- Departamento de Biotecnología y Bioquímica, Centro de Investigación y de Estudios Avanzados del I.P.N. (Cinvestav), Unidad Irapuato, Irapuato, Mexico
The functional characterization of an Amaranthus hypochondriacus Natterin-4-Like-1 gene (AhN4L-1) coding for an unknown function protein characterized by the presence of an aerolysin-like pore-forming domain in addition to two amaranthin-like agglutinin domains is herewith described. Natterin and nattering-like proteins have been amply described in the animal kingdom. However, the role of nattering-like proteins in plants is practically unknown. The results described in this study, obtained from gene expression data in grain amaranth and from AhN4L-1-overexpressing Arabidopsis thaliana plants indicated that this gene was strongly induced by several biotic and abiotic conditions in grain amaranth, whereas data obtained from the overexpressing Arabidopsis plants further supported the defensive function of this gene, mostly against bacterial and fungal plant pathogens. GUS and GFP AhN4L-1 localization in roots tips, leaf stomata, stamens and pistils also suggested a defensive function in these organs, although its participation in flowering processes, such as self-incompatibility and abscission, is also possible. However, contrary to expectations, the overexpression of this gene negatively affected the vegetative and reproductive growth of the transgenic plants, which also showed no increased tolerance to salinity and water-deficit stress. The latter despite the maintenance of significantly higher chlorophyll levels and photosynthetic parameters under intense salinity stress. These results are discussed in the context of the physiological roles known to be played by related lectins and AB proteins in plants.
Introduction
The genus Amaranthus consists of 60−70 species (Das, 2016). They consist of C4 type herbaceous plants, many of which are notorious annual weeds (Ward et al., 2013). Some are consumed as vegetables (Shukla et al., 2010; Achigan-Dako et al., 2014; Joshi et al., 2018; Sarker and Oba, 2019), while others have ornamental value, are used as a source of pigments (Teng et al., 2015) or for grain production. Grain amaranths are native of the American continent and comprise three species: Amaranthus cruentus, A. hypochondriacus, and A. caudatus (Shukla et al., 2003), whose origin and taxonomic relationships are still uncertain (Trucco and Tranel, 2011; Kietlinski et al., 2014; Adhikary and Pratt, 2015; Stetter and Schmid, 2017; Stetter et al., 2017, 2020; Wu and Blair, 2017). They represent marginally cultivated pseudo-cereal crops that yield highly nutritional, protein-rich grains (Písaríkov et al., 2005; Caselato-Sousa and Amaya-Farfán, 2012; Rastogi and Shukla, 2013; Venskutonis and Kraujalis, 2013; Montoya-Rodríguez et al., 2015; De Ron et al., 2017).
Grain amaranths are considered stress-tolerant species that can readily adapt to drought, severe defoliation and poor soils (Caselato-Sousa and Amaya-Farfán, 2012; Castrillón-Arbeláez et al., 2012; Vargas-Ortiz et al., 2013, 2015). This trait has been associated with various anatomical, physiological and biochemical adaptations (Miller et al., 1984; Lal and Edwards, 1996; Johnson and Henderson, 2002; Omamt et al., 2006; Aguilar-Hernández et al., 2011; Huerta-Ocampo et al., 2011, 2014; González-Rodríguez et al., 2019; Cisneros-Hernández et al., 2021). Additionally, grain amaranths are generally known to be tolerant to insect pests and pathogens (Brenner et al., 2000; Délano-Frier et al., 2004; Sánchez-Hernandez et al., 2004; Casarrubias-Castillo et al., 2014).
A transcriptomic analysis of A. hypochondriacus plants subjected to diverse stress conditions further revealed that a substantial number of the genes that underwent changes in expression levels were orphan genes or had unknown function (Délano-Frier et al., 2011). Subsequent over-expression of a number of these genes in Arabidopsis thaliana suggested their participation in diverse mechanisms utilized by Amaranthus plant species to thrive in unfavorable environmental conditions (Massange-Sanchez et al., 2015; Massange-Sánchez et al., 2016; Palmeros-Suárez et al., 2015, 2017; Cabrales-Orona, 2017; Cabrales-Orona and Délano, 2021).
This study follows this line of research by describing the results derived from the functional characterization of an amaranth Natterin-4-Like-1 gene. Natterins and nattering-like proteins are characterized by the presence of an aerolysin-like pore-forming domain characteristic of Aeromonas species (Szczesny et al., 2011). These proteins were initially described in Thalassophryne nattereri a venomous fish species; now, their presence has been reported several others Eukaryotic organisms, including arbuscular mycorrhizal fungi, oysters, insects, birds and plants (Lopes-Ferreira et al., 1998; Tamura et al., 2011; Xue et al., 2012; Gacesa et al., 2015; Gudbrandsson et al., 2015; Unno et al., 2016; Rajan et al., 2017; Wu et al., 2017; Lima et al., 2021). They are considered to play a role as host defense molecules against pathogens in some of these organisms (Chen et al., 2018), whereas Natterin-4 genes have been found to be responsive to severe water-deficit stress (WDS) in maize (Shao et al., 2015; Jin et al., 2019). In addition, the occurrence of related chimeric proteins containing amaranthin-like domain(s) and an aerolysin-like domain are widespread in plants (Dang et al., 2017; Shang et al., 2017).
The results described in this study further supported a role for plant Nattering-4-like proteins in protection against biotic stress responses. Thus, the A. hypochondriacus Nattering-4-like-1 gene, or AhN4L-1, was found to be encode an AB protein with a lectin region conformed of two N-terminal agglutinin-like domains in addition to a C-terminal aerolysin-like domain. This gene was actively induced by various (a)biotic stress conditions in grain amaranths, including insect herbivory, defoliation, pathogen infection, salt stress, and WDS. Moreover, its overexpression in A. thaliana suggested that, apart from defense- and stress-related functions, the AhN4L-1 gene could be involved in plant development processes, such as flowering. These results are discussed in the context of the defensive and development roles known to be played by agglutinin-type lectins and AB protein in plants.
Materials and Methods
Plant Material
The three grain amaranth species (Amaranthus hypochondriacus cv. “Revancha,” A. cruentus cv. “Dorada,” A. cruentus cv. “Tarasca” and A. caudatus) employed in this study were provided by Dr. Eduardo Espitia Rangel, INIFAP, México, curator of the Mexican amaranth germplasm collection. The plants were germinated in a conditioned growth chamber, as described previously (Délano-Frier et al., 2011). Briefly, seeds were germinated in 60-well germinating trays containing a sterile soil preparation composed of a general soil mixture (three parts Sunshine Mix 3TM [SunGro Horticulture, Bellevue, WA], one part loam, two parts mulch, one part vermiculite [SunGro Hort] and one part perlite [Termolita S.A., Nuevo León, México] and coconut paste [Hummert de México, Morelos, México] in a 1:1 v/v relation). Germination occurred shortly after in a growth chamber maintained at 27 ± 1°C, ≈75% relative humidity (RH) and within a 16: 8 h light (under a photosynthetically active radiation [PAR] of ~300 μmol m−2 s−1) dark photoperiod. Three weeks after germination, the seedlings were transplanted to 1.3 L plastic pots containing 250 g of a general substrate and were grown in the same conditions as above. They were fertilized once, 1 week after transplant, with a 20:10:20 (N: P: K) + micronutrients nutrient soil drench solution according to the manufacturer's instructions (Peters Professional; Scotts-Sierra Horticultural Products, Marysville, OH, USA). Grain amaranth plants having 10–12 expanded leaves, were employed for all (a)biotic stress treatments, except defoliation (see below).
Arabidopsis thaliana plants, ecotype Columbia, were used for the generation of transgenic plants. A. thaliana seeds were germinated and grown following standard methodologies (Rivero et al., 2014). Briefly, seeds were sterilized by rinsing once with 70% ethanol, for 2 min and then in a 20% hypochlorite solution for 5 min. They were washed thrice with sterile distilled de-ionized (dd) water and kept under-water for 48 h at 4°C. Vernalized seeds were germinated on 100 × 15 mm Petri dishes containing 0.1 × Murashige and Skoog (MS) media (Murashige and Skoog, 1962) prepared with 0.43 g/ L MS salts (Caisson laboratories, North Logan, UT, USA), plus 0.5% sucrose, 100 mg/ L myo-inositol and 1% agar (Research Organics Inc., Cleveland, OH, USA). The dishes were transferred to growth chambers maintained under controlled conditions of light (PAR of 60–80 μmol m−2 s−1), photoperiod (16 h light/8 h dark) and temperature (22 ± 1°C). Plants were grown for 10 d post germination before transferal to 125 mL plastic pots filled with 60 g of a soil mixture composed of three parts Sunshine Mix 618 3TM (Sun-Gro Horticulture), one part loam, two parts mulch, one part vermiculite (SunGro Horticulture) and one part perlite (Termolita S.A.) and fertilized with a 20:10:20 (N: P: K) + micronutrients solution (Peters Professional). Plants were subsequently cultivated in growth rooms conditioned at 22°C 16 h light/ 8 h dark photo-period.
Identification of the Nattering-4-like-1 (AhN4L-1) Gene in A. hypochondriacus
The gene and mRNA sequences of the grain amaranth Nattering-4-like-1 gene (AhN4L-1) were retrieved from the A. hypochondriacus genome (Phytozome accession number: AHYPO_022599-RA CDS). The intronic and exonic regions of the AhN4L-1 gene were determined directly via the Phytozome platform (Goodstein et al., 2012).
A MatInspector Release professional 8.0.5 program (Genomatix), in addition to the New PLACE database of plant cis-acting regulatory DNA elements (Higo et al., 1999) were used to detect regulatory elements in the 1,600 bp promoter region of the AhN4L-1 gene. The protein encoded by the open reading frame (ORF) of the AhN4L-1 cDNA was deduced with the aid of ExpasyTranslate platform (https://web.expasy.org/translate/). The AhN4L-1 protein sequence was used to perform BLASTp searches against different plant genomes within the NCBI platform. Multiple alignment with amino acid sequences of related proteins in other plant species, based on the NCBI taxonomy, was used to construct the phylogenetic trees, which were generated with MEGA software (version 11.0) using the maximum like-hood method based on the JTT matrix-based model (Jones et al., 1992).
Molecular Modeling of the AhN4L-1 Protein
The AhN4L-1 protein was modeled entering the amino acid sequence, in FASTA format, into the Iterative Threading ASSEmbly Refinement or I-TASSER server platform (http://zhang.bioinformatics.ku.edu/I-TASSER), able to accept sequences ranging from 10 to 1,500 aa. The link predicted the most viable 3D structures which were modeled and visualized using the PYMOL software (http://pymol.sourceforge.net) (Roy et al., 2010).
Stress Treatments in Grain Amaranths
Groups of six grain amaranth plants (i.e., A. hypochondriacus, A. cruentus cv. “Dorada” or cv. “Tarasca” and A. caudatus) were used to perform all stress experiments. These were performed in the same chamber under used for germination and growth. The plants were subjected to acute salt stress by watering the 1.3 L pots for eight consecutive days with 100 mL of a 400 mM NaCl solution. The electrical conductivity of the soil at field capacity at the end of the stress treatment was ≈ 12.1 dS/m. It was measured using a portable HI 98130 conductometer (Hanna instruments Inc., Woonsocket, RI, USA). The conductivity reached by the soil in the salt treatment was within a range known to affect moderately salt-sensitive crops such as alfalfa, corn, millet, sugar cane, tomato, potato, and spinach (http://www.fao.org/docrep/005/y4263e/y4263e0e.htm). Control plants were maintained in the same conditions and were watered with dd water. The water stress treatments were performed using A. cruentus cv. “Dorada” as described previously (González-Rodríguez et al., 2019). Insect herbivory experiments were performed by placing three 3rd instar Trichoplusia ni (Lepidoptera, Noctuidae) larvae per plant. Larvae were starved for 16 h prior to the assays. All plants were covered with a net to restrict larval movement. Larvae were allowed to feed for 8 h and were retired from the plant. Plant tissue sampling of the herbivore-damaged plants was done 6, 12, and 24 h posterior to larval removal. Damaged and undamaged leaves, in addition to stems and roots, were collected. The bacteria infection assays were performed with Pseudomonas syringae pv. syringae 3525 (Pss) and Clavibacter michiganensis subsp. Michiganensis acr42 (Cmm). These pathogens were previously shown to interact with grain amaranth as avirulent and virulent bacterial pathogens, respectively (Casarrubias-Castillo et al., 2014). Briefly, aliquots of Pss and Cmm bacterial cultures were diluted to an OD600 of 0.2 (Pss) and 0.3 (Cmm) with sterile 0.05 M phosphate buffer, pH 7.0. They were subsequently used to infect the amaranth plants (16 plants per species) by spraying 400 μL of the respective cultures on the abaxial surface of the leaves closest to the apical meristem. For the Cmm assays, an additional 100 μL were inoculated in the stem, in a section adjacent to these leaves. The plants were incubated at 27 ± 1°C and infected leaves, uninfected leaves, stems and roots of 12 plants were sampled 6, 12, and 24 h after inoculation. Control plants were maintained in the same conditions after treatment with phosphate buffer only. Defoliation treatments were performed using A. cruentus plants cv. “Tarasca” as described before by Vargas-Ortiz et al. (2015) and Cisneros-Hernández et al. (2021). The choice of the “Tarasca” cultivar reflected its previous use as the model plant for ample defoliation-related research in grain amaranth (Castrillón-Arbeláez et al., 2012; Vargas-Ortiz et al., 2013, 2015). Briefly, the plants were subjected to severe defoliation at two different vegetative and reproductive development stages, respectively. Plants at the “vegetative 1” (V1) stage were utilized 4–5 weeks after germination (wag) and had 6-to-8 expanded leaves, whereas V2 plants were used at 6-to-7 wag and had 11–14 expanded leaves. Plants at panicle emergence (PE) were defoliated 9–10 wag, while those at the flowering stage (FL), were damaged 11–12 wag, when they had reached ~50% anthesis. The defoliation treatment was done with a punch-hole borer at a rate of 1/3 loss of foliar tissue area per diem for the first two days. On the third day, the leaf tissues remaining after ca. 66% percent leaf area loss, were cut from the base of the petioles to reach total plant defoliation and were immediately flash frozen in liquid nitrogen and stored for subsequent analysis. Twenty-four h later, leaves, roots, stems and panicles (when applicable) were sampled from intact plants (controls), whereas only roots, stems and panicles were collected from defoliated plants. All plant tissues/organs sampled were stored at −80°C until needed for analysis. Then, they were ground to a fine powder in liquid N2 with frozen mortar and pestle.
Quantitative PCR Assays
Total RNA was extracted and subsequently purified from 100 to 200 mg of frozen and ground plant tissues as described previously (Palmeros-Suárez et al., 2015). The integrity of the purified RNA was assessed by visualizing the 18S and 28S RNA bands after electrophoretic separation in ethidium bromide-containing denatured formaldehyde/formamide 1% agarose gels. RNA purity and concentration were determined in a NanoDrop 2000 apparatus as instructed (ThermoFisher Scientific; Waltham, MA, USA). cDNA synthesis from 4 μg of total RNA was subsequently realized using 200 units of the reverse transcriptase SuperScript II enzyme as instructed by the manufacturer (Invitrogen, Carlsbad, CA, USA). The cDNA produced was diluted 25-fold with dd water prior to quantitative PCR (qPCR) analysis. All qPCR assays were performed using SYBR Green detection chemistry in a CFX96 Real Time System (Bio-Rad, Hercules, CA, USA). qPCR reactions were prepared using the primers listed in Supplementary Table S1, which were designed using the following programs: Primer3 (http://bioinfo.ut.ee/primer3/), Beacon Designer (http://www.premierbiosoft.com), UNAFold (http://www.idtdna.com/UNAFold) and Oligo Evaluator (http://www.oligoevaluator.com) (Thornton and Basu, 2011). Reaction mixtures, 20 μL total volume, contained 2 μL cDNA solution, 4 μL of each 2 μM oligonucleotide solutions, 8 μL de SYBR Green Jumpstart TAQ Ready Mix (Sigma-Aldrich St. Louis, MO, USA) and 2 μL of sterile dd water. The amplification process was performed within the following parameters: 15 min at 95°C to activate the Taq Polymerase, followed by 40 cycles of denaturation (95°C/15 s) annealing (60°C/1 min) and extension (72°C/30 s) and a final extension step (60°C/1 min). AhN4L-1 expression levels were determined in different tissues of grain amaranth plants subjected to (a)biotic stress. These were reported in relation to the expression levels in representative tissues of control plants, which were set to a value of 1. In transgenic A. thaliana, foliar AhN4L-1 transgene expression levels were calculated relative to trace background levels in WT plants. Relative gene expression was calculated using the 2−ΔΔCT comparative cycle threshold method (Livak and Schmittgen, 2001). The genes employed as normalizing controls were the AhACT7 and AhβTubulin (for grain amaranth) and actin and EF-1α (for A. thaliana) housekeeping genes. In all cases, plant tissues for qPCR assays were obtained from combined pools of six plants. Each pool was subsequently subjected to three independent sampling procedures prior to analysis. qPCR data are reported as the mean of three repetitions ± SE of one representative experiment. qRT-PCR expression analyses were validated in two independent experiments.
Generation of P35S::AhN4L-1 Overexpressing (OE) Arabidopsis thaliana Transgenic Plants
The 1581 base pair (bp) open reading frame of AhN4L-1 was PCR amplified from A. hypochondriacus cDNA using specific primers (Table 1) and purified using a standardized procedure (Protocol kit; Qiagen, Germatown, MD, USA). Plasmids for expression of the AhN4L-1 under the control of the CaMV 35S promoter were assembled using the Gateway TA Cloning technology (Invitrogen). AhN4L-1 coding sequences were amplified as PCR products, having an additional deoxyadenosine at their 3′-ends, using cDNA obtained from RNA extracted from 9-day-old plant leaves from A. hypochondriacus L. cv. Revancha. The complete attB PCR product was obtained using, nested PCR using the primers listed in Table S1. The PCR program was run using the following steps: initial 5 min at 94°C; then, 30 cycles (30 s at 94°C, 30 s at 65°C and 1.5 min at 72°C), and a final elongation step for 10 min at 72°C. Subsequently, the fragments were inserted into the pCR8/GW/TOPO TA entry vector (Invitrogen). After sequencing of the entry clones, the LR reaction was performed for subsequent cloning in the pB7WG2D destination vector (Cambia, Canberra, Australia), under the control of 35S CaMV promoter. In both cases, spectinomycin resistance (at 50 μg/mL) was used for selection of transformed E. coli TOP10 cells. The binary construct was extracted and purified following the manufacturer's instructions (Invitrogen). After confirmation of correct orientation and size of the insert with the BglI, PstI, and XbaI restriction enzymes and subsequent sequencing (Instituto de Biotecnología de la UNAM, Cuernavaca, Morelos, México) it was electroporated into Agrobacterium tumefaciens strain GV2260. After a 48 h incubation period at 28°C on LB media spiked with rifampicin (25 μg/mL) and spectinomycin (100 mg/L), they were used to transform A. thaliana plants by the floral dip method (Clough and Bent, 1998).
Transformed plants were grown to maturity under controlled conditions, as described above, and the seeds were harvested at maturity. One-week old T1 transgenic A. thaliana plants were selected by growth in MS medium containing N-acetyl-L-phosphinothricin (PPT) (20 μg/mL), by monitoring the emission of GFP-related fluorescence under a Zeiss Primo Star fluorescence microscope (Carl-Zeiss GmbH, Oberkochen Germany) and by the PCR amplification of the GFP marker gene using specific primers (Table 1). T2 seeds were collected from individual transformants (T1) and plated again on the selection medium to determine PPT-resistant vs. PPT-sensitive plants segregation ratios. Homozygous T3 transgenic plants produced no PPT-sensitive seedlings from seeds of T2 plants. All further analysis of AhN4L-1 OE lines was performed using T3 plants homozygous for the transgene.
Seeds of WT controls and T3 homozygous A. thaliana transgenic plant lines differing in AhN4L-1 transgene dosage (i.e., L5, L6, and L17; Supplementary Figure S1), were germinated and grown as described previously (Massange-Sanchez et al., 2015; Palmeros-Suárez et al., 2015). Independent sets of OE transgenic and wild-type plants were grown for the different analyses performed.
Generation of PAhN4L-1::GUS Plants for AhN4L-1 Promoter Activity Analysis in A. thaliana
A 1600 bp DNA fragment covering the 5'flanking region of the A. hypochondriacus AhN4L-1 gene was PCR-amplified from A. hypochondriacus genomic DNA. The primer pairs used in the PCR reaction are listed in Table 1. The PCR DNA amplicon was double-strand sequenced to verify its authenticity. Using the Gateway technology described above, it was inserted first into the pCR8/GW/TOPO TA vector and then into the pFAST-G04 vector harboring a GUS reporter gene. This vector was electroporated into A. tumefaciens GV2260 cells, which were selected by incubation for 48 h and 28°C on LB media supplemented with rifampicin (50 mg/L) and spectinomycin (100 mg/L). Transformed agrobacteria were subsequently used for A. thaliana transformation as described above.
Generation of GFP::AhN4L-1 Plants for AhN4L-1 Protein Localization Analysis in A. thaliana
Plasmids for expression of the AhN4L-1 sequence N-terminally fused to GFP under the control of the CaMV 35S promoter were constructed using the Gateway Cloning technology (Invitrogen), as above. Coding sequences were amplified as PCR products via nested PCR with the primers listed in Table S1. After the entry clone was validated by sequencing, it was inserted into the pk7WGF2 destination vector in order to fuse the GFP sequence N-terminally to AhN4L-1 (Karimi et al., 2002). These vectors were electroporated into A. tumefaciens GV2260 cells, which were selected and subsequently used for A. thaliana transformation as described above.
Histochemical β-Glucuronidase (GUS) Staining Assay in A. thaliana
GUS histochemical staining was performed in diverse organs of transgenic A. thaliana plants (line 4, having the highest transgene expression; G. Cabrales-Orona, personal communication) harboring the pAhN4L-1::GUS fusion construct at different development stages (5 and 35 day-old plants). GUS staining buffer was prepared in 5 mM sodium phosphate buffer, pH 7.0, containing 40 mM potassium ferricyanide (K3Fe(CN)6), 40 mM potassium ferrocyanide (K4Fe(CN)6 and 0.004% Triton X-100. This buffer was stored at −20°C until required. Five μg/ mL of 5-bromo, 4-chloro, 3indolyl-β- D-glucuronide (X-Gluc) was dissolved in DMSO and added to the buffer. The different plant organs were incubated in X-Gluc solution for 16 h at 37°C. The visualization of the blue stained organ sections indicative of GUS activity was possible after repeatedly rinsing with buffer Z (40 mM NaH2PO4, 60 mM NaHPO4, 10 mM KCl, 1 mM MgSO4) and 70% ethanol, followed by chlorophyll removal with an acetone: methanol (1:3 v/v) solution (Jefferson et al., 1987). The images of blue-colored plant organs and plants at different developmental stages were recorded using a ZEISS Stemi 2000-C stereo microscope (Carl Zeiss, Jena, Germany). GUS-stained organs and plants shown in this study were obtained from a representative experiment, performed with the L4 transgenic plant line, that was repeated thrice with similar results.
Subcellular Localization of AhN4L-1 Proteins in A. thaliana
Fluorescent images of roots and flowers were obtained from 3- and 35-day old transgenic plants (Line 34; Supplementary Figure S1), respectively, transformed with the N-terminal GFP::AhN4l-1 construct. They were recorded using a confocal laser scanning inverted microscope LSM 800 (Carl Zeiss, Jena, Germany). Propidium iodide (PI) at 0.01 mg/mL was used as a counterstain to highlight root cell morphology. GFP and PI were excited using the 488-nm and 514-nm lines, respectively, of an Argon laser. GFP emission was filtered with a 500–550-nm bandpass filter and PI emission was filtered with a 575-nm long pass filter. Images were captured using a Rebel T3 camera (Canon USA, Inc., Melville, NY, USA).
Measurement of Germination Rate, Vegetative, and Reproductive Growth and Seed Yield in AhN4L-1 Transgenic OE A. thaliana Plants
The effect on vegetative and reproductive growth and seed yield was determined in the three transgenic OE A. thaliana plants lines (L5, L6, and L17; Supplementary Figure S1) having differential expression levels of the AhN4L-1 gene. Germination rate was determined 3, 6, and 9 days after placing 100 seeds in Petri dishes (100 × 15 mm) containing sterile solid MS medium (0.5 × MS salt, 1% sucrose, and 1% agar, at pH 5.8). Root growth was similarly determined in 3, 5, and 7 days-old seedlings grown vertically on MS-containing 100 × 15 mm Petri dishes. Leaf number, leaf area, plant rosette area, inflorescence height and seed yield and weight were also determined in 5–6 weeks-old transgenic A. thaliana plants. The onset of flowering was also recorded. To measure yield, dry seeds were harvested manually from fully matured plants, approximately after 8 weeks of growth. Seed weight was determined as the measure of 1,000 seeds. All experiments were performed in the A. thaliana conditioned growth room, as described above.
Abiotic Stress Resistance Assays in Transgenic A. thaliana Plants
The L5, L6, and L17 lines were used to perform the stress experiments, as follows. Twenty 3-weeks old plants of each transgenic line were grown individually in single 125 mL plastic pots containing the A. thaliana soil mixture described above. All plants were kept in the conditioned growth room maintained at constant temperature of 22°C and under a 16 h light/8 h dark photo-period. Salt stress was applied by watering the plants with 20 mL each of a 400 mM NaCl solution on day 1 and with an identical volume of a 100 mM NaCl solution on day 3. The soil salinity at this point (8.2 dS/m), measured according to the NOM-021-RECNAT-2000 environmental norm (DOF, 2002) with a Hanna HI 98130 conductometer (Hanna instruments Inc., Woonsocket, RI, USA) corresponded to moderate to high saline soils (Artiola et al., 2019). In planta determination of photosynthetic parameters (see below) were performed immediately after the application of the second 100 mM NaCl solution, whereas the foliage and roots of all plants, divided into 2 pools of 10 plants each, were collected 3 weeks later. The plant tissues were flash frozen with liquid N2 and stored at −70°C until needed for analysis. Soluble and insoluble non-structural carbohydrate (NSC) content, as well as the levels of chlorophyll, carotene, total phenols and flavonoids, in addition to the catalase and superoxide dismutase enzymatic activities (see below) were determined in control and salt-stressed plants. The water deficit stress assays were performed according to Massange-Sanchez et al. (2015) and Palmeros-Suárez et al. (2015).
Measurement of Physiological and Biochemical Parameters: Photosynthetic Activity
A portable CIRAS-3 equipment (PP Systems, Amesbury, MA, USA) was employed to measure CO2 assimilation (A) and transpiration (E) rates, respectively, in addition to stomatal conductance (gs) and water use efficiency (WUE). The equipment parameters were set at 380 ppm CO2, 55% RH and a PAR of 1,000 μmol m−2 s−1. The Fv/Fm measurement of the photosystem II efficiency in a dark-adapted state was determined in A. thaliana plants kept in the dark for 30 min using a portable chlorophyll fluorometer (Pocket PEA Chlorophyll Fluorimeter; Hansatech Instruments; Norfolk, England).
Measurement of Physiological and Biochemical Parameters: Total Chlorophyll and Carotene Content Determination
Total chlorophyll content in A. thaliana leaf tissues was determined after extraction with cold 80% acetone according to Arnon (1949). Briefly, 200 mg of frozen leaf tissue was extracted twice with 800 μL of 80% acetone for 1 h at 4°C and centrifuged. Both extracts were combined to spectroscopically measure total chlorophyll levels in a UV-visible xMark microplate absorbance spectrophotometer (Bio-Rad) by recording the absorbance at 645 (A645) and 663 nm (A663). An acetone blank solution was used to calibrate the apparatus. Total chlorophyll was determined with the following equation: 8.02 × A663 + 20.2 × A645. Total carotene content was calculated according to Biehler et al. (2010) with some modifications. Thus, 100 mg of frozen leaf tissue, previously ground to a fine powder in liquid N2, together with 20 mg of calcium carbonate, was extracted with 400 μL metanol and 100 μL of 30% KOH in methanol for 15 min in the dark. The resulting extract, clarified by centrifugation and mixed with 1.5 mL of a saturated NaCl solution, was extracted twice with 500 μL of an hexane:chloroform (1:1 v/v) solution. The combined extracts were dried overnight by vacuum centrifugation (Maxi-Dry Lyo; Heto-Holten, Denmark) and re-dissolved in 0.5 mL acetone. Total carotenes were determined in a UV-visible spectrophotometer, as above, by recording the absorbance at 450 nm.
Measurement of Physiological and Biochemical Parameters: Soluble and Insoluble NSCs, Proline, Total Phenols and Flavonols, Catalase and Superoxide Dismutase Enzyme Activities
Soluble (i.e., glucose [Glc], fructose [Fru] and sucrose [Suc]) and insoluble (i.e., starch) NSC contents in lyophilized ground tissues were determined enzymatically using a coupled assay as described previously (Cisneros-Hernández et al., 2021). Proline was assayed colorimetrically, at 520 nm, via the measurement of the red chromogen produced by its reaction with ninhydrin at low pH (Bates et al., 1973). Total phenols and flavonols were determined according to colorimetric methods described before by Maranz et al. (2003) and Sakanaka et al. (2005), respectively. Catalase (CAT; E.C. 1.11.1.6) and superoxide dismutase (SOD; E.C.1.15.1.1) activities were determined using commercially available kits as described before (Palmeros-Suárez et al., 2015).
Biotic Stress I: Bacterial Infection With Pseudomonas syringae
These experiments were performed with Pseudomonas syringae pv. tomato DC3000 (Pst3000) as described by Yao et al. (2013). Briefly, bacterial glycerol stocks were removed from freeze-storage at −70°C, were plated on Luria-Bertani (LB) agar and were incubated at 28°C for 48 h. A single colony was used to inoculate 5 mL of liquid LB media which was incubated at 28°C for ≈ 12 h until the bacterial growth reached an OD600 of between 0.8 and 1.0. The culture was centrifuged at 5,000 rpm for 10 min at room temperature and the resulting bacterial cell pellet was resuspended in sterile dd water to an OD600 of 0.002 which was equivalent to 1 × 106 colony forming units/mL (CFUs/mL). Three fully expanded leaves of WT and transgenic A. thaliana plants (five 20-day old plants per genotype: WT, L5, L6, and L17, respectively) were each inoculated, at their abaxial surface, with 10 μL aliquots of the Pst3000 bacterial suspension. An identical number of control plants were similarly inoculated with sterile dd water. The plants were subsequently incubated for 3 days at 28°C. At this time, photographic register was obtained and leaves were sampled to determine the bacterial titers as CFUs/cm2. The latter were obtained as follows: the leaves were surface sterilized by submersion in a 15% ethanol solution for 15 s and rinsed by dipping in sterile dd water for 10 to 15 s. Leaf discs, 0.5 cm2, were then obtained from the leaves using a cork borer. Each disc was then placed in a 1.5 mL Eppendorf tube containing 100 μL of sterile dd water, where the leaf tissue was thoroughly macerated. The macerated tissue was diluted with the addition of 900 μL dd water and was vortexed. Serial 10 × dilutions of the extracts were produced by adding 100 μL aliquots in 900 μL of dd water and 10 μL of each were plated on LB agar plates. The plates were sealed with parafilm material and incubated at 28°C for 24 to 30 h until colonies were visible. These were counted in order to calculate the number of UFCs/cm2 of leaf tissue. A total of 3 leaf discs per plant per four plants were analyzed to reach the final bacterial counts. The experiment was repeated thrice.
Biotic Stress II: Fungal Infection With Fusarium oxysporum
Agar squares containing spores of F. oxysporum f. sp. lycopersici were placed on freshly prepared potato-dextrose agar plates and incubated at 28°C for 7 days to obtain dense fungal growth. Spores collected from these cultures were resuspended in 2 mL of sterile dd water. Spore counts were performed using a hemocytometer, followed by dilution with sterile dd water to reach a final count of 106 spores/mL. Volumes of this suspension (10 mL) were employed to infect the A. thaliana plants by their direct application to the soil as drench solutions. A total of twenty 20-day old plants (5 per genotype: WT, L5, L6, and L17) per assay were employed. The plants were incubated at 28°C for 15–25 days, when the disease symptoms started to be apparent. Photographic register of the plants and roots was obtained 25 days after infection. The fresh weight of the shoot and roots was also determined. Root samples were further processed for DNA extraction which was subsequently used for PCR amplification of Fusarium oxysporum-specific ITS amplicons. Root DNA was extracted using the urea-phenol method described by Shure et al. (1983). Briefly, 100 mg of frozen root tissue was ground in liquid N2 and mixed with 700 μL of an extraction buffer composed of 7 M urea, 0.35 M NaCl, 50 mM Tris-HCL (pH 8.0), 20 mM EDTA, 0.5% sarcosyl, 0.25 mL of molecular grade phenol and 0.2 mL of a 20% SDS detergent solution. The mixture was homogenized by vortexing at room temperature and extracted with 700 μL of a phenol: chloroform; isoamyl alcohol (50:48:2 v/v/v) solvent mixture. It was centrifuged at 12,000 rpm for 10 min at 4°C. The upper aqueous phase was transferred to a fresh tube where 400 μL of cold isopropanol and 40 μL of a potassium acetate solution were added and mixed by gently inverting the tube. The tube was centrifuged again at 12,000 rpm for 10 min. After discarding the supernatant, the resulting pellet was washed with 1 mL of a 99% ethanol solution and centrifuged again at 12,000 rpm for 3 min. The washed DNA pellet was dissolved in 100 μL of sterile dd water, spiked with 1 μL of ARNase (Invitrogen) and incubated at 37°C for 30 min. The resulting DNA was used as template for a PCR reaction performed using the ITS1 and ITS4 primers listed in Table 1. The PCR amplification program used was the following: denaturing at 94°C/3 min followed by 30 cycles of 30 s/94°C, 30 s/60°C and 1 min/72°C. The PCR products were separated via 1% agarose gel electrophoresis and the resulting bands, visualized with ethidium bromide, were photographed using a photo-documenter (Bio-Rad).
Statistical Analysis
All the experiments were performed using a completely randomized design with 20 plants per genotype. Data generated were analyzed using a one-way analysis of variance (ANOVA) to examine the effect of different stress conditions on the expression levels of the AhN4L-1 gene in grain amaranth plants and of the overexpression of this gene on various physiological variables in A. thaliana plants. The means were compared using the Tukey-Kramer test to identify statistically significant differences between them. The statistical analysis was performed with the aid of R (http://r-project.org/) Rstudio (https://www.rstudio.com) and JMT Pro13 (jmp.com) statistical software.
Results
Domain Architecture and Sequence of the AhN4L-1 Gene
A gene coding for an uncharacterized A. hypochondriacus Nattterin-4-like1 protein (AhN4L-1) possessing an AAT domain arrangement was identified and characterized. The AhN4L-1 protein contains two successive agglutinin (A) domains linked to a single toxin domain (or T domain), with sequence similarity to aerolysin, the pore-forming toxin produced by Aeromonas sp. It differs from the amaranthin-like AAT proteins recently reported in Caryophillales, including A. hypochondriacus, and several other monocot and dicot plant orders (Dang et al., 2017). Sequence alignment of the mRNA and the genomic contig sequences revealed the 10754 bp gene has three exons of 394, 795, and 392 bp, respectively, that code for a 526 aa protein (Supplementary Figure S2). It has a homolog of 518 aa (AhN4L-2 protein Phytozome accession number: AHYPO_022596-RA CDS; Supplementary Figure S2). The A domains of the AhN4L-1 protein are localized at positions 14-102 and 175-274, respectively, whereas the T domain is present in position 294-423 (Figures 1A,C). Bio-informatic analyses (https://zhanglab.ccmb.med.umich.edu/I-TASSER/output/S553256/) predicted a 3D structure with 2 agglutinin-like domains within the N-terminal half of the protein, and one aerolysin-like domain at the C-terminal end (Figure 1B). A phylogenetic tree (Figure 2) showed that the AhN4L-1 and AhN4L-2 proteins from A. hypochondriacus are grouped together with other uncharacterized N4L proteins present in Beta vulgaris and Chenopodium quinoa. Blast and Blast4OneKp alignments retrieved a maximum resemblance of 43% with the LOC104887222 protein from B. vulgaris. No homologous sequences were detected in A. thaliana. The tree indicated lower homology levels with ATT and Nattering-like proteins identified in other monocot and dicot plants species.
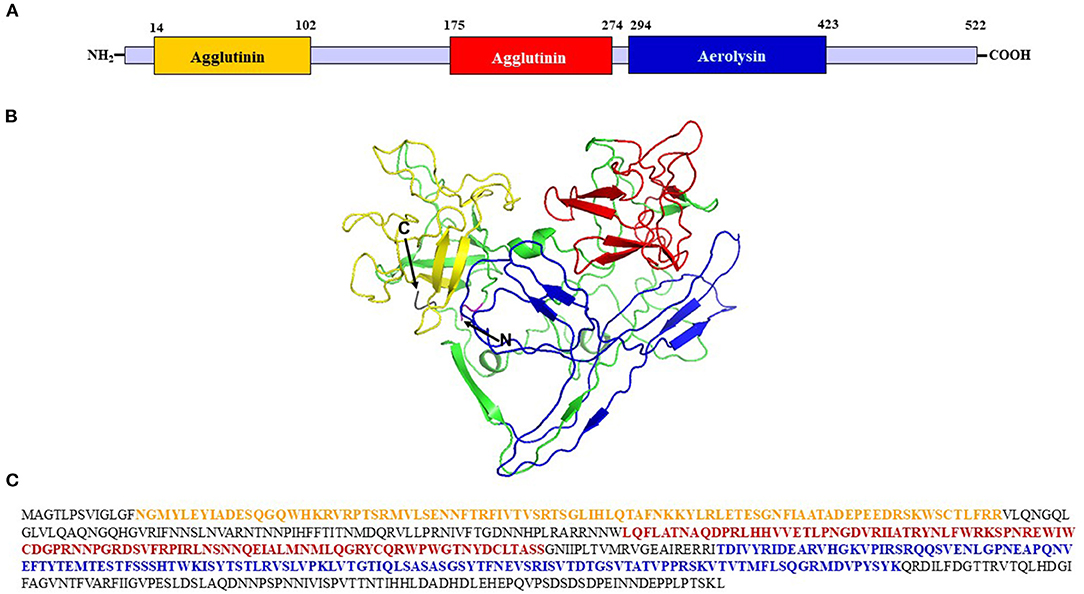
Figure 1. Structural characterization of the AhN4L-1 protein. (A) Diagram showing the length of the AhN4L-1 protein and the localization of the two agglutinin-like domains and the sole aerolysin domain. (B) The molecular modeling of AhN4L-1 shows both agglutinin monomers at the N-terminal end of the protein, colored yellow and red, respectively, connected to an aerolysin-like domain, colored blue. The model was generated using the tools described in https://zhanglab.ccmb.med.umich.edu/I-TASSER. (C) The 522-long amino acid sequence of the AhN4L-1 protein. The segments corresponding to the agglutinin-like and aerolysin-like domains are colored in yellow, red and blue, respectively.
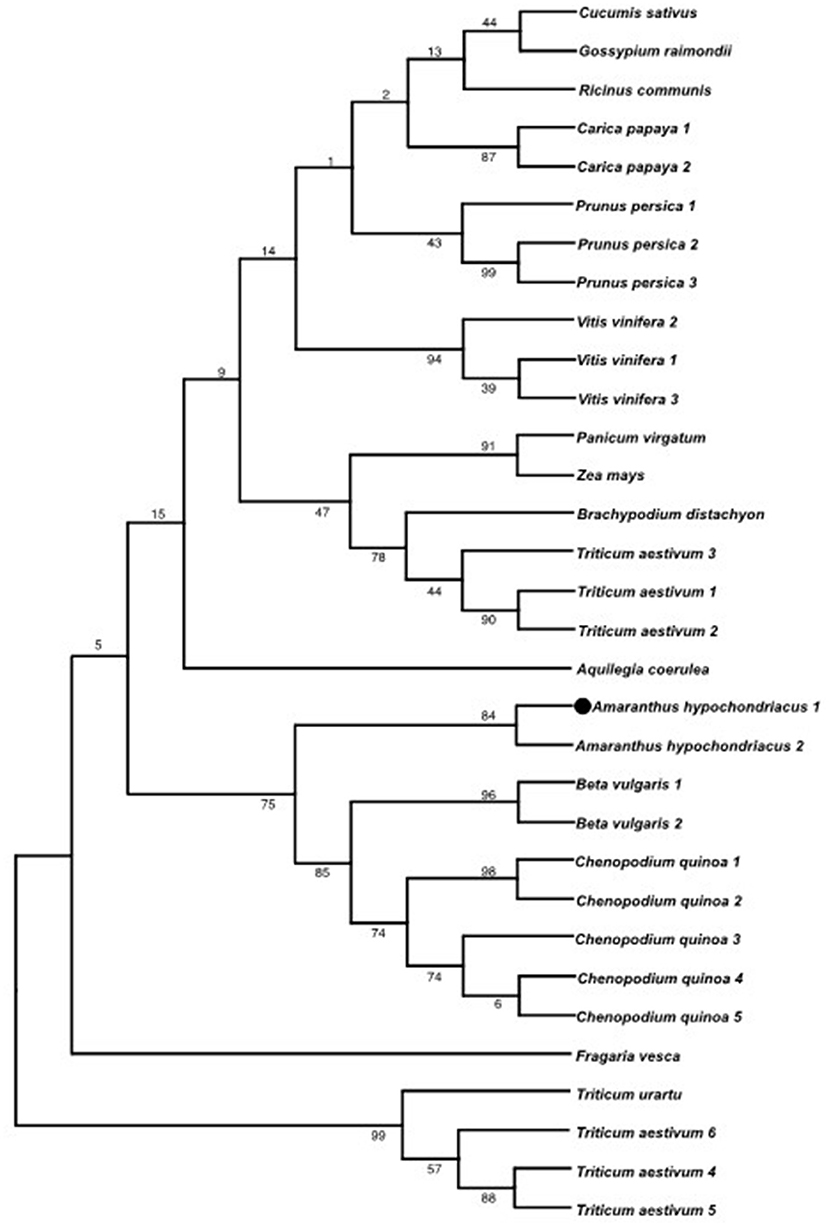
Figure 2. Phylogenetic analysis of the AhN4L-1 protein. The phylogeny shown, obtained by the maximum likelihood method, shows the relationship of AhN4L-1 (represented in the tree as Amaranthus hypochondriacus 1 and marked with a circle) and its homolog, AhN4L-2 (Amaranthus hypochondriacus 2), with related proteins reported in closely related plant species (i.e., Beta vulgaris and Chenopodium quinoa) and with other monocot and dicot plants. The tree was built using MEGA software (version 11.0) using the maximum like-hood method based on the JTT matrix-based model (Jones et al., 1992). The bootstrap value next to the branches was estimated using the bootstrap test (1,000 replicates).
The AhN4L-1 Gene Is Responsive to Various (a)Biotic Stress Stimuli in Grain Amaranth
Previous transcriptomic data of AhN4L-1 in A. hypochondriacus plants revealed that this gene was strongly induced by water-deficit and salinity stress and by chewing insect herbivory (Délano-Frier et al., 2011). These results were corroborated by qPCR data obtained from plants of the three grain amaranth species subjected to different stress conditions (Table 1). Thus, its responsiveness to insect herbivory was confirmed by its strong, rapid and stable induction in leaves damaged by chewing insect herbivory in all three species, particularly in A. cruentus. A weaker systemic expression of this gene was also observed in A. cruentus and A. hypochondriacus but was absent in A. caudatus. Bacterial infection with virulent Cmm infection predominantly induced this gene in the stems of the three grain amaranth species 12 h after infection whereas treatment with avirulent Pss induced a rapid (6 h) expression of this gene in most organs of A. hypochondriacus, except roots, and an intermediate to late (12 to 24 h) transcript accumulation in the roots of the three grain amaranths. Salinity led to a predominant expression of this gene in stems and roots, while moderate WDS and subsequent recovery induced its expression in leaves and roots of A. cruentus cv. Dorada plants. Severe defoliation of A. cruentus cv. Tarasca plants also led to the induction of this gene, mostly in leaves and roots and during V1 and V2 vegetative stages and during PE. The expression of this gene was limited to the stem when A. cruentus plants were defoliated at FL.
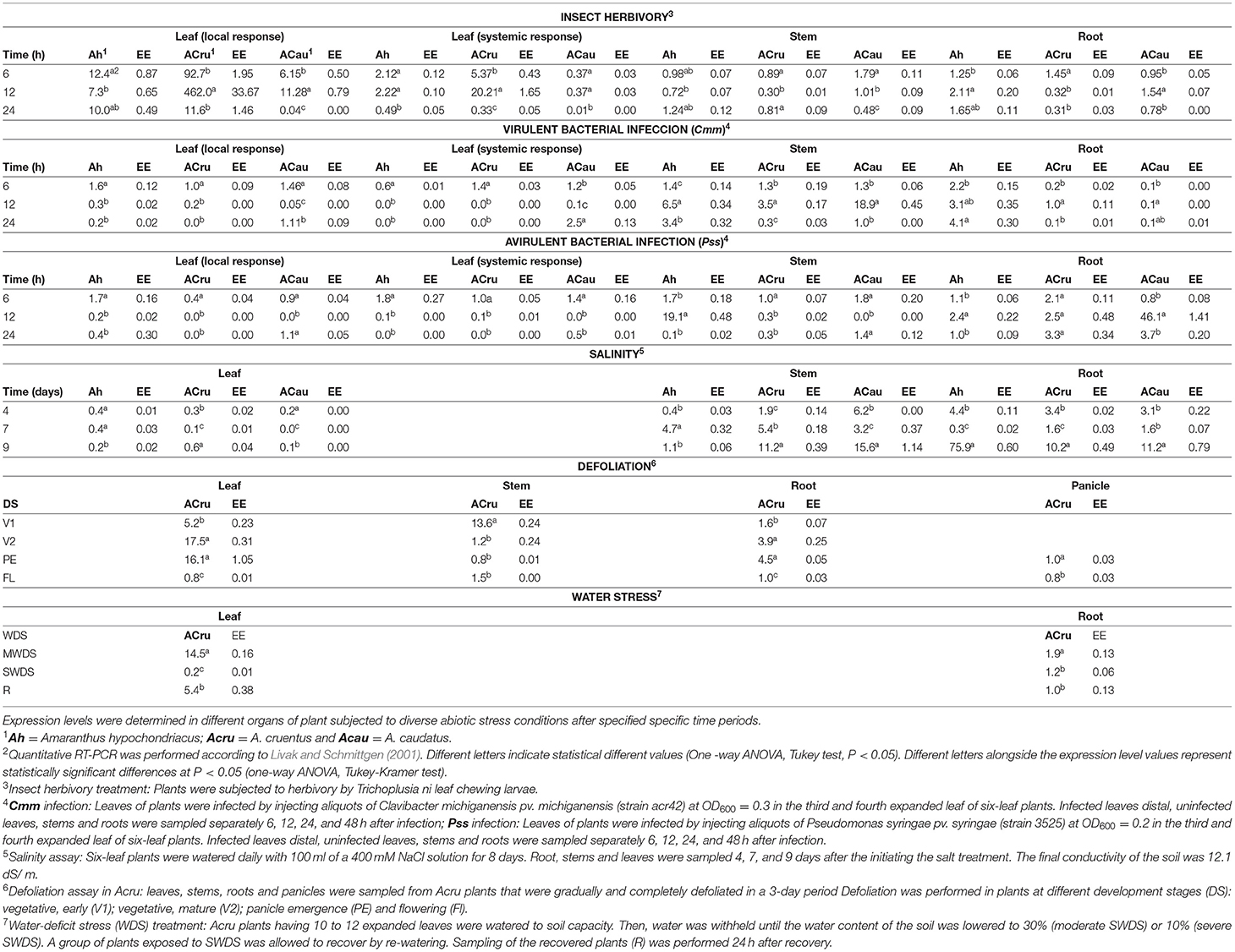
Table 1. Relative expression of the AhN4L-1 gene in grain amaranths in response to (a) biotic stress.
GUS Reporter Localization of AhN4L1 Gene Expression During Development of Transgenic A. thaliana Plants
To investigate the promoter activity of the AhN4L1 gene, a genomic DNA fragment (1.6 kbp) containing the 5′ flanking region of this gene was cloned after PCR amplification. This promoter region was fused to the E. coli uidA reporter gene encoding a GUS enzyme and introduced into A. thaliana through Agrobacterium-mediated gene transfer. Transgenic T3 A. thaliana L4 plants containing the homozygous recombinant transgene were subjected to histochemical staining for GUS activity. An in silico analysis of this promoter region segment unveiled the prominence of five elements (AHBP, DOF, GTBX, L1BX and MADS) involved in development and (a)biotic stress responses. These were also prominent in the promoter region of the AhN4L-2 gene. Interestingly, four cis-regulatory elements associated with abscisic acid (ABA) and ethylene signaling, root and apical meristem regulation and control of storage proteins synthesis, were only found in the promoter region of the AhN4L-1 gene (Supplementary Table S2). The prevalent elements present in the AhN4L-1 promoter were in agreement with the gene expression patterns observed in these transgenic plants (Figure 3). Thus, the detection of GUS staining along the stem in young germinated plantlets (Figure 3A) and in developing root tips (Figures 3C,D) concurred with the regulation of development-related functions by genes rich in AHBX and GT-box elements, as shown in Supplementary Table S2. Likewise, the expression in developing flowers, particularly in the anthers (Figure 3E), pollen (Figure 3G), and segments of the pistil (Figure 3F), coincided with the control of flower development, including flowering structures and floral meristems, that characterizes genes rich in L1- and MADS-boxes (Supplementary Table S2).
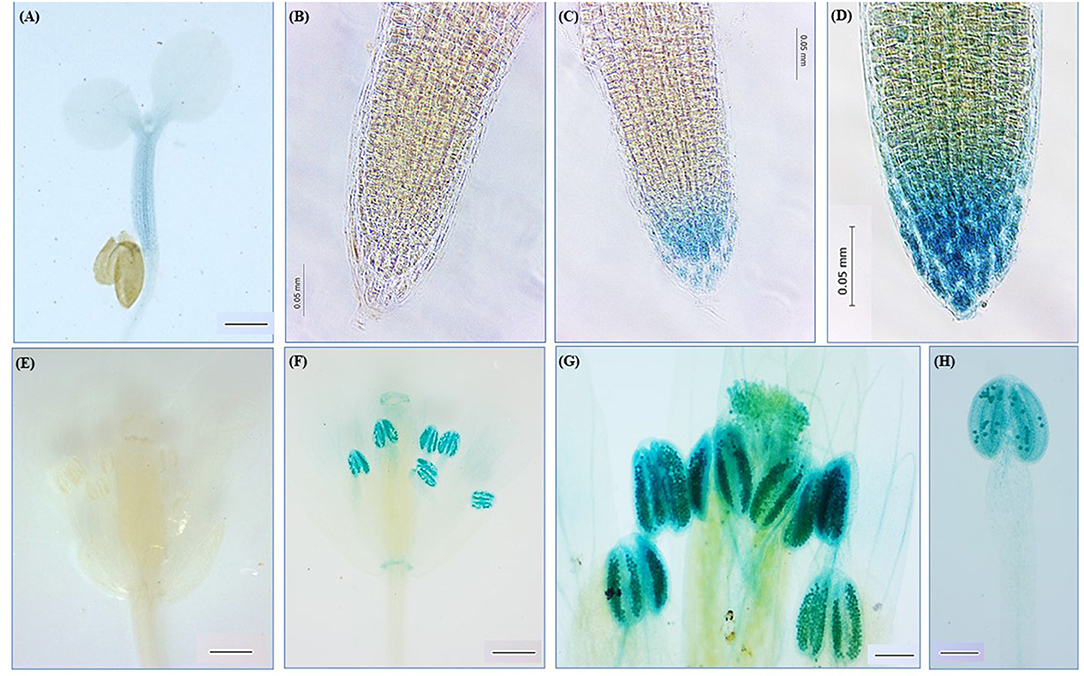
Figure 3. Histochemical assay of β-glucuronidase (GUS) activity in transgenic Arabidopsis thaliana plants expressing the AhN4L-1 promoter-GUS fusion gene. GUS activity was detected in the stem of germinated seedlings (A), the root tips (C,D), and the stamens and pistil (F–H) of transgenic plants. Images of roots (B) and flowers (E) of untransformed WT controls are shown. Bars = 100 μM in (A); (E,F) and 0.05 mm in (B–D,F–H).
Subcellular Localization of the AhN4L-1 Protein
To investigate the subcellular localization of the A. hypochondriacus AhN4L-1 protein, N-terminal GFP fusion constructs were generated and expressed in A. thaliana plants. The fluorescence emitted by the cells of the transgenic plants was analyzed using confocal microscopy. The localization of the green fluorescence emitted by A. thaliana transgenic plants able to constitutively express GFP- AhN4L-1 fusion proteins coincided with the GUS-activity detected in growing root tips (Figures 4C,D) and in segments of the pistil, around the style and pollen tube (Figures 4O,P), and in the anthers (Figures 4S,T). A GFP signal was also observed in the stomata of leaves (Figures 4K,L), suggesting a role in the regulation of stomatal movement, and in the root vasculature (Figures 4G,H). The images indicated that these proteins appeared as punctate agglomerates, perhaps in the periphery of protein bodies and/or the cell wall-protoplast interface. Equivalent images obtained from root tips (Figures 4A,B), roots (Figures 4E,F), leaf surface (Figures 4I,J), pistils (Figures 4M,N) and anthers (Figures 4Q,R) of untransformed control plants are shown.
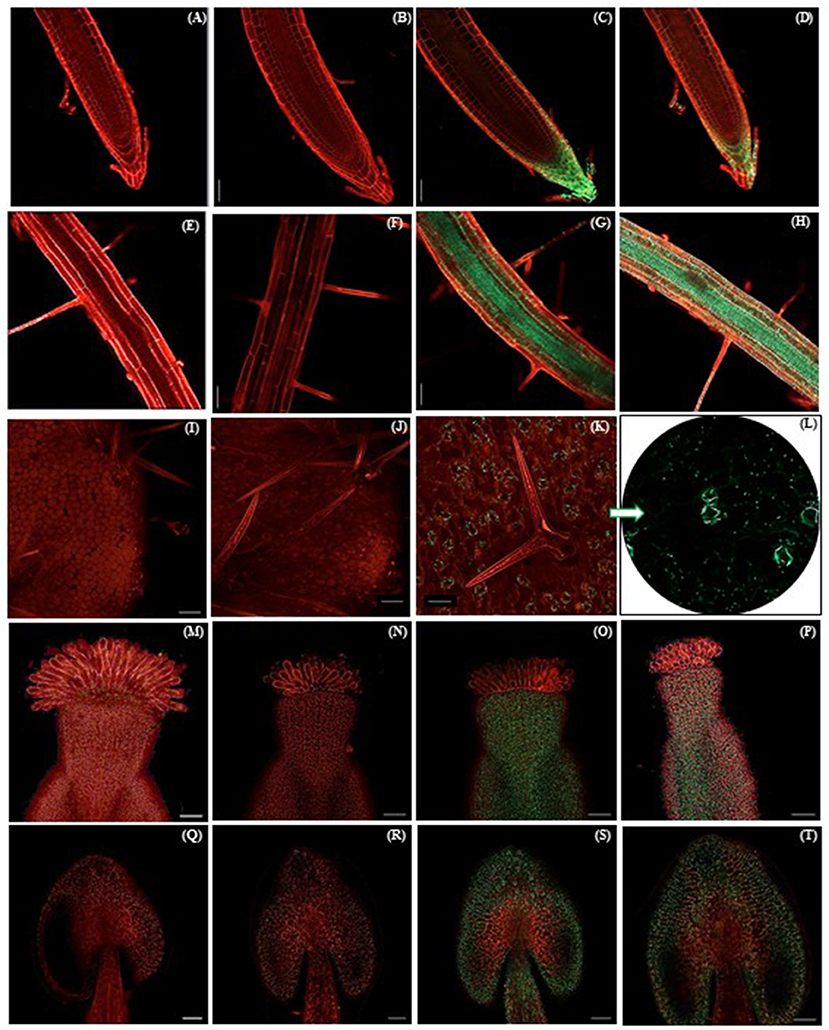
Figure 4. GFP fluorescence emission under UV light in different organs of transgenic Arabidopsis thaliana plants expressing the N-terminal eGFP::AhN4L-1 fusion gene. GFP emission against a background of propidium iodide stained tissues, was detected in the root tips (C,D), root vasculature and root hairs (G,H), stomata on the adaxial leaf surface (K,L), the base of the flower pistil (O,P) and the anthers (S,T). Images of propidium iodide stained root tips (A,B), root vasculature (E,F), the adaxial surface of leaves (I,J), pistils (M, N) and stamens (Q,R) of untransformed WT controls are shown. Bars = 0.05 mm in all mages.
Functional Characterization of AhN4L-1 Transgenic OE A. thaliana Plants
Three A. thaliana transgenic plants lines with differing AhN4L-1 expression levels (high [L17], middle [L5], and low [L6]) were selected for experimentation; see above). Various physiological parameters were affected in these plants. The germination percentage of seeds generated by the three transgenic plant lines, measured at 3, 6, and 9 days after placing on germinating media, was significantly lower than untransformed controls (Figure 5A). Although the number of leaves remained unchanged, they were significantly smaller in 30-day old transgenic OE lines (Figure 5B). The inflorescences were also significantly smaller than control plants (Figure 5C) whereas seed weight (Figure 5D), but not seed yield (Figure 5E), were generally lower in the transformed plants. The advent of flowering was similar in WT and transgenic plants. Root length was also significantly reduced in the transgenic A. thaliana plants (Figure 6). Compared to WT plants, the transgenic plants showed no increased tolerance to WDS or high salinity stress (Supplementary Figure S3 and G. Cabrales-Orona, personal communication). Despite the fact that AhN4L-1 overexpressing plants showed no increased salinity tolerance, they nevertheless maintained their significantly basal higher chlorophyll content plants after 21-days of saline stress. However, carotenes were mostly reduced in response to salt-stress, particularly in the L6 and L17 transgenic lines. The accumulation of proline and Suc in response to salt stress was also less pronounced in these transgenic plant lines, whereas the L17 line showed significantly higher Glc and Fru, but lower starch levels, in response to salt stress. No change in the total phenols and total flavonoids content due to salt stress, which were similar to those in WT plants, was observed in all three transgenic plant lines examined (Supplementary Figure S4). Also, no changes in CAT and SOD activity levels were observed between WT and transgenic plants kept under control and salinity stress conditions (Supplementary Figure S5). On the other hand, all the photosynthetic parameters tested (i.e., A, E, gs and WUE) remained above zero in the L6 and L17 transgenic lines under salt stress conditions, whereas, in WT plants, E and gs were significantly lower and A and WUE became negative (Supplementary Figure S6). Fv/Fm variations in response to stress were similar in WT and AhN4L-1 overexpressing plants (G. Cabrales-Orona, personal communication).
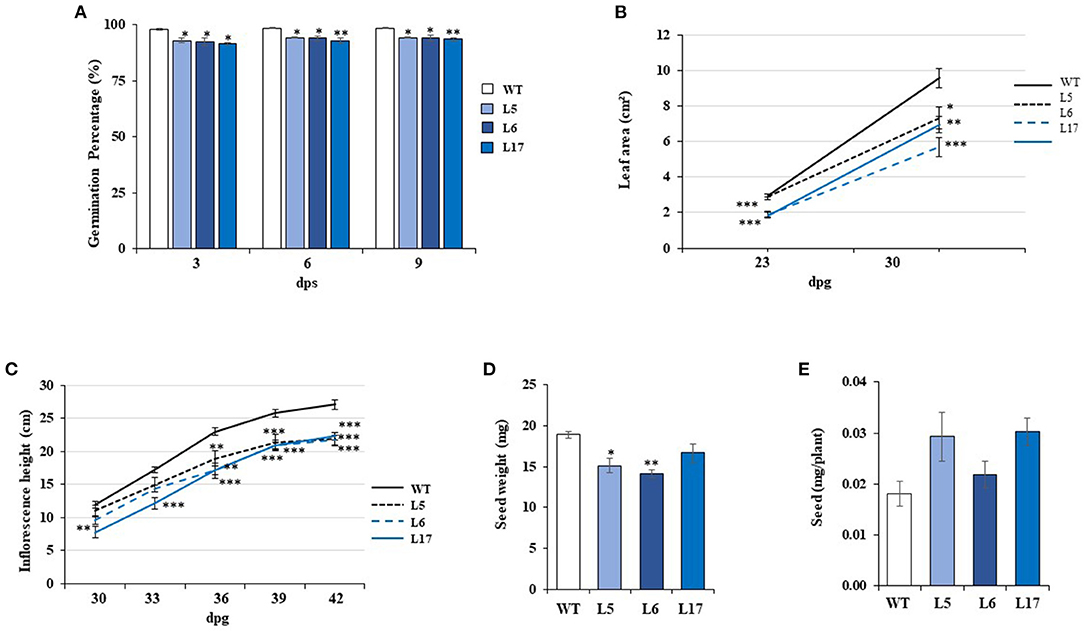
Figure 5. The overexpression of AhN4L-1 in Arabidopsis thaliana plants negatively affects germination and vegetative and reproductive growth. (A) Germination rate, (B) leaf area, (C) inflorescence length, (D) seed weight, and (E) seed yield, were determined in wild type plants (WT) and in three transgenic plant lines (L5, L6, and L17) overexpressing the AhN4L-1 gene at different dosage under long-day conditions. (E) The bars and points in the linear graphs represent the means ± SE of three independent experiments (n = 10). Asterisks over the bars denote significant differences at P < 0.05 (*), P < 0.01 (**), or P < 0.001 (***) (One-way ANOVA, Tukey-Kramer test).
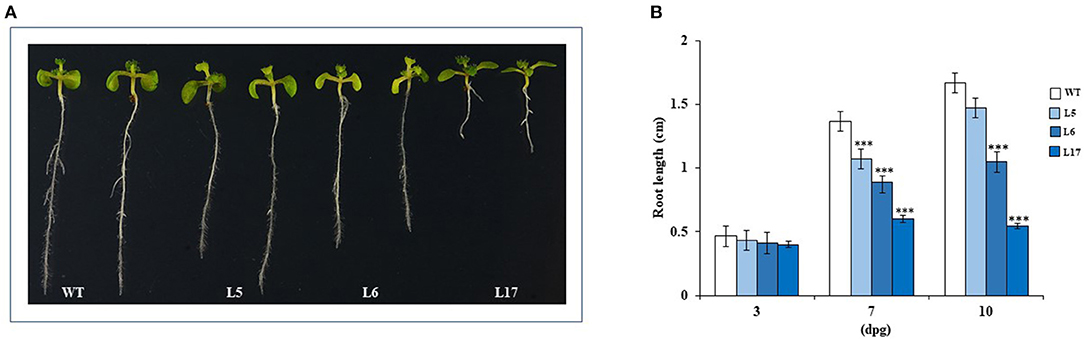
Figure 6. Root elongation is negatively altered in AhN4L-1 overexpressing Arabidopsis thaliana plants. (A) Comparison of root length between three lines of AhN4L-1 transgenic plant lines (L5, L6, and L17) having significantly different transgene expression dosage and wild-type (WT) untransformed plants. Seeds of WT and transgenic plant lines were germinated and grown on MS medium for 10 days. (B) Root length was measured at 3, 7, and 10 days after germination. The bars are means (n = 20) ± SE of three independent experiments. Asterisks over the bars denote statistically significant differences at P < 0.001 (***) (One-way ANOVA, Tukey-Kramer test).
In contrast to the abiotic stress assays, the overexpression of AhN4L-1 in A. thaliana plants conferred resistance to both biotrophic bacterial and hemi-biotrophic fungal pathogens in a dose-dependent manner, since it was mostly observed in the L17 line having the highest transgene expression levels, Thus, counts of Pst3000 CFUs were significantly lower after 3-day post-inoculation periods in this transgenic plant line (Figure 7A), whose plants were also less developmentally affected by bacterial infection (Figure 7B). Likewise, L17 transgenic line plants were significantly less susceptible to F. oxysporum fungal infection. They showed better and healthier vegetative and reproductive development (Figures 8A,B), characterized by less affectation of shoot and root growth (Figures 8C,D). Moreover, the pathogen load was lower, as determined by PCR amplification of the ITS marker gene sequence (Figure 8E).
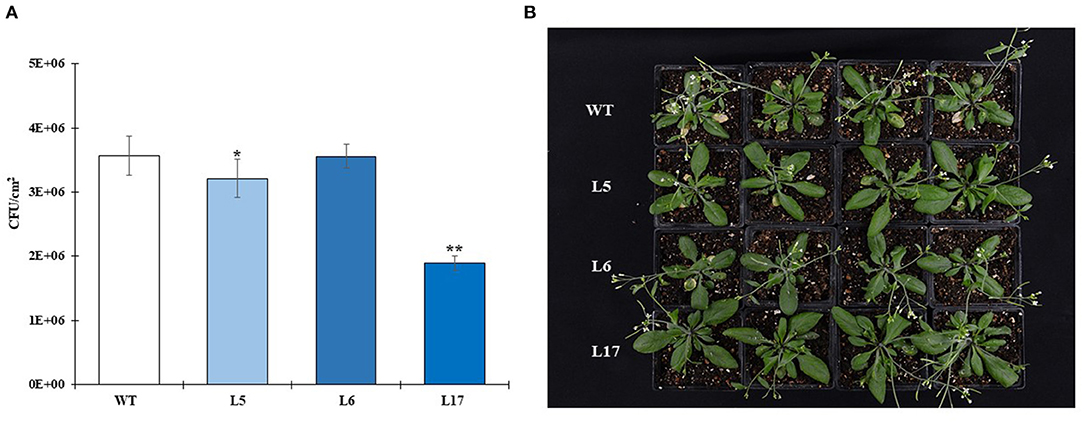
Figure 7. The overexpression of the AhN4L-1 gene in transgenic Arabidopsis thaliana plants confers resistance to bacterial infection in a dose-dependent manner. (A) The growth of Pseudomonas syringae pv. tomato DC3000 was evaluated 72 h after inoculation of 20-day old plants with 10 μL aliquots of a bacterial suspension containing colony forming units/mL (CFUs/mL) to three fully expanded leaves of each plant tested. These were transgenic plant lines (L5, L6, and L17) having significantly different transgene expression dosage and wild-type (WT) untransformed plants. The bars represent the mean number ± SE of CFUs in leaves of five plants for each line examined. Three independent experiments with similar results were performed. Asterisks over the bars denote significant differences at P < 0.05 (*) or P < 0.01 (**) (One-way ANOVA, Tukey-Kramer test). Three independent experiments were performed with similar results. (B) Bacterial infection had a more drastic effect on the growth of WT plants, which also showed increased disease symptoms in the inoculated leaves. The protection to bacterial infection in the transgenic lines was more pronounced in the L17 line having the highest transgene expression levels.
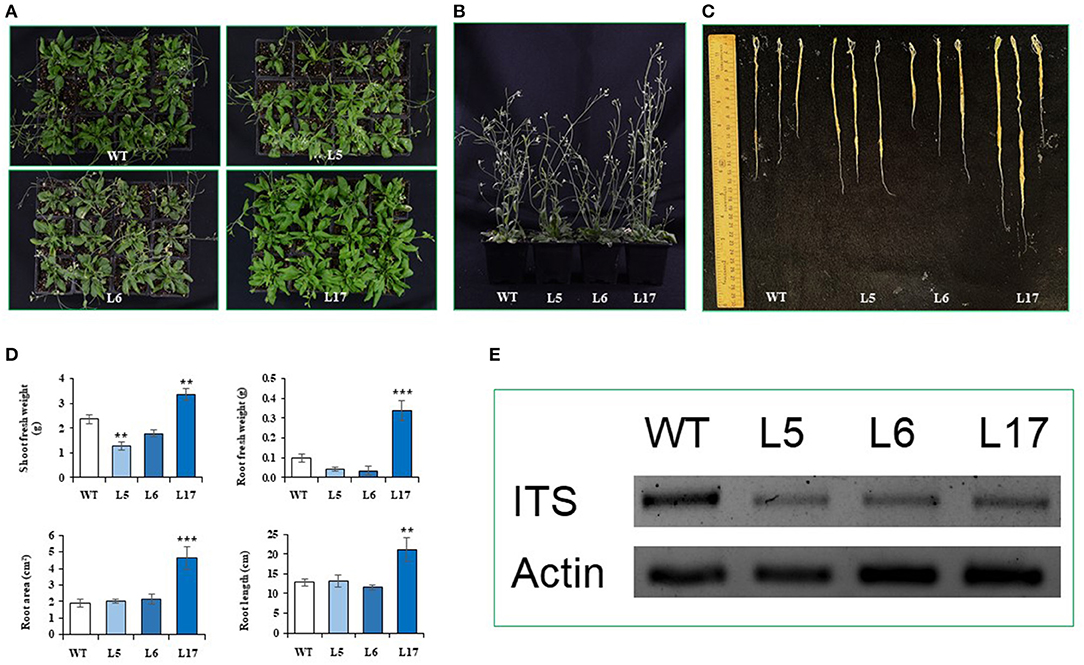
Figure 8. The overexpression of the AhN4L-1 gene in transgenic Arabidopsis thaliana plants confers resistance to fungal infection in a dose-dependent manner. Growth-related parameters were differentially affected in untransformed wild-type (WT) plants compared to transgenic plant lines (L5, L6, L17) overexpressing the AhN4L-1 gene in dose-dependent manner is shown 25 days after infection, via soil drench, with a spore suspension (1 × 106 spores) of Fusarium oxysporum f. sp. lycopersici as shown by better (A) vegetative plant growth, (B) inflorescence height and (C) root length observed in the L17 line having the highest transgene expression levels. This was supported by data shown in (D) where root and shoot fresh weights as well as root area and root length were significantly higher in the L17 line. Here, the bars represent the mean values ± SE of these parameters obtained from five plants of each line examined. Asterisks over the bars denote significant differences at P < 0.01 (**) or P < 0.001 (***) (One-way ANOVA, Tukey-Kramer test). Three independent experiments with similar results were performed. (E) PCR amplicons obtained using the ITS1/ITS4 primers (refer to Table 1) and DNA extracted from roots of untransformed (WT) and transgenic (L5, L6, and L17) A. thaliana plants 25 days after infection with spores of F. oxysporum f. sp. lycopersici. The amplification of the constitutively expressed A. thaliana Actin gene was used as loading standard.
Discussion
This study describes the functional characterization of a grain amaranth Natterin-like-1 (AhN4L-1) gene. The phylogenetic analysis indicated that the AhN4L-1 protein coded by this gene, although modularly similar to AAT proteins having amaranthin-like proteins linked to aerolysin domains previously described in A. hypochondriacus, cucumber, wheat and several other plants species (Dang et al., 2017) was dissimilar enough to be grouped into a well-defined and different clade together with few other distantly related proteins in B. vulgaris and C. quinoa. It was also different from a Nattering-4 protein from Triticum urartu recently included in a report describing the identification of Natterin and Natterin-like proteins across a wide range of organisms including, in addition to the fish species where these proteins were initially discovered and characterized, several insects, birds, plants and others (Lima et al., 2021). The phylogenetic distance from these proteins could have been partly due to the presence of agglutinin domains, instead of the predominant amaranthin AA lectin domains, in the N-terminal end of the AhN4L-1 protein.
The expression pattern of the AhN4L-1 in grain amaranths plants subjected to different stress conditions indicated that this gene could be involved in the protective responses against several biotic and abiotic insults, including water deficit stress, high salinity, severe defoliation, insect herbivory and bacterial infection. Likewise, the strong expression levels of this gene detected in leaves that had lost two thirds of their surface area during the defoliation treatments and also in distal root and stem tissues of completely defoliated plants was indicative that this gene may be also induced locally and systemically in response to mechanical wounding. The activated expression of the AhN4L-1 gene by the exposure to several biotic and abiotic stimuli, including wounding and insect herbivory, was similar to the characteristic behavior of a group of multiple stress-inducible lectins that are believed to play a role in plant defense (Van Damme et al., 2004; Lannoo et al., 2007). Moreover, the observed induction by biotic stressors was in agreement with the widespread consideration of Natterin proteins as components of immune defense responses, first suggested after the detection of highly toxic Natterin protein-containing venoms in the Brazilian toadfish Thalassophryne nattereri and various other fish species (Lima et al., 2021). It also coincided with data showing that transcript abundance of ATT genes in cucumber was increased by cold, salt and drought stresses (Dang et al., 2017) and was consistent with the induced expression of the Hfr-2 gene, coding for an AAT domain wheat protein, observed in response to infestation by highly damaging insect pests such as the Hessian fly (Mayetiola destructor), the fall armyworm (Spodoptera frugiperda), and the bird cherry-oat aphid (Rhopalosiphum padi) (Puthoff et al., 2005). The proposed involvement of these proteins in plant defense was further suggested by studies showing that: i) the OE of amaranthin in transgenic cotton and potato plants increased their resistance against aphids (Wu et al., 2006; Yang et al., 2011); ii) the insecticidal effects caused by the Nicotiana tabacum agglutinin (or Nictaba), amaranthin, and other lectins (Chen et al., 2002; Vandenborre et al., 2011) and iii) the observation that several transcripts coding for flax (Linum usitatissimum) amaranthin-like lectins were strongly induced by the exogenous application of the defense-related phytohormones methyl jasmonate or salicylic acid (Faruque et al., 2015). These plant protective properties, associated with reduced insect survival, fertility, feeding, and delayed development (Macedo et al., 2015), have been proposed to stem from their lectin domains, sometimes in combination with the aerolysin domain. Thus, plant lectins are assumed to exert their biological effect by perceiving environmental signals through the reversible binding of carbohydrates and/or by lectin-mediated protein-carbohydrate interactions in the cytoplasm and nucleus, that are translated into phenotypical responses (Esch and Schaffrath, 2017). Curiously, and contrary to the transcriptomic data generated in grain amaranths, the overexpression of AhN4L-1 in A. thaliana provided no protection against herbivory by chewing Manduca sexta larvae (G. Cabrales-Orona, personal communication).
The GUS and GFP localization assays in transgenic A. thaliana plants provided additional information about the possible function, in plants, of the AhN4L-1 protein. As previously reported for wheat germ agglutinin (WGA) (Mishkind et al., 1982; Dubiel et al., 2020a), the highly localized accumulation of both GUS and GFP signals in root tips was in concordance with the demonstrated defensive function of agglutinin-containing proteins against soil fungal pathogens (Mirelman et al., 1975). This localization also agreed with the anti-fungal effect against F. oxysporum, a notorious soil-borne pathogen of several plant crops, observed in overexpressing AhN4L-1 A. thaliana plants. In addition to AhN4L-1, several other lectins have been reported to suppress fungal growth, although less efficiently than the well-known pathogenesis-related defensin and thionin proteins (Tsaneva and Van Damme, 2020; Petrova et al., 2021).
Similar to the subcellular localization of WGA, AhN4L-1 appeared to be located in the periphery of protein bodies and in the cell wall-protoplast interface. However, and contrary to WGA, AhN4L-1 was visualized in the vasculature of the stems of young seedlings and in the vascular tissues of adult plant roots, as well as in the leaves, specifically in the stomata, and in floral tissues. The localization of the AhN4L-1 protein in stomata further supports its role as a possible physiological factor regulating stomatal movement, similar to a particular Arabidopsis lectin (Van Hove et al., 2015), that could have a defensive function against bacterial pathogens. The possible participation in stomatal innate immunity signaling has also been reported for lectin receptor kinases, and others (Singh and Zimmerli, 2013; Wang and Bouwmeester, 2017). The proposed relation between stomatal localization and immunity against bacterial pathogens was in accordance with the dose-dependent resistance against Pseudomonas infection observed in line 17 of AhN4L-1 overexpressing A. thaliana transgenic plants.
The distinctive localization of the AhN4L-1 protein in floral tissues also agreed with ample experimental evidence supporting the functionality of lectins in the flowering process and/or in the protection of the vulnerable floral organs. It coincided with the transcript accumulation of amaranthin-like proteins with AAT domains in floral tissues of flax (Faruque et al., 2015) and Rumex acetosa (Manzano et al., 2017) and with a previous study showing that transcripts encoding the Nictaba agglutinin accumulated in the stigma/style of tobacco plants. The results of the latter study were interpreted as a developmentally regulated pistil-specific defense response to protect the nutrient rich stigma/style from potential pathogen attack (Quiapim et al., 2009). In another study, the expression of an agglutinin gene in the stems and both male and female flowers of Castanea crenata trees was interpreted as a possible regulatory step controlling the interaction between male gametophyte and pistil sporophytic tissues (Nakamura et al., 2004). This evidence concurs with other reports describing the localization of lectins in floral tissues of several other plant species where they have been proposed to play roles in diverse reproduction-related processes such as pollen and pollen tube development, pollen germination, male fertility and self and non-self pollen-stigma interactions (Clarke and Knox, 1978; Wu et al., 1993; Santos Sousa et al., 2013; Vakhania et al., 2014; Peng et al., 2020). Additionally, the localization of AhN4L-1 in the abscission zone of the stigma and stamens suggested the possibility that this protein could also be involved in the abscission of floral organs in plants, which is a process that requires the generation of weakened abscission zones produced, among other factors, by the activity of cell wall remodeling hemicellulose- and pectin-degrading proteins (González-Carranza et al., 2002, 2012). Thus, the proposed participation of the AhN4L-1 protein in floral organ abscission could be associated with a yet to be defined capacity to bind to galacturonic acids, similar to D-galactose-specific Ricinus communis agglutinins and to a pumpkin plant lectin able to bind rhamnogalacturonan-I containing pectic polysaccharides (Benhamou et al., 1988; Itakura et al., 2007; Zhao et al., 2017). This function was also in agreement with the detection of transcripts coding for a mannose-binding lectin and a jacalin lectin family protein, respectively, in laser-micro dissected stamen abscission zones in A. thaliana (Cai and Lashbrook, 2008).
The overexpression of the AhN4L-1 gene in A. thaliana provided no increased protection against WDS and salinity stress. This occurred despite the maintenance of significantly higher chlorophyll, Glc and Fru accumulation and sustained parameters of photosynthetic activity and efficiency, at least in the L17 line, under salt-stress conditions. However, they were in accordance with a generally weaker proline accumulation in the salt-stressed transgenic plants. This outcome was also incompatible with the robust induction of this gene in grain amaranth plants subjected to diverse abiotic stresses, with the reported transcript accumulation of several cucumber ATT protein genes in response to cold, drought, salt, and exogenous ABA treatment (Dang et al., 2017) and with the drought-responsive nature of a Natterin-4 gene in maize (Jin et al., 2019). It was also contrary to the response and stress-triggered re-localization into stress granules of an ABA-inducible A. thaliana Euonymus europaeus-related lectin, ArathEULS3, homologous to the E. europaeus agglutinin (Fouquaert et al., 2009), also activated by drought, salt, and osmotic stresses (Van Hove et al., 2015; Dubiel et al., 2020a) and to the tolerance to salt stress observed in A. thaliana transgenic plants overexpressing a soybean agglutinin presumed to interact with the core histones H2A, H2B, and H4 (Van Holle et al., 2016).
The negative effect on vegetative and reproductive growth caused by the overexpression of this gene in A. thaliana was also an unexpected outcome considering that is was contrary to published data describing lectin-induced mitosis in some root apical meristem tissues (Bonner, 2009) and to lectin-dependent modulation of sugar metabolism and/or signaling leading to increased plant productivity (Nonomura et al., 2020; Moradi et al., 2021). On the other hand, it was in agreement with a study in which detrimental effects on plant growth and development were reported in A. thaliana plants overexpressing a lectin receptor kinase used as a sensor for extracellular nicotinamide adenine dinucleotide (Wang et al., 2017) and with the suppressed growth observed in A. cruentus hairy roots transformed with AhN4L-2, an homolog of AhN4L-1 (Castellanos-Arévalo, 2020). It is possible that the negative effects on plants development observed in AhN4L-1 OE transgenic A. thaliana plants were the result of fitness costs often associated with plants manipulated to have enhanced disease resistance (Bowling et al., 1994; Mauch et al., 2001) and/or due to a yet to be determined toxic effect caused by the undue accumulation of the AhN4L-1 protein. Another possible explanation of the marked root growth inhibition produced by AhN4L-1 overexpression could invoke the effects caused by a knock-out mutation of the ArathEULS3 lectin in Arabidopsis plants, which produced significantly longer primary roots, presumably as a result of reduced ABA sensitivity (Dubiel et al., 2020b). This similarity suggests that the development-related effects produced by the over expression of AhN4L-1 in Arabidopsis could have been partially due to an association with ABA signaling. Support for this proposition can be derived from the fact that both AhN4L-1 and the ArathEULS3 lectin are thought to be involved in the regulation of stomatal closure (see above). Moreover, the eventual recovery of the root system in AhN4L-1 OE plants, whose development in adult plant was not different from that in untransformed Arabidopsis plants, suggests that the negative effects on vegetative and reproductive development produced as a consequence of overexpression of this gene could be attenuated concomitantly with the development of the plants, similar to experimental evidence showing that Arabidopsis roots can display different growth responses to ABA and ABA-regulated stress responses depending on their rank and age (Rosales et al., 2019).
Conclusions
This study provided a functional characterization of a Natterin-4-like gene, AhN4L-1, an unknown function gene originally identified in a transcriptomic analysis as a stress-inducible gene in A. hypochondriacus. That property was confirmed by quantitative PCR data showing a widespread responsiveness to biotic and abiotic stress conditions in all three grain amaranth species. However, its overexpression in transgenic A. thaliana plants was unable to confer increased tolerance to water deficit and salinity stress conditions, indicating that its abiotic stress functionality might depend on the presence of other, unidentified, genes. However, additional evidence gathered from transgenic A. thaliana plants clearly suggest a role in defense against bacterial and fungal pathogens, particularly in roots tips, leaves and floral tissues. Part of this defensive function could be associated with a control of stomatal movement, as suggested by the localization of the AhN4L-1 protein leaf stomata. A role in the regulation of other reproductive processes is also possible. These properties coincided with those reported for agglutinin containing lectins, other lectin types and with AAT proteins and were supportive of the physiological importance of the Natterin and Natterin-like proteins in plants.
Data Availability Statement
The original contributions presented in the study are included in the article/Supplementary Materials. Further inquiries can be directed to the corresponding authors.
Author Contributions
JD-F and GC-O: conceived and designed the experiments. GC-O and NM-G: performed the experiments. JD-F, NM-G, and GC-O: analyzed the data. JD-F: wrote the paper. JD-F and GC-O: revised the manuscript. JD-F, GC-O, and NM-G: contributed reagents, materials, and analysis tools. All authors read and approved the final version of the manuscript.
Funding
This research was funded by CONACyT, México (Grant 707403, to GC-O).
Conflict of Interest
The authors declare that the research was conducted in the absence of any commercial or financial relationships that could be construed as a potential conflict of interest.
Publisher's Note
All claims expressed in this article are solely those of the authors and do not necessarily represent those of their affiliated organizations, or those of the publisher, the editors and the reviewers. Any product that may be evaluated in this article, or claim that may be made by its manufacturer, is not guaranteed or endorsed by the publisher.
Supplementary Material
The Supplementary Material for this article can be found online at: https://www.frontiersin.org/articles/10.3389/fsufs.2021.814188/full#supplementary-material
References
Achigan-Dako, E. G., Sogbohossou, O. E. D., and Maundu, P. (2014). Current knowledge on Amaranthus spp.: research avenues for improved nutritional value and yield in leafy amaranths in sub-Saharan Africa. Euphytica 197, 303–317. doi: 10.1007/s10681-014-1081-9
Adhikary, D., and Pratt, D. B. (2015). Morphologic and taxonomic analysis of the weedy and cultivated Amaranthus hybridus species complex. Syst. Bot. 40, 604–610. doi: 10.1600/036364415X688376
Aguilar-Hernández, H. S., Santos, L., León-Galván, F., Barrera-Pacheco, A., Espitia-Rangel, E., De León-Rodríguez, A., et al. (2011). Identification of calcium stress induced genes in amaranth leaves through suppression subtractive hybridization. J. Plant Physiol. 168, 2102–2109. doi: 10.1016/j.jplph.2011.06.006
Arnon, D. I. (1949). Copper enzymes in isolated chloroplasts. polyphenol oxidase in Beta vulgaris. Plant Physiol. 24, 1–15. doi: 10.1104/pp.24.1.1
Artiola, J. F., Walworth, J. L., Musil, S. A., and Crimmins, M. A. (2019). “Soil and Land Pollution” in Environmental and Pollution Science,3rd Edn. eds Brusseau, M., Pepper, I., and Gerba, C. (Netherlands: Academic Press; Elsevier), 219–235. doi: 10.1016/B978-0-12-814719-1.00014-8
Bates, L. S., Waldren, R. P., and Teare, I. D. (1973). Rapid determination of free proline for water-stress studies. Plant Soil 39, 205–207. doi: 10.1007/BF00018060
Benhamou, N., Chamberland, H., Ouellette, G. B., and Pauzé, F. J. (1988). Detection of galactose in two fungi causing wilt diseases and in their host tissues by means of gold-complexed Ricinus communis agglutinin I. Physiol. Mol. Plant Pathol. 32, 249–266. doi: 10.1016/S0885-5765(88)80021-3
Biehler, E., Mayer, F., Hoffmann, L., Krause, E., and Bohn, T. (2010). Comparison of 3 spectrophotometric methods for carotenoid determination in frequently consumed fruits and vegetables. J. Food Sci. 75, C55–C61. doi: 10.1111/j.1750-3841.2009.01417.x
Bowling, S. A., Guo, A., Cao, H., Gordon, A. S., Klessig, D. F., and Dong, X. (1994). A mutation in Arabidopsis that leads to constitutive expression of systemic acquired resistance. Plant Cell 6, 1845–1857. doi: 10.1105/tpc.6.12.1845
Brenner, D., Baltensperger, D., Kulakow, P., Lehmann, J., Myers, R., Slabbert, M., et al. (2000). Genetic resources and breeding of Amaranthus. Plant Breed. Rev. 19, 227–285. doi: 10.1002/9780470650172.ch7
Cabrales-Orona, G. (2017). Análisis de genes de función desconocida de amaranto de grano que se expresan en múltiples condiciones de estrés. [Dissertation/master's thesis]. [Irapuato, Gto., Mexico]: Cinvestav, Unidad Irapuato.
Cabrales-Orona, G., and Délano, Frier, J. P. (2021). “Searching for an identity: Functional characterization of taxonomically restricted genes in grain amaranth” in The Amaranth Genome, eds D. Adhikary, M. K. Deyholos, and J. P. Délano-Frier (Springer Nature), 97–124. doi: 10.1007/978-3-030-72365-1_7
Cai, S., and Lashbrook, C. C. (2008). Stamen abscission zone transcriptome profiling reveals new candidates for abscission control: enhanced retention of floral organs in transgenic plants overexpressing Arabidopsis ZINC FINGER PROTEIN2. Plant Physiol. 146, 1305–1321. doi: 10.1104/pp.107.110908
Casarrubias-Castillo, K., Martínez-Gallardo, N. A., and Délano-Frier, J. P. (2014). Treatment of Amaranthus cruentus with chemical and biological inducers of resistance has contrasting effects on fitness and protection against compatible Gram positive and Gram negative bacterial pathogens. J. Plant Physiol. 171, 927–939. doi: 10.1016/j.jplph.2014.02.004
Caselato-Sousa, V. M., and Amaya-Farfán, J. (2012). State of knowledge on amaranth grain: a comprehensive review. J. Food Sci. 77, R93–R104. doi: 10.1111/j.1750-3841.2012.02645.x
Castellanos-Arévalo (2020). Transformación genética de amaranto de grano mediada por Agrobacterium rhizogenes y su potencial biotecnológico. [Dissertation/Ph.D thesis]. [Irapuato, Gto., Mexico]: Cinvestav, Unidad Irapuato.
Castrillón-Arbeláez, P. A., Martinez-Gallardo, N., Arnaut, H. A., Tiessen, A., and Delano-Frier, J. P. (2012). Metabolic and enzymatic changes associated with carbon mobilization, utilization and replenishment triggered in grain amaranth (Amaranthus cruentus) in response to partial defoliation by mechanical injury or insect herbivory. BMC Plant Biol. 12, 163. doi: 10.1186/1471-2229-12-163
Chen, L. L., Xie, J., Cao, D. D., Jia, N., Li, Y. J., Sun, H., et al. (2018). The pore-forming protein Aep1 is an innate immune molecule that prevents zebrafish from bacterial infection. Dev. Comp. Immunol. 82, 49–54. doi: 10.1016/j.dci.2018.01.003
Chen, Y., Peumans, W. J., Hause, B., Bras, J., Kumar, M., Proost, P., et al. (2002). Jasmonic acid methyl ester induces the synthesis of a cytoplasmic/nuclear chito-oligosaccharide binding lectin in tobacco leaves. FASEB J. 16, 905–907. doi: 10.1096/fj.01-0598fje
Cisneros-Hernández, I., Vargas-Ortiz, E., Sánchez-Martínez, E. S., Martínez-Gallardo, N., Soto González, D., and Délano-Frier, J. P. (2021). Highest defoliation tolerance in Amaranthus cruentus plants at panicle development is associated with sugar starvation responses. Front. Plant Sci. 12, 658977. doi: 10.3389/fpls.2021.658977
Clarke, A. E., and Knox, R. B. (1978). Cell recognition in flowering plants. Q. Rev. Biol. 53:3. doi: 10.1086/410333
Clough, S. J., and Bent, A. F. (1998). Floral dip: a simplified method for Agrobacterium-mediated transformation of Arabidopsis thaliana. Plant J. 16, 735–743. doi: 10.1046/j.1365-313x.1998.00343.x
Dang, L., Roug,é, P., and Van Damme, E. J. M. (2017). Amaranthin-like proteins with aerolysin domains in plants. Front. Plant Sci. 8:1368. doi: 10.3389/fpls.2017.01368
Das, S. (2016). “Distribution and maintenance of amaranth germplasm worldwide,” in Amaranthus: A Promising Crop of Future ed. Das S. (Berlin: Springer) 99–106. doi: 10.1007/978-981-10-1469-7_7
De Ron, A. M., Sparvoli, F., Pueyo, J. J., and Bazile, D. (2017). Protein crops: food and feed for the future. Front. Plant Sci. 8:105. doi: 10.3389/fpls.2017.00105
Délano-Frier, J. P., Avilés-Arnaut, H., Casarrubias-Castillo, K., Casique-Arroyo, G., Castrillón-Arbeláez, P. A., Herrera-Estrella, L., et al. (2011). Transcriptomic analysis of grain amaranth (Amaranthus hypochondriacus) using 454 pyrosequencing: comparison with A. tuberculatus, expression profiling in stems and in response to biotic and abiotic stress. BMC Genomics 12:363. doi: 10.1186/1471-2164-12-363
Délano-Frier, J. P., Martínez-Gallardo, N. A., Martínez-de la Vega, O., Salas-Araiza, M., Barbosa-Jaramillo, E. R., Torres, A., et al. (2004). The effect of exogenous jasmonic acid on induced resistance and productivity in amaranth (Amaranthus hypochondriacus) is influenced by environmental conditions. J. Chem. Ecol. 30, 1001–1034. doi: 10.1023/B:JOEC.0000028464.36353.bb
DOF (2002). NOM-021-RECNAT-2000. Especificaciones de fertilidad, salinidad y clasificación de suelos, estudio, muestreo y análisis. Diario Oficial de la Federación. D.F., México.
Dubiel, M., Beeckman, T., Smagghe, G., and Van Damme, E. J. M. (2020b). Arabidopsis lectin EULS3 is involved in ABA signaling in roots. Front. Plant Sci. 11:437. doi: 10.3389/fpls.2020.00437
Dubiel, M., De Coninck, T., Osterne, V. J. S., Verbeke, I., Van Damme, D., Smagghe, G., et al. (2020a). The ArathEULS3 lectin ends up in stress granules and can follow an unconventional route for secretion. Int. J. Mol. Sci. 21:1659. doi: 10.3390/ijms21051659
Esch, L., and Schaffrath, U. (2017). An update on jacalin-like lectins and their role in plant defense. Int. J. Mol. Sci. 18:1592. doi: 10.3390/ijms18071592
Faruque, K., Begam, R., and Deyholos, M. K. (2015). The amaranthin-like lectin (LuALL) genes of flax: a unique gene family with members inducible by defence hormones. Plant Mol. Biol. Report. 33, 731–741. doi: 10.1007/s11105-014-0791-4
Fouquaert, E., Peumans, W. J., Vandekerckhove, T. T. M., Ongenaert, M., and Van Damme, E. J. M. (2009). Proteins with an Euonymus lectin-like domain are ubiquitous in Embryophyta. BMC Plant Biol. 9:136. doi: 10.1186/1471-2229-9-136
Gacesa, R., Chung, R., Dunn, S. R., Weston, A. J., Jaimes-Becerra, A., Marques, A. C., et al. (2015). Gene duplications are extensive and contribute significantly to the toxic proteome of nematocysts isolated from Acropora digitifera (Cnidaria: Anthozoa: Scleractinia). BMC Genom. 16:774. doi: 10.1186/s12864-015-1976-4
González-Carranza, Z. H., Shahid, A. A., Zhang, L., Liu, Y., Ninsuwan, U., and Roberts, J. A. (2012). A novel approach to dissect the abscission process in Arabidopsis. Plant Physiol. 160, 1342–1356. doi: 10.1104/pp.112.205955
González-Carranza, Z. H., Whitelaw, C. A., Swarup, R., and Roberts, J. A. (2002). Temporal and spatial expression of a polygalacturonase during leaf and flower abscission in oilseed rape and Arabidopsis. Plant Physiol. 128, 534–543. doi: 10.1104/pp.010610
González-Rodríguez, T., Cisneros-Hernández, I., Acosta Bayona, J., Ramírez-Chavez, E., Martínez-Gallardo, N., Mellado-Mojica, E., et al. (2019). Identification of factors linked to higher water-deficit stress tolerance in Amaranthus hypochondriacus compared to other grain amaranths and A. hybridus, their shared ancestor. Plants 8:239. doi: 10.3390/plants8070239
Goodstein, D. M., Shu, S., Howson, R., Neupane, R., Hayes, R. D., Fazo, J., et al. (2012). Phytozome: a comparative platform for green plant genomics. Nucleic Acids Res. 40, D1178–D1186. doi: 10.1093/nar/gkr944
Gudbrandsson, J., Ahi, E. P., Franzdottir, S. R., Kapralova, K. H., Kristjansson, B. K., Steinhaeuser, S. S., et al. (2015). The developmental transcriptome of contrasting Arctic charr (Salvelinus alpinus) morphs. F1000Res. 4:136. doi: 10.12688/f1000research.6402.1
Higo, K., Ugawa, Y., Iwamoto, M., and Korenaga, T. (1999). Plant cis-acting regulatory DNA elements (PLACE) database: 1999. Nucleic Acids Res. 27, 297–300. doi: 10.1093/nar/27.1.297
Huerta-Ocampo, J. A., Barrera-Pacheco, A., Mendoza-Hernández, C. S., Espitia-Rangel, E., Mock, H. P., and Barba de la Rosa, A. P. (2014). Salt stress-induced alterations in the root proteome of Amaranthus cruentus L. J. Proteome Res. 13, 3607–3627. doi: 10.1021/pr500153m
Huerta-Ocampo, J. A., Leon-Galvan, M. F., Ortega-Cruz, L. B., Barrera-Pacheco, A., De Leon-Rodriguez, A., Mendoza-Hernández, G., et al. (2011). Water stress induces up-regulation of DOF1 and MIF1 transcription factors and down-regulation of proteins involved in secondary metabolism in amaranth roots (Amaranthus hypochondriacus L.). Plant Biol. 13, 472–482. doi: 10.1111/j.1438-8677.2010.00391.x
Itakura, Y., Nakamura-Tsuruta, S., Kominami, J., Sharon, N., Kasai, K., and Hirabayashi, J. (2007). Systematic comparison of oligosaccharide specificity of Ricinus communis agglutinin I and Erythrina lectins: a search by frontal affinity chromatography. J. Biochem. 142, 459–469. doi: 10.1093/jb/mvm153
Jefferson, R. A., Kavanagh, T. A., and Bevan, M. W. (1987). GUS fusions: beta-glucuronidase as a sensitive and versatile gene fusion marker in higher plants. EMBO J. 6, 3901–3907. doi: 10.1002/j.1460-2075.1987.tb02730.x
Jin, H., Liu, S., Zenda, T., Wang, X., Liu, G., and Duan, H. (2019). Maize leaves drought-responsive genes revealed by comparative transcriptome of two cultivars during the filling stage. PLoS ONE 14:e0223786. doi: 10.1371/journal.pone.0223786
Johnson, B. L., and Henderson, T. L. (2002). Water use patterns of grain amaranth in the northern Great Plains. Agron. J. 94, 1437–1443. doi: 10.2134/agronj2002.1437
Jones, D. T., Taylor, W. R., and Thornton, J. M. (1992). The rapid generation of mutation data matrices from protein sequences. Comput. Appl. Biosci. 8, 275–282. doi: 10.1093/bioinformatics/8.3.275
Joshi, D. C., Sood, S., Hosahatti, R., Kant, L., Pattanayak, A., Kumar, A., et al. (2018). From zero to hero: the past, present and future of grain amaranth breeding. Theor. Appl. Genet. 131, 1807–1823. doi: 10.1007/s00122-018-3138-y
Karimi, M., Inz,é, D., and Depicker, A. (2002). GATEWAY vectors for Agrobacterium- mediated plant. Trends Plant Sci. 7, 193–195. doi: 10.1016/S1360-1385(02)02251-3
Kietlinski, K. D., Jimenez, F., Jellen, E. N., Maughan, P. J., Smith, S. M., and Pratt, D. B. (2014). Relationships between the weedy Amaranthus hybridus (Amaranthaceae) and the grain amaranths. Crop Sci. 54, 220–228. doi: 10.2135/cropsci2013.03.0173
Lal, A., and Edwards, E. (1996). Analysis of inhibition of photosynthesis under water stress in the C4 species Amaranthus cruentus and Zea mays: Electron transport, CO2 fixation and carboxylation capacity. Aust. J. Plant Physiol. 23, 403–412. doi: 10.1071/PP9960403
Lannoo, N., Vandenborre, G., Miersch, O., Smagghe, G., Wasternack, C., Peumans, W J., et al. (2007). The jasmonate-induced expression of the Nicotiana tabacum leaf lectin. Plant Cell Physiol. 48, 1207–1218. doi: 10.1093/pcp/pcm090
Lima, C., Disner, G. R., Falcão, M. A. P., Seni-Silva, A. C., Maleski, A. L. A., Souza, M. M., et al. (2021). The Natterin proteins diversity: a review on phylogeny, structure, and immune function. Toxins 13:538. doi: 10.3390/toxins13080538
Livak, K. J., and Schmittgen, T. D. (2001). Analysis of relative gene expression data using real-time quantitative PCR and the 2−ΔΔCt method. Methods 25, 402–408. doi: 10.1006/meth.2001.1262
Lopes-Ferreira, M., Barbaro, K. C., Cardoso, D. F., Moura-Da-Silva, A. M., and Mota, I. (1998). Thalassophryne nattereri fish venom: biological and biochemical characterization and serum neutralization of its toxic activities. Toxicon 36, 405–410. doi: 10.1016/S0041-0101(97)00115-3
Macedo, M. L. R., Oliveira, C. F., and Oliveira, C. T. (2015). Insecticidal activity of plant lectins and potential application in crop protection. Molecules 20, 2014–2033. doi: 10.3390/molecules20022014
Manzano, S., Megias, Z., Martinez, C., Garcia, A., Aguado, E., Chileh, T., et al. (2017). Overexpression of a flower-specific aerolysin-like protein from the dioecious plant Rumex acetosa alters flower development and induces male sterility in transgenic tobacco. Plant J. 89, 58–72. doi: 10.1111/tpj.13322
Maranz, S., Wiesman, Z., and Garti, N. (2003). Phenolic constituents of shea (Vitellaria paradoxa) kernels. J. Agric. Food Chem. 51, 6268–6273. doi: 10.1021/jf034687t
Massange-Sánchez, J. A., Palmeros-Suárez, P. A., Espitia-Rangel, E., Rodríguez-Arévalo, I., Sánchez-Segura, L., Martínez-Gallardo, N. A., et al. (2016). Overexpression of grain amaranth (Amaranthus hypochondriacus) AhERF or AhDOF transcription factors in Arabidopsis thaliana increases water deficit- and salt-stress tolerance, respectively, via contrasting stress-amelioration mechanisms. PLoS ONE 11:e0164280. doi: 10.1371/journal.pone.0164280
Massange-Sanchez, J. A., Palmeros-Suarez, P. A., Martinez-Gallardo, N. A., Castrillon-Arbelaez, P. A., Avilés-Arnaut, H., Alatorre-Cobos, F., et al. (2015). The novel and taxonomically restricted Ah24 gene from grain amaranth (Amaranthus hypochondriacus) has a dual role in development and defense. Front. Plant Sci. 6:602. doi: 10.3389/fpls.2015.00602
Mauch, F., Mauch-Mani, B., Gaille, C., Kull, B., Haas, D., and Reimmann, C. (2001). Manipulation of salicylate content in Arabidopsis thaliana by the expression of an engineered bacterial salicylate synthase. Plant J. 25, 67–77. doi: 10.1111/j.1365-313X.2001.00940.x
Miller, T. E., Wing, J. S., and Huete, A. R. (1984). The agricultural potential of selected C4 plants in arid environments. J. Arid Environ. 7, 275–286.
Mirelman, D. E., Galun, E., Sharon, N., and Lotan, R. (1975). Inhibition of fungal growth by wheat germ agglutinin. Nature 256, 414–416. doi: 10.1038/256414a0
Mishkind, M., Raikhel, N. V., Palevitz, B. A., and Keegstra, K. (1982). Immunocytochemical localization of wheat germ agglutinin in wheat. J. Cell Biol. 92, 753–764. doi: 10.1083/jcb.92.3.753
Montoya-Rodríguez, A., Gómez-Favela, M. A., Reyes-Moreno, C., Milán-Carrillo, J., and González de Mejía, E. (2015). Identification of bioactive peptide sequences from amaranth (Amaranthus hypochondriacus) seed proteins and their potential role in the prevention of chronic diseases. Compr. Rev. Food Sci. Food Saf. 14, 139–158. doi: 10.1111/1541-4337.12125
Moradi, A., El-Shetehy, M., Gamir, J., Austerlitz, T., Dahlin, P., Wieczorek, K., et al. (2021). Expression of a fungal lectin in Arabidopsis enhances plant growth and resistance toward microbial pathogens and a plant-parasitic nematode. Front. Plant Sci. 12:657451. doi: 10.3389/fpls.2021.657451
Murashige, T., and Skoog, F. (1962). A revised medium for rapid growth and bioassays with tobacco tissue cultures. Physiol. Plant. 15, 473–497. doi: 10.1111/j.1399-3054.1962.tb08052.x
Nakamura, S., Ikegami, A., Mizuno, M., Yagi, F., and Nomura, K. (2004). The expression profile of lectin differs from that of seed storage proteins in Castanea crenata trees. Biosci. Biotechnol. Biochem. 68, 1698–1705. doi: 10.1271/bbb.68.1698
Nonomura, A. M., Shevela, D., Komath, S. S., Biel, K. V., and Govindjee, G. (2020). The carbon reactions of photosynthesis: role of lectins and glycoregulation. Photosynthetica 58, 1090–1097. doi: 10.32615/ps.2020.064
Omamt, E. N., Hammes, P. S., and Robbertse, P. J. (2006). Differences in salinity tolerance for growth and water-use efficiency in some amaranth (Amaranthus spp.) genotypes. N. Z. J. Crop Hort. Sci. 34, 11–22. doi: 10.1080/01140671.2006.9514382
Palmeros-Suárez, P. A., Massange-Sánchez, J. A., Martínez-Gallardo, N. A., Montero-Vargas, J. M., Gómez-Leyva, J. F., and Délano-Frier, J. P. (2015). The overexpression of an Amaranthus hypochondriacus NF-YC gene modifies growth and confers water deficit stress resistance in Arabidopsis. Plant Sci. 240, 25–40. doi: 10.1016/j.plantsci.2015.08.010
Palmeros-Suárez, P. A., Massange-Sánchez, J. A., Sánchez-Segura, L., Martínez-Gallardo, N. A., Espitia Rangel, E., Gómez-Leyva, J. F., et al. (2017). AhDGR2, an amaranth abiotic stress-induced DUF642 protein gene, modifies cell wall structure and composition and causes salt and ABA hyper-sensibility in transgenic Arabidopsis. Planta 245, 623–640. doi: 10.1007/s00425-016-2635-y
Peng, X., Wang, M., Li, Y., Yan, W., Chang, Z., Chen, Z., et al. (2020). Lectin receptor kinase OsLecRK-S.7 is required for pollen development and male fertility. J. Integr. Plant Biol.62, 1227–1245. doi: 10.1111/jipb.12897
Petrova, N., Nazipova, A., Gorshkov, O., Mokshina, N., Patova, O., and Gorshkova, T. (2021). Gene expression patterns for proteins with lectin domains in flax stem tissues are related to deposition of distinct cell wall types. Front. Plant Sci. 12:634594. doi: 10.3389/fpls.2021.634594
Písaríkov,á, B., Kráčmar, S., and Herzig, I. (2005). Amino acid contents and biological value of protein in various amaranth species. Czech J. Anim. Sci. 50, 169–174. doi: 10.17221/4011-CJAS
Puthoff, D. P., Sardesai, N., Subramanyam, S., Nemacheck, J. A., and Williams, C. E. (2005). Hfr-2, a wheat cytolytic toxin-like gene, is up-regulated by virulent Hessian fly larval feeding. Mol. Plant Pathol. 6, 411–423. doi: 10.1111/j.1364-3703.2005.00289.x
Quiapim, A. C., Brito, M. S., Bernardes, L. A., Dasilva, I., Malavazi, I., DePaoli, H. C., et al. (2009). Analysis of the Nicotiana tabacum stigma/style transcriptome reveals gene expression differences between wet and dry stigma species. Plant Physiol. 149, 1211–1230. doi: 10.1104/pp.108.131573
Rajan, B., Patel, D. M., Kitani, Y., Viswanath, K., and Brinchmann, M. F. (2017). Novel mannose binding natterin-like protein in the skin mucus of Atlantic cod (Gadus morhua). Fish Shellfish Immunol. 68, 452–457. doi: 10.1016/j.fsi.2017.07.039
Rastogi, A., and Shukla, S. (2013). Amaranth: a new millennium crop of nutraceutical values. Crit. Rev. Food Sci. Nutr. 53, 109–125. doi: 10.1080/10408398.2010.517876
Rivero, L., Scholl, R., Holomuzki, N., Crist, D., Grotewold, E., and Brkljacic, J. (2014). “Handling Arabidopsis plants: Growth, preservation of seeds, transformation, and genetic crosses” in Arabidopsis Protocols. Methods in Molecular Biology (Methods and Protocols), eds J. Sanchez-Serrano and J. Salinas (Totowa, NJ: Humana Press),1062, 3–25. doi: 10.1007/978-1-62703-580-4_1
Rosales, M. A., Maurel, C., and Nacry, P. (2019). Abscisic acid coordinates dose-dependent developmental and hydraulic responses of roots to water deficit. Plant Physiol. 180, 2198–2211. doi: 10.1104/pp.18.01546
Roy, A., Kucukural, A., and Zhang, Y. (2010). I-TASSER: a unified platform for automated protein structure and function prediction. Nat. Protoc. 5, 725–738. doi: 10.1038/nprot.2010.5
Sakanaka, S., Tachibana, Y., and Okada, Y. (2005). Preparation and antioxidant properties of extracts of Japanese persimmon leaf tea (kakinoha-cha). Food Chem. 89, 569–575. doi: 10.1016/j.foodchem.2004.03.013
Sánchez-Hernandez, C., Martinez-Gallardo, N., Guerrero-Rangel, A., Valdes-Rodriguez, S., and Delano-Frier, J. (2004). Trypsin and alpha-amylase inhibitors are differentially induced in leaves of amaranth (Amaranthus hypochondriacus) in response to biotic and abiotic stress. Physiol. Plant. 122, 254–264. doi: 10.1111/j.0031-9317.2004.00398.x
Santos Sousa, A., Lima Rego, E., and Ribeiro dos Santos, F. (2013). Viability and Action of CPL Lectin on in vitro germinability of pollen grains of Malpighia emarginata DC.-(Malpighiaceae). Am. J. Plant Sci. 4, 53–58. doi: 10.4236/ajps.2013.47A1007
Sarker, U., and Oba, S. (2019). Nutraceuticals, antioxidant pigments, and phytochemicals in the leaves of Amaranthus spinosus and Amaranthus viridis weedy species. Sci. Rep. 9:20413. doi: 10.1038/s41598-019-50977-5
Shang, C., Dang, L., and Van Damme, E. J. M. (2017). “Plant AB Toxins with Lectin Domains” in Plant Toxins. Toxinology. eds. P. Gopalakrishnakone, C. Carlini, and R. Ligabue-Braun (Dordrecht: Springer) 183–198. doi: 10.1007/978-94-007-6464-4_4
Shao, R., Xin, L., Mao, J., Li, L., Kang, G., and Yang, Q. (2015). Physiological, ultrastructural and proteomic responses in the leaf of maize seedlings to polyethylene glycol-stimulated severe water deficiency. Int. J. Mol. Sci. 16, 21606–21625. doi: 10.3390/ijms160921606
Shukla, S., Bhargava, A., Chatterjee, A., Pandey, A. C., and Mishra, B. K. (2010). Diversity in phenotypic and nutritional traits in vegetable amaranth (Amaranthus tricolor), a nutritionally under-utilised crop. J. Sci. Food Agric. 90, 139–144. doi: 10.1002/jsfa.3797
Shukla, S., Pandey, V., Pachauri, G., Dixit, B. S., Banerji, R., and Singh, S. P. (2003). Nutritional contents of different foliage cuttings of vegetable amaranth. Plant Foods Hum. Nutr. 58, 1–8. doi: 10.1023/B:QUAL.0000040338.33755.b5
Shure, M., Wessler, S., and Fedoroff, N. (1983). Molecular identification and isolation of the Waxy locus in maize. Cell 35, 225–233. doi: 10.1016/0092-8674(83)90225-8
Singh, P., and Zimmerli, L. (2013). Lectin receptor kinases in plant innate immunity. Front. Plant Sci. 4:124. doi: 10.3389/fpls.2013.00124
Stetter, M., Vidal-Villarejo, M., and Schmid, K. J. (2020). Parallel seed color adaptation during multiple domestication attempts of an ancient new world grain. Mol. Biol. Evol. 37, 1407–1419. doi: 10.1093/molbev/msz304
Stetter, M. G., Müller, T., and Schmid, K. J. (2017). Genomic and phenotypic evidence for an incomplete domestication of South American grain amaranth (Amaranthus caudatus). Mol. Ecol. 26, 871–886. doi: 10.1111/mec.13974
Stetter, M. G., and Schmid, K. J. (2017). Analysis of phylogenetic relationships and genome size evolution of the Amaranthus genus using GBS indicates the ancestors of an ancient crop. Mol. Phylogenet. Evol. 109, 80–92. doi: 10.1016/j.ympev.2016.12.029
Szczesny, P., Iacovache, I., Muszewska, A., Ginalski, K., van der Goot, F. G., and Grynberg, M. (2011). Extending the aerolysin family: from bacteria to vertebrates. PLoS ONE 6:e20349. doi: 10.1371/journal.pone.0020349
Tamura, S., Yamakawa, M., and Shiomi, K. (2011). Purification, characterization and cDNA cloning of two natterin-like toxins from the skin secretion of oriental catfish Plotosus lineatus. Toxicon 58, 430–438. doi: 10.1016/j.toxicon.2011.08.001
Teng, X. L., Chen, N., and Xiao, X. G. (2015). Identification of a catalase-phenol oxidase in betalain biosynthesis in red Amaranth (Amaranthus cruentus). Front. Plant Sci. 6:1228. doi: 10.3389/fpls.2015.01228
Thornton, B., and Basu, C. (2011). Real-time PCR (qPCR) primer design using free online software. Biochem. Mol. Biol. Educ. 39, 145–154. doi: 10.1002/bmb.20461
Trucco, F., and Tranel, P. J. (2011). “Amaranthus” in Wild Crop Relatives: Genomic and Breeding Resources, ed. C. Kole (Berlin: Springer), 11–21. doi: 10.1007/978-3-642-20450-0_2
Tsaneva, M., and Van Damme, E. J. M. (2020). 130 years of plant Lectin Research. Glycoconj. J. 37, 533–551. doi: 10.1007/s10719-020-09942-y
Unno, H., Matsuyama, K., Tsuji, Y., Goda, S., Hiemori, K., Tateno, H., et al. (2016). Identification, characterization, and X-ray crystallographic analysis of a novel type of mannose-specific lectin CGL1 from the pacific oyster Crassostrea gigas. Sci. Rep. 5:29135. doi: 10.1038/srep29135
Vakhania, M. A., Alexidze, G. I., and Aleksidze, N. G. (2014). Allocation of lectins in flower organs of aloe plant (Aloe aristata) and their biochemical characteristics. Ann. Agrar. Sci. 12, 22–26.
Van Damme, E. J. M., Barre, A., Rougé, P., and Peumans, W. J. (2004). Cytoplasmic/nuclear plant lectins: a new story. Trends Plant Sci. 9, 484–489. doi: 10.1016/j.tplants.2004.08.003
Van Holle, S., Smagghe, G., and Van Damme, E. J. M. (2016). Overexpression of Nictaba-like lectin genes from Glycine max confers tolerance toward Pseudomonas syringae infection, aphid infestation and salt stress in transgenic Arabidopsis plants. Front. Plant Sci. 7:1590. doi: 10.3389/fpls.2016.01590
Van Hove, J., De Jaeger, G., De Winne, N., Guisez, Y., and Van Damme, E. J. M. (2015). The Arabidopsis lectin EULS3 is involved in stomatal closure. Plant Sci. 238, 312–322. doi: 10.1016/j.plantsci.2015.07.005
Vandenborre, G., Smagghe, G., and Van Damme, E. J. M. (2011). Plant lectins as defense proteins against phytophagous insects. Phytochemistry 72, 1538–1550. doi: 10.1016/j.phytochem.2011.02.024
Vargas-Ortiz, E., Délano-Frier, J. P., and Tiessen, A. (2015). The tolerance of grain amaranth (Amaranthus cruentus L.) to defoliation during vegetative growth is compromised during flowering. J. Plant Physiol. Biochem. 91, 36–40. doi: 10.1016/j.plaphy.2015.03.007
Vargas-Ortiz, E., Espitia-Rangel, E., Tiessen, A., and Délano-Frier, J. P. (2013). Grain amaranths are defoliation-tolerant crop species capable of utilizing stem and root carbohydrate reserves to sustain vegetative and reproductive growth after leaf loss. PLoS ONE 8:e67879. doi: 10.1371/journal.pone.0067879
Venskutonis, P. R., and Kraujalis, P. (2013). Nutritional components of amaranth seeds and vegetables: a review on composition, properties, and uses. Compr. Rev. Food Sci. Food Saf. 12, 381–412. doi: 10.1111/1541-4337.12021
Wang, C., Zhou, M., Zhang, X., Yao, J., Zhang, Y., and Mou, Z. (2017). A lectin receptor kinase as a potential sensor for extracellular nicotinamide adenine dinucleotide in Arabidopsis thaliana. eLife 6:e25474. doi: 10.7554/eLife.25474.031
Wang, Y., and Bouwmeester, K. (2017). L-type lectin receptor kinases: new forces in plant immunity. PLoS Pathog. 13:e1006433. doi: 10.1371/journal.ppat.1006433
Ward, S. M., Webster, T. M., and Steckel, L. E. (2013). Palmer amaranth (Amaranthus palmeri): a review. Weed Technol. 27, 12–27. doi: 10.1614/WT-D-12-00113.1
Wu, F., Feng, B., Ren, Y., Wu, D., Chen, Y., Huang, S., et al. (2017). A pore-forming protein implements VLR-activated complement cytotoxicity in lamprey. Cell Discov. 19:17033. doi: 10.1038/celldisc.2017.33
Wu, H., Zou, J., May, B., Gu, Q., and Cheung, A. Y. (1993). A tobacco gene family for flower cell wall proteins with a proline-rich domain and a cysteine-rich domain. Proc. Natl. Acad. Sci. U.S.A. 90, 6829–6833. doi: 10.1073/pnas.90.14.6829
Wu, J., Luo, X., Guo, H., Xiao, J., and Tian, Y. (2006). Transgenic cotton, expressing Amaranthus caudatus agglutinin, confers enhanced resistance to aphids. Plant Breed. 125, 390–394. doi: 10.1111/j.1439-0523.2006.01247.x
Wu, X., and Blair, M. W. (2017). Diversity in grain amaranths and relatives distinguished by genotyping by sequencing (GBS). Front. Plant Sci. 8:1960. doi: 10.3389/fpls.2017.01960
Xue, Z., Liu, X., Pang, Y., Yu, T., Xiao, R., Jin, M., et al. (2012). Characterization, phylogenetic analysis and cDNA cloning of natterin-like gene from the blood of lamprey, Lampetra japonica. Immunol. Lett. 148, 1–10. doi: 10.1016/j.imlet.2012.08.005
Yang, X., Zhang, X. R., Zhang, M. J., Guo, W. C., Tian, W. C., Tian, Y. C., et al. (2011). Transgenic potato overexpressing the Amaranthus caudatus agglutinin gene to confer aphid resistance. Crop Sci. 51, 2119–2124. doi: 10.2135/cropsci2010.11.0650
Yao, J., Withers, J., and He, S. Y. (2013). “Pseudomonas syringae infection assays in Arabidopsis” in Jasmonate Signaling. Methods in Molecular Biology (Methods and Protocols), Vol 1011, eds A. Goossens and L. Pauwels (Totowa, NJ: Humana Press), 63–81. doi: 10.1007/978-1-62703-414-2_6
Keywords: aerolysin, agglutinin, biotic stress, development, flowering, grain amaranth, Natterin-like
Citation: Cabrales-Orona G, Martínez-Gallardo N and Délano-Frier JP (2022) Functional Characterization of an Amaranth Natterin-4-Like-1 Gene in Arabidopsis thaliana. Front. Sustain. Food Syst. 5:814188. doi: 10.3389/fsufs.2021.814188
Received: 12 November 2021; Accepted: 06 December 2021;
Published: 10 January 2022.
Edited by:
Autar Krishen Mattoo, Agricultural Research Service (USDA), United StatesReviewed by:
Rakesh K. Upadhyay, United States Department of Agriculture (USDA), United StatesGeorgios Merkouropoulos, Hellenic Agricultural Organisation DIMITRA, Greece
Copyright © 2022 Cabrales-Orona, Martínez-Gallardo and Délano-Frier. This is an open-access article distributed under the terms of the Creative Commons Attribution License (CC BY). The use, distribution or reproduction in other forums is permitted, provided the original author(s) and the copyright owner(s) are credited and that the original publication in this journal is cited, in accordance with accepted academic practice. No use, distribution or reproduction is permitted which does not comply with these terms.
*Correspondence: John Paul Délano-Frier, am9obi5kZWxhbm8mI3gwMDA0MDtjaW52ZXN0YXYubXg=