-aggregates and depletion of the large macroaggregate OC pool. Even so, the gain in OC in the small macro- and micro-aggregate fractions (~10 g C kg−1 soil) more than compensated for the OC loss in the large macroaggregate fraction (6 g C kg−1 soil). However, there was no change in aggregate C:N (Figure 5C).
NMDS analyses confirmed the significant effect of sampling time (PERMANOVA, p = 0.008; Figure 4B) and separated the sampling times into two groups: July and August were clustered together (pairwise PERMANOVA, p = 0.057), and significantly different from June (pairwise PERMANOVA, p = 0.001). These groups were separated along NMDS1, which corresponds to a gradient of increasing soil physical stabilization and protection of organic matter, i.e., increasing large macroaggregate abundance and OC, MWD, AMF hyphal length, and labile C. NMDS2 corresponds to a gradient of increasing macroaggregate abundance within each month.
Covariates of WSA abundance and OC
Most soil factors were significantly positively or negatively correlated with soil moisture (Figure 3), including large macroaggregate abundance and OC (positive) and small macro- and micro-aggregate abundance and OC (negative; Supplementary Table S7). After accounting for the effects of soil moisture, mixed models showed that large macroaggregate abundance and OC level were significantly and positively correlated with AMF hyphal length, SIR and labile SOC, with the largest effect size () associated with AMF hyphal length (Table 1; Figure 3; Supplementary Figure S3). These properties were also strongly inter-correlated among themselves (Figure 3) and with MWD (e.g., AMF hyphal length).
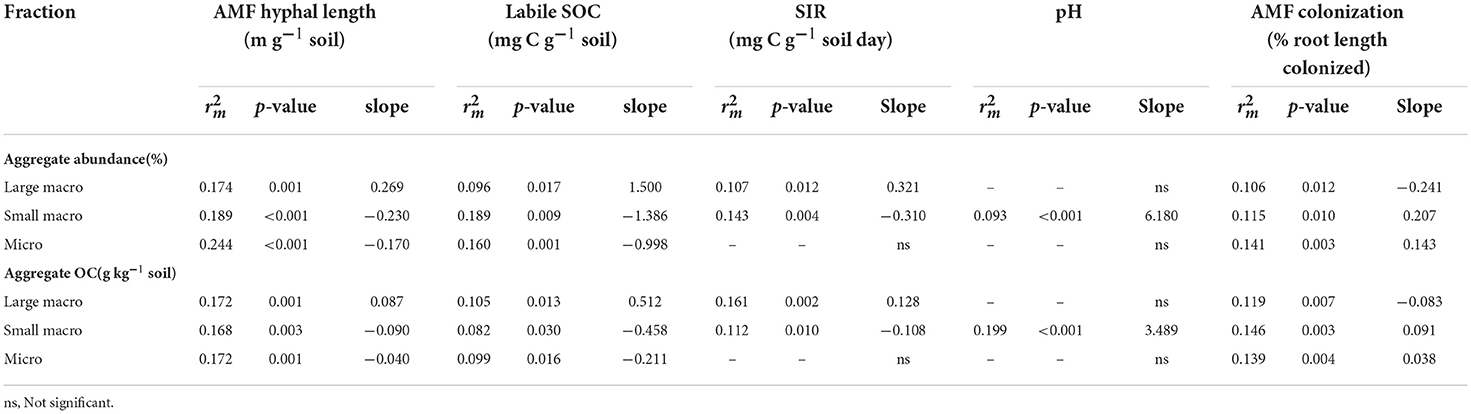
Table 1. Significant correlates of aggregate abundance and aggregate OC level expressed as marginal and slope of the line.
Small macro- and micro-aggregate abundance and OC levels were also significantly correlated with AMF hyphal length, labile SOC, and SIR (p < 0.03; Table 1; see also Figures 5D,E) but the direction of response was opposite to large macroaggregates (i.e., negative) since small macro- and micro-aggregates were the result of large macroaggregate disruption. Instead, small macroaggregate abundance and OC levels were positively correlated with AMF root colonization and soil pH (Table 1; Figures 3, 5F; Supplementary Figure S3). However, soil pH was negatively correlated with AMF hyphal length and SIR (Figure 3). WSA abundances and OC levels were not related to soil sand, clay or silt content, plant-available N and P levels, or bulk SOC and %N (Supplementary Table S7).
Discussion
In the context of calls to transition annual grain agriculture to perennial grain agriculture (Crews et al., 2018), we initially hypothesized that converting an old field to perennial IWG would produce increases in soil health comparable to prairie habitat and greater than annual crops (wheat, forage grasses). In support of this hypothesis, we found a large positive effect of IWG biculture and, to a lesser extent IWG alone, on small macroaggregate OC levels. Recent studies have also found promising soil health effects of IWG compared with annuals (e.g., Audu et al., 2022; Martin and Sprunger, 2022). However, our findings must be tempered by the observation that both annual and perennial crops encouraged other early improvements in soil health. Both annual and perennial systems resulted in rapid and substantial increases in macro- and micro-aggregate abundance, stability (MWD) and OC relative to pre-conversion levels and belowground allocation that replenished AMF abundance and microbial activity.
Establishment of both annual and perennial systems resulted in soils dominated by large (>2 mm), relatively unstable macroaggregates. The underlying factor(s) responsible for large macroaggregates were likely AMF hyphal abundance and labile SOC, factors that have been extensively discussed as key drivers of aggregation (Rillig et al., 2015). For example, AMF hyphae physically enmesh soil particles to promote macroaggregate formation (Six et al., 2006). Labile SOC, which includes water-soluble polysaccharides exuded by roots or released by microbial decomposition of green residues, provides the glue or binding agents that stabilize macroaggregates and induces fungal growth (Baumert et al., 2018). Nevertheless, these are transient binding agents and prone to degradation by environmental conditions (Cambardella and Elliot, 1993).
Indeed, the initial increase in large macroaggregate abundance was followed by disruption as evidenced by the loss in large macroaggregate abundance, reduced MWD, and a concomitant increase in the abundance of smaller aggregate subunits. Larger aggregates are generally considered more susceptible to disruption than smaller aggregate fractions (Tisdall and Oades, 1982; Cambardella and Elliot, 1993). In our study, large macroaggregate abundance was strongly and positively correlated with soil moisture. One possibility is that macroaggregate failure may have occurred naturally upon soil drying or with wet-dry cycles (Amézketa, 1999; Denef et al., 2001). The large biomass of the new crops may have intensified wetting-drying cycles owing to water uptake by roots (Amézketa, 1999) or amplified mechanical stresses via root penetration of micro-scale structures (Angers and Caron, 1998). Soil moisture may also control aggregation via its influence on biotic mechanisms, including the microbial decomposition of plant residues (Baumert et al., 2018) and/or production of polysaccharides required for aggregate stabilization (Regelink et al., 2015); the correlations between soil moisture and microbial factors in our study support this possibility. However, further experiments are needed to partition the relative contributions of plant roots, local soil hydrologic functions, and microbes in WSA stability.
Small macroaggregate abundance and OC were positively correlated with soil pH, independent of any pH effects on large macroaggregates. In part, this result suggests a direct and abiotic cause for the formation of smaller macroaggregates, possibly through chemical or electrostatic interactions (Denef et al., 2002). Another possibility is that interactions between soil pH and microbial properties (AMF, SIR) influenced aggregate OC pools indirectly by altering the contribution of microbial necromass in aggregates (Yang et al., 2022) or pH-induced changes in microbial community composition (Bååth and Anderson, 2003).
Even allowing for large macroaggregate disruption, land use change achieved substantial increases in aggregate OC, implying a positive scenario for soil health restoration as noted by Sprunger and Robertson (2018). We detected two AMF factors that explained the increases in large and small macroaggregate OC. Large macroaggregate OC was best correlated with AMF hyphal abundance. AMF hyphae play substantial roles in SOC sequestration by forming a surface for mineral and SOM adsorption (Totsche et al., 2018) and the transfer of plant root-derived OC into aggregates (Frey et al., 2003; Kallenbach et al., 2016). In addition, AMF-colonized fine roots were the main mechanism for improving small macroaggregate OC levels. Fine roots can physically entangle soil particles meaning that sloughed cell walls, root hairs, mucilages, and cell debris, including AMF structures, may have been incorporated into aggregates (von Lützow et al., 2006). In our study, the contribution of AMF root fragments to aggregate OC likely reflects the greater root biomass and associated increases in AMF biomass and activity following site conversion. Other microbial factors were also important.
Materials of microbial origin, including polysaccharides, may have contributed to aggregate OC levels. These inputs can be deduced from WSA C:N levels, all of which appear to be close to the C:N stoichiometry of the soil microbial biomass. In pre-conversion soils, WSA C:N (C:N 16) largely mirrored the old-field plant community indicating plant debris to be the major source of C. Following site conversion, however, the C:N ratio of WSA fractions declined to ~4–7, which is consistent with a substantial contribution from root exudates or labile, microbially-derived substrates to aggregate OC (bacteria C:N 6, fungi C:N 5–17; Cleveland and Liptzin, 2007) and that SOC is largely dependent on inputs of microbial origin (Yang et al., 2022). This is reasonable given that the addition of organic matter to soils stimulated microbial activity (SIR), and macroaggregates form around fresh residues and become enriched in labile (low C:N) substrates derived from the microbial decomposition of residues (Jastrow et al., 2007). The narrow C:N coupled with increasing total %N over time also indicates a substantial contribution of N-containing materials including extracellular polymeric substances (EPS, glycoproteins) that are major agents for aggregating mineral particles and binding OM onto mineral surfaces (Kleber et al., 2007). Taken together, these observations suggest that all treatments resulted in high C inputs with relatively fast decomposition rates (Vesterdal et al., 2002).
Despite the large increases in aggregate-held OC, plant productivity, and organic matter inputs across all treatments, site conversion resulted in a loss of bulk SOC levels. In part, tillage likely forced the physical destruction of existing soil aggregates and the rapid turnover of SOC by soil microbes (Six et al., 2006; Sprunger and Robertson, 2018). Another possibility is that increased availability of easily degradable OC, e.g., labile C or microbial residues, may have initiated the “priming effect” (Blagodatskaya and Kuzyakov, 2008; Kuzyakov, 2010). Because soil microbes are generally C limited (Cleveland and Liptzin, 2007), large inputs of fresh labile C from the new crops may have stimulated the microbial decomposition of SOM, as indicated by the increased SIR. Under these conditions, macroaggregates may also be more prone to enhanced degradation, which may explain the tendency of large macroaggregates to disaggregate into smaller aggregate fractions in this study site. While observations of substantial increases in labile C, microbial biomass and soil respiration (SIR) after planting may be consistent with priming, more detailed studies are needed to address this possibility.
Implications
Our findings show that the first year of annual or perennial plant establishment substantially and rapidly improved soil aggregation and aggregate-associated OC, two key components of soil health (Golchin et al., 1994; Six et al., 2004; Chivenge et al., 2011; Lal, 2015; Sprunger and Robertson, 2018). The most effective and sensitive integrators of these processes were AMF and labile C. Specifically, AMF hyphae in concert with labile C were the main factors correlated with improved aggregate abundance and stability, while AMF hyphae and AMF-colonized root fragments were of paramount importance in increasing macroaggregate OC over time. This result confirms the importance of AMF in soil health, as has been noted elsewhere (e.g., Rillig et al., 2015). The link between labile C and aggregation, however, brings focus to the importance of the active soil C pool in soil health. This pool fuels microbial metabolism and the subsequent production of aggregate-binding agents and necromass (Peixoto et al., 2020), interactions that are key to stabilizing C. Recently, Martin and Sprunger (2022) noted that soil health indicators reflecting labile C pools were sensitive to temporal fluctuations in soil health under annual vs. perennial crops. Measurements of AMF and labile C should therefore be considered for future assessments of soil health.
On the other hand, it was difficult to detect changes in soil health induced by the different plant systems. Only small macroaggregate OC emerged as a signal of management effects (Sprunger et al., 2018) despite the documented sensitivity of other indicators, such as labile C, to management effects (Xia and Wander, 2022). The contradictory findings in this and other studies (e.g., Sprunger et al., 2018) may reflect high spatial variability levels of soil properties across the plots that masked the effects of crop systems (De et al., 2020) or it may reflect differences in establishment methods (especially since this is a one-year study). There is also growing evidence that soil enzyme activities related to nutrient cycling (i.e., N, P), as well as analyses of readily decomposable pools of SOM, such as permanganate-oxidizable C (reactive carbon), may have potential as early and more sensitive indicators of soil ecological restoration (Martin and Sprunger, 2022; Xia and Wander, 2022). Although these factors comprise a relatively small fraction of SOM, they have turnover rates of weeks to months and may be more sensitive to soil health changes with management and land use practices.
Detecting active soil health recovery may also depend on the depth of measurement. We analyzed soils within the top 15 cm of soil profile, where the expansive shallow root systems of plants are expected to readily replenish SOC (Matamala et al., 2008; Syswerda et al., 2011; Jaikumar et al., 2012). Future analyses may need to consider the depth distribution of soil health attributes, such as SOC, associated with the deep roots of perennial plants and their capacity to deliver organic matter inputs at depth (DuPont et al., 2014; Peixoto et al., 2020).
Further, we compared crops established by seeding (annual, perennial crops) vs. broadcasting (forage, prairie). While we used cultivation methods typical of current practices, our approach may have inadvertently constrained the net positive effects of kernza mono- and bicultures on soil health. For example, the mean root biomass in kernza mono- and bi-cultures (73 ± 17 g/m2) was lower than in forage (143 ± 91 g/m2) and prairie plots (112 ± 12 g/m2; E. Kilbane and R. Dybzinski, unpublished data). Even so, kernza mono- and bi-cultures and forage grasses showed similar levels of aggregation. Thus, integrating plant and soil properties by scaling the levels of aggregate OC per unit root biomass (or other plant functional trait) may provide a more nuanced indicator of land-use change on soil health than soil properties alone.
Finally, it has yet to be seen whether perennial agroecosystems can retain as much or greater C stocks as restored prairies. In our study, the IWG and biculture treatments showed promise for soil C accrual. This is consistent with soil resilience, or the capacity of soil to recover after disturbance. However, our study only reports the results of the first growing season, and it is yet to be seen whether IWG (or other crops) can continue to improve SOC levels in the second and subsequent years after crop establishment in our site; work is in progress to test this possibility. Like tallgrass prairie restorations, perennial crops may require time to fully develop differences in aggregation and OC accrual following conversion to an agroecosystem (Virto et al., 2012; Anderson-Teixeira et al., 2013; Novelli et al., 2017; De et al., 2020). Both experimental studies (Steinbeiss et al., 2008) and meta-analyses (Deng et al., 2014) demonstrated that restoration age was the most important factor influencing soil C stocks whereby C accrual increases with site age. This is consistent with the observations that perennial taxa require more time to develop an extensive root system that re-establishes nutrient and water cycling, microbial community succession and metabolism in tandem with the absence of physical disruptions such as tilling (Matamala et al., 2008; DuPont et al., 2014). Further, plant species diversity may be more important in increasing soil C stocks than rooting depth (Steinbeiss et al., 2008) owing to differences in plant root traits (Freschet et al., 2021). If this is the case in our study system, SOC accrual may accelerate over time in tallgrass prairie plots (vs. crops) with the build-up of new OC pools. Taken together, we suggest that determining which factors drive soil health after site conversion to perennial agroecosystems requires long-term monitoring, a consideration of crop species biomass and diversity, and a more nuanced approach to soil property measurements.
Data availability statement
The original contributions presented in the study are included in the article/Supplementary material, further inquiries can be directed to the corresponding author.
Author contributions
LC, RD, NZ, and LE-W conceived, designed, and installed the experimental plots and procured funding for the research. LC, TA, RD, and LE-W analyzed samples, and analyzed and interpreted the data. LC wrote the first draft of the manuscript. All authors contributed to editing the manuscript and approved the final submission.
Funding
This study was supported by research funds from the Finite Earth Initiative (Northwestern University), the National Science Foundation (DBI-1757800), and the Kurtis Conservation Foundation.
Acknowledgments
We thank Tim Crews and The Land Institute for providing seed, planting advice and feedback on the study, and our research team for their help in the field and the lab: Erin Kilbane, Lindsey Gohd, Ellinore Porter, Megan Wei, Anna Wang, Ben Sanchez, Ben Wyatt, and Olivia Niosi. We are indebted to Bill Kurtis, Donna LaPietra, and the Kurtis Conservation Foundation for their generosity and support of the research.
Conflict of interest
The authors declare that the research was conducted in the absence of any commercial or financial relationships that could be construed as a potential conflict of interest.
Publisher's note
All claims expressed in this article are solely those of the authors and do not necessarily represent those of their affiliated organizations, or those of the publisher, the editors and the reviewers. Any product that may be evaluated in this article, or claim that may be made by its manufacturer, is not guaranteed or endorsed by the publisher.
Supplementary material
The Supplementary Material for this article can be found online at: https://www.frontiersin.org/articles/10.3389/fsufs.2022.1010298/full#supplementary-material
Supplementary Figure S1. (A) Location of study plots in northeastern Illinois; (B) aerial view of study plots(courtesy Google Earth), each plot measures 9 × 9 m with a 1 m wide aisle between plots; (C) plot view north in spring; (D) plot view north in late summer.
Supplementary Figure S2. NMDS output based: (A) crop treatment as annual, perennial or mixed; (B) mono- or poly-culture status; (C) crop treatment; (D) month. Ellipses based on k-means clustering, p-value byPERMANOVA.
Supplementary Figure S3. Significant correlations between large macroaggregate abundances and (A) AMF hyphal length, (B) labile C, and (C) SIR, and (D) small macroaggregates and soil pH.
Supplementary Table S1. Pre-treatment beta-diversity.
Supplementary Table S2. Seed mix used for tall grass prairie treatment.
Supplementary Table S3. Correlations between soil moisture and soil properties.
Supplementary Table S4. Summary of soil properties before and after site conversion.
Supplementary Table S5. Summary of soil properties by crop treatment.
Supplementary Table S6. Summary of soil properties between months.
Supplementary Table S7. Summary of correlation analyses between aggregate abundance and OC level and soil properties.
References
Amézketa, E. (1999). Soil aggregate stability: a review. J. Sustain. Agric. 14, 83–151. doi: 10.1300/J064v14n02_08
Anderson-Teixeira, K. J., Masters, M. D., Black, C. K., Zeri, M., Hussain, M. Z., Bernacchi, C. J., et al. (2013). Altered belowground carbon cycling following land-use change to perennial bioenergy crops. Ecosystems 16, 508–520. doi: 10.1007/s10021-012-9628-x
Angers, D. A., and Caron, J. (1998). Plant-induced changes in soil structure: processes and feedbacks. Biogeochemistry 42, 55–72. doi: 10.1023/A:1005944025343
Audu, V., Rasche, F., Dimitrova Mårtensson, L.-M., and Emmerling, C. (2022). Perennial cereal grain cultivation: implication on soil organic matter and related soil microbial parameters. Appl. Soil Ecol.174:104414. doi: 10.1016/j.apsoil.2022.104414
Bååth, E., and Anderson, T. H. (2003). Comparison of soil fungal/ bacterial ratios in a pH gradient using physiological and PLFA-based techniques. Soil Biol. Biochem. 35, 955–963. doi: 10.1016/S0038-0717(03)00154-8
Baumert, V. L., Vasilyeva, N. A., Vladimirov, A. A., Meier, I. C., Kögel-Knabner, I., and Mueller, C. W. (2018). Root exudates induce soil macroaggregation facilitated by fungi in subsoil. Front. Environ. Sci. 6:140. doi: 10.3389/fenvs.2018.00140
Baykov, A. A., Evtushenko, O. A., and Avaeva, S. M. (1988). A malachite green procedure for orthophosphate determination and its use in alkaline phosphate-based enzyme immunoassay. Anal. Biochem. 171, 266–270. doi: 10.1016/0003-2697(88)90484-8
Bergmann, J., Verbruggen, E., Heinze, J., Xiang, D., Chen, B., Joshi, J., et al. (2016). The interplay between soil structure, roots, and microbiota as a determinant of plant–soil feedback. Ecol. Evol. 6, 7633–7644. doi: 10.1002/ece3.2456
Blagodatskaya, E., and Kuzyakov, Y. (2008). Mechanisms of real and apparent priming effects and their dependence on soil microbial biomass and community structure: critical review. Biol.Fertil. Soils 45, 115–131. doi: 10.1007/s00374-008-0334-y
Bünemann, E. K., Bongiorno, G., Bai, Z., Creamer, R. E., De Deyn, G., de Goede, R., et al. (2018). Soil quality—a critical review. Soil Biol. Biochem. 120, 105–125. doi: 10.1016/j.soilbio.2018.01.030
Cambardella, C. A., and Elliot, E. T. (1993). Carbon and nitrogen distribution in aggregates from cultivated and native grassland soils. Soil Sci. Soc. Am. J. 57, 1071–1076. doi: 10.2136/sssaj1993.03615995005700040032x
Chivenge, P., Vanlauwe, B., Gentile, R., and Six, J. (2011). Organic resource quality influences short-term aggregate dynamics and soil organic carbon and nitrogen accumulation. Soil Biol. Biochem. 43, 657–666. doi: 10.1016/j.soilbio.2010.12.002
Cleveland, C. C., and Liptzin, D. (2007). C:N:P stoichiometry in soil: is there a “Redfield ratio” for the microbial biomass? Biogeochemistry 85, 235–252. doi: 10.1007/s10533-007-9132-0
Crews, T. E., Carton, W., and Olsson, L. (2018). Is the future of agriculture perennial? Imperatives and opportunities to reinvent agriculture by shifting from annual monocultures to perennial polycultures. Glob. Sustain. 1:e11. doi: 10.1017/sus.2018.11
Culman, S. W., Snapp, S. S., Ollenburger, M., Basso, B., and DeHann, L. R. (2013). Soil and water quality rapidly responds to the perennial grain kernza wheatgrass. Agron. J. 105, 735–744. doi: 10.2134/agronj2012.0273
Culman, S. W., Young-Mathews, A., Hollander, A. D., Ferris, H., Sánchez-Moreno, S., O'Geen, A. T., et al. (2010). Biodiversity is associated with indicators of soil ecosystem functions over a landscape gradient of agricultural intensification. Landsc. Ecol. 25, 1333–1348. doi: 10.1007/s10980-010-9511-0
De Deyn, G. B., Cornelissen, J. H., and Bardgett, R. D. (2008). Plant functional traits and soil carbon sequestration in contrasting biomes. Ecol. Lett. 11, 516–531. doi: 10.1111/j.1461-0248.2008.01164.x
De, M., Riopel, J. A., Cihacek, L. J., Lawrinenko, M., Baldwin-Kordick, R., Hall, S. J., et al. (2020). Soil health recovery after grassland reestablishment on cropland: the effects of time and topographic position. Soil Sci. Soc. Am. J. 84, 568–586. doi: 10.1002/saj2.20007
Degens, B. P., and Harris, J. A. (1997). Development of a physiological approach to measuring the catabolic diversity of soil microbial communities. Soil Biol. Biochem. 29, 1309–1320. doi: 10.1016/S0038-0717(97)00076-X
Denef, K., Six, J., Bossuyt, H., Frey, S. D., Elliott, E. T., Merckx, R., et al. (2001). Influence of dry–wet cycles on the interrelationship between aggregate, particulate organic matter, and microbial community dynamics. Soil Biol. Biochem. 33, 1599–1611. doi: 10.1016/S0038-0717(01)00076-1
Denef, K., Six, J., Merckx, R., and Paustian, K. (2002). Short-term effects of biological and physical forces on aggregate formation in soils with different clay mineralogy. Plant Soil. 246, 185–200. doi: 10.1023/A:1020668013524
Deng, L., Liu, G.-B., and Shangguan, Z.-P. (2014), Land-use conversion changing soil carbon stocks in China's ‘Grain-for-Green' Program: a synthesis. Glob. Change Biol. 20, 3544–3556. doi: 10.1111/gcb.12508
Doane, T. A., and Horwáth, W. R. (2003). Spectrophotometric determination of nitrate with a single reagent. Anal. Lett. 36, 2713–2722. doi: 10.1081/AL-120024647
DuPont, S. T., Beniston, J., Glover, J. D., Hodson, A., Culman, S. W., Lal, R., et al. (2014). Root traits and soil properties in harvested perennial grassland, annual wheat and never-tilled annual wheat. Plant Soil. 381, 405–420. doi: 10.1007/s11104-014-2145-2
Fernández-Ugalde, O., Jones, A., and Meuli, R. G. (2020). Comparison of sampling with a spade and gouge auger for topsoil monitoring at the continental scale. Eur. J. Soil Sci. 7, 137–150. doi: 10.1111/ejss.12862
Franzluebbers, A. J. (2016). Should soil testing services measure soil biological activity? Agric. Environ. Lett. 1:150009. doi: 10.2134/ael2015.11.0009
Freschet, G. T., Roumet, C., Comas, L. H., Weemstra, M., Bengough, A. G., Rewald, B., et al. (2021). Root traits as drivers of plant and ecosystem functioning: current understanding, pitfalls and future research needs. New Phytol. 232, 1123–1158. doi: 10.1111/nph.17072
Frey, S. D., Six, J., and Elliott, E. T. (2003). Reciprocal transfer of carbon and nitrogen by decomposer fungi at the soil-litter interface. Soil Biol. Biochem. 35, 1001–1004. doi: 10.1016/S0038-0717(03)00155-X
Glover, J. D., Culman, S. W., DuPont, S. T., Broussard, W., Young, L., Mangan, M. E., et al. (2010). Harvested perennial grasslands provide ecological benchmarks for agricultural sustainability. Agric. Ecosyst. Environ. 137, 3–12. doi: 10.1016/j.agee.2009.11.001
Golchin, A., Oades, J. M., Skjemstad, J. O., and Clarke, P. (1994). Soil structure and carbon cycling. Soil Res. 32, 1043–1068. doi: 10.1071/sr9941043
Jaikumar, N. S., Snapp, S. S., Murphy, K., and Jones, S. S. (2012). Agronomic assessment of perennial wheat and perennial rye as cereal crops. Agron. J. 104, 1716–1726. doi: 10.2134/agronj2012.0291
Jakobsen, I., Abbott, L. K., and Robson, A. D. (1992). External hyphae of vesicular-arbuscular mycorrhizal fungi associated with Trifolium subterraneum L. 1. Spread of hyphae and phosphorus inflow into roots. New Phytol. 120, 371–380. doi: 10.1111/j.1469-8137.1992.tb01077.x
Jastrow, J. D., Amonette, J. E., and Bailey, V. L. (2007). Mechanisms controlling soil carbon turnover and their potential application for enhancing carbon sequestration. Clim. Change 80, 5–23. doi: 10.1007/s10584-006-9178-3
Johnson, A. M., Gamble, A. V., Balkcom, K. S., and Hull, N. R. (2021). Influence of cover crop mixtures on soil health in southeastern crop production systems. Agrosyst. Geosci. Environ. 4:e20202. doi: 10.1002/agg2.20202
Kallenbach, C. M., Frey, S. D., and Grandy, A. S. (2016). Direct evidence for microbial-derived soil organic matter formation and its ecophysiological controls. Nat. Commun. 7:13630. doi: 10.1038/ncomms13630
Kardol, P., and Wardle, D. A. (2010). How understanding aboveground–belowground linkages can assist restoration ecology. Trends Ecol. Evol. 25, 670–679. doi: 10.1016/j.tree.2010.09.001
Kemper, W. D., and Rosenau, R. (1986). Soil cohesion as affected by time and water content. Soil Sci. Soc. Am. J. 48, 1001–1006. doi: 10.2136/sssaj1984.03615995004800050009x
Kleber, M., Sollins, P., and Sutton, R. (2007). A conceptual model of organo-mineral interactions in soils: self-assembly of organic molecular fragments into zonal structures on mineral surfaces. Biogeochemistry 85, 9–24. doi: 10.1007/s10533-007-9103-5
Koske, R. E., and Gemma, J. N. (1989). A modified procedure for staining roots to detect VA mycorrhizas. Mycol. Res. 92, 486–505. doi: 10.1016/S0953-7562(89)80195-9
Kuzyakov, Y. (2010). Priming effects: interactions between living and dead organic matter. Soil Biol. Biochem. 42, 1363–1371. doi: 10.1016/j.soilbio.2010.04.003
Lal, R. (2015). Restoring soil quality to mitigate soil degradation. Sustainability 7, 5875–5895. doi: 10.3390/su7055875
Ledo, A., Smith, P., Zerihun, A., Whitaker, J., Vicente-Vicente, J. L., Qin, Z., et al. (2020). Changes in soil organic carbon under perennial crops. Glob. Chang. Biol. 26, 4158–4168. doi: 10.1111/gcb.15120
Martin, T., and Sprunger, C. D. (2022). Sensitive measures of soil health reveal carbon stability across a management intensity and plant biodiversity gradient. Front. Soil Sci. 2:917885. doi: 10.3389/fsoil.2022.917885
Masuko, T., Minami, A., Iwasaki, N., Majima, T., Nishimura, S. I., and Lee, Y. C. (2005). Carbohydrate analysis by a phenol-sulfuric acid method in microplate format. Anal. Biochem. 339, 69–72. doi: 10.1016/j.ab.2004.12.001
Matamala, R., Jastrow, J. D., Miller, R. M., and Garten, C. T. (2008). Temporal changes in C and N stocks of restored prairie: implications for C sequestration strategies. Ecol. Appl. 18, 1470–1488. doi: 10.1890/07-1609.1
Miller, W. P., and Miller, D. M. (1987). A micro-pipette method for soil mechanical analysis. Commun. Soil Sci. Plant Anal. 18, 1–15. doi: 10.1080/00103628709367799
Newman, E. I. (1966). A method of estimating the total length of root in a sample. J. Appl. Ecol. 3, 139–145. doi: 10.2307/2401670
Novelli, L. E., Caviglia, O. P., and Piñeiro, G. (2017). Increased cropping intensity improves crop residue inputs to the soil and aggregate-associated soil organic carbon stocks. Soil Tillage Res. 165, 128–136. doi: 10.1016/j.still.2016.08.008
Peixoto, L., Elsgaard, L., Rasmussen, J., Kuzyakov, Y., Banfield, C. C., Dippold, M. A., et al. (2020). Decreased rhizodeposition, but increased microbial carbon stabilization with soil depth down to 3.6 m. Soil Biol. Biochem. 150:108008. doi: 10.1016/j.soilbio.2020.108008
Post, W. M., and Kwon, K. C. (2010). Soil carbon sequestration and land-use change: processes and potential. Glob. Change Biol. 6, 317–327. doi: 10.1046/j.1365-2486.2000.00308.x
Pugliese, J. Y., Culman, S. W., and Sprunger, C. D. (2019). Harvesting forage of the perennial grain crop kernza (Thinopyrum intermedium) increases root biomass and soil nitrogen. Plant Soil. 437, 241–254 doi: 10.1007/s11104-019-03974-6
R Core Team (2017). R: A Language and Environment for Statistical Computing. R Foundation for Statistical Computing. Available online at: http://www.R-project.org/ (accessed December 1, 2018).
Regelink, I. C., Stoof, C. R., Rousseva, S., Weng, L., Lair, G. J., Kram, P., et al. (2015). Linkages between aggregate formation, porosity and soil chemical properties. Geoderma. 247–248, 24–37. doi: 10.1016/j.geoderma.2015.01.022
Rillig, M. C., Aguilar-Trigueros, C. A., Bergmann, J., Verbruggen, E., Veresoglou, S. D., and Lehmann, A. (2015). Plant root and mycorrhizal fungal traits for understanding soil aggregation. New Phytol. 205, 1385–1388. doi: 10.1111/nph.13045
Six, J., Bossuyt, H., Degryze, S., and Denef, K. (2004). A history of research on the link between (micro)aggregates, soil biota, and soil organic matter dynamics. Soil Tillage Res. 79, 7–31. doi: 10.1016/j.still.2004.03.008
Six, J., Frey, S. D., Thiet, R. K., and Batten, K. M. (2006). Bacterial and fungal contributions to carbon sequestration in agroecosystems. Soil Sci. Soc. Am. J. 70, 555–569. doi: 10.2136/sssaj2004.0347
Sprunger, C., Culman, S., Robertson, G., and Snapp, S. (2018). Perennial grain on a midwest alfisol shows no sign of early soil carbon gain. Renew. Agric. Food Syst. 33, 360–372. doi: 10.1017/S1742170517000138
Sprunger, C. D., Culman, S. W., Peralta, A. L., DuPont, S. T., Lennon, J. T., and Snapp, S. S. (2019). Perennial grain crop roots and nitrogen management shape soil food webs and soil carbon dynamics. Soil Biol. Biochem. 137, 107573. doi: 10.1016/j.soilbio.2019.107573
Sprunger, C. D., Culman, S. W., Robertson, G. P., and Snapp, S. S. (2017). Perennial grain on a midwest alfisol shows no sign of early soil carbon gain. Renew. Agric. Food Sys. 33, 360–372. doi: 10.1017/s1742170517000138
Sprunger, C. D., and Robertson, R. P. (2018). Early accumulation of active fraction soil carbon in newly established cellulosic biofuel systems. Geoderma 318, 42–51. doi: 10.1016/j.geoderma.2017.11.040
Steinbeiss, S., Besler, H., Engels, C., Temperton, V. M., Buchmann, N., Roscher, C., et al. (2008). Plant diversity positively affects short-term soil carbon storage in experimental grasslands. Glob. Change Biol. 14, 2937–2949. doi: 10.1111/j.1365-2486.2008.01697.x
Syswerda, S. P., Basso, B., Hamilton, S. K., Tausig, J. B., and Robertson, G. P. (2012). Long-term nitrate loss along an agricultural intensity gradient in the Upper Midwest USA. Agric. Ecosyst. Environ. 149, 10–19. doi: 10.1016/j.agee.2011.12.007
Syswerda, S. P., Corbin, A. T., Mokma, D. L., Kravchenko, A. N., and Robertson, G. P. (2011). Agricultural management and soil carbon storage in surface vs. deep layers. Soil Sci. Soc. Am. J. 75, 92–101. doi: 10.2136/sssaj2009.0414
Tennant, D. (1975). A test of a modified line intersect method of estimating root length. J. Ecol. 63, 995–1001. doi: 10.2307/2258617
Tisdall, J. M., and Oades, J. M. (1982). Organic matter and water stable aggregates in soils. J. Soil Sci. 33, 141–163. doi: 10.1111/j.1365-2389.1982.tb01755.x
Totsche, K. U., Amelung, W., Gerzabek, M. H., Guggenberger, G., Klumpp, E., Knief, C., et al. (2018). Microaggregates in soils. J. Plant Nutr. Soil Sci. 181, 104–136. doi: 10.1002/jpln.201600451
USDA-NRCS Soil Science Division Staff. (2019). Web Soil Survey, Soil Data Explorer Tool. Available online at: https://websoilsurvey.sc.egov.usda.gov/App/HomePage (accessed July 21, 2022).
Vance, E. D., Brookes, P. C., and Jenkinson, D. S. (1987). An extraction method for measuring soil microbial biomass C. Soil Biol. Biochem. 19, 703–707. doi: 10.1016/0038-0717(87)90052-6
Vesterdal, L., Ritter, E., and Gundersen, P. (2002). Change in soil organic carbon following afforestation of former arable land. For. Ecol. Manage. 169, 137–147. doi: 10.1016/S0378-1127(02)00304-3
Virto, I., Barré, P., Burlot, A., and Chenu, C. (2012). Carbon input differences as the main factor explaining the variability in soil organic C storage in no-tilled compared to inversion tilled agrosystems. Biogeochemistry 108, 17–26. doi: 10.1007/s10533-011-9600-4
von Lützow, M., Kögel-Knabner, I., Ekschmitt, K., Matzner, E., Guggenberger, G., et al. (2006). Stabilization of organic matter in temperate soils: mechanisms and their relevance under different soil conditions—a review. Eur. J. Soil Sci. 57, 426–445. doi: 10.1111/j.1365-2389.2006.00809.x
Weatherburn, M. W. (1967). Phenol-hypochlorite reaction for determination of ammonia. Anal. Chem. 39, 971–974. doi: 10.1021/ac60252a045
Xia, Y., and Wander, M. (2022). Evaluation of indirect and direct scoring methods to relate biochemical soil quality indicators to ecosystem services. Soil Sci. Soc. Am. J. 86, 678–702. doi: 10.1002/saj2.20370
Keywords: agroecosystem, intermediate wheatgrass, soil organic carbon, soil aggregation, arbuscular mycorrhizal fungi
Citation: Chamberlain LA, Aguayo T, Zerega NJC, Dybzinski R and Egerton-Warburton LM (2022) Rapid improvement in soil health following the conversion of abandoned farm fields to annual or perennial agroecosystems. Front. Sustain. Food Syst. 6:1010298. doi: 10.3389/fsufs.2022.1010298
Received: 02 August 2022; Accepted: 06 October 2022;
Published: 21 October 2022.
Edited by:
Jacob Jungers, University of Minnesota Twin Cities, United StatesReviewed by:
Jessica L. M. Gutknecht, University of Minnesota Twin Cities, United StatesJoshua Gamble, Agricultural Research Service (USDA), United States
Copyright © 2022 Chamberlain, Aguayo, Zerega, Dybzinski and Egerton-Warburton. This is an open-access article distributed under the terms of the Creative Commons Attribution License (CC BY). The use, distribution or reproduction in other forums is permitted, provided the original author(s) and the copyright owner(s) are credited and that the original publication in this journal is cited, in accordance with accepted academic practice. No use, distribution or reproduction is permitted which does not comply with these terms.
*Correspondence: Louise M. Egerton-Warburton, bGVnZXJ0b24mI3gwMDA0MDtjaGljYWdvYm90YW5pYy5vcmc=