- 1School of Science, School of Humanities, Arts, and Social Sciences (SHASS), Massachusetts Institute of Technology, Cambridge, MA, United States
- 2New Harvest, Cambridge, MA, United States
Cellular agriculture, the manufacturing of animal-sourced foods by cell cultures, may promote food security by providing a food source that is available, accessible, utilized, and stable. The extent to which cellular agriculture can promote food security, however, will depend in part on the supply system by which it produces food. Many cellular agriculture companies appear poised to follow a centralized supply system, in which production is concentrated within a small number of large plants and products are distributed over a wide area. This model benefits from economies of scale, but has several weaknesses to food security. By being built of a handful of plants with products distributed by a large transportation network, the centralized model is vulnerable to closures, as became clear for animal-sourced centralized system during the COVID-19 pandemic. Cellular agriculture systems are being built now; therefore, alternative supply system models of decentralized and distributed systems should be considered as the systems of cellular agriculture production are established. This paper defines both the requirements of food security and three possible supply system models that cellular agriculture could take and evaluates each model based on the requirements of food security.
Introduction
The Food Security Challenges Addressed by Cellular Agriculture
Cellular agriculture, the manufacturing of animal-sourced foods by cell culture, has garnered increased interest in the past decade as an alternative to the animal-sourced meat, seafood, dairy, and egg industries. The field can be divided into two subfields: cultured meat, cellular products made of cultured animal cells, and precision fermentation products, acellular products such as milk protein isolated from microbial cultures engineered specifically to produce these products (Mendly-Zambo et al., 2021). Most support for cellular agriculture has come from the potential benefits that culturing animal products could have to animal welfare, sustainability, and reduced need for antibiotics; however, as a new food source, widely adopted cellular agriculture may also improve global food security (Painter et al., 2020).
Global food security requires that food to be accessible, available, utilized, and stable for all people. These requirements may be promoted by cellular agriculture with proper consideration during the establishment of the field.
First, production by cellular agriculture circumvents some of the most dangerous pathways for disease in human history. COVID-19 is just the latest zoonotic disease pandemic, including the 1918 influenza and H1N1 swine flu, to originate from hunted or farmed animals (Graham et al., 2008; Tomley and Shirley, 2009; Worobey et al., 2014). These animals also generate a large amount of biowaste (manure, blood, pus, mucus) on which pathogens such as E. coli, salmonella, and viruses can survive for months and contaminate water sources and meat (Gerba and Smith, 2005; Sapkota et al., 2007; BC Cook Articulation Committee, 2015). In contrast, cellular agriculture produces isolated products from cultures within a bioreactor, such that there would be minimum biowaste and any infection would be quickly identified and discarded by producers (Stacey, 2011).
Second, cellular agriculture could be more resilient to supply chain disruptions than animal-sourced industries, providing food without requiring the timely transport of animals. A hog needs to grow 5–10 months before it reaches peak weight and composition (Ziegler, 1991), and because animals have strict timelines where they must be shipped to slaughterhouses while they are the ideal size, disruptions in the supply chain cause them to quickly lose viability and become a burden to farmers (Ijaz et al., 2021; U.S. Department of Agriculture, 2021). In comparison, cellular agriculture bioreactor designs predict that batches will reach their final growth (cell density) in a matter of weeks, allowing quick adaptation to changes in demand or disruptions in the supply system (Allan et al., 2019). Long-lasting inputs like dried media and cryopreservation of cell stocks could be stored as stable backup.
Third, cellular agriculture could provide culturally important and nutritious animal products. Many culturally relevant and nutritious meats are expensive because the demand exceeds the available supply, such as octopus or salmon, or their production requires additional processing, such as skinless chicken. A 2013 Harvard study of 10 high-income countries found that the healthiest meats/proteins are about $0.29 more expensive per day per 2,000 kcal diet than the least healthy proteins (Rao et al., 2013). In contrast, co-culturing of fat and muscle cells has been difficult for cellular agriculture, suggesting that lean meats may be cheaper and more readily available than fatty cuts (Post et al., 2020). Some cultured meats could even have enhanced nutrition compared to their animal-sourced counterparts (Simsa et al., 2019). Cultured meat can also raise the supply of culturally relevant but expensive foods, as could be the case for a cultured meat company's recent partnership with a Wagyu beef producer (Just Inc., 2018). In sum, cellular agriculture could make food more resilient and safer, and make culturally relevant food more accessible.
In other regards, however, the degree to which cellular agriculture can promote food security will be determined in part by which of the three supply systems that cell ag companies may adapt: centralized, decentralized, or distributed.
The Benefits of Cellular Agriculture to Food Security Will Depend on the Supply System
As cell ag moves to realization, the desire to reduce costs by scaling up may inevitably lead to centralized production. The large amount of venture capital investments in cellular agriculture will especially encourage companies to maximize profits by scaling up, potentially at the cost of ignoring other values including food security (Mouat and Prince, 2018; Van Eenennaam, 2019). Centralized meat supply systems have been revealed in recent memory to be both susceptible to and exacerbate public health crises related to food.
The COVID-19 pandemic highlighted the vulnerability of centralized food production. In March 2020, the WHO formally declared COVID-19 a pandemic, and centralized meat production was hit hard by outbreaks in processing plants. Meat production had centralized in recent decades such that 36 plants processed more than 88% of all cattle slaughtered in the US (MacDonald et al., 2000; Lombardo, 2020; National Agricultural Statistics Service, 2020). During the pandemic, meat processing plants quickly became major hotspots for COVID-19, forcing 57 plants to shut down by 3 months into the pandemic (McCarthy and Danley, 2020). The USDA estimated that, as COVID-19 caused plants to reduce operations or shut down, total beef production in April 2020 was 20% lower, and both total pork and total turkey production were 10% lower than in April 2019 (Johansson, 2020). With processing plants closed, farmers were forced to depopulate their herds while consumers faced shortages and price increases. The U.S. Consumer Price Index for food rose sharply by 2.56% in June 2020 compared to March 2020, led by a sharp 9.28% rise in the Consumer Price Index for meats, poultry, fish, and eggs over the same period (Johansson, 2020; U.S. Department of Labor and Bureau of Labor Statistics, 2020). During the early months of the COVID-19 pandemic, centralized meat production struggled to keep food physically and economically accessible. As cellular agriculture systems become established and seek to promote food security, it will be important to consider whether they should use similarly centralized production.
The purpose of this review is not to be prescriptive of one system model over others. There are advantages and disadvantages of each model as they relate specifically to food security. But with the recent memory of centralized system disruptions and the promise of cellular agriculture to promote food security, it is important to be aware of the possible supply system models that cellular agriculture may take. Before discussing the possible options, however, we must define the requirements of food security.
Food Security
Defining Requirements of Food Security
In 2012, the FAO's Committee on World Food Security defined the concept of food security (Food and Agricultural Organization, 2012) as the following:
Food security exists when all people, at all times, have physical, social and economic access to sufficient, safe and nutritious food that meets their dietary needs and food preferences for an active and healthy life.
This definition is broken into four pillars, as shown in Figure 1, to evaluate how cellular agriculture systems could promote food security: availability, access, utilization, and stability. Availability requires that there is a national supply of food of sufficient quantity and quality. It is determined by domestic food production, food stocks, net trade and food aid. Access requires that individuals have adequate physical and economic access to food, as an adequate national supply of food does not guarantee that individuals are able to acquire food. It is determined by incomes, food prices, and transport infrastructure that can reach all individuals. Utilization requires that individuals are able to acquire adequate nutrition from available and accessible food. It is determined by consumer acceptance and proper use of food, food safety, and food nutrient composition. Stability requires that the three preceding pillars are continuously maintained. It is determined by stable political, economic, and climate conditions, as well as resiliency of food supply and access during crisis. For food security to be achieved, all four pillars must be fulfilled (Food and Agricultural Organization, EC - FAO Food Security Programme, 2008; Charlton, 2016).
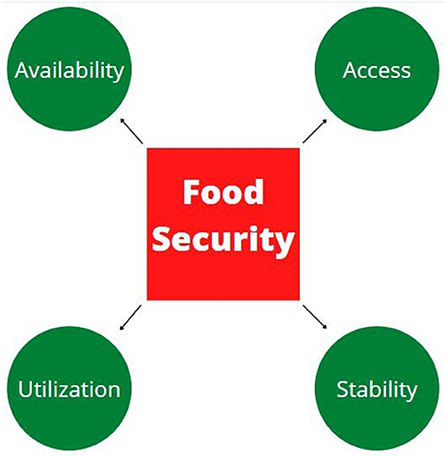
Figure 1. Food security requires that food be safe, sustainably and equitably accessible, and utilized by all people to meet their dietary needs and food preferences. In regards to cellular agriculture, these requirements can be broken into the elements used in section Supply System Models: Centralized, Decentralized, and Distributed to evaluate the potential supply systems of cellular agriculture.
Defining Food Security Challenges for Cellular Agriculture Regardless of Supply System
Though cellular agriculture addresses some of the food security challenges of conventional meat production (as discussed in section The Food Security Challenges Addressed by Cellular Agriculture), it comes with new challenges as well. The new questions that cellular agriculture poses in each pillar of food security are discussed below.
Availability
Cellular agriculture does not yet produce a significant amount of food, and there remains uncertainty on what amount of food could be possible to produce. Recent techno-economic analyses of cultured meat production have estimated different potential costs per kilogram ranging by several orders of magnitude (Humbird, 2020; Risner et al., 2021; Vergeer et al., 2021; Zhang and Dullaghan, 2021). Animal cell cultures also have several technical challenges and biological limits that affect the maximum possible cell density and bioreactor size. This may limit the amount of food it is feasible to produce from animal cell cultures, and therefore the extent to which cultured meat may contribute to food supply (Humbird, 2020; Risner et al., 2021; Vergeer et al., 2021). Several microbial cell culture technologies, however, have been previously scaled to extremely large production volumes (e.g., fuel ethanol, baker's yeast, lysine for animal feed, wastewater treatment) (Humbird, 2020). Research on precision fermentation is limited, but if precision fermentation is able to follow these technologies, it could become a significant contribution to food supply.
Access
Cellular agriculture production is currently prohibitively expensive for widespread consumer access. It remains to be seen if prices of cultured meat can become economically comparable to conventional meat; many of the cost estimates for cultured meat would leave these products out of reach for consumers. To make cultured meat economically comparable to conventional meat, it is predicted that there will need to be significant technological improvements including more efficient use of media by cells, cost reductions in media ingredients, reduced capital costs, increased maximum cell density and volume, and reduced production run time (Humbird, 2020; Risner et al., 2021; Vergeer et al., 2021; Zhang and Dullaghan, 2021). Research on the accessibility of precision fermentation products is again limited.
Utilization
Cellular agriculture may not meet the preferences of all consumers. Numerous studies have noted that some consumers are reluctant to eat cellular agriculture products due to perceived unnaturalness that evokes disgust. As a new method of food production reliant on biotechnology, cellular agriculture will have to gain the familiarity and acceptance of consumers (Bryant and Barnett, 2018; Bryant et al., 2019; Siegrist and Hartmann, 2020).
As cellular agriculture is a novel food production method, there are potential biological hazards in adventitious agents and product safety, another aspect of food utilization, that need to be addressed before regulatory approval. The foodborne pathogens introduced by enteric waste during slaughter would not exist in cultured tissue harvested from a lab (Ong et al., 2021). Cellular agriculture may use antibiotics to prevent undesired bacterial or fungal growth, depending on the scale of production, which would require further scrutiny for downstream food and environmental safety. Though it is likely that several of the foodborne pathogens introduced into animal-sourced meat would not exist in cultured meat, pathogens may enter the cultured meat production process through novel routes. In the case that pathogens do manage to contaminate and not kill the culture, they could pose a safety risk requiring new assessment (Ong et al., 2021). Both the initially sourced primary cells and the cells in the final product of cultured meat may undergo PCR, PERT, or immune-based assays in order to test for adventitious agents and STR profiling or COI gene assays to validate the cells' identity (Zoon, 1993; European Medicines Agency, 1998; Food and Drug Administration, 2006; Food Drug Administration, 2010; Komitopoulou, 2011; Gombold et al., 2014; Barone et al., 2020). The target product must also be approved as safe. One review has suggested that, given the great number of cell multiplications taking place in culturing meat, some dysregulation of cell lines is likely to occur as happens in cancer cells, which could have unknown effects on consumer health (Chriki and Hocquette, 2020). In addition, though genome modification is not required for cultured meat production, it could be used to improve process or product characteristics, and resulting novel cell lines will need to be confirmed safe for consumption (European Food Safety Authority, 2008; World Health Organization, 2008).
Contamination of the end product by process residuals such as scaffolding or growth factors may also be a new challenge for cellular agriculture. At early stages in production, cells may be in contact with recombinant proteins or small molecules to assist growth and differentiation. Cultured cells may also produce substances at higher levels than in an intact animal. Companies may remove these inputs from the final product and then use residue testing to identify whether contaminants are left, and allergenicity testing to identify potential new allergens (European Food Safety Authority, 2014; Food Safety Inspection Service, 2014; Mazzucchelli et al., 2018; Ong et al., 2021). Existing safety regulations surrounding the use of culture inputs and genome modification and the presence of allergens will also have to be adapted to the industry to ensure that the food produced by cellular agriculture is safe for consumption.
Stability
The dependence of cellular agriculture on a continuous energy supply could be detrimental to resilient production and sustainability, both important factors in the stability of food security. In regions where electricity is not readily or constantly available, cellular agriculture would not be possible given current cell culture technology, which requires a high level of environmental control. Depending on the duration of a disruption to electricity such as a natural disaster or accident, active cell cultures may be lost along with any inputs that are not shelf-stable. Different life cycle assessments have also found that cultured meat would have a greenhouse gas impact comparable to conventional meat (Tuomisto, 2019). If cultured meat contributes to climate change, the resulting increase of natural disasters, adverse farming conditions, and geopolitical conflict could negatively affect the security of not just cultured meat, but food security as a whole (Food and Agricultural Organization, 2008). Cellular agriculture must therefore adopt solutions for energy efficiency and clean, secure energy sources in order to positively affect food security.
For similar reasons of resilient access and sustainability, the inputs required for cellular agriculture will need to be recyclable or be resiliently sourced. Cellular agriculture production requires input of cell lines, cell media, and scaffolding. For cellular agriculture to ensure food access at all times, these inputs must be kept readily available, affordable, and functional. Several potential solutions will need to be explored further, including shelf-stable inputs, input recycling processes, and open-access databases to support and disseminate the design of locally-crafted inputs.
Supply System Models: Centralized, Decentralized, and Distributed
Several requirements of food security are influenced by the model of system used to supply food. The following supply systems describe how a consumer product is made and delivered, from procurement of raw materials to distribution of the final product to consumers (Council of Supply Chain Management Professionals, 2013). Different system models may better promote food stability compared to others by being more resilient to disruption and contributing less to risks like climate change and biothreats that endanger food access. The system models also differ in how well they may promote food availability and access by making cellular agriculture production and products more or less economically feasible or reliant on large transportation networks. Finally, the model of system will affect the extent of food utilization, especially adoption by consumers.
The three main supply system models may be categorized as centralized, decentralized, and distributed (visualized in Figure 2). Centralized systems can be defined by a small number of large facilities producing all supply; decentralized systems can be defined by a larger number of small facilities producing supply; distributed systems can be defined by each household producing their own supply (Baran, 1964; Council of Supply Chain Management Professionals, 2013). In this section, the three models as applied to cellular agriculture are evaluated based on the pillars of food security, with results summarized in Table 1.
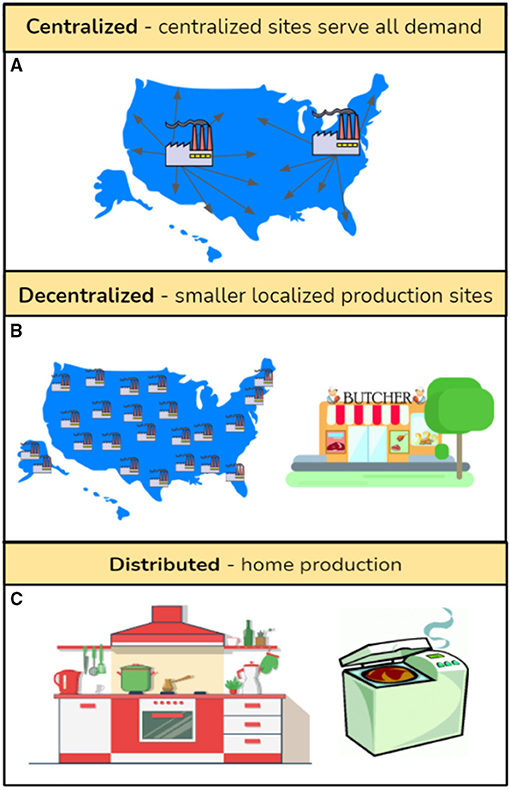
Figure 2. Visual summary of the three supply systems: (A) Centralized, (B) Decentralized, and (C) Distributed Supply Systems.

Table 1. Overview of how potential cellular agriculture systems compare relative to each other on elements of food security.
Centralized Plants
Centralized supply systems are the current norm of many industries and benefit from economies of scale with a dependency on cheap global transportation. The model can be defined as having a small number of very large production plants that satisfy the whole demand in the country and possibly overseas via exports (Baran, 1964; Almena et al., 2019). This introduces a large, complex supply chain.
For cellular agriculture, a centralized supply system would involve a small number of large plants that culture and process food, supplied by a network of input suppliers and distributed to another network of transporters to retailers and restaurants. Each plant would contain industrial bioreactors (2,000 to 10,000 L working volume) where cells proliferate, differentiate, and mature; extraction and harvesting equipment; and lines from which harvested tissue is chopped, dried, flavored, texturized, packaged and labeled as necessary (Food and Drug Administration, 2004).
Availability
Centralized systems in general benefit from the ability to follow cheap labor costs and maximize technological economies of scale. The location of centralized production plants can be selected by the cost of labor and taxes in the region, and how local laws are otherwise conducive to their business (Matt et al., 2015; Almena et al., 2019). Large plants allow companies to buy inputs in bulk, which often have discounts in per unit cost. Additionally, centralized plants can use concentrated investment in specialized machinery that would be too costly for smaller plants (Gervais et al., 2008; Harrison et al., 2018; Almena et al., 2019). The increased efficiency of centralized plants may increase the availability of cellular agriculture products.
Access
The ability of centralized systems to maximize economies of scale and select for favorable production locations could allow them to provide the lowest prices to consumers. However, these benefits to economic access may be counter-acted in part by the need for large transportation chains. Transportation would need to be incorporated into the consumer cost, and poor transportation networks could further increase the cost to the consumer (Matt et al., 2015). The combination of economies of scale and transportation logistics costs will produce the true relative cost of centrally produced products to consumers (Almena et al., 2019).
Utilization
Aseptic technique is an important example of specialized technology that would benefit larger cellular agriculture plants. When cellular agriculture companies boast that they will have decreased dependence on antibiotics compared to conventional meat, they are likely planning to do so by having their plants be kept as aseptic environments (U.S. Department of Agriculture and Food and Drug Administration, 2018). Aseptic environments are maintained by strict practices outlined by the Food and Drug Administration (FDA), and minimize the amount of microbes in the environment in order to prevent contamination of the product and infection of the workers (Food and Drug Administration, 2004). Centralized cellular agriculture systems could best use aseptic techniques because they can concentrate investment in the infrastructure required, from air filtration systems to sterilize-in-place technology, to a single plant.
Concentrating cellular agriculture production in large plants may also facilitate government regulation to ensure cultured product safety and safe waste disposal. The trend of consolidation of conventional meat production has been attributed partially to federal laws that require costly inspections that are more affordable in centralized slaughterhouses compared to smaller plants due to economies of scale (Massie, 2019). Cell and gene therapies (CGT) also require quality control tests that require special technical expertise that may not be available in every locale, as well as specialized tools, such that centralized quality control of CGT is less expensive per dose than completely decentralized QC (Bravery et al., 2013; Harrison et al., 2018). Regulation of cellular agriculture products may similarly be easier in a centralized supply system.
Finally, it could be difficult for a centralized cellular agriculture system to fulfill the dietary needs and food preferences of each region serviced. To serve a number of communities with the same plant, it may be necessary for the system to produce a standardized consumer product. This cannot address food preferences that rely on regional specialties or animals that only reside locally, and may in fact replace local knowledge with a more homogenized global pattern, a process that has been shown in the past to be extremely harmful to health and culture (Raschke and Cheema, 2008; Burnett et al., 2016; Coté, 2016; Weerasekara et al., 2018). It also requires that food travel a long way to reach the consumer's mouth, possibly losing dietary value in the process, as well as lowering consumer acceptance as consumers show preference for fresh and locally grown foods (Rickman et al., 2007; Van der Weele and Driessen, 2013).
Because centralized cellular agriculture systems would likely not serve local specialties, they would not be able to replace the hunting and marketing of wildlife that may be sources of disease or may be facing extinction. These systems are not likely to render obsolete, for example, the Chinese wildlife markets suspected to have birthed SARS-CoV-2 (World Health Organization, 2020a). The biosafety threat imposed by wildlife markets would therefore not be addressed by a centralized model. Though illegal poaching of endangered animals is popularly considered to be caused by elite demand for exotic or rare goods, much illegal hunting is in fact due to the animal product's cultural importance to local communities (Lubilo and Hebinck, 2019). Centralized systems are unlikely to meet the needs of these communities, so loss of biodiversity and illegal hunting would likely continue. By producing a standardized product, these cellular agriculture systems could not address the serious threats involved in current wildlife consumption.
Stability
The supply chain on which centralized cellular agriculture production would rely could introduce multiple vulnerabilities to the food system. During transport and storage, food can be subject to contamination, spoilage, and other loss due to factors such as poor management of temperature and humidity, poor handling, and prolonged storage. According to the Food and Agricultural Organization (FAO), more than 9 percent of all food in North America and Oceania is lost in the steps between production and consumption (Food and Agricultural Organization, 2019). Additionally, disruptions to transportation such as trade restrictions can prevent food from reaching its next destination, which if prolonged can lead to similar situations to that discussed in section Introduction, where farmers are forced to throw away food and depopulate herds, while consumers see shortages in stores. Long and multiple transportation stages are both vulnerable to interruption and endanger the food being transported.
The concentration of cellular agriculture production in a handful of large plants could increase the blow to the food system when one plant needs to shut down for any reason. This could lead to similar crises to those faced by the meat industry during the COVID-19 pandemic, when shutdowns of large plants led to a 20% decrease in the amount of beef processed in the US. In addition to pandemics, other risks such as contamination scares, local power outages, or natural disasters could quickly destroy the linchpin of the supply chain if processing is concentrated to a small number of large plants.
Centralization has both costs and benefits relative to alternatives with regards to environmental sustainability. The long supply chains of centralized systems introduce food waste and greenhouse gases and other pollution emitted by shipping, storage, and the cold chain (James and James, 2010). As centrally produced animal-sourced red meat typically requires 20,400 km of transport, a life-cycle assessment found that its transport produces 10.8 g of CO2eq per kCal, greater than any other food group (Weber and Matthews, 2008). Transportation vehicles also emit smog, soot, nitrogen oxides, sulfur dioxide, and carbon monoxide that contribute to poor air quality dangerous to breathe, and leak fluids that damage water sources and marine environments. Yet studies have also found that centralized production may be more energy efficient than local production for many industries. Economies of scale such as transportation by larger trucks result in lower emissions per unit than when transported in smaller vehicles or consumer cars (Wakeland et al., 2012). The location of production centers can also be chosen by the location's natural comparative advantage in growing food (Schlich and Fleissner, 2005). This may be a factor for the security of cellular agriculture products. A life cycle analysis by Tuomisto and Teixeira de Mattos found that land, water, and energy usage and GHG emissions differed based on whether production was modeled to take place in California, Thailand, or Spain (Tuomisto and Teixeira de Mattos, 2011). Depending on the efficiency gained and the added cost, centralized cellular agriculture systems may or may not be more environmentally friendly than cellular agriculture systems with more localized production.
Decentralized Systems
Decentralized supply systems have gained popularity in recent years as alternatives to centralized models. The model can be defined by smaller production sites geographically dispersed in different markets and countries, with each site serving as a centralized hub for a subsection of participants (Baran, 1964). For cellular agriculture, this would include several different pathways, from geographically dispersed plants under the same company, to craft-cellular-agriculture businesses, to community-owned lab spaces1. Decentralized production would take place under the supervision of trained staff, and the food sent to home consumers, local stores, or eaten on-site after safety inspection. Each site may produce the same standardized good, or have some ability to produce different goods that better address local demand, as shown in Figure 2 (Matt et al., 2015).
One cellular agriculture company, Future Meat Technologies (FMT), has already announced its intentions to pursue a decentralized model. Unlike other cellular agriculture companies, FMT plans to market its animal cell lines, media, and cell-culturing equipment to existing meat companies, farmers, retailers, and restaurants rather than sell under its own branding alone (Future Meat, 2020). Their decision to pursue a decentralized model and their supporting technology for this model have attracted $16.6 million dollars of Series A investment, as investors see in FMT the promise to bring cultured meat to an affordable price without a huge amount of new capital to build a new centralized plant (Future Meat, 2020). FMT has patented a small-scale perfusion bioreactor designed to recycle media. The perfusion model requires cheaper media and maximizes cell concentrations compared to batch or fed-batch bioreactors that would otherwise be used in small scales of production, and the small scale abolishes the need for a seed train (Nahmias, 2018). The patented design aims to reduce the inputs, costs, and complexities of small-scale production, therefore potentially making the small scale production of a decentralized model more resilient and affordable than other small-scale production methods of cellular agriculture.
In another form of decentralized system, the community biology labs Counter Culture Labs and Biocurious has been researching the production of cheese proteins since 2014. Community labs such as these offer shared space, equipment, funding, and mentorship, and host a variety of public events and workshops for biology projects. Counter Culture Labs and Biocurious launched the Real Vegan Cheese project in 2014 as a community project (Wilbanks, 2017; Real Vegan Cheese, 2018). After funding from both a crowdfunding campaign and a private foundation, the project has produced all four cheese proteins and purified three of them (Real Vegan Cheese, 2018). They plan to send DNA kits with the genes of cheese production to the backers of their crowdfunding campaign, and to continue to publish all information on their process as open-source in the public domain (Real Vegan Cheese, 2018).
Availability
Decentralized cellular agriculture systems would require innovation to keep production inputs readily stocked for each facility in order to ensure the availability of cultured food. Media recycling, renewable local energy sources, energy-efficient equipment, immortal cell lines, and local derivation of antimicrobial agents and other inputs are existing inventions that could be further developed in order to reach self-sufficiency in cellular agriculture (Maqsood et al., 2013; Goldthau, 2014; Nahmias, 2018). Otherwise, even with a short post-production supply chain, inputs would have to be acquired by an extensive pre-production supply chain that could endanger the availability of cellular agriculture products.
Access
Decentralized cellular agriculture production could be more or less economically accessible than centralized cellular agriculture production. Decentralization would lower the needed transportation infrastructure of cellular agriculture. Even with reduced transportation, however, the lost economies of scale of smaller plants could raise the price of products, making them less accessible to lower-income households (Almena et al., 2019). For conventional meat, the cost of inspection and sanitation procedures required by law in processing plants has contributed to pushing smaller firms out of business, contributing to that industry's centralization (Taylor, 2008). The reduced ability to select locations may also increase the cost of decentralized systems. Whereas, centralized cellular agriculture plants can be built where there are favorable conditions for production, such as favorable economic policies, easy access to inputs, and lower labor costs, decentralized models would optimize for proximity to the consumer (Veldhuis et al., 2019). Further investigation is required to model these cost tradeoffs in centralized and decentralized cellular agriculture production.
The accessibility of decentralized cellular agriculture production may also differ based on the ownership structure of the system and the need for specialized workers. Decentralized systems of many smaller plants owned by a single company could still invest in specialized equipment up front for more efficient production. With less capital than large corporations, local or community cellular agriculture organizations could face problems gaining and maintaining the necessary equipment and educated staff to be viable operations (MacDonald and Ollinger, 2000; Lopez et al., 2002; Gervais et al., 2008). This could sacrifice the affordability of locally owned cellular agriculture facilities and make them more vulnerable to closure compared to facilities owned by larger companies.
Wider governmental policies could improve the economic access of decentralized cellular agriculture systems compared to larger centralized facilities. In the U.S., the bipartisan PRIME act has been proposed to support local farms and meat processing facilities by relaxing the federal safety regulations that burden small processing facilities (Massie, 2017). Existing national grants and partnerships with local organizations such as hospitals and schools could further fund and support smaller local cellular agriculture plants (Martinez et al., 2010). NGOs and government organizations could also provide training in how to grow and sustain locally owned or communal facilities, and promote collaboration between small producers (Martinez et al., 2010). Applied to cellular agriculture, these policies and others could improve the viability of decentralized models.
Utilization
Decentralization may benefit the utilization of cultured foods. Producing food in decentralized facilities has been recognized by many food industries as a way to meet the increasing desire of consumers for fresher, locally-produced food (Matt et al., 2015). In addition, some models of decentralized cellular agriculture companies could be able to fine-tune products to meet the nutritional and cultural needs of consumers. A hypothetical example of this could be a cultured heritage beef company that develops a generalizable bioprocess with flexibility in the cell type cultured in order to use cells from local heritage breeds or species. This example produces food that local consumers already desire and know how to prepare, with minimal redundancy in R&D costs (Szejda and Parry, 2020). Research has shown that consumer concerns about the novelty of cultured products are eased by the idea that it could be produced locally, as consumers associate local foods with freshness, health, and connection to the food they eat (Van der Weele and Driessen, 2013; Nielsen, 2015; Román et al., 2017). The addition of jobs to the local economy may further goodwill between plants and their customers. By locally producing the foods that consumers in each region desire, decentralized cellular agriculture systems could be more readily utilized compared to centralized models.
Locally owned decentralized systems could go further than utilization by promoting food sovereignty. First stated in the 1996 World Food Summit by Via Campesina, food sovereignty may be defined as “the peoples'… right to define their agricultural and food policy” (Via Campesina., 2003). Food sovereignty has been seen as an important concept because it prioritizes the right of consumers to choose how and what food is produced. In local or community owned cellular agriculture systems, production would be more in tune with local demand and need, as most decisions would be made by local agents. Locally owned decentralized plants could therefore allow consumers to feel more connection to their food's production compared to a decentralized plant owned by a larger company (Windfuhr and Jonsén, 2005).
Stability
Decentralization reduces key fragilities of centralized systems. They use shorter supply chains, so that there are fewer points where supply could be disrupted and greenhouse gas emissions from transport are lowered (Almena et al., 2019). By decreasing the fraction of total demand met by individual plants and the number of workers at each plant, decentralization may reduce contamination or disease spread and the impact of individual plant shutdowns. Locally owned decentralized systems could also allow communities to become more self-sufficient, insulating them from external political or economic pressures that come from reliance on a larger company (Baer-Nawrocka and Sadowski, 2019).
In the event that a decentralized cellular agriculture facility had to temporarily close, the impact on global food security would be small in this model. In order to secure local food security, however, neighboring facilities will need to be able to increase production quickly or temporary facilities will need to be set up depending on access to new equipment or ability to move equipment from the closed facility. Coordination between facilities may be easier in a decentralized system owned by a single company than in systems with many locally owned facilities. Without a larger governing body, locally owned systems would require some other force of cooperation between systems. Therefore, safety plans would need to be developed beforehand to minimize regional disruption to food access and ensure stability.
Decentralized cellular agriculture production could be more or less sustainable than centralized cellular agriculture production for reasons similar to those discussed for economic access in section Access. Though decentralization could have energy savings from reduced transportation, the lost economies of scale of smaller plants could lead to lessened energy efficiency in production. Almena et al. recently found that a centralized supply system of baby food produces the lowest amount of CO2/kg of a food compared to models of decentralization, sharing economies, on-demand economies, and home production, as industrial-scale machinery was more efficient (Almena et al., 2019). The reduced ability to select locations adds to the complexity of whether decentralized cellular agriculture production is more sustainable than centralization. Whereas, centralized cellular agriculture plants can be built where there are favorable conditions for production, such as access to sustainable energy, warmer climates, and easy access to inputs, decentralized models would optimize for proximity to the consumer (Veldhuis et al., 2019). Combined with the footprint of transportation and the reduced economies of scale, it is currently unclear whether decentralized cellular agriculture supply systems would produce fewer GHG emissions in total than would centralized systems (Edwards-Jones, 2010; Avetisyan et al., 2014; Brunori et al., 2016). Further comparative sustainability assessments of cellular agriculture supply system models are required.
Distributed Home Systems
Distributed systems can be defined as systems where every participant can communicate with one another without going through a centralized point (Baran, 1964). In distributed cellular agriculture systems, households could produce their own cultured goods in their home. Individuals may buy their own equipment and supplies, or may rent all or some combination of inputs from sharing economies or cellular agriculture libraries similar to existing Libraries of Things2 for kitchen equipment, maker tools, and seeds (American Library Association, 2014; Landgraf, 2015).
Availability
Further innovation would be required in order to make distributed cellular agriculture an available method of food production. Similar to the readily available and accessible inputs of a decentralized systems, individual households would also need access to such resource in order to be self-sufficient. Methods and technology would also have to be simplified so that a home cook understands how to safely produce cultured products for their family. Though DIY biology has become the interest of a small but dedicated network of biomakers, the large majority of cellular agriculture engineering projects are still undertaken by teams with relevant college degrees and training (Landrain et al., 2013; Seyfried et al., 2014; Vaage, 2017). A Library of Things model could address some knowledge gaps by hosting public seminars and swaps of recipes and cell culture at the library. Open-source databases and forums could also facilitate the spread of information and support for home production. Still, in order for home cellular agriculture production to be an accessible source of food, the information to perform cellular agriculture will need to be open-access. Cellular agriculture's challenges are requiring the investment of hundreds of educated scientists and millions of dollars, many of which is venture capital funding that may make open access difficult. A future where the technology is refined and available enough to be used by someone without a scientific background or even a non-traditional biomaker education will take sustained effort and investment.
Access
If distributed cellular agriculture systems did become available, they may allow cultured products to be accessible to a larger population. Unlike other forms of production, home production does not require labor or transportation costs, making it possibly more economically accessible than models with more economies of scale (Almena et al., 2019). Distributed systems could also support physical access to cultured products, as they do not rely on, or only minimally rely on, transportation to or from centralized hubs. Though these systems will require a large amount of initial investment in order to become feasible or available, once established, they could reduce barriers to access.
Utilization
These systems could have the best ability to provide individuals with food that meets their preferences and nutritional needs, as they empower users to produce their own food in their own space. Households would become the primary decision makers in what they consume, so that they can customize for both cultural relevance and health needs. Producing at home, when the product is wanted, could also guarantee the product's freshness (Van der Weele and Driessen, 2013; Nielsen, 2015; Román et al., 2017). Households would also have an increased connection to the production of the food they eat compared to the case of centralized production; this process increases familiarization with cultured products, which has been shown to increase a novel food's acceptance (Tuorila and Hartmann, 2020). All of these factors could improve the capability of cellular agriculture to contribute to the utilization of relevant food.
Stability
Distributed cellular agriculture models could benefit from resilient access due to the lack of a post-production supply chain. In situations of global emergency such as the COVID-19 pandemic, food systems that don't rely on long transport chains and temporary immigrant labor will be more likely to resist disruptions that impose travel restrictions. With the means of production in the hands of the consumer, the importance of global movement of food is decreased. Consumers could be able to grow food in distributed cellular agriculture systems even when it is not possible for them to purchase food because they are out of work or there are shortages or lockdowns, currently a major driver of food crises worldwide (Food Security Information Network, 2020). The absence of a post-production supply chain could also reduce the environmental impact of cellular agriculture due to decreased need for transport and storage, though there would be a trade-off in economy of scale similar to that mentioned for decentralized systems in section Stability.
Even with public seminars, open-access information, and simplified production methods, distributed cellular agriculture would still pose novel biosafety and biosecurity challenges. Without access to industrial labs, producers would not be able to rely on aseptic facilities to keep their culture sterile and could instead feel pressure to use antibiotics. Widespread and frequent use of antibiotics, however, would inevitably breed antibiotic-resistant strains of pathogens (Davies and Davies, 2010). This could be a risk for producing infectious diseases more resistant to the drugs we have with which to fight them, and therefore more expensive and difficult to treat (World Health Organization, 2020b). Other potential risks could result from accidents, such as chemical spills or equipment malfunctions, that could harm the household, their broader community, or the environment (Presidential Commission for the Study of Bioethical Issues, 2010; Gorman, 2011).
The simplification and spread of cellular agriculture infrastructure could also invite malicious misuse. It has been cautioned that simplified synthetic biology systems could be used to produce illicit drugs; as the semisynthetic antimalarial drug artemisinin has been produced in engineered yeast, it is not unreasonable to predict that current semisynthetic drugs like heroin or cocaine may also be possible (Schmidt, 2008). There is also concern that DIY biomaker systems could facilitate bioterrorism (Vaage, 2017). If cellular agriculture added the incentive of food production to the development of simplified synthetic biology tools, the technology would be made available to more people more quickly. The increased access of these tools to communities worldwide could increase the chances of malicious actors having access to them.
Distributed cellular agriculture would be difficult to regulate, and therefore would require new measures in order to prevent biosafety and biosecurity risks. It would be infeasible to have local governments check every household for the safety of their cellular agriculture set-up. Instead, cellular agriculture could be kept safer by regulations on what inputs are distributed to households and safeguards built-in to those inputs. Discussion of biomarker ethics have already raised numerous suggestions, such as embedding kill switches or suicide genes in order to prevent environmental spread, or making publicly-available cell lines have minimally-viable genomes in order to ensure the cells die if they are manipulated or mutate naturally (Presidential Commission for the Study of Bioethical Issues, 2010; Landrain et al., 2013). Public forums, such as the “Ask A Biosafety Expert” portal on DIYbio website, and instruction guides could also help guide DIY producers to safely perform cellular agriculture (Schmidt, 2008; DIYbio, 2009, 2017). New regulations will be needed for the case of DIY cellular agriculture specifically, where synthetic biology tools are widespread and being used to produce food.
The largest DIY group devoted to cellular agriculture is Shojinmeat. Based in Japan, the group develops protocols and invents equipment, facilitates ethics discussions with Kyoto University, and creates public communications to raise awareness of cellular agriculture (Shojinmeat Project, 2020). They have forums in both Japanese and English to share experiments, research, and questions, which could be used in the future to further develop a support network for DIY home cooks of cultured meat (Van Der Walt, 2019; Shojinmeat Project, 2020). With hundreds of new participants contributing open-access information, this DIY cellular agriculture group shows the model's potential to reach the public.
Conclusions
Each model of cellular agriculture will have relative advantages and disadvantages for food availability, access, utilization, and stability when applied to cellular agriculture. Though the centralized model maximizes economies of scale, the transportation it requires has significant cost in carbon footprint and money and makes food access vulnerable to plant closures or disruptions in the supply chain. Decentralized models, where smaller plants are dispersed throughout the market, would shorten the supply chain and could allow for more regional specialization and flexibility but lack the same efficiency of centralized systems. Distributed systems may provide the best resilient access in the face of disasters, as well as providing the shortest supply and decision chains, but they raise unique issues of availability, sterility and safety.
Cellular agriculture could potentially have the ability to address food security challenges currently faced by animal agriculture. Zoonotic diseases may not be nearly as common with cellular agriculture as they have become with centralized animal agriculture. Automation and fewer processing stages would allow cellular agriculture production to continue even when viral respiratory diseases, like COVID-19, require social distancing measures. Flexibility in product type and production status will likely be easier when working with cells that take days or weeks to grow compared to animals that take months or years. As an added food production method, cellular agriculture would increase the supply of animal-sourced products without expanding animal agriculture.
There are also, however, several challenges in the food security of cellular agriculture. It remains to be seen whether cellular agriculture will be able to produce significant supplies of food at a price accessible to consumers and to what extent consumers will utilize food produced by this new method. Cellular agriculture production raises new food safety concerns about potential adventitious agents, contaminants, and product safety that need to be addressed before regulatory approval. The bioreactors of cellular agriculture will have to use clean and resilient energy sources to minimize cellular agriculture's carbon footprint and vulnerability to disruption of electricity. For similar reasons, the inputs required for cellular agriculture will need to be recyclable or be resiliently sourced.
Further research can address the open questions surrounding the sustainability and safety of different models of cellular agriculture. Though distributed cellular agriculture systems have gripped public attention, they have been largely ignored in academic literature. Open-source designs must be developed that consider the unique challenges of a home environment compared to a lab, and a life cycle analysis could evaluate their sustainability. Furthermore, decreasing reliance on centralized hubs will require experimentation on immortalizing cell lines, recycling media and scaffolding, and use of locally sourced ingredients as inputs should be given precedence. Non-centralized systems may provide a resilient alternative to traditional meat production, but will require further consideration and preparation in order to be available. For cellular agriculture to best promote food security, we invite further research in how its systems can be constructed to provide consumers sustainable access to sufficient, safe, and nutritious food that meets their dietary needs and food preferences.
Author Contributions
Writing, conceptualization, and investigation were done by ES. Supervision and editing were done by JJ. All authors have read and agreed to the published version of the manuscript.
Conflict of Interest
JJ reports that he is a full-time employee of New Harvest, a donor-funded, 501(c)(3) research institute working to maximize the positive impact of cellular agriculture. ES reports that she previously served as a research intern for New Harvest.
Publisher's Note
All claims expressed in this article are solely those of the authors and do not necessarily represent those of their affiliated organizations, or those of the publisher, the editors and the reviewers. Any product that may be evaluated in this article, or claim that may be made by its manufacturer, is not guaranteed or endorsed by the publisher.
Acknowledgments
The authors thank Dr. Marcos Sanchez-Plata for sharing his expertise in food security and safety (as well as Samuel S. Peabody IV for facilitating this interaction) and Michela Caffrey for her help in editing the figures.
Footnotes
1. ^Community labs, biomakerspaces, or biohackerspaces are biology and biological engineering labs that are communal spaces that provide shared instrumentation, reagents, management, and communal projects without the need for a formal background in research. Projects tend to be open-access, though entrepreneurs may use them to incubate start-up ideas. Though a number of professional researchers are part of these communities, lab members also include high school students, artists, programmers, and more (Scheifele and Burkett, 2016; Tachibana, 2019). Biomakers, biohackers, DIY biologists, or citizen scientists are individuals who experiment with biology and biological engineering outside of professional academia or industry, and may work in their own home labs or in communal spaces (Scheifele and Burkett, 2016).
2. ^Libraries of Things are libraries that offer for lending items outside the traditional fare of books and magazines. Offerings span from seeds, to ukeleles, to microscopes. In seed libraries, seeds and information on how to save seeds from gardens are provided, and the library asks that users give back to the seed library some of the seeds saved from their garden the following year (Cornell Cooperative Extension, 2017). A map by the American Libraries magazine illustrates some of the items offered by Libraries of Things (Dankowski and Mead, 2017).
References
Allan, S. J., De Bank, P. A., and Ellis, M. J. (2019). Bioprocess design considerations for cultured meat production with a focus on the expansion bioreactor. Front. Sustain. Food Syst. 3:44. doi: 10.3389/fsufs.2019.00044
Almena, A., Fryer, P. J., Bakalis, S., and Lopez-Quiroga, E. (2019). Centralized and distributed food manufacture: a modeling platform for technological, environmental and economic assessment at different production scales. Sustain. Prod. Consumpt. 19, 181–193. doi: 10.1016/j.spc.2019.03.001
American Library Association (2014). Sharing Economy. Text. Tools, Publications and Resources. Available online at: http://www.ala.org/tools/future/trends/sharingeconomy
Avetisyan, M., Hertel, T., and Sampson, G. (2014). Is local food more environmentally friendly? The GHG emissions impacts of consuming imported versus domestically produced food. Environ. Resourc. Econ. 58, 415–462. doi: 10.1007/s10640-013-9706-3
Baer-Nawrocka, A., and Sadowski, A. (2019). Food security and food self-sufficiency around the world: a typology of countries. PLoS ONE 14:e0213448. doi: 10.1371/journal.pone.0213448
Baran, P. (1964). On distributed communications networks. IEEE Transac. Commun. Syst. 12, 1–9. doi: 10.1109/TCOM.1964.1088883
Barone, P. W., Wiebe, M. E., Leung, J. C., Hussein, I. T. M., Keumurian, F. J., Bouressa, J., et al. (2020). Viral contamination in biologic manufacture and implications for emerging therapies. Nat. Biotechnol. 38, 563–572. doi: 10.1038/s41587-020-0507-2
BC Cook Articulation Committee (2015). “Diseases associated with meat,” in Meat Cutting and Processing for Food Service. BCcampus. Available online at: https://opentextbc.ca/meatcutting/chapter/diseases-associated-with-meat/ (accessed November 11, 2021).
Bravery, C., Carmen, J., Fong, T., Oprea, W., Hoogendoorn, K., Woda, J., et al. (2013). Potency assay development for cellular therapy products: an ISCT* review of the requirements and experiences in the industry. Cytotherapy 15, 9–19. doi: 10.1016/j.jcyt.2012.10.008
Brunori, G., Galli, F., Barjolle, D., and Van Broekhuizen, R. (2016). Are local food chains more sustainable than global food chains? considerations for assessment. Sustainability 8:449. doi: 10.3390/su8050449
Bryant, C., and Barnett, J. (2018). Consumer acceptance of cultured meat: a systematic review. Meat Sci. 143, 8–17. doi: 10.1016/j.meatsci.2018.04.008
Bryant, C., Szejda, K., Parekh, N., Deshpande, V., and Tse, B. (2019). A survey of consumer perceptions of plant-based and clean meat in the USA, India, and China. Front. Sustain. Food Syst. 3:11. doi: 10.3389/fsufs.2019.00011
Burnett, K., Hay, T., and Chambers, L. (2016). Settler colonialism, indigenous peoples and food: federal indian policies and nutrition programs in the Canadian North since 1945. J. Colonial. Colonial Hist. 17. doi: 10.1353/cch.2016.0030
Charlton, K. E. (2016). Food security, food systems and food sovereignty in the 21st century: a new paradigm required to meet Sustainable Development Goals. Nutr. Diet. 73, 3–12. doi: 10.1111/1747-0080.12264
Chriki, S., and Hocquette, J. F. (2020). The myth of cultured meat: a review. Front. Nutr. 7:7. doi: 10.3389/fnut.2020.00007
Cornell Cooperative Extension. (2017). Free Seed Cabinets. Available online at: http://ccetompkins.org/gardening/help-for-gardeners/free-seed-cabinets (accessed November 29, 2020).
Coté, C. (2016). ‘Indigenizing' food sovereignty. revitalizing indigenous food practices and ecological knowledges in Canada and the United States. Humanities 5:57. doi: 10.3390/h5030057
Council of Supply Chain Management Professionals (2013). SCM Definitions and Glossary of Terms. Available online at: https://cscmp.org/CSCMP/Educate/SCM_Definitions_and_Glossary_of_Terms.aspx (accessed November 28, 2020).
Dankowski, T., and Mead, B. (2017). The Library of Things. American Libraries Magazine. Available online at: https://americanlibrariesmagazine.org/2017/06/01/library-of-things/
Davies, J., and Davies, D. (2010). Origins and evolution of antibiotic resistance. Microbiol. Mol. Biol. Rev. MMBR 74, 417–433. doi: 10.1128/MMBR.00016-10
DIYbio (2009). Local. Available online at: https://diybio.org/local/
DIYbio (2017). Ask a Biosafety Expert — Closed, 2017. Available online at: https://diybio.org/ask-biosafety-notice/
Edwards-Jones, G. (2010). Does eating local food reduce the environmental impact of food production and enhance consumer health? Proc. Nutr. Soc. 69, 582–591. doi: 10.1017/S0029665110002004
European Food Safety Authority (2008). Safety and Nutritional Assessment of GM Plants and Derived Food and Feed: The Role of Animal Feeding Trials. Available online at: https://www.efsa.europa.eu/en/efsajournal/pub/1057
European Food Safety Authority (2014). Scientific opinion on the evaluation of allergenic foods and food ingredients for labelling purposes. EFSA J. 12:3894. doi: 10.2903/j.efsa.2014.3894
European Medicines Agency (1998). Q 5 D Quality of Biotechnological Products: Derivation and Characterisation of Cell Substrates Used for Production of Biotechnological/Biological Products. Available online at: https://www.ema.europa.eu/en/ich-q5d-derivation-characterisation-cell-substrates-used-production-biotechnologicalbiological
Food Safety and Inspection Service (2014). Residue Sampling, Testing and Other Verification Procedures Under the National Residue Program for Meat and Poultry Products. 10,800.1 Rev.1. Washington, DC. Available online at: https://www.fsis.usda.gov/policy/fsis-directives/10800.1
Food Security Information Network (2020). 2020 Global Report on Food Crises – Joint Analysis for Better Decisions. Available online at: https://docs.wfp.org/api/documents/WFP-0000114546/download/?_ga=2.154569272.1242223253.1592846239-1129986998.1592846239
Food and Agricultural Organization (2008). Climate Change and Food Security: A Framework Document. Rome. Available online at: http://www.fao.org/3/k2595e/k2595e00.pdf
Food and Agricultural Organization (2012). Global Strategic Framework for Food Security and Nutrition,” 1st Version. Rome. Available online at: http://www.fao.org/3/ME498E/ME498E.pdf
Food and Agricultural Organization (2019). The State of Food and Agriculture 2019: Moving Forward on Food Loss and Waste Reduction. Rome. Available online at: https://www.fao.org/3/ca6030en/ca6030en.pdf
Food and Agricultural Organization EC - FAO Food Security Programme. (2008). An Introduction to the Basic Concepts of Food Security. Food Security Information for Action Practical Guides. Available online at: http://www.fao.org/3/al936e/al936e.pdf
Food and Drug Administration (2004). Guidance for Industry - Sterile Drug Products Produced by Aseptic Processing - Current Good Manufacturing Practice. Rockville, MD.
Food and Drug Administration (2006). Guidance for Industry and FDA Staff - Minimal Manipulation of Structural Tissue - Jurisdictional Update. Rockville, MD.
Food and Drug Administration (2010). Guidance for Industry - Characterization and Qualification of Cell Substrates and Other Biological Materials Used in the Production of Viral Vaccines for Infectious Disease Indications. Rockville, MD.
Future Meat (2020). Homepage. Available online at: https://future-meat.com/ (accessed November 10, 2021).
Gerba, C. P., and Smith, J. E. (2005). Sources of pathogenic microorganisms and their fate during land application of wastes. J. Environ. Qual. 34, 42–48. Available online at: https://slunik.slu.se/kursfiler/TE0014/20061.1920/F13._Sources_of_Pathogenic_Microorganisms_and_Their_Fate_during_Land_Application_of_Wastes._Gerba_(2007).pdf
Gervais, J. P., Bonroy, O., and Couture, S. (2008). A province-level analysis of economies of scale in canadian food processing. Agribusiness 24, 538–556. doi: 10.1002/agr.20178
Goldthau, A. (2014). Rethinking the governance of energy infrastructure: scale, decentralization and polycentrism. Energy Res. Soc. Sci. 1, 134–140. doi: 10.1016/j.erss.2014.02.009
Gombold, J., Karakasidis, S., Niksa, P., Podczasy, J., Neumann, K., Richardson, J., et al. (2014). Systematic evaluation of in vitro and in vivo adventitious virus assays for the detection of viral contamination of cell banks and biological products. Vaccine 32, 2916–2926. doi: 10.1016/j.vaccine.2014.02.021
Gorman, B. J. (2011). Patent Office as Biosecurity Gatekeeper: Fostering Responsible Science and Building Public Trust in DIY Science, 10 J. Marshall Rev. Intell. Prop. L. 423. Review of Intellectual Property Law. Available online at: https://repository.law.uic.edu/ripl/vol10/iss3/1/ (accessed November 11, 2021).
Graham, J. P., Leibler, J. H., Price, L. B., Otte, J. M., Pfeiffer, D. U., Tiensin, T., et al. (2008). The animal-human interface and infectious disease in industrial food animal production: rethinking biosecurity and biocontainment. Public Health Rep. 123, 282–99. doi: 10.1177/003335490812300309
Harrison, R. P., Rafiq, Q. A., and Medcalf, N. (2018). Centralised versus decentralised manufacturing and the delivery of healthcare products: a United Kingdom exemplar. Cytotherapy 20, 873–890. doi: 10.1016/j.jcyt.2018.05.003
Humbird, D. (2020). Scale-Up Economics for Cultured Meat: Techno-Economic Analysis and Due Diligence. Available online at: https://engrxiv.org/795su/ (accessed November 11, 2021).
Ijaz, M., Yar, M. K., Badar, I. H., Ali, S., Islam, M. S., Jaspal, M. H., et al. (2021). Meat production and supply chain under COVID-19 scenario: current trends and future prospects. Front. Vet. Sci. 8:660736. doi: 10.3389/fvets.2021.660736
James, S. J., and James, C. (2010). The food cold-chain and climate change. Food Res. Int. 43, 1944–1956. doi: 10.1016/j.foodres.2010.02.001
Johansson, R. (2020). Another Look at Availability and Prices of Food Amid the COVID-19 Pandemic. Available online at: https://www.usda.gov/media/blog/2020/05/28/another-look-availability-and-prices-food-amid-covid-19-pandemic (accessed November 28, 2020).
Just Inc. (2018). JUST Makes History with Toriyama Wagyu Beef. Available online at: https://medium.com/justegg/a-new-tradition-721e5039de4
Komitopoulou, E. (2011). “16 - Microbiological challenge testing of foods,” in Food and Beverage Stability and Shelf Life, eds D. Kilcast and P. Subramaniam (Sawston: Woodhead Publishing Series in Food Science, Technology and Nutrition. Woodhead Publishing), 507–523.
Landgraf, G. (2015). Not Your Garden-Variety Library. American Libraries Magazine. Available online at: https://americanlibrariesmagazine.org/2015/01/05/not-your-garden-variety-library/ (accessed January 5, 2015).
Landrain, T., Meyer, M., Martin Perez, A., and Sussan, R. (2013). Do-it-yourself biology: challenges and promises for an open science and technology movement. Syst. Synth. Biol. 7, 115–126. doi: 10.1007/s11693-013-9116-4
Lombardo, K. (2020). Smithfield Foods To Close Sioux Falls, SD Plant Indefinitely Amid COVID-19. Smithfield Foods. Available online at: https://www.smithfieldfoods.com/press-room/company-news/smithfield-foods-to-close-sioux-falls-sd-plant-indefinitely-amid-covid-19
Lopez, R. A., Azzeddine Azzam, M., and Lirón-España, C. (2002). Market power and/or efficiency: a structural approach. Rev. Indust. Organ. 20, 115–126. doi: 10.1023/A:1013867114881
Lubilo, R., and Hebinck, P. (2019). ‘Local Hunting' and community-based natural resource management in namibia: contestations and livelihoods. Geoforum 101, 62–75. doi: 10.1016/j.geoforum.2019.02.020
MacDonald, J. M., and Ollinger, M. E. (2000). Scale economies and consolidation in hog slaughter. Am. J. Agric. Econ. 82, 334–346. doi: 10.1111/0002-9092.00029
MacDonald, J. M., Ollinger, M. E., Nelson, K. E., and Handy, C. R. (2000). Consolidation in U.S. Meatpacking. USDA Agricultural Economic Report, No. 785.
Maqsood, M. I., Matin, M. M., Bahrami, A. R., and Ghasroldasht, M. M. (2013). Immortality of cell lines: challenges and advantages of establishment. Cell Biol. Int. 37, 1038–1045. doi: 10.1002/cbin.10137
Martinez, S., Hand, M., Da Pra, M., Pollack, S., Ralston, K., Smith, T., et al. (2010). Local Food Systems: Concepts, Impacts, and Issues. ERR-97 87. USDA, Washington, DC. Available online at: https://www.ers.usda.gov/publications/pub-details/?pubid=46395.
Massie, T. (2017). Text - H.R.2657 - 115th Congress (2017-2018): PRIME Act. Webpage. 2017/2018. Available online at: https://www.congress.gov/bill/115th-congress/house-bill/2657/text (accessed June 26, 2017).
Massie, T. (2019). Representatives Massie and Pingree Introduce Bipartisan PRIME Act to Empower Local Cattle Farmers, Meet Consumer Demand. Available online at: https://massie.house.gov/news/documentsingle.aspx?DocumentID=395237 (accessed November 11, 2021).
Matt, D. T., Rauch, E., and Dallasega, P. (2015). “Trends towards distributed manufacturing systems and modern forms for their design,” in Procedia CIRP, 9th CIRP Conference on Intelligent Computation in Manufacturing Engineering - CIRP ICME'14 (Capri) 33, 185–190.
Mazzucchelli, G., Holzhauser, T., Cirkovic Velickovic, T., Diaz-Perales, A., Molina, E., Roncada, P., et al. (2018). Current (Food) allergenic risk assessment: is it fit for novel foods? Status Quo and identification of gaps. Mol. Nutr. Food Res. 62. doi: 10.1002/mnfr.201700278
McCarthy, R., and Danley, S. (2020). Map: COVID-19 Meat Plant Closures. Meat + Poultry. Available online at: https://www.meatpoultry.com/articles/22993-covid-19-meat-plant-map (accessed November 28, 2020).
Mendly-Zambo, Z., Powell, L. J., and Newman, L. L. (2021). “Dairy 3.0: cellular agriculture and the future of milk. Food Cult. Soc. 24, 675–693. doi: 10.1080/15528014.2021.1888411
Mouat, M. J., and Prince, R. (2018). Cultured meat and cowless milk: on making markets for animal-free food. J. Cult. Econ. 11, 315–329. doi: 10.1080/17530350.2018.1452277
Nahmias, Y. (2018). Systems and Methods for Growing Cells in vitro. World Intellectual Property Organization WO2018011805A9, filed July 11, 2017 and issued May 24, 2018. Available online at: https://patents.google.com/patent/WO2018011805A9/en
National Agricultural Statistics Service (2020). Livestock Slaughter (2019). Summary. 68. Available online at: https://downloads.usda.library.cornell.edu/usda-esmis/files/r207tp32d/34850245n/5712mr72x/lsan0420.pdf
Nielsen (2015). January (2015). Global Health and Wellness Report. Available online at: https://www.nielsen.com/wp-content/uploads/sites/3/2019/04/january-2015-global-health-and-wellness-report.pdf (accessed November 28, 2020).
Ong, K. J., Johnston, J., Datar, I., Sewalt, V., Holmes, D., and Shatkin, J. A. (2021). Comprehensive reviews in food science and food safety. Comprehens. Rev. 20, 5421–5448. doi: 10.1111/1541-4337.12853
Painter, J., Brennen, J. S., and Kristiansen, S. (2020). The coverage of cultured meat in the US and UK traditional media, 2013–2019: drivers, sources, and competing narratives. Clim. Change 162, 2379–2396. doi: 10.1007/s10584-020-02813-3
Post, M. J., Levenberg, S., Kaplan, D. L., Genovese, N., Fu, J., Bryant, C. J., et al. (2020). Scientific, sustainability and regulatory challenges of cultured meat. Nat. Food 1, 403–415. doi: 10.1038/s43016-020-0112-z
Presidential Commission for the Study of Bioethical Issues (2010). New Directions: The Ethics of Synthetic Biology and Emerging Technologies. Washington, DC.
Rao, M., Afshin, A., Singh, G., and Mozaffarian, D. (2013). Do healthier foods and diet patterns cost more than less healthy options? A systematic review and meta-analysis. BMJ Open 3:e004277. doi: 10.1136/bmjopen-2013-004277
Raschke, V., and Cheema, B. (2008). Colonisation, the new world order, and the eradication of traditional food habits in east Africa: historical perspective on the nutrition transition. Public Health Nutr. 11, 662–674. doi: 10.1017/S1368980007001140
Real Vegan Cheese (2018). Project Update: Giving Tuesday Press Release - Nov 2018. Available online at: https://www.realvegancheese.org/ (accessed November 10, 2021).
Rickman, J. C., Barrett, D. M., and Bruhn, C. M. (2007). Nutritional comparison of fresh, frozen and canned fruits and vegetables. part 1. vitamins c and b and phenolic compounds. J. Sci. Food Agricu. 87, 930–944. doi: 10.1002/jsfa.2825
Risner, D., Li, F., Fell, J. S., Pace, S. A., Siegel, J. B., Tagkopoulos, I., et al. (2021). Preliminary techno-economic assessment of animal cell-based meat. Foods 10:3. doi: 10.3390/foods10010003
Román, S., Sánchez-Siles, L. M., and Siegrist, M. (2017). The importance of food naturalness for consumers: results of a systematic review. Trends Food Sci. Technol. 67, 44–57. doi: 10.1016/j.tifs.2017.06.010
Sapkota, A. R., Curriero, F. C., Gibson, K. E., and Schwab, K. J. (2007). Antibiotic-resistant enterococci and fecal indicators in surface water and groundwater impacted by a concentrated swine feeding operation. Environ. Health Perspect. 115, 1040–1045. doi: 10.1289/ehp.9770
Scheifele, L. Z., and Burkett, T. (2016). The first three years of a community lab: lessons learned and ways forward. J. Microbiol. Biol. Educ. 17, 81–85. doi: 10.1128/jmbe.v17i1.1013
Schlich, E., and Fleissner, U. (2005). The ecology of scale: assessment of regional energy turnover and comparison with global food. Int. J. Life Cycle Assess. 10, 219–223. doi: 10.1065/lca2004.09.180.9
Schmidt, M. (2008). Diffusion of synthetic biology: a challenge to biosafety. Syst. Synth. Biol. 2, 1–6. doi: 10.1007/s11693-008-9018-z
Seyfried, G., Pei, L., and Schmidt, M. (2014). European Do-It-Yourself (DIY) biology: beyond the hope, hype and horror. Bioessays 36, 548–551. doi: 10.1002/bies.201300149
Shojinmeat Project (2020). (Blog). Available online at: https://shojinmeat.com/wordpress/en/ (accessed November 29, 2020).
Siegrist, M., and Hartmann, C. (2020). Consumer acceptance of novel food technologies. Nat. Food 1, 343–350. doi: 10.1038/s43016-020-0094-x
Simsa, R., Yuen, J., Stout, A., Rubio, N., Fogelstrand, P., and Kaplan, D. L. (2019). Extracellular heme proteins influence bovine myosatellite cell proliferation and the color of cell-based meat. Foods 8:521. doi: 10.3390/foods8100521
Stacey, G. N. (2011). “Cell culture contamination,” in Cancer Cell Culture: Methods and Protocols, Methods in Molecular Biology, ed A. Ian Cree (Totowa, NJ: Humana Press), 79–91.
Szejda, K., and Parry, J. (2020). Strategies to Accelerate Consumer Adoption of Plant-Based Meat - Recommendations From a Comprehensive Literature Review. Available online at: https://gfi.org/images/uploads/2020/03/FINAL-Consumer-Adoption-Strategic-Recommendations-Report.pdf (accessed November 11, 2021).
Tachibana, C. (2019). Community science: not just a hobby. Science. Available online at: https://www.sciencemag.org/features/2019/08/community-science-not-just-hobby
Taylor, D. A. (2008). Does One Size Fit All? Small Farms and U.S. Meat Regulations. Environ. Health Perspect. 116, A529–A531. doi: 10.1289/ehp.116-a528
Tomley, F. M., and Shirley, M. W. (2009). Livestock infectious diseases and zoonoses. Philos. Transac. R. Soc. B Biol. Sci. 364, 2637–2642. doi: 10.1098/rstb.2009.0133
Tuomisto, H. L., and Teixeira de Mattos, J. M. (2011). Environmental impacts of cultured meat production. Environ. Sci. Technol. 45, 6117–6123. doi: 10.1021/es200130u
Tuorila, H., and Hartmann, C. (2020). Consumer responses to novel and unfamiliar foods. Curr. Opin. Food Sci. Sens. Sci. Consumer Percept. Food Phys. Mater. Sci. 33, 1–8. doi: 10.1016/j.cofs.2019.09.004
U.S. Department of Agriculture (2021). USDA to Provide Pandemic Assistance to Livestock Producers for Animal Losses. Release No. 0155.21. Available online at: https://www.usda.gov/media/press-releases/2021/07/13/usda-provide-pandemic-assistance-livestock-producers-animal-losses
U.S. Department of Agriculture and Food and Drug Administration (2018). USDA and FDA Joint Public Meeting on the Use of Cell Culture Technology to Develop Products Derived from Livestock and Poultry. Available online at: https://www.fsis.usda.gov/news-events/events-meetings/usda-and-fda-joint-public-meeting-use-cell-culture-technology-develop
U.S. Department of Labor and Bureau of Labor Statistics (2020). Consumer Price Index - June 2020. USDL-20-1376. Available online at: https://www.bls.gov/news.release/archives/cpi_07142020.pdf
Van Der Walt, J. (2019). Announcement: English Slack Channel for the Shojinmeat Project. Medium. Available online at: https://medium.com/@jaysonvdw/announcement-english-slack-channel-for-the-shojinmeat-project-a800e3886799 (accessed November 26, 2019).
Van der Weele, C., and Driessen, C. (2013). Emerging profiles for cultured meat; ethics through and as design. Animals 3, 647–662. doi: 10.3390/ani3030647
Van Eenennaam, A. L. (2019). “Alternative meats and alternative statistics: what do the data say?,” Range Beef Cow Symposium XXVI, 36–49. Available online at: https://animalbiotech.ucdavis.edu/sites/g/files/dgvnsk501/files/inline-files/RBCS%202019%20Proceedings%20Van%20Eenennaam.pdf
Veldhuis, A. J., Glover, J., Bradley, D., Behzadian, K., López-Avilés, A., Cottee, J., et al. (2019). Re-distributed manufacturing and the food-water-energy nexus: opportunities and challenges. Prod. Plann. Control 30, 593–609. doi: 10.1080/09537287.2018.1540055
Vergeer, R., Sinke, P., and Odegard, I. (2021). TEA of Cultivated Meat, Future Projections of Different Scenarios. CE Delft. Available online at: https://cedelft.eu/publications/tea-of-cultivated-meat/
Via Campesina. (2003). Food Sovereignty : Via Campesina. Available online at: https://viacampesina.org/en/food-sovereignty/
Wakeland, W., Cholette, S., and Venkat, K. (2012). “Food transportation issues and reducing carbon footprint,” in Green Technologies in Food Production and Processing, Food Engineering Series. eds I. Joyce Boye and Y. Arcand (Boston, MA: Springer US), 211–236.
Weber, C. L., and Matthews, H. S. (2008). Food-miles and the relative climate impacts of food choices in the United States. Environ. Sci. Technol. 42, 3508–3513. doi: 10.1021/es702969f
Weerasekara, P. C., Withanachchi, C. R., Ginigaddara, G. A. S., and Ploeger, A. (2018). Nutrition transition and traditional food cultural changes in Sri Lanka during colonization and post-colonization. Foods 7:111. doi: 10.3390/foods7070111
Wilbanks, R. (2017). Real vegan cheese and the artistic critique of biotechnology. Engaging Sci. Technol. Soc. 3, 180–205. doi: 10.17351/ests2017.53
Windfuhr, M., and Jonsén, J. (2005). Food Sovereignty: Towards Democracy in Localized Food Systems. Rugby: Practical Action Publishing.
World Health Organization (2008). Codex Alimentarius Commission. Annex 2: Food Safety Assessment of Foods Derived from Recombinant-DNA Plants Modified for Nutritional or Health Benefits in Guideline for the Conduct of Food Safety Assessment of Foods Using Recombinant DNA Plants. Doc CAC/GL 45-2003. Rome.
World Health Organization (2020a). Origin of SARS-CoV-2. Available online at: https://apps.who.int/iris/bitstream/handle/10665/332197/WHO-2019-nCoV-FAQ-Virus_origin-2020.1-eng.pdf (accessed November 28, 2020).
World Health Organization (2020b). Antibiotic Resistance. Available online at: https://www.who.int/news-room/fact-sheets/detail/antibiotic-resistance (accessed November 29, 2020).
Worobey, M., Han, G. Z., and Rambaut, A. (2014). A synchronized global sweep of the internal genes of modern avian influenza virus. Nature 508, 254–257. doi: 10.1038/nature13016
Zhang, L., and Dullaghan, N. (2021). Cultured Meat: A Comparison of Techno-Economic Analyses. Rethink Priorities. Available online at: https://rethinkpriorities.org/publications/cultured-meat-a-comparison-of-techno-economic-analyses
Ziegler, J. (1991). Guidelines for Slaughtering, Meat Cutting and Further Processing. Food and Agricultural Association, Rome. Available online at: http://www.fao.org/3/t0279e/t0279e05.htm
Zoon, K. (1993). Points to Consider in the Characterization of Cell Lines Used to Produce Biologicals. Food and Drug Administration. Available online at: https://www.fda.gov/media/76255/download
Keywords: food security, cellular agriculture, cultured meat, centralization, decentralization, distributed, local
Citation: Soice E and Johnston J (2021) How Cellular Agriculture Systems Can Promote Food Security. Front. Sustain. Food Syst. 5:753996. doi: 10.3389/fsufs.2021.753996
Received: 05 August 2021; Accepted: 23 October 2021;
Published: 26 November 2021.
Edited by:
Steffanie Scott, University of Waterloo, CanadaReviewed by:
Evan Fraser, University of Guelph, CanadaLenore Newman, University of the Fraser Valley, Canada
Copyright © 2021 Soice and Johnston. This is an open-access article distributed under the terms of the Creative Commons Attribution License (CC BY). The use, distribution or reproduction in other forums is permitted, provided the original author(s) and the copyright owner(s) are credited and that the original publication in this journal is cited, in accordance with accepted academic practice. No use, distribution or reproduction is permitted which does not comply with these terms.
*Correspondence: Jeremiah Johnston, amVyZW1pYWhAbmV3LWhhcnZlc3Qub3Jn