- 1Department of Cell Biology, The Franciszek Górski Institute of Plant Physiology, Polish Academy of Sciences, Kraków, Poland
- 2Department of Biotechnology, Faculty of Natural Sciences, University of St. Cyril and Methodius in Trnava, Trnava, Slovakia
- 3Plant Protection Institute, Centre for Agricultural Research, Budapest, Hungary
- 4Department of Biology, Faculty of Natural Sciences, University of St. Cyril and Methodius in Trnava, Trnava, Slovakia
In this review, we describe and integrate the latest knowledge on the signaling role of proteins and peptides in the stress-induced microspore embryogenesis (ME) in some crop plants with agricultural importance (i.e., oilseed rape, tobacco, barley, wheat, rice, triticale, rye). Based on the results received from the most advanced omix analyses, we have selected some inconspicuous but possibly important players in microspores reprogramming toward embryogenic development. We provide an overview of the roles and downstream effect of stress-related proteins (e.g., β-1,3-glucanases, chitinases) and small signaling peptides, especially cysteine—(e.g., glutathione, γ-thionins, rapid alkalinization factor, lipid transfer, phytosulfokine) and glycine-rich peptides and other proteins (e.g., fasciclin-like arabinogalactan protein) on acclimation ability of microspores and the cell wall reconstruction in a context of ME induction and haploids/doubled haploids (DHs) production. Application of these molecules, stimulating the induction and proper development of embryo-like structures and green plant regeneration, brings significant improvement of the effectiveness of DHs procedures and could result in its wider incorporation on a commercial scale. Recent advances in the design and construction of synthetic peptides–mainly cysteine-rich peptides and their derivatives–have accelerated the development of new DNA-free genome-editing techniques. These new systems are evolving incredibly fast and soon will find application in many areas of plant science and breeding.
Introduction
By 2035, the human population is expected to reach around 8.9 billion, and by the middle of the 21st century nearly 10 billion (https://www.prb.org/international/geography/world/). This demographic tendency, coinciding with environmental change, increases the demand for food supply (Calicioglu et al., 2019). In 2050, food production will need to be increased by about 50% compared to that in 2012 (Global monitoring report 2015/2016). This is a big challenge for agricultural producers and breeders, especially that the progress is hampered by the exacerbated climate change and substantial loss of biodiversity. Meeting this demand would require that agricultural sector including plant breeders increase sustainable agricultural production by introducing more efficient methods of obtaining improved varieties of high quality and stable yield in a short time. Conventional breeding is labor intensive and time-consuming process, which needs to be complemented with modern biotechnological approaches to improve its efficiency. The abovementioned problems point to the necessity to deliver new plant varieties with enhanced productivity and improved adaptation toward abiotic and biotic stresses. For this purpose, microspore embryogenesis (ME), known also as androgenises, seems to be an unrivaled biotechnological tool to speed up the progress of plant breeding. During ME, the differentiation of immature male gametophyte cells (microspores) into pollen grains is blocked and redirected toward embryo development. This process is triggered by stress and requires in vitro culture techniques. From one side, the difficulties associated with optimization of in vitro culture conditions are often limiting factors in ME incorporation as a research model or tool in biotechnology or breeding, on a larger scale. On the other hand, the use of in vitro culture provides direct insight into the process of ME, which follows a pattern very similar to the development of the zygotic embryo in planta.
The final products of ME are doubled haploids (DHs), homozygous at all loci, what brings significant benefits to many basic research areas and plant breeding. Elimination of heterozygosity simplifies genome sequencing, reverse breeding, quantitative genetic research and discovering of recessive, dominant and deleterious mutations. That's why DHs are interesting objects of studies in many research areas including physiology, molecular biology, genetics and epigenetics (Maluszynski et al., 1996; Castillo et al., 2001; Touraev et al., 2001; Forster et al., 2007; Szarejko and Forster, 2007 and references therein; Dirks et al., 2009; Dunwell, 2010; Chauhan and Khurana, 2011; Ferrie and Möllers, 2011; Krzewska et al., 2012, 2017; Marathi et al., 2012; Żur et al., 2014a,b, 2015a,b; Barakat et al., 2017; Ren et al., 2017; Song et al., 2017; Shchukina et al., 2018; Tyrka et al., 2018; Nowicka et al., 2019; Shi et al., 2019; Testillano, 2019; Wajdzik et al., 2019; Bilichak et al., 2020; Malaga et al., 2020). Furthermore, DH technology combined with marker assisted selection (MAS) enables precise identification of plants with enhanced/silenced expression of even one gene of interest within the genome. Due to the fact that total homozygosity is received in one generation, incorporation of DH technology into breeding programmes saves time necessary to develop and release new, improved cultivars (reviewed in Kasha and Maluszynski, 2003; Germanà, 2006, 2011; Forster et al., 2007; Wedzony et al., 2009; Dwivedi et al., 2015). DH lines can be considered as a new variety when self-pollinated or can be used as a parental inbred line for the production of hybrid varieties after cross-pollination and in germplasm conservation. DH technology is also used to fix traits obtained through transformation and mutagenesis and to develop genetically-fixed molecular mapping populations.
ME is one of the simplest and most effective methods available for haploids/DHs production for a wide range of crops (Wedzony et al., 2009; Ferrie and Möllers, 2011; Germanà, 2011). Established protocols for the ME induction are used in various species, varieties, breeding lines and are based on different experimental approaches, what limits the ability to identify universal solutions leading to redirection of microspores toward sporophytic development, formation of embryo-like structures (ELS) and differentiation into haploid plantlets. Finally, green and fertile DH plants are obtained by spontaneous or chemically induced genome doubling. Our understanding of the processes that occur during ME, come generally from two dicotyledonous species: rapeseed (Brassica napus) and tobacco (Nicotiana tabacum), as well as three monocots: wheat (Triticum aestivum), barley (Hordeum vulgare) and rice (Oryza sativa) (Hosp et al., 2007; Ferrie and Möllers, 2011; Germanà, 2011; Soriano et al., 2013; Wedzony et al., 2014; Seifert et al., 2016; Bélanger et al., 2018, 2020; Shahmir and Pauls, 2021). However, the majority of information regarding the control and regulation of ME induction is based on studies on single, highly responsive genotypes within a given species, like cv. Igri (Jacquard et al., 2009) and cv. Gobernadora (Bélanger et al., 2018) in barley and wheat cv. Svilena (Seifert et al., 2016), and rapeseed DH line 4,079 (Joosen et al., 2007) or producing the embryogenic callus DH line 12,075 (Soriano et al., 2013, 2014; Corral-Martínez et al., 2020). Therefore, a large-scale examination, comparing genotypes of high embryogenic potential with genotypes recalcitrant to ME under the same inducible conditions is of great value and could provide direction for more effective approaches to DH production and its wider incorporation into breeding programmes. Recently, the group of crop species that has been considered as a subject of ME has been extended to hexaploid triticale (× Triticosecale Wittm.) and its parental species, rye (Secale cereale L.). Both species belong to cereals economically valuable in Northern Europe and North America, due to its yield potential, specific nutritional values of grain and high tolerance to environmental conditions. Studies conducted on several DH lines (triticale) and F1 breeding lines (rye) highly differentiated in respect of ME effectiveness, gave us the possibility for more accurate identification of factors related to ME effectiveness (Żur et al., 2008, 2009, 2012, 2014a, 2015a,b, 2019; Krzewska et al., 2012, 2017; Nowicka et al., 2019; Zieliński et al., 2020, 2021). The phenomenon of ME was for the first time observed in 1964 (Guha and Maheshwari, 1964), but its complex and multifaceted nature makes it difficult to investigate, so still the mechanisms of molecular control and regulation have not yet been precisely described (Maraschin et al., 2005; Hosp et al., 2007; Elhiti et al., 2013; Seifert et al., 2016). It is known that the effectiveness of ME is determined by many internal and external factors and their complex interactions. Induction of microspore reprogramming and then initiation of its embryogenic development is accompanied with many changes in molecular, biochemical, physiological and cytological processes (e.g., gene expression, DNA methylation, chromatin organization, redox and hormonal homeostasis) (Pauls et al., 2006; Seguí-Simarro and Nuez, 2008; El-Tantawy A.-A. et al., 2014; Żur et al., 2014a,b, 2015a,b; Solís et al., 2015; Berenguer et al., 2017).
Microspore reprogramming can be induced by various stress factors (e.g., low temperature, heat, starvation, chemical agents and their combinations), which can be applied to the whole plants, harvested spikes, isolated anthers or microspores. However, the effect of the treatment depends on the donor plant genotype, its physiological condition and growing season—all of which influence the level of cell stress tolerance (Żur et al., 2019). The exposure to these stress factors leads to oxidative stress that is induced by increased levels of reactive oxygen species (ROS) including free radicals such as superoxide anion () and hydroxyl radical (OH·), and non-radicals like hydrogen peroxide (H2O2), singlet oxygen (1O2) and lipid peroxides (LOOH). It was hypothesized, that recalcitrance to ME could be caused by low ability to counter the oxidative stress induced during initiation of ME and/or transfer of microspores to in vitro culture conditions (Żur et al., 2008, 2009, 2014a; Jacquard et al., 2009). ROS together with other reactive compounds derived from ROS-mediated oxidative damage to cellular macromolecules (oxylipins, peptides, mRNAs, DNA) modulate cellular signal transduction and the post-transcriptional gene expression associated with secondary metabolism, stress responses and cellular detoxification (e.g., genes encoding phosphatases, kinases and transcription factors) (Chmielowska-Bak et al., 2015; Schnaubelt et al., 2015; He et al., 2018). These oxidative modifications result in changes in expression, structure and/or function of the proteins. Aggregation or fragmentation of the polypeptide chains activate proteolysis and cell damage, which can lead finally to the cell death (Shan et al., 2007).
When ROS generation overwhelms the antioxidative capacity in plant cells, various signaling pathways are activated that trigger physiological, biochemical, and molecular responses of cellular metabolism, probably necessary for microspore reprogramming and embryogenesis initiation (Żur et al., 2008, 2009, 2012, 2014a, 2015a, 2019). What is more, even high level of ROS does not endanger microspore viability as long as the cells exhibit high activity of ROS-scavenging enzymes (Żur et al., 2014a, 2019, 2021).
There are two main cellular systems controlling ROS: non-enzymatic, low molecular weight antioxidants (e.g., ascorbate, tocopherols, reduced glutathione, flavonoids, etc.) and antioxidative enzymes such as superoxide dismutase (SOD), catalase (CAT) and various peroxidases (Foyer and Noctor, 2003; AbdElgawad et al., 2016). All these antioxidants support sustaining the redox balance in the cell and play a crucial role in the defense against oxidative stress.
The capability of antioxidants to counteract the effect of ROS is related to their structure and chemical properties. It may arise from the presence of: (i) metal ions (Mn, Cu/Zn and Fe in SOD; Fe–in CAT; S–in other metalloproteins, glutathione peroxidase (GPX) and glutathione S-transferase (GST); (ii) conjugated double bonds like in ascorbic acid, tocopherol and β-carotene; (iii) aromatic rings like in flavonoids; and (iv) the thiol (or “sulfhydryl”) group like in glutathione and thioredoxin (TrX) (Flora, 2009; Rahantaniaina et al., 2013). The thiol/thiolate group of the redox-reactive cysteine (Cys) gives the ability to form disulfide bonds with nearby cysteines (–S–S–) or undergo further oxidation to sulfinic (–SO2H) or sulfonic (–SO3H) acid. Any oxidation-reduction (redox) modifications of cysteinyl residues lead to post-translational modifications (PTMs) that impact on molecular functions important to cellular processes, including signal transduction (Poole, 2015). Both PTMs and the amino acids sequence determine protein features and functions (Zhang et al., 2021).
To learn more about molecules which belong to the most important factors enhancing ME induction efficiency, scientists developed a suite of highly advanced research methods and biotechnological tools e.g., next generation sequencing (NGS), improved biochemical isolation procedures, gene prediction/annotation bioinformatics tools and genome-editing approaches (Sahu et al., 2020). The manipulation of metabolic pathways by non-GMO genetic engineering is a strategy that may increase the tolerance of plants against abiotic stress. Recent technological advances in crop genomics allow to discover genetic variation in breeding material and permit genome-based breeding to deliver cultivars for the projected food requirements for 2050 (Rasheed and Xia, 2019). Due to the development of modern techniques, several potential candidates for stimulation of induction and proper development of ELS–mainly among cysteine-rich or small signaling peptides and proteins–have been reported in recently published papers. These results allow us to address the roles of these inconspicuous but possibly important molecular players in the regulation of ME. Inspired by previous and recent findings, we review how stress tolerance-related proteins (e.g., β-1,3-glucanases, chitinases) and small signaling peptides, especially cysteine-(Cys, e.g., glutathione, γ-thionins, rapid alkalinization factor, lipid transfer, phytosulfokine) and/or glycine-rich peptides and other proteins (e.g., fasciclin-like arabinogalactan protein), particularly those involved in the regulation of cellular redox potential or the cell wall reconstruction could be promising tools for improving DH production in crop plants.
Stress-Related Proteins
The balance between ROS generation and scavenging determines the level of stress tolerance in plants by modifying the profile of defense-related genes coding for molecules like pathogenesis-related (PR) proteins as β-1,3-glucanases (PR2), chitinases (PR3) or γ-thionins (PR13) (Chinnusamy and Zhu, 2009; Sahu et al., 2013; Wojtasik et al., 2019).
β-1,3-glucanases (EC 3.2.1.39) are hydrolytic enzymes (GH17) that catalyze the cleavage of 1,3-ß-D-glucosidic linkages in ß-1,3-glucan, commonly referred to as callose (Levy et al., 2007; Chen et al., 2009; Wu et al., 2018). Callose is a component of cell walls or cell walls associated structures; and forms the barriers that regulate intercellular trafficking (Chen and Kim, 2009; Wu et al., 2018). In higher plants, callose plays important roles in many biological processes as well as in plant defense response (Chen et al., 2009; Wu et al., 2018; Wang et al., 2021). β-1,3-glucanases together with callose synthases regulate callose homeostasis (Chen et al., 2009; Wu et al., 2018). Based on their structure, plant β-1,3-glucanases have been classified into four classes (I–IV) (Doxey et al., 2007). The class I comprises basic vacuolar β-1,3-glucanases whereas classes II–IV include acidic extracellular enzymes (Grover, 2012). In plants, these hydrolases are called PR2 proteins, as they are expressed when plants are exposed to biotic (Moravčíková et al., 2004; Żur et al., 2013) and/or abiotic stresses (Mészáros et al., 2011; Żur et al., 2013; Gregorová et al., 2015). Moreover, these enzymes are undoubtedly essential for plant growth and development including microsporogenesis, pollen development or seed germination (Leubner-Metzger and Meins, 1999; Michalko et al., 2017). Their activities, leading to the production of (1 → 3)-linked β-glucan oligosaccharides with signaling properties were reported in both zygotic (Petrovská et al., 2010), somatic (Helleboid et al., 2000; Fráterová et al., 2013) as well as in microspore embryogenesis (Borderies et al., 2004; Muñoz-Amatriaín et al., 2009; Leljak-Levanić et al., 2015; Zieliński et al., 2021). Several β-1,3-glucanases were detected in stress pre-treated anthers of several plant species (Table 1). Recently, Zieliński et al. (2021) identified an anther-specific and stress-responsive β-1,3-glucanase fraction of 26 kDa and several acidic isoforms in rye. Since direct correlation between the activity of β-1,3-glucanases and the final efficiency of the ME was not observed, it was supposed that β-1,3-glucanases mediate defense responses in the early stages of ME induction. We also assume that their activity is important only for the inhibition of gametophytic pollen development, because no activity of β-1,3-glucanases was found in competent and embryogenic microspores in the rye line responsive to ME (Zieliński et al., 2021). It might be related to the microspore wall remodeling via a rapid production of abnormal, callose-rich sub intimal layer of cell walls (Dubas et al., 2013; Parra-Vega et al., 2015; Rivas-Sendra et al., 2019). Thickening of the cell wall with an extra osmoprotective sub intimal callose deposition protects the microspores from cell death and physically isolates them from the outer environment (Parra-Vega et al., 2015; Rivas-Sendra et al., 2019). It stimulates the rearrangements of microspore structure, such as location of the nucleus (central), vacuolization (numerous small vacuoles) and the distribution of cytoplasmic bands (numerous cytoplasmic bridges). Microspores, with poor callose layer are more exposed to the risk of blocked reprogramming (Rivas-Sendra et al., 2019). β-1,3-glucanases activity might be important later for the regulation of embryo differentiation (Helleboid et al., 2000; Borderies et al., 2004).
Besides β-1,3-glucanases, chitinases are also expressed in a response to stress-initiated microspore reprogramming (Zieliński et al., 2021). Plant chitinases (PR3, EC 3.2.1. 14) are glycoside hydrolases that cleave the β-1,4 glycosidic linkages of chitin. As chitin is a structural component of most fungal cell walls (Fesel and Zuccaro, 2016), chitinases were mainly studied in relation to defense response against fungal pathogens (Moravčíková et al., 2004; Żur et al., 2013). Structurally, plant chitinases are categorized into six classes (I–VI). The classes I, II, IV and VI belong to the subfamily GH19 that is exclusively present in plants. The classes III and V comprise chitinases of the subfamily GH18 that are found in bacteria, fungi, viruses, animals, and few plant species (Minic, 2008; Grover, 2012). Like β-1,3-glucanases, chitinases are upregulated under both biotic (Żur et al., 2013) and abiotic stress (Mészáros et al., 2011; Żur et al., 2013; Gregorová et al., 2015) and their synergistic effect in plant defense has been observed in many plant species (Moravčíková et al., 2004; Żur et al., 2013). These enzymes are also active in healthy plants in an organ-specific and developmentally regulated pattern (Kasprzewska, 2003). Activities of chitinases have been reported to be associated with embryogenesis: zygotic (Sánchez-Díaz et al., 2013), somatic and ME (De Vries et al., 1988; Coutos-Thevenot et al., 1992; Hilbert et al., 1992; Nielsen and Hansen, 1992) (Table 1). At least three chitinase fractions (~30, ~34, and ~95 kDa) were identified in stress pre-treated anthers of two rye breeding lines regardless of the type of the stress treatment (Zieliński et al., 2021). Although, these chitinases were not anther-specific, their presence (especially 28–35 kDa fractions) was associated with the plant defense responses according to literature data (Ferreira et al., 2007; Kuwabara and Imai, 2009; Żur et al., 2013). Chitinases of a ~25 and ~28 were detected in the induction medium, obviously secreted by maize microspores during embryo generic development (Borderies et al., 2004). In the earlier stages of ME in rye anther culture, chitinases were also detected although their activity was very low (Zieliński et al., 2021). The presence of chitinases may result in the generation of molecules that have a stimulating effect on changing microspore structure which precede an ELS development (Matthys-Rochon, 2005).
The morphological changes are a consequence of molecular events and are connected with modified expression of genes encoding endochitinases (CHIT1, CHIT2) (Zieliński et al., 2021), arabinogalactan-like proteins (ECA) and lipid transfer proteins (LTP) (Vrinten et al., 1999; Hosp et al., 2007; Malik et al., 2007), which may be involved in membrane and cell wall remodeling at the initial phases of ME and during an ELS development (Malik et al., 2007). Lower plasma membrane fluidity in microspores of B. napus line of high embryogenic potential seems to maintain proper cell protection and may lead to efficient embryogenesis induction (Dubas et al., 2013). The cell wall arabinogalactan proteins (AGP) contain N-acetyl glucosamine residues can be a target for endochitinase cleavage (van Hengel et al., 2001; van Hengel and Roberts, 2002; Kasprzewska, 2003; Minic, 2008) and thereby involved in both wall architecture and cellular regulatory processes (Pfeifer et al., 2020).
The monosaccharide derivative of glucose, N-acetyl glucosamine (GlcNAc) is cross-linked by short peptides that may have functions in cell signaling (Konopka, 2012; Naseem et al., 2012). Intracellular GlcNAcylation of serine and threonine residues is a well-known and widely investigated post-translational modification in plant cells. Modifications of cysteine (Cys) S-linked N-acetyl glucosamine (S-GlcNAcylation) were recently found as a new post-translational modification in mammals (Maynard et al., 2016). S-GlcNAc is a sulfur-linked analog of O-GlcNAc, which modification is enzymatically stable at both peptide and protein levels (Olszewski et al., 2010; De Leon et al., 2017). The presence of such process is a subject of intense research in plants.
Thionins, peptides composed of 45 to 48 amino acid residues with the molecular weight of ~5 kDa, containing six or eight cysteines, and three or four disulfide bonds belong to the other molecules undergoing PTMs (Melo et al., 2002; Lyapina et al., 2019; Li et al., 2021). Due to their three-dimensional structure, thionins (sulfur-containing cysteine residues) are divided into α-thionins, β-thionins, and γ-thionins (belonging to PR13 and to the part of the defensins PR12) (Lacerda et al., 2014; Nawrot et al., 2014; Plattner et al., 2015; Salas et al., 2015; Tam et al., 2015). As thionins are involved inter alia in signaling, their possible involvement in ME will be detailed described in the chapter below.
Cysteine-Rich Tripeptide (Glutathione)
The most abundant small thiol molecule—the tripeptide glutathione (γ-glutamyl cysteinyl glycine; GSH) is synthesized in all living cells and belongs to the major redox regulators in plant cells (Poole, 2015). The availability of Cys, produced in mitochondria, is the rate limiting step of GSH synthesis (Foyer and Rennenberg, 2000; Noctor et al., 2012), which takes place in the cytosol by the sequential action of γ-glutamylcysteine synthase (γ-GCS) and GSH synthase (GS) (Queval et al., 2011; Zechmann, 2020). Strongly influenced by day/night illumination, GSH content varies among different developmental stages, organs, tissues or cell compartments (Diaz Vivancos et al., 2010a,b; Chiu and Dawes, 2012) but its relatively high and stable level is important for plant development. GSH occurs in millimolar concentrations (0.3–15 mM) in vegetative tissues, with the highest concentration in mitochondria, followed by nuclei, peroxisomes, cytosol and plastids (Zechmann et al., 2008; Zechmann and Müller, 2010). Almost 7.2% of cellular GSH can be detected in mitochondria according to Queval et al. (2011). Although GSH can be found at much lower concentrations in immature pollen grains (vacuolated microspores), it plays a pivotal role in pollen germination (Zechmann et al., 2011). The compartmentation of GSH, maintaining intracellular redox homeostasis, is of great importance for many physiological processes and metabolic regulation (Bowsher and Tobin, 2001; Hartmann et al., 2003). Under stress conditions, excess ROS (e.g., H2O2) may stimulate the oxidation of the thiol group of Cys residue to glutathione disulfide (GSSG), which in turn is reduced back to GSH by glutathione reductase (GR), which utilizes NADPH as a reductant (Edwards et al., 1990; Schwarzländer et al., 2008; Marty et al., 2009; García-Quirós et al., 2017). GSH is also used as a substrate in reactions catalyzed by GPXs in the ascorbate–glutathione cycle (Vanderauwera et al., 2011; Tuzet et al., 2019). Moreover, GSSG reacts non-enzymatically with protein thiol groups creating protein–SSG mixed disulfides (Kalinina and Novichkova, 2021). An appropriate GSH/GSSG ratio is crucial for cellular redox homeostasis and regulates cell metabolism including the accumulation and transport of Cys, and participates in the regulation of gene expression, DNA and protein synthesis, regulation of cell cycle, cell differentiation, and PCD (Foyer et al., 2001; Foyer and Noctor, 2005; Maughan and Foyer, 2006; Szalai et al., 2009; Queval and Foyer, 2012; Deponte, 2013; Schnaubelt et al., 2015).
Regarding the regulatory role of GSH in cellular processes, recent work has identified several GSH-responsive genes in Arabidopsis thaliana including transcription factors (SPATULA, MYB5, MYB75) and proteins involved in the regulation of redox potential (e.g., thioredoxins, glutaredoxin), cell divisions, auxin biosynthesis, its transport and transcriptional response (HECATE) (Schnaubelt et al., 2015). In vitro-cultured microspores challenged by stress factors during ME seem to be a perfect model for investigation of GSH homeostasis in single cells and microspore-derived embryos. Increasing number of data revealed the expression of genes encoding GSTs during the initiation of ME by exogenous stress (Maraschin et al., 2006; Jacquard et al., 2009; Sánchez-Díaz et al., 2013; Żur et al., 2014b; Bélanger et al., 2018). The up regulation of GST genes has been identified during ME in both the initial phase (Vrinten et al., 1999; Maraschin et al., 2006; Muñoz-Amatriaín et al., 2006, 2009; Jacquard et al., 2009; Sánchez-Díaz et al., 2013; Żur et al., 2014b) and throughout the multicellular embryo formation (Joosen et al., 2007; Malik et al., 2007; Tsuwamoto et al., 2007). The maintenance of a reduced cellular environment (high GSH availability) during the early phases induces microspore reprogramming, promotes cell proliferation, and increases the number of produced ELSs by enhancing nucleotide synthesis and mitotic activity (Stasolla, 2010; Żur et al., 2021). Presumably, high GSH level in nuclei of microspores is required for the G1 to S phase (phases preceding mitosis) transition. However, a more oxidative environment where glutathione pool is switched toward an oxidized state favors the continuation of embryo development.
Increased expression of GSTs functioning as GPXs is important for protection against oxidative injuries especially to detoxify harmful organic hydroperoxides of fatty acids (Dixon et al., 2002; Dixon and Edwards, 2009; Kim et al., 2011; Kayum et al., 2018). Such multifunctionality of GSTs, some of which have been differentially expressed during ME induction, has prompted scientists to manipulate GSTs expression, but also to look for new, more specific gene candidates.
In our studies, in order to manipulate GSH biosynthesis or GPXs activities for more effective ME induction, exogenous tillers pre-treatment with GSH, specific GSH precursors (L-2-oxothiazolidine-4-carboxylic acid; OTC) and inhibitor of the rate-limiting enzyme in GSH synthesis (buthionine sulfoximine; BSO) were used. Exogenous application of GSH or OTC may support antioxidant defense and alter the redox status regulating cell proliferation what was shown in several studies on stress-induced non-zygotic embryo development (Żur et al., 2019 and references therein; Żur et al., 2021). However, the effect of the treatment was strongly dependent on the genotype-specific activity of endogenous antioxidative system. As some threshold level of ROS is necessary for microspore reprogramming (Żur et al., 2008, 2009, 2012) excessive elimination of these signaling molecules could increase microspore vitality but simultaneously diminish the frequency of ME initiation (Żur et al., 2012, 2019; Zieliński et al., 2020). Recent studies conducted on triticale and rye revealed that ME-recalcitrance could be to some extent overcome by a treatment with a combination of low temperature (LT), mannitol (MAN) and GSH (Żur et al., 2019, 2021; Zieliński et al., 2020). The applied 0.3–0.7 M MAN can be considered as either a mild osmotic stress inducing agent, an osmoprotectant or a quencher of ROS (Meena et al., 2015). However, the effect of the treatment was influenced by various endogenous and environmental factors and fluctuated significantly (see the detailed description below).
Embryogenic competence of plants may be linked to the endogenous glutathione content and its estimation in flag leaves and anthers of the pre-treated tiller can be used as a marker of embryogenic potential. Although the reduced form of glutathione (GSH) predominates over the oxidized form (GSSG) in typical eukaryotic cells, representing more than 99% of the total glutathione pool, the predominant form of glutathione in rye leaves was the oxidized form (GSSG), at average concentrations ranging from 1.463 to 2.149 μM g−1 FW−1 in ME-recalcitrant and ME-responsive lines, respectively (Zieliński et al., 2018). The GSSG content was about 68% of the total glutathione content [GSH + GSSG] in the recalcitrant lines, 55.5% in the moderately responsive lines and 50% in the responsive lines. It was shown that high GSH concentration in rye anthers associated with an increase in ROS production and an increase in GSH:GSSG ratio enables relatively efficient induction of ME. It was observed that the concentration of GSH in anthers of ME-recalcitrant lines was 1.13-fold lower than in ME-responsive lines and 1.22-fold lower than in lines characterized as moderately responsive. Increase in GSH level could be achieved by MAN treatment of tillers, which decreases the rate of GSH oxidation, reduces the environment of the cytoplasm and stimulates ME induction (Zieliński et al., 2019).
As redox state regulates epigenetic mechanisms represented by DNA methylation, and histone acetylation and methylation (Wang et al., 2016; Locato et al., 2018), this raises the possibility that the main cell redox regulator—GSH, might affect the expression of genes coding for cell cycle regulators (Locato et al., 2015, 2018). In mammalian cells, GSH levels influence the chromatin structure by means of glutathionylation of histone H3 (García-Giménez et al., 2013; García-Giménez and Pallardo, 2014; Huang et al., 2019). In addition, GSH can work in the opposite way, inhibiting the activity of enzymes involved in the synthesis of S-adenosyl-methionine (SAM, sulfur containing methionine), important for cell functioning and survival. This molecule is used by DNA methyltransferases (DNMTs) and histone methyltransferases (HMTs) as a substrate for DNA and histone methylation, respectively (García-Giménez and Pallardo, 2014; García-Giménez et al., 2017). Moreover, SAM could be also utilized in trans-sulfuration reactions (conversion of methionine into cysteine) and is an intermediate in the biosynthesis of polyamines, nicotianamine, biotin and ethylene (Roeder et al., 2009). Finally, based on the transcriptional profiling of barley microspores (embryogenic cv. Igri and non-embryogenic cv. Golden Promise; GP), it can be speculated that MAN may also participate in epigenetic processes, inhibiting the activity of the enzymes involved in the synthesis of SAM (our unpublished results). Our interpretation is partially supported by the work of Castillo et al. (2020), who found that the histone deacetylase inhibitor trichostatin A (TSA) together with MAN treatment led to microspore reprogramming, increased the number of embryogenic microspores and regenerated green plants in bread wheat. However, this hypothesis needs to be further investigated.
Other Cysteine-Rich and the Small Post-translationally Modified Peptides
The interplay between ROS and acylation might play important roles in the PTMs of peptides and proteins associated with many metabolic processes during plant growth and environmental stress responses (Simon and Dresselhaus, 2015; Zhou et al., 2018). Among small and secreted peptides involved in plant signaling and cell-to-cell communication there are two major classes: (i) the cysteine-rich peptides (CRPs) and (ii) the small post-translationally modified peptides (PTMPs) derived from a proteolytic processing (Murphy et al., 2012; Albert, 2013; Czyzewicz et al., 2013; Matsubayashi, 2014; Simon and Dresselhaus, 2015; Tavormina et al., 2015; De Coninck and De Smet, 2016). Proteolytic breakdown of proteins leads to the formation of smaller than 10 kDa polypeptides (many amino acids), oligopeptides (e.g., 2 to 20 amino acids), or amino acids differently regulating the activities of other molecules within the cell. Many of these peptides usually harbor certain sequence patterns or motifs i.e., rich in a Cys, glycine or tyrosine. For example, CRPs (~5 kDa) which contain 2 to 16 Cys residues have been shown to form disulfide bridges and mainly function as antimicrobial peptides (van der Weerden et al., 2013; Tavormina et al., 2015; reviewed by De Coninck and De Smet, 2016). The CRs also regulate stomatal patterning and density, symbiosis and a wide range of reproductive processes such as pollen tube germination, guidance and burst, gamete activation, and seed development (Hara et al., 2007; Sugano et al., 2010; Maróti et al., 2015; Bircheneder and Dresselhaus, 2016; De Coninck and De Smet, 2016).
Among signaling peptides possibly involved in ME, a special focus should be given to the γ-thionins, rapid alkalinization factor (RALF), lipid transfer proteins (LTP) from CRPs and phytosulfokine (PSK) as well as glycine-reach proteins (GRPs) from PTMPs.
It is especially interesting to consider the possible involvement of γ-thionins in ME induction and ELS development since, due to their positive charge, thionins are able to interact with glycolipids, glucoceramides and sphingolipids (Yamuna et al., 2019). Plant γ-thionins can occur in all tissues including the female gametophyte, flowers, pollen, shoots, cotyledons, leaves, roots, bark, the endosperm of immature kernels and fruits. Their functional roles depend on the specific interactions with the plasma membrane (reviewed by Carvalho and Gomes, 2009, 2011; De Coninck and De Smet, 2016; Nikte et al., 2020). Several plant γ-thionins have been shown to induce the accumulation of intracellular ROS and to initiate PCD that are part of regulatory cascades leading to the protection or tolerance against pathogens (Hegedüs and Marx, 2013). The involvement of γ-thionins in other defense reactions, including responses to cold, salt and drought stresses has also been reported (reviewed by De Coninck et al., 2013). Thionin gene silencing in plants is associated with enhanced susceptibility to pathogens, while its overexpression confers improved resistance (Chan et al., 2005; Lee et al., 2008). It was also suggested, that the regulations between thionins and WRKY transcription factors (TFs) are important for pollen development and functioning. WRKY TFs have been demonstrated to play critical roles in plant development and stress responses (Lei et al., 2017). WRKY33 negatively regulates ABA signaling (Liu S. et al., 2015), WRKY34 is required in the early stages of pollen development (Guan et al., 2014), whereas WRKY2 plays a role in embryo development (Ueda et al., 2011). Transcriptional profiling of responsive and recalcitrant barley genotypes (cv. Igri and cv. Golden Promise, respectively) revealed a novel HORVU.MOREX.r2.5HG0368810.1 gene coding for a thionin family protein which possibly contribute to the acquisition of embryogenic potential by microspores under osmotic stress. We found a significantly higher (4.7-fold) expression of this microspore-specific thionin gene in highly responsive cv. Igri in comparison with the recalcitrant cv. GP, what suggests its important role in stress defense mechanism leading to ME induction. Its possible function may be associated with modifications in the hydrophobic region of the membrane of the microspore (Gene Ontology ID; GO: 0016021).
Another interesting candidate selected through barley transcriptome analysis is a Cys-rich peptide hormone RALF. It is processed from a larger precursor by a Golgi-localized subtilisin-like protease activity (Morimoto and van der Hoorn, 2019) and possibly released into the extracellular matrix like in animals and yeast (Covey et al., 2010). It is produced in response to rapidly changing environmental conditions and found to be biologically active in many developmental processes, including ME. RALF precursors are found to be encoded by single genes or members of multigene families and expressed in different tissues (leaves, elements of flowers) and organs from several species like Arabidopsis, soybean, Primula vulgaris, tomato, Solanum chacoense, Solanum lycopersicum, broccoli and Brassica campestris. RALF is a 49 amino acid peptide (5 kDa) with four cysteine residues that form two disulfide bridges. It is ubiquitous and has been associated with stress responses and cell elongation by controlling vacuolar expansion (Dünser et al., 2019; Blackburn et al., 2020). When added to the medium of suspension cultures of tobacco, RALF causes a pH increase followed by changes in proton flux and MAP kinase activation (Pearce et al., 2001), as well as changes in ROS generation and cytoplasmic Ca2+ level (Guerreiro et al., 2013 and citations therein). By that way RALF may regulate diverse receptor kinase complexes during growth and development, or for environmental sensing (Stegmann et al., 2017). Exogenous pollen-specific tomato RALF (SlPRALF) inhibits pollen tube growth (Covey et al., 2010).
Recently, we have found HvRALF gene (HORVU.MOREX.r2.3HG0195730.1) as one of the 48 most abundant transcripts in embryogenic microspores of ME-responsive barley cv. Igri. The same transcripts were found at lower level in embryogenic structures after the first symmetrical divisions in in vitro culture, what suggests its involvement in the acquisition of embryogenic potential by microspores.
Changes in pH affect not only stiffness of the cell wall, but also the entry of auxin and other pH-responsive hormones into cells, and the activity of many enzymes. For example, RALFs may modulate cell wall by influencing the activity of pH-sensitive cell wall modification proteins, including pectin methylesterases, exo-β-glucanases and/or expansins. We found that up regulation of RALF gene may be associated, with lower expression (21.7-fold) of expansin gene (HORVU.MOREX.r2.2HG0156970.1) in barley embryogenic microspores. Expansins (EXP), extensins (EXT), and as mentioned before, AGPs are well-characterized in regulating cell wall expansion. EXP belongs to cell wall-loosening proteins, stimulating wall expansion at acidic pH, similar to AGPs that specifically accumulates at pH 6.0 (Li et al., 2012). Acidification can be one of the factors necessary for ME initiation through cascade of chemical and structural changes of wall polysaccharides leading to weaker cellulose–pectin interactions and excessive hydration of both cellulose micro fibrils and matrix polysaccharides. These changes lead to the cell wall loosening and expansion, and may occur both independently or as a result of protein-mediated wall loosening (Cosgrove, 2000; Phyo et al., 2018). Because AGPs contains endochitinase cleavage sites (van Hengel et al., 1998; Showalter, 2001), these enzymes can split AGP into small oligosaccharides which may be used as signaling molecules involved in various processes, among others in induction of embryogenic development.
AGPs belong to the subfamily of hydroxyproline-rich glycoproteins that are generally located in cell walls, plasma membranes or secreted into the apoplast (Mareri et al., 2018; Leszczuk et al., 2019; Testillano, 2019). AGPs play a key role in many developmental processes. They are associated mainly with the proliferation, expansion, elongation and differentiation of cells, pollen tube growth, root formation or preventing PCD (Tang et al., 2006; Leszczuk et al., 2019). AGPs have been shown to stimulate both zygotic (Pennell et al., 1991; Paire et al., 2003; Qin et al., 2007) and non-zygotic embryogenesis (Seguí-Simarro et al., 2011; El-Tantawy A. A. et al., 2013; Shu et al., 2014; Duchow et al., 2016; Corral-Martínez et al., 2019). It was assumed that AGPs might be directly involved in ME induction (Seguí-Simarro et al., 2011; Corral-Martínez et al., 2019). Expression of AGPs is strongly affected by stress and their accumulation can be interpreted as plant defense response associated with changes in plasma membrane fluidity and cell–cell communication (Brown et al., 2005; Seifert and Roberts, 2007; Mareri et al., 2018). They also serve as Ca2+ binding molecules (Lamport and Várnai, 2013) and might be involved in the regulation of the specific stages of plant development and adaptation of cells to stress conditions. Since stress is a prerequisite for ME, up-regulated AGP serve as a potential source of signal molecules that are important in the following steps of microspore reprogramming. A high level of Ca2+ is a prerequisite for the formation of a callose-rich sub intimal layer that is associated with the efficiency of somatic and microspore embryogenesis (Rivas-Sendra et al., 2019). An exogenous application of cell-surface-released oligosaccharides has been shown to be an effective stimulus for initiation of somatic embryogenesis (Leljak-Levanić et al., 2015). Several authors have reported an involvement of Ca2+ in the induction of chitinases (Schneider-Müller et al., 1994; Saito et al., 2003; Stressmann et al., 2004) whose possible role in ME was discussed above.
AGP expression and distribution during ME have been studied using monoclonal antibodies (JIM4, JIM8, JIM13, JIM14, JIM16, MAC207, LM2 or LM6) specific for cell wall components (Konieczny et al., 2007; El-Tantawy A. A. et al., 2013; Corral-Martínez et al., 2019; Zieliński et al., 2021). In several studies, AGPs recognized by JIM13, JIM14 or JIM4 were associated with early stages of ME (El-Tantawy A. A. et al., 2013; Corral-Martínez et al., 2019; Zieliński et al., 2021). AGPs recognized by JIM13 and JIM14 were identified as early markers of Brassica ME (El-Tantawy A. A. et al., 2013). Corral-Martínez et al. (2019) and also showed a massive expression of JIM13 epitopes in Brassica embryogenic microspores. It is assumed that high levels of JIM13 epitopes could be related to cell totipotency and embryogenic competence. Moreover, it is outlined that JIM13 epitopes could act as Ca2+ capacitor that serves as source of cytosolic Ca2+. As mentioned, Ca2+ is important for formation of the sub intimal layer, an osmoprotective barrier that enhances the viability of induced microspores (Rivas-Sendra et al., 2019). Zieliński et al. (2021) identified epitopes for JIM4 and JIM13 likely involved in rye ME. Epitopes for JIM4 were previously described in the context of somatic embryogenesis and are crucial for embryo development (Šamaj et al., 1990; Stacey et al., 1990; Chapman et al., 2000). As AGPs are heterogeneous in nature, some authors suggest that AGPs should have more than one specific role (El-Tantawy A. A. et al., 2013). One of the AGP subclasses, Fasciclin-like Arabinogalactan Proteins (FLA) are involved in interaction between cell exterior and the cell surface, acting as cell-adhesion molecules and playing important role during plant development and in response to abiotic stress (Pereira et al., 2016). There are at least 36 FLA in the annotated Hordeum vulgare (HORVU.MOREX.r2.) genome and one of them FASCICLIN-Like Arabinogalactan 2 (HORVU.MOREX.r2.2HG0087710.1) detected in barley transcriptome analysis may be important for embryogenic competence acquisition by microspores induced to ME. Similarly, FLA1 and FLA2 transcripts are described as significant factors in the process of competence acquisition during callus formation and in the induction of shoot development in Arabidopsis (Johnson et al., 2003).
Microspore-to-microspore interactions allow the communication by providing signals protecting cells against stress and triggering ME. In embryogenic microspores of Brassica napus, such signals lead to changes in the cell wall and formation of additional callose-rich and cellulose-deficient sub intimal layers (Parra-Vega et al., 2015; Rivas-Sendra et al., 2019).
Pollen wall is primarily composed of the primexine consisting of the polysaccharide cellulose (Ariizumi and Toriyama, 2011) and sporopollenin, a highly cross-linked polymer (Paxson-Sowders et al., 1997), pectins, xylan, and some AGPs that together form a complex interactive network known as the extracellular matrix (ECM; Li et al., 2017). It covers an inner gametophyte-derived intine layer and an outer sporophyte-derived exine layer upon which a lipid-rich pollen coat is deposited (Ariizumi and Toriyama, 2011; Quilichini et al., 2015). Among ECM proteins there are also enzymes (such as hydrolases, proteases, glycosidases, peroxidases, and esterases), expansins, wall-associated kinases (WAK), and hydroxyproline (Hyp)-rich glycoproteins (Li et al., 2017). Such ECM composition determines not only the biomechanical properties of cell wall, cell adhesion and tissue integrity, but also enables the transmission of external signals to cells (review in Stavolone and Lionetti, 2017). A fibrillar ECM-like structure was also observed on the surface of epidermis of the globular microspore-derived embryos of B. napus at later stages of ME that may regulate the active exchange of information between embryo cells (Dubas et al., 2014a).
The environment via interaction with ECM/cell wall is involved in the modulation of signaling pathways that determine cell fate (Yeats and Rose, 2009). Various secreted peptides are involved in this process, with structures that allow binding of the β-glucosyl Yariv (β-GlcY) and function to facilitate lipid transfer to the cell (Mashiguchi et al., 2004). Among such candidates for the extracellular signaling are Cys rich Lipid-Transfer Proteins (LTP) (Pereira et al., 2016).
The plant LTPs are small (usually below 10 kDa) highly conserved, and soluble extracellular CRPs abundantly expressed in most tissues (Kader, 1996). They possess four or five α-helices, which are stabilized by four conserved disulfide bridges formed by an eight-Cys motif (8 CM). The LTPs are synthesized as pre-proteins with an N-terminal signal peptide that localizes the protein to spaces exterior to the plasma membrane. Several LTPs are involved in a variety of biological processes including growth, reproduction (pollen exine formation) and seed formation (embryo, pericarp, endosperm), adaptations to (a)biotic stress and defense reactions (cutin and suberin deposition) (Kader, 1996; Wang et al., 2005; Chae et al., 2010; review in Salminen et al., 2016). LTPs stabilize membranes and play role in cell wall organization and signal transduction (Liu F. et al., 2015). The importance of LTPs in cell wall loosening, by active phospholipid binding and transferring to membranes, was revealed in tobacco in vitro cultures (Nieuwland et al., 2005). Floral bud and early zygotic embryo-specific genes (VrLTP1.2 and VrLTP1.3) were found to be expressed in mung bean (Liu and Lin, 2003). Some LTPs may be involved in cuticle formation in developing embryos (Sterk et al., 1991). In Arabidopsis, non-specific lipid transfer protein 1 (AtLPT1), rich in eight Cys residues is specifically expressed and binds calmodulin in a Ca2+-independent manner (Wang et al., 2005). The expression of another AtLTPd9 (END1) gene in dividing nuclei, endosperm nodules and in the developing embryos at the globular stage in Arabidopsis seems to be more specific for embryogenesis (Li M. et al., 2014). The occurrence of LTP1 epitopes in A. thaliana explants differentiated embryogenic from non-embryogenic cells where somatic embryos developed (Potocka et al., 2012). Some indirect data support the possibility that LTPs also play a role in ME initiation in barley (Vrinten et al., 1999 and our study). Transcriptomic experiments have revealed that LTPs associated with embryogenic potential acquisition by barley microspores. The amount of LTPs transcripts appears to be correlated with the growth of microspores achieved by turgor-driven expansion and limited by the extensibility of the sporoderm [a complex extracellular matrix with an intine and an exine layer upon which a lipid-rich coat (tryphine) is deposited; Quilichini et al., 2015].
At later stages of ME initiation, when microspores intensively divide and form embryogenic structures that are able to regenerate green plants, PTMPs, such as PSK and GRP seems to play important roles (Chen et al., 2010; Soriano et al., 2013, 2014; Asif et al., 2014).
PSK [Tyr(SO3H)-Ile-Tyr(SO3H)-Thr-Gln], a disulfated pentapeptide seems to be an interesting molecule. It controls microspore proliferation and differentiation what results in ELS development. PSK increases cytosolic Ca2+ and activates auxin-mediated pathways that enhance resistance and promote cell growth and proliferation (Hanai et al., 2000). A previous study revealed that exogenous PSK-α promoted not only cell division cycle and cell growth but also helped quiescent microspores arrested in G1 stage to re-enter the cell cycle to the S-phase and mitosis (Eun et al., 2003). PSK used as culture medium-supplement in in vitro systems stimulated somatic embryogenesis in Daucus carota and Cryptomeria japonica (Kobayashi et al., 1999; Hanai et al., 2000; Igasaki et al., 2003), cell divisions and regeneration of Brassica oleracea protoplasts (Kiełkowska and Adamus, 2019) and ME in rice, triticale and wheat isolated microspore cultures (Chen et al., 2010; Asif et al., 2014). In the case of ME, supplement of 10−7 M PSK-α promoted production of ELS and green plants regeneration, also in the absence of nursing ovaries (Asif et al., 2014). It also reduced the frequency of albino plant formation but only in wheat regenerants, what is in accordance with earlier reported PSK ability for enhancing chlorophyll synthesis in seedlings of cucumber and Arabidopsis (Yamakawa et al., 1998, 1999).
Because only multicellular and compact ELSs with well-developed protoderm are capable to radial and polar histo-differentiation into all cell types like zygotic embryos produced in planta (Telmer et al., 1995; Yeung et al., 1996; Ilić-Grubor et al., 1998), GRPs could be considered as a good marker for prediction of microspore derived-embryos to develop successfully (Soriano et al., 2013, 2014).
Glycine-rich proteins (GRP) classes I and II are secreted and localized in the extracellular space. These GRP classes contain N-terminal signal peptides followed by glycine-or cysteine-rich regions. Notably, such signal peptide in class I GRP is followed by a region extremely rich in glycine and containing (GGX)n repeats. In class II, two important regions are present, the first with [GG(X)3GG]n glycine-rich repeats and the second with a specific cysteine-rich motif at the C-terminus (Czolpinska and Rurek, 2018). GRPs are known to participate in post-transcriptional regulation of gene expression (Kim et al., 2005). Emerging evidence suggests that class I and II GRP members are crucial for the regulation of plant cell and organ growth. Moreover, they are also active components of the plant cell wall. Park et al. (2001) reported that class II of GRPs interacts with cell wall-associated kinases, thereby initiating the recognition of environmental stimuli and participating in signal transduction. The expression of GRP genes is modulated by stress (Czolpinska and Rurek, 2018) in the key developmental stages (e.g., pollen and embryo development), however, most of them are expressed at low levels in pollen in comparison to other tissues. Soriano et al. (2014) suggested that GRP expression is a suitable marker for determining the embryo cell fate in ME. Although many microspores are initially programmed to develop as ELS, only ~0.5% of the sporophytic structures will eventually produce the compact structures that form histo-differentiated embryos (Li H. et al., 2014), suggesting that additional signaling events are required to ensure further ELS growth and differentiation. An initial auxin response seems to be the most important signaling pathway for microspores initially programmed as an embryo proper, as it was marked by DR5 expression in B. napus (Dubas et al., 2014b). Interestingly, the failure of certain GRP-marked embryogenic structures (i.e., asymmetrically divided microspores and callus-like cells) to establish an auxin response suggests that these structures are associated with a different pathway of haploid embryo development in which the formation of an embryo proper is not initiated or is delayed. GRP expression marks the basal pole of the embryo and the future columella at the site, where the pollen wall remnants are present (Soriano et al., 2014).
Importantly, as described in this paper, various proteins and peptides with their signaling properties influence microspores fate under in vitro conditions (Figure 1), but it is not known if other factors are equally required for ME initiation. To validate the involvement of these molecules, application of modern biotechnological technologies using synthetic peptides is a future perspective.
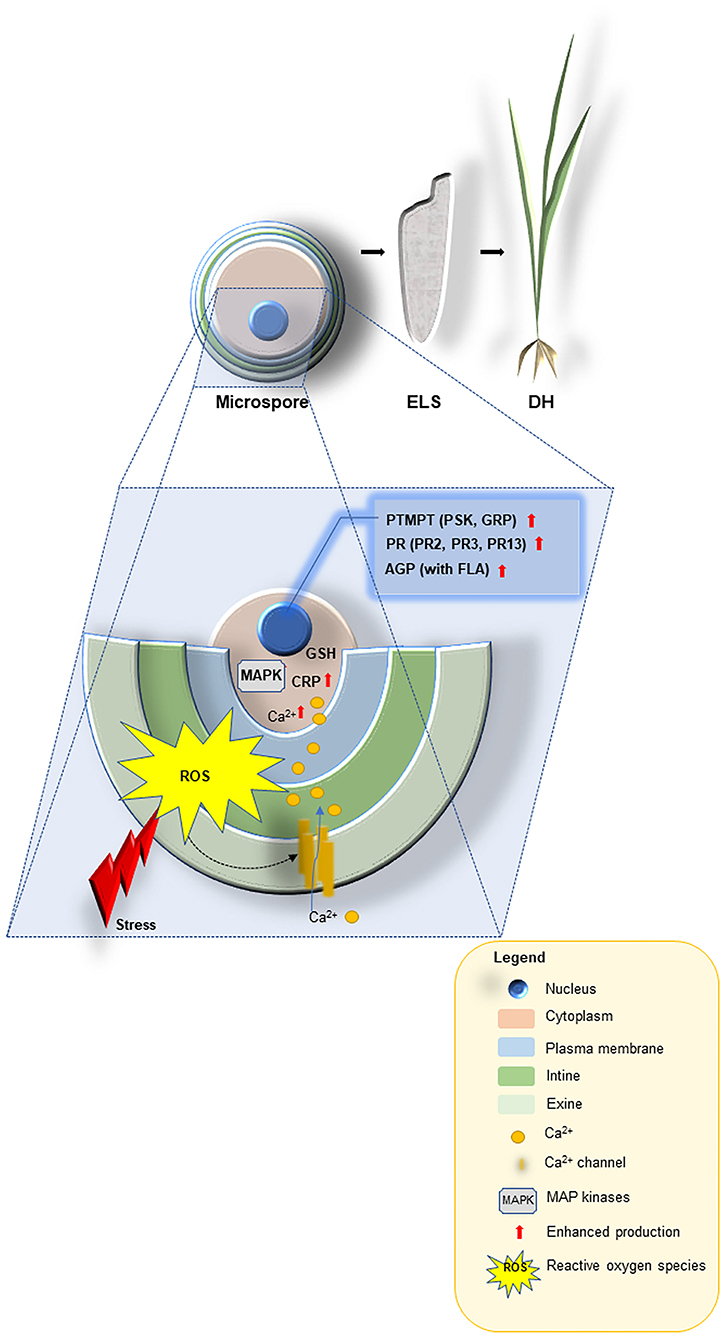
Figure 1. A schematic model describing the proposed functions for stress-related proteins, cysteine-rich tripeptide glutathione, other cysteine-rich and the small post-translationally modified peptides (PTMPs) in microspore embryogenesis signaling and their use as promising tools for improving doubled-haploid (DH) production in crop plants. Abiotic stresses are perceived at the microspore surface (exine, intine and plasma membrane), and linked to cellular messengers such as ROS, Ca2+, cysteine-rich peptides [CRP e.g., rapid alkalinization factor (RALF), lipid transfer (LTP) and MAP kinases] to render the signals through glutathione (GSH) into a large-scale transcriptional reprogramming that ultimately leads to enhanced production of the most appropriate defense responses: the small PTMPs [e.g., phytosulfokine (PSK), glycine-reach proteins (GRP)], pathogenesis-related proteins (PR2, PR3, PR13) and arabinogalactan proteins AGP [with fasciclin-like arabinogalactan proteins (FLA)] in order to induce microspore-derived embryo-like structures (ELS) able to regenerated green DH lines.
Synthetic Cysteine-Rich Peptides
Modern approaches to construct synthetic peptides and their derivatives with diversified structures have accelerated current research and development of DNA-free genome editing used in DH technology improvement. Functional studies must be complete with suitable knock-out lines. These would greatly profit from targeted genome modification technology (so-called genome-editing), such as zinc finger nucleases (ZFN), transcriptional activator-effector nucleases (TALEN) and CRISPR-related endonuclease Cas9 (CRISPR/Cas9). ZNF, TALEN, CRISPR/Cas9 allow specific mutations in the critical coding regions of the peptides (Yamaguchi et al., 2013; Razzaq et al., 2019) and coupled with DH technology and traditional breeding of crop plants with modified antioxidant apparatus will contribute to guarantee safe food production in the next decades.
Recently Bilichak et al. (2020), reported nucleic acid-free genome editing in wheat microspores and haploid embryos mediated by an alternative ZFN delivery of proteins complexed with Cys-rich cell-penetrating peptides (CPP) used as vector molecules through a receptor-independent pathway without inducing toxicity. For this, ZFN-R monomer was complexed with synthetic peptide with an activated cys(Npys)-(D-R)9 CPP [a Cys residue added to their N-terminus with a 3-nitro-2-pyridinesulfenyl (Npys) group on the thiol group] to facilitate formation of (D-R)9-ZFN through asymmetrical disulphide bond that can be dissociated under reducing conditions inside a cell (Liu et al., 2014). The final conjugate effectively delivered the target peptide into cells and then released the therapeutics such as siRNA or some target peptide in the reducing environment. This conjugation made this peptide more cell-permeable including cells of microspore-derived embryos (ELS). The valuation of ZFN cleavage activity in ELS either regenerated from transfected microspores or directly treated with CPP-ZFN complexes revealed that the level of indels at the target region is higher for the sub genome A as compared to the B sub genome. Although, the developed method eliminates many concerns associated with similar nucleic acid-driven technologies, CRISPR/Cas system and its variations are more popular worldwide and has more genome editing efficiency than TALEN, ZFN and other sequence-specific nucleases (SSNs) and play a crucial role to fine-tune genomics-assisted breeding efforts (Gao, 2018).
Future Perspectives
Our knowledge of the physiological responses of crop plants to abiotic stresses has significantly improved with the ongoing development of techniques including in vitro and biotechnology systems (Rai et al., 2011; Pérez-Clemente and Gómez-Cadenas, 2012; Maleki et al., 2019). The increasing availability of the sequenced genomes of crop species: the hexaploid wheat (International Wheat Genome Sequencing Consortium, 2014; https://urgi.versailles.inra.fr; Chapman et al., 2015; Monat et al., 2019), barley (BARLEX; Beier et al., 2017; Mascher et al., 2017; Sato, 2020), maize (Schnable et al., 2009), rice (Li J.-Y. et al., 2014), Brassica rapa (Zhang et al., 2018) in combination with advances whole genome shotgun sequencing techniques (WGS), allow in-depth molecular studies of non-model species (Rabanus-Wallace et al., 2021; e.g., in rye Li et al., 2021). The information found in genomic databases provides a powerful tool to identify markers (including genes coding small peptides) for desired traits for breeders and to advance our knowledge of the effects of potential modulations (including genome-editing) of synthetic pathways that underlies abiotic stress responses in crops. Certainly, more detailed examination is necessary to understand its role and identify the mechanism of action.
For these purposes, the most promising among identified peptides acting as growth regulators alleviating stresses and altering plant development, can be synthesized and tested on plants, with goals of extending and strengthening responsiveness to ME in DH technology. This novel solution, by using native small synthetic peptides applied as microspore coatings, will help us to increase new germplasm in parallel with saving time and costs.
Author Contributions
All authors listed have made a substantial, direct and intellectual contribution to the work, and approved it for publication.
Funding
mRNA seq supported by the National Science Center, Poland, grant number 2015/18/M/NZ3/00348.
Conflict of Interest
The authors declare that the research was conducted in the absence of any commercial or financial relationships that could be construed as a potential conflict of interest.
Publisher's Note
All claims expressed in this article are solely those of the authors and do not necessarily represent those of their affiliated organizations, or those of the publisher, the editors and the reviewers. Any product that may be evaluated in this article, or claim that may be made by its manufacturer, is not guaranteed or endorsed by the publisher.
Abbreviations
BSO, buthionine sulfoximine the inhibitor of GSH synthesis; CAT, catalase; CHIT1, CHIT2, endochitinases; CRISPR/Cas9, CRISPR-related endonuclease Cas9; CRP, cysteine-rich peptides; Cys, cysteine; DH, doubled haploid; DNMT, DNA methyltransferases; ECA, arabinogalactan-like proteins; ECM, extracellular matrix; ELS, embryo-like structures; EXP, expansins; EXT, extensins; FLA, fasciclin-like arabinogalactan proteins; GlcNAc, N-acetyl glucosamine; Glu, glutamine; Gly, glycine; GPX, glutathione peroxidase; GRP, glycine-reach proteins; GSH, reduced form of glutathione; GSSG, oxidized form of glutathione; GST, glutathione S-transferase; H2O2, hydrogen peroxide; HECATE, transcription factor; HMT, histone methyltransferases; LT, low temperature; LTP, lipid transfer proteins; MAN, mannitol; MAP, mitogen-activated protein kinases; MAS, marker assisted selection; ME, microspore embryogenesis; MYB5, transcription factor; MYB75, transcription factor; NGGS, genome sequencing; 1O2, singlet oxygen; O2·–, superoxide anion; OH·, hydroxyl radical; OTC, L-2-oxothiazolidine-4-carboxylic acid precursor of GSH; PCD, plant cell death; PR, Pathogenesis-related proteins; PR12, γ-thionins, the family of PR proteins; PR13, defensins, the family of PR proteins; PR2, β-1,3-glucanases, the family of PR proteins; PR3, chitinases, the family of PR proteins; PSK, phytosulfokine; PTMP, small post-translationally modified peptides; PTMs, post-translational modifications; RALF, rapid alkalinization factor; ROS, reactive oxygen species; SAM, S-adenosyl-methionine; S-GlcNAc, acylated S-linked N-acetyl glucosamine; SOD, superoxide dismutase; SPATULA, transcription factor; SSNs, sequence-specific nucleases; TALEN, transcriptional activator-effector nucleases; TFs, transcription factors; TrX, thioredoxin; TSA, trichostatin A the histone deacetylase inhibitor; WAK, wall-associated kinases; WGS, shotgun sequencing techniques; WRKY, the zinc-finger transcription factors; ZFN, zinc finger nucleases.
References
AbdElgawad, H., Zinta, G., Hegab, M. M., Pandey, R., Asard, H., et al. (2016). High salinity induces different oxidative stress and antioxidant responses in maize seedlings organs. Front. Plant Sci.7:276. doi: 10.3389/fpls.2016.00276
Albert, M. (2013). Peptides as triggers of plant defence. J. Exp. Bot. 64, 5269–5279. doi: 10.1093/jxb/ert275
Ariizumi, T., and Toriyama, K. (2011). Genetic regulation of sporopollenin synthesis and pollen exine development. Annu. Rev. Plant Biol. 62, 437–460. doi: 10.1146/annurev-arplant-042809-112312
Asif, M., Eudes, F., Goyal, A., Amundsen, E., Randhawa, H., and Spaner, D. (2013). Organelle antioxidants improve microspore embryogenesis in wheat and triticale. In Vitro Cell. Dev. Biol. Plant 49, 489–497 doi: 10.1007/s11627-013-9514-z
Asif, M., Eudes, F., Randhawa, H., Amundsen, E., and Spaner, D. (2014). Phytosulfokine alpha enhances microspore embryogenesis in both triticale and wheat. Plant Cell Tissue Organ Cult. 116, 125–130 doi: 10.1007/s11240-013-0379-y
Barakat, M. N., Al-Doss, A. A., Ghazy, A. I., Moustafa, K. A., Elshafei, A. A., and Ahmed, E. I. (2017). Doubled haploid wheat lines with high molecular weight glutenin alleles derived from microspore culture. N. Z. J. Crop Hort. Sci. 46, 198–211. doi: 10.1080/01140671.2017.1368674
Beier, S., Himmelbach, A., Colmsee, C., Zhang, X.-Q., Barrero, R. A., Zhang, Q., et al. (2017). Construction of a map-based reference genome sequence for barley, Hordeum vulgare L. Sci. Data 4:170044. doi: 10.1038/sdata.2017.44
Bélanger, S., Baldrich, P., Lemay, M.-A., Marchand, S., Esteves, P., Meyers, B. C., et al. (2020). The commitment of barley microspores into embryogenesis correlates with miRNA-directed regulation of members of the SPL, GRF and HD-ZIPIII transcription factor families. Plant Direct 4, 1–15. doi: 10.1101/2020.06.11.146647
Bélanger, S., Marchand, S., Jacques, P.-É., Meyers, B., and Belzile, F. (2018). Differential expression profiling of microspores during the early stages of isolated microspore culture using the responsive barley cultivar gobernadora. G3-Genes Genom. Genet. 8, 1603-1614. doi: 10.1534/g3.118.200208
Belmonte, M. F., Ambrose, S. J., Ross, A. R. S., Abrams, S. R., and Stasolla, C. (2006). Improved development of microspore-derived embryo cultures of Brassica napus cv Topaz following changes in glutathione metabolism. Physiol. Plant 127, 690–700. doi: 10.1111/j.1399-3054.2006.00707.x
Berenguer, E., Bárány, I., Solís, M.-T., Pérez-Pérez, Y., Risueño, M. C., and Testillano, P. S. (2017). Inhibition of histone H3K9 methylation by BIX-01294 promotes stress-induced microspore totipotency and enhances embryogenesis initiation. Front. Plant Sci. 8:1161. doi: 10.3389/fpls.2017.01161
Bilichak, A., Sastry-Dent, L., Sriram, S., Simpson, M., Samuel, P., Webb, S., et al. (2020). Genome editing in wheat microspores and haploid embryos mediated by delivery of ZFN proteins and cell-penetrating peptide complexes. Plant Biotechnol. J. 18, 1307-1316. doi: 10.1111/pbi.13296
Bircheneder, S., and Dresselhaus, T. (2016). Why cellular communication during plant reproduction is particularly mediated by CRP signalling. J. Exp. Bot. 67, 4849–4861. doi: 10.1093/jxb/erw271
Blackburn, M. R., Haruta, M., and Moura, D. S. (2020). 20 years of progress in physiological and biochemical investigation of RALF peptides. Plant Physiol. 182, 1657–1666. doi: 10.1104/pp.19.01310
Borderies, G., le Bechec, M., Rossignol, M., Lafitte, C., Le Deunff, E., Beckert, M., et al. (2004). Characterization of proteins secreted during maize microspore culture: Arabinogalactan proteins (AGPs) stimulate embryo development. Eur. J. Cell Biol. 83, 205–212. doi: 10.1078/0171-9335-00378
Bowsher, C. G., and Tobin, A. K. (2001). Compartmentation of metabolism within mitochondria and plastids. J. Exp. Bot. 52, 513–527. doi: 10.1093/jexbot/52.356.513
Brown, D. M., Zeef, L. A. H., Ellis, J., Goodacre, R., and Turner, S. (2005). Identification of novel genes in Arabidopsis involved in secondary cell wall formation using expression profiling and reverse genetics. Plant Cell 17, 2281–2295. doi: 10.1105/tpc.105.031542
Calicioglu, O., Flammini, A., Bracco, S., Bellù, L., and Sims, R. (2019). The future challenges of food and agriculture: an integrated analysis of trends and solutions. Sustainability 11:222. doi: 10.3390/su11010222
Carvalho, A. O., and Gomes, V. M. (2009). Plant defensins-prospects for the biological functions and biotechnological properties. Peptides 30, 1007–1020. doi: 10.1016/j.peptides.2009.01.018
Carvalho, A. O., and Gomes, V. M. (2011). Plant defensins and defensin-like peptides—biological activities and biotechnological applications. Curr. Pharm. Design 17, 4270-4293. doi: 10.2174/138161211798999447
Castillo, A. M., Cistue, L., Romagosa, I., and Valles, M. (2001). Low responsiveness of six-rowed genotypes to androgenesis in barley does not have a pleiotropic basis. GENOME 44, 936–940. doi: 10.1139/g01-034
Castillo, A. M., Valero-Rubira, I., Burrell, M. Á., Allué, S., Costar, M. A., and Vallés, M. P. (2020). Trichostatin A affects developmental reprogramming of bread wheat microspores toward an embryogenic route. Plants (Basel) 9:1442. doi: 10.3390/plants9111442
Chae, K., Gonong, B. J., Kim, S.-C., Kieslich, C. A., Morikis, D., Balasubramanian, S., et al. (2010). A multifaceted study of stigma/style cysteine-rich adhesin (SCA)-like Arabidopsis lipid transfer proteins (LTPs) suggests diversified roles for these LTPs in plant growth and reproduction. J. Exp. Bot. 61, 4277–4290. doi: 10.1093/jxb/erq228
Chan, Y. L., Prasad, V., Sanjaya, Chen,. K. H., Liu, P., Chan, M., and Cheng, C.-P. (2005). Transgenic tomato plants expressing an Arabidopsis thionin (Thi2.1) driven by fruit-inactive promoter battle against phytopathogenic attack. Planta 221, 386–393. doi: 10.1007/s00425-004-1459-3
Chapman, A., Blervacq, A. S., Vasseur, J., and Hilbert, J. L. (2000). Arabinogalactan-proteins in Cichorium somatic embryogenesis: effect of β-glucosyl Yariv reagent and epitope localisation during embryo development. Planta 211, 305–314. doi: 10.1007/s004250000299
Chapman, J. A., Mascher, M., Buluc, A., Barry, K., Georganas, E., Session, A., et al. (2015). A whole-genome shotgun approach for assembling and anchoring the hexaploid bread wheat genome. Genome Biol. 16:26. doi: 10.1186/s13059-015-0582-8
Chauhan, H., and Khurana, P. (2011). Use of haploid technology for development of stable drought tolerant bread wheat (Triticum aestivum L.) transgenics. Plant Biotech. J. 9, 408–417. doi: 10.1111/j.1467-7652.2010.00561.x
Chen, D., Li, S., Xiang, Y., Zhang, Z., and Peng, Y. (2010). Preliminary studies on the effects of plant phytosulfokine PSK-α in rice anther culture. Southwest China J. Agric. Sci. 23, 1447–1450.
Chen, X. Y., and Kim, J. Y. (2009). Callose synthesis in higher plants. Plant Signal. Behav. 4, 489–492. doi: 10.4161/psb.4.6.8359
Chen, X. Y., Liu, L., Lee, E., Han, X., Rim, Y., Kim, S.-W., et al. (2009). The Arabidopsis callose synthase gene GSL8 is required for cytokinesis and cell patterning. Plant Physiol. 150, 105–13. doi: 10.1104/pp.108.133918
Chinnusamy, V., and Zhu, J.-K. (2009). Epigenetic regulation of stress responses in plants. Curr. Opin. Plant Biol. 12, 133–139. doi: 10.1016/j.pbi.2008.12.006
Chiu, J., and Dawes, I. W. (2012). Redox control of cell proliferation. Trends Cell Biol. 22, 592–601. doi: 10.1016/j.tcb.2012.08.002
Chmielowska-Bak, J., Izbianska, K., and Deckert, J. (2015). Products of lipid, protein and RNA oxidation as signals and regulators of gene expression in plants. Front. Plant Sci. 6:405. doi: 10.3389/fpls.2015.00405
Cistué, L., Batlle, R. F., and Echávarri, B. (2009). Improvements in the production of doubled haploids in durum wheat (Triticum turgidum L.) through isolated microspore culture. Plant Cell Rep. 28, 727–735. doi: 10.1007/s00299-009-0690-6
Corral-Martínez, P., Driouich, A., and Seguí-Simarro, J. M. (2019). Dynamic changes in arabinogalactan-protein, pectin, xyloglucan and xylan composition of the cell wall during microspore embryogenesis in Brassica napus. Front. Plant Sci. 10:332. doi: 10.3389/fpls.2019.00332
Corral-Martínez, P., Siemons, C., Horstman, A., Angenent, G. C., de Ruijter, N., and Boutilier, K. (2020). Live Imaging of embryogenic structures in Brassica napus microspore embryo cultures highlights the developmental plasticity of induced totipotent cells. Plant Reprod. 33, 143–158. doi: 10.1007/s00497-020-00391-z
Cosgrove, D. J. (2000). Loosening of plant cell walls by expansins. Nature 407, 321-326. doi: 10.1038/35030000
Coutos-Thevenot, P., Maes, O., Jouenne, T., Claude Mauro, M., Boulay, M., Deloire, A., et al. (1992). Extracellular protein patterns of grapevine cell suspensions in embryogenic and non-embryogenic situations. Plant Sci. 86, 137–145. doi: 10.1016/0168-9452(92)90159-J
Covey, P. A., Subbaiah, C. C., Parsons, R. L., Pearce, G., Lay, F. T., Anderson, M. A., et al. (2010). A pollen-specific RALF from tomato that regulates pollen tube elongation. Plant Physiol. 153, 703–715. doi: 10.1104/pp.110.155457
Czolpinska, M., and Rurek, M. (2018). Plant glycine-rich proteins in stress response: an emerging, still prospective story. Front. Plant Sci. 9:302. doi: 10.3389/fpls.2018.00302
Czyzewicz, N., Yue, K., Beeckman, T., and De Smet, I. (2013). Message in a bottle: small signalling peptide outputs during growth and development. J. Exp. Bot. 64, 5281–5296. doi: 10.1093/jxb/ert283
De Coninck, B., Cammue, B. P. A., and Thevissen, K. (2013). Modes of antifungal action and in planta functions of plant defensins and defensin-like peptides. Fungal Biol. Rev. 26, 109–120. doi: 10.1016/j.fbr.2012.10.002
De Coninck, B., and De Smet, I. (2016). Plant peptides–taking them to the next level. J. Exp. Bot. 67, 4791–4795. doi: 10.1093/jxb/erw309
De Leon, C. A., Levine, P. M., Craven, T. W., and Pratt, M. R. (2017). The sulfur-linked analogue of O-GlcNAc (S-GlcNAc) is an enzymatically stable and reasonable structural surrogate for O-GlcNAc at the peptide and protein levels. Biochemistry 56, 3507–3517. doi: 10.1021/acs.biochem.7b00268
De Vries, S. C., Booij, H., Meyerink, P., Huisman, G., Wilde, H. D., Thomas, T. L., et al. (1988). Acquisition of embryogenic potential in carrot cell-suspension cultures. Planta 176, 196–204. doi: 10.1007/BF00392445
Deponte, M. (2013). Glutathione catalysis and the reaction mechanisms of glutathione-dependent enzymes. Biochim. Biophys. Acta 1830, 3217–3266. doi: 10.1016/j.bbagen.2012.09.018
Diaz Vivancos, P., Dong, Y., Ziegler, K., Markovic, J., Pallardo, F. V., Pellny, T. K., et al. (2010a). Recruitment of glutathione into the nucleus during cell proliferation adjusts whole-cell redox homeostasis in Arabidopsis thaliana and lowers the oxidative defence shield. Plant J. 64, 825–838. doi: 10.1111/j.1365-313X.2010.04371.x
Diaz Vivancos, P., Wolff, T., Markovic, J., Pallardo, F. V., and Foyer, C. H. (2010b). A nuclear glutathione cycle within the cell cycle. Biochem. J. 431, 169–178. doi: 10.1042/BJ20100409
Dirks, R., van Dun, K., de Snoo, C. B., van den Berg, M., Lelivelt, C. L. C., Voermans, W., et al. (2009). Reverse breeding: a novel breeding approach based on engineered meiosis. Plant Biotechnol. J. 7, 837–845. doi: 10.1111/j.1467-7652.2009.00450.x
Dixon, D. P., and Edwards, R. (2009). Selective binding of glutathione conjugates of fatty acid derivatives by plant glutathione transferases. J. Biol. Chem. 284, 21249–21256. doi: 10.1074/jbc.M109.020107
Dixon, D. P., Lapthorn, A., and Edwards, R. (2002). Plant glutathione transferases. Genome Biol. 3:REVIEWS3004. doi: 10.1186/gb-2002-3-3-reviews3004
Doxey, A. C., Yaish, M. W. F., Moffatt, B. A., Griffith, M., and McConkey, B. J. (2007). Functional divergence in the Arabidopsis β-1,3-glucanase gene family inferred by phylogenetic reconstruction of expression states. Mol. Biol. Evol. 24, 1045–1055. doi: 10.1093/molbev/msm024
Dubas, E., Custers, J., Kieft, H., Wedzony, M., and van Lammeren, A. A. M. (2014a). Characterization of polarity development through 2-and 3-D imaging during the initial phase of microspore embryogenesis in Brassica napus L. Protoplasma 251, 103–113. doi: 10.1007/s00709-013-0530-y
Dubas, E., Janowiak, F., Krzewska, M., Hura, T., and Zur, I. (2013). Endogenous ABA concentration and cytoplasmic membrane fluidity in microspores of oilseed rape (Brassica napus L.) genotypes differing in responsiveness to androgenesis induction. Plant Cell Rep. 32, 1465–1475. doi: 10.1007/s00299-013-1458-6
Dubas, E., Moravčíková, J., Libantova, J., Matusikova, I., Benkova, E., Zur, I., et al. (2014b). The influence of heat stress on auxin distribution in transgenic B. napus microspores and microspore-derived embryos. Protoplasma 251, 1077–1087. doi: 10.1007/s00709-014-0616-1
Duchow, S., Dahlke, R. I., Geske, T., Blaschek, W., and Classen, B. (2016). Arabinogalactan-proteins stimulate somatic embryogenesis and plant propagation of Pelargonium sidoides. Carbohydr. Polym. 152, 149–155. doi: 10.1016/j.carbpol.2016.07.015
Dünser, K., Gupta, S., Herger, A., Feraru, M. I., Ringli, C., and Kleine-Vehn, J. (2019). Extracellular matrix sensing by FERONIA and leucine-rich repeat extensins controls vacuolar expansion during cellular elongation in Arabidopsis thaliana. EMBO J. 38:e100353. doi: 10.15252/embj.2018100353
Dunwell, J. M. (2010). Haploids in flowering plants: origins and exploitation. Plant Biotechnol. J. 8, 377–424 doi: 10.1111/j.1467-7652.2009.00498.x
Dwivedi, S. L., Britt, A. B., Tripathi, L., Sharma, S., Upadhyaya, H. D., and Ortiz, R. (2015). Haploids: constraints and opportunities in plant breeding. Biotechnol. Adv. 33, 812–829. doi: 10.1016/j.biotechadv.2015.07.001
Edwards, E. A., Rawsthorne, S., and Mullineaux, P. M. (1990). Subcellular distribution of multiple forms of glutathione reductase in leaves of pea (Pisum sativum L.). Planta 180, 278–284. doi: 10.1007/BF00194008
Elhiti, M., Stasolla, C., and Wang, A. (2013). Molecular regulation of plant somatic embryogenesis. In Vitro Cell. Dev. Biol. Plant 49, 631–642. doi: 10.1007/s11627-013-9547-3
El-Tantawy, A.-A., Solis, M.-T., Risueno, M. C., and Testillano, P. S. (2014). Changes in DNA methylation levels and nuclear distribution patterns after microspore reprogramming to embryogenesis in barley. Cytogenet. Genome Res. 143, 200–208. doi: 10.1159/000365232
El-Tantawy, A. A., Solís, M. T., Da Costa, M. L., Coimbra, S., Risueño, M. C., and Testillano, P. S. (2013). Arabinogalactan protein profiles and distribution patterns during microspore embryogenesis and pollen development in Brassica napus. Plant Reprod. 26, 231–243. doi: 10.1007/s00497-013-0217-8
Eun, C.-H., Ko, S.-M., Higashi, K., Yeo, D., Matsubayashi, Y., Sakagami, Y., et al. (2003). Phytosulfokine-alpha; a peptidyl plant growth factor, stimulates cell cycle progression in carrot non-embryogenic cells. Plant Biotechnol. 20, 195–205. doi: 10.5511/plantbiotechnology.20.195
Ferreira, R. B., Monteiro, S., Freitas, R., Santos, C. N., Chen, Z., Batista, L. M., et al. (2007). The role of plant defence proteins in fungal pathogenesis. Mol. Plant Pathol. 8, 677–700. doi: 10.1111/j.1364-3703.2007.00419.x
Ferrie, A. M. R., and Möllers, C. (2011). Haploids and doubled haploids in Brassica spp. for genetic and genomic research. Plant Cell Tissue Organ Cult. 104, 375–386. doi: 10.1007/s11240-010-9831-4
Fesel, P. H., and Zuccaro, A. (2016). β-glucan: crucial component of the fungal cell wall and elusive MAMP in plants. Fungal Gen. Biol. 90, 53–60. doi: 10.1016/j.fgb.2015.12.004
Flora, S. J. S. (2009). Structural, chemical and biological aspects of antioxidants for strategies against metal and metalloid exposure. Oxid. Med. Cellular Longev. 2, 191–206. doi: 10.4161/oxim.2.4.9112
Forster, B. P., Heberle-Bors, E., Kasha, K. J., and Touraev, A. (2007). The resurgence of haploids in higher plants. Trends Plant Sci. 12, 368–375. doi: 10.1016/j.tplants.2007.06.007
Foyer, C. H., and Noctor, G. (2003). Redox sensing and signaling associated with reactive oxygen in chloroplasts, peroxisomes and mitochondria. Physiologia Plant. 119, 355–364. doi: 10.1034/j.1399-3054.2003.00223.x
Foyer, C. H., and Noctor, G. (2005). Redox homeostasis and antioxidant signaling: a metabolic interface between stress perception and physiological responses. Plant Cell 17, 1866–1875. doi: 10.1105/tpc.105.033589
Foyer, C. H., and Rennenberg, H. (2000). “Regulation of glutathione synthesis and its role in abiotic and biotic stress defence,” in Sulfur Nutrition and Sulfur Assimilation in Higher Plants, eds C. Brunold, H. Rennenberg, L. J. De Kok, I. Stulen, and J. C. Davidian (Bern: Paul Haupt), 127–153.
Foyer, C. H., Theodoulou, F. L., and Delrot, S. (2001). The functions of inter-and intracellular glutathione transport systems in plants. Trends Plant Sci. 6, 486–492. doi: 10.1016/S1360-1385(01)02086-6
Fráterová, L., Salaj, T., Matušíková, I., and Salaj, J. (2013). The role of chitinases and glucanases in somatic embryogenesis of black pine and hybrid firs. Open Life Sci. 8, 1172–1182. doi: 10.2478/s11535-013-0234-5
Gao, C. (2018). The future of CRISPR technologies in agriculture. Nat. Rev. Mol. Cell Biol. 19, 275–276. doi: 10.1038/nrm.2018.2
García-Giménez, J. L., Olaso, G., Hake, S. B., Boenisch, C., Wiedemann, S. M., Markovic, J., et al. (2013). Histone H3 glutathionylation in proliferating mammalian cells destabilizes nucleosomal structure. Antioxid. Redox Signal. 19, 1305–1320. doi: 10.1089/ars.2012.5021
García-Giménez, J. L., and Pallardo, F. V. (2014). Maintenance of glutathione levels and its importance in epigenetic regulation. Front. Pharmacol. 5:88. doi: 10.3389/fphar.2014.00088
García-Giménez, J. L., Roma-Mateo, C., Perez-Machado, G., Peiro-Chova, L., and Pallardo, F. V. (2017). Role of glutathione in the regulation of epigenetic mechanisms in disease. Free Radic. Biol. Med. 112, 36–48. doi: 10.1016/j.freeradbiomed.2017.07.008
García-Quirós, E., Carmona, R., Zafra, A., Claros, M. G., and de Dios Alché, J. (2017). “Identification and in silico analysis of glutathione reductase transcripts expressed in Olive (Olea europaea L.) pollen and pistil,” in Proceedings of the 5th International Work-Conference, IWBBIO, eds I. Rojas, and F. Ortuño (Granada), 185–195. doi: 10.1007/978-3-319-56154-7_18
Germanà, M. A. (2006). Doubled haploid production in fruit crops. Plant Cell Tissue Organ Cult. 86, 131–146. doi: 10.1007/s11240-006-9088-0
Germanà, M. A. (2011). Gametic embryogenesis and haploid technology as valuable support to plant breeding. Plant Cell Rep. 30, 839–857. doi: 10.1007/s00299-011-1061-7
Gregorová, Z., Kováčik, J., Klejdus, B., Maglovski, M., Kuna, R., Hauptvogel, P., et al. (2015). Drought-induced responses of physiology, metabolites, and PR proteins in Triticum aestivum. J. Agric. Food Chem. 63, 8125–8133. doi: 10.1021/acs.jafc.5b02951
Grover, A. (2012). Plant chitinases: genetic diversity and physiological roles. Crit. Rev. Plant Sci.31, 57–73. doi: 10.1080/07352689.2011.616043
Guan, Y., Meng, X., Khanna, R., LaMontagne, E., Liu, Y., and Zhang, S. (2014). Phosphorylation of a WRKY transcription factor by MAPKs is required for pollen development and function in Arabidopsis. PLoS Genet. 10:e1004384. doi: 10.1371/journal.pgen.1004384
Guerreiro, J. R., Pearce, G., Silva-Filho, M. C., and Moura, D. S. (2013). RALF peptides, in Handbook of Biologically Active Peptides, 2nd Edn, ed A. J. Kastin (San Diego: Academic Press), 46–49. doi: 10.1016/B978-0-12-385095-9.00009-9
Guha, S., and Maheshwari, S. C. (1964). In vitro production of embryos from anthers of Datura. Nature 204:497. doi: 10.1038/204497a0
Hanai, H., Matsuno, T., Yamamoto, M., Matsubayashi, Y., Kobayashi, T., Kamada, H., et al. (2000). A secreted peptide growth factor, phytosulfokine, acting as a stimulatory factor of carrot somatic embryo formation. Plant Cell Physiol. 41, 27–32. doi: 10.1093/pcp/41.1.27
Hara, K., Kajita, R., Torii, K. U., Bergmann, D. C., and Kakimoto, T. (2007). The secretory peptide gene EPF1 enforces the stomatal one-cell-spacing rule. Genes Dev. 21, 1720–1725. doi: 10.1101/gad.1550707
Hartmann, T. N., Fricker, M. D., Rennenberg, H., and Meyer, A. J. (2003). Cell-specific measurement of cytosolic glutathione in poplar leaves. Plant Cell Environ. 26, 965–975. doi: 10.1046/j.1365-3040.2003.01031.x
He, H., Van Breusegem, F., and Mhamdi, A. (2018). Redox-dependent control of nuclear transcription in plants. J. Exp. Bot. 69, 3359-3372. doi: 10.1093/jxb/ery130
Hegedüs, N., and Marx, F. (2013). Antifungal proteins: more than antimicrobials? Fungal Biol. Rev. 26, 132–145. doi: 10.1016/j.fbr.2012.07.002
Helleboid, S., Hendriks, T., Bauw, G., Inzé, D., Vasseur, J., and Hilbert, J. L. (2000). Three major somatic embryogenesis related proteins in Cichorium identified as PR proteins. J. Exp. Bot. 51, 1189–1200. doi: 10.1093/jexbot/51.348.1189
Hilbert, J. L., Dubois, T., and Vasseur, J. (1992). Detection of embryogenesis-related proteins during somatic embryo formation in Cichorium. Plant Physiol. Biochem. 30, 733–741.
Hoseini, M., Ghadimzadeh, M., Ahmadi, B., and Teixeira da Silva, J. A. (2014). Effects of ascorbic acid, alpha-tocopherol, and glutathione on microspore embryogenesis in Brassica napus L. In Vitro Cell. Develop. Biol. Plant 50, 26–35. doi: 10.1007/s11627-013-9579-8
Hosp, J., de Faria Maraschin, S., Touraev, A., and Boutilier, K. (2007). Functional genomics of microspore embryogenesis. Euphytica 158, 275–285. doi: 10.1007/s10681-006-9238-9
Huang, H., Ullah, F., Zhou, D.-X., Yi, M., and Zhao, Y. (2019). Mechanisms of ROS Regulation of Plant Development and Stress Responses. Front. Plant Sci. 10:800. doi: 10.3389/fpls.2019.00800
Igasaki, T., Akashi, N., Ujino-Hara, T., Matsubayashi, Y., Sakagami, Y., and Shinohara, K. (2003). Phytosulfokine stimulates somatic embryogenesis in Cryptomeria japonica. Plant Cell Physiol. 44, 1412–1416. doi: 10.1093/pcp/pcg161
Ilić-Grubor, K., Attree, S. M., and Fowke, L. C. (1998). Comparative morphological study of zygotic and microspore-derived embryos of Brassica napus L. as revealed by scanning electron microscopy. Ann. Bot. 82, 157–165. doi: 10.1006/anbo.1998.0661
International Wheat Genome Sequencing Consortium (2014). A chromosome-based draft sequence of the hexaploid bread wheat (Triticum aestivum) genome. Science 345:1251788. doi: 10.1126/science.1251788
Jacquard, C., Mazeyrat-Gourbeyre, F., Devaux, P., Boutilier, K., Baillieul, F., and Clement, C. (2009). Microspore embryogenesis in barley: anther pre-treatment stimulates plant defence gene expression. Planta 229, 393–402. doi: 10.1007/s00425-008-0838-6
Johnson, K. L., Jones, B. J., Bacic, A., and Schultz, C. J. (2003). The fasciclin-like arabinogalactan proteins of arabidopsis. A multigene family of putative cell adhesion molecules. Plant Physiol. 133, 1911–1925. doi: 10.1104/pp.103.031237
Joosen, R., Cordewener, J., Supena, E. D. J., Vorst, O., Lammers, M., Maliepaard, C., et al. (2007). Combined transcriptome and proteome analysis identifies pathways and markers associated with the establishment of rapeseed microspore-derived embryo development. Plant Physiol. 144, 155–172. doi: 10.1104/pp.107.098723
Kader, J. (1996). Lipid-transfer proteins in plants. Annu. Rev. Plant. Physiol. Plant Mol. Biol. 47, 627-654. doi: 10.1146/annurev.arplant.47.1.627
Kalinina, E., and Novichkova, M. (2021). Glutathione in protein redox modulation through S-glutathionylation and S-nitrosylation. Molecules 26:435. doi: 10.3390/molecules26020435
Kasha, K., and Maluszynski, M. (2003). “Production of doubled haploids in crop plants. An introduction,” in Doubled Haploid Production in Crop Plants, eds M. Maluszynski, K. J. Kasha, B. P. Forster, and I. Szarejko (Dordrecht: Kluwer Academic Publishers), 1-4. doi: 10.1007/978-94-017-1293-4_1
Kayum, M. A., Nath, U. K., Park, J.-I., Biswas, M. K., Choi, E. K., Song, J.-Y., et al. (2018). Genome-wide identification, characterization, and expression profiling of glutathione S-transferase (GST) family in pumpkin reveals likely role in cold-stress tolerance. Genes 9:84. doi: 10.3390/genes9020084
Kiełkowska, A., and Adamus, A. (2019). Peptide growth factor phytosulfokine-α stimulates cell divisions and enhances regeneration from B. oleracea var. capitata L. protoplast culture. J. Plant Growth Regul. 38, 931–944. doi: 10.1007/s00344-018-9903-y
Kim, S.-I., Andaya, V. C., and Tai, T. H. (2011). Cold sensitivity in rice (Oryza sativa L.) is strongly correlated with a naturally occurring 199V mutation in the multifunctional glutathione transferase isoenzyme GSTZ2. Biochem. J. 435, 373–380. doi: 10.1042/BJ20101610
Kim, Y. O., Kim, J. S., and Kang, H. (2005). Cold-inducible zinc finger-containing glycine-rich RNA-binding protein contributes to the enhancement of freezing tolerance in Arabidopsis thaliana. Plant J. 42, 890–900. doi: 10.1111/j.1365-313X.2005.02420.x
Kobayashi, T., Eun, C. H., Hanai, H., Matsubayashi, Y., Sakagami, Y., and Kamada, H. (1999). Phytosulphokine-alpha, a peptidyl plant growth factor, stimulates somatic embryogenesis in carrot. J. Exp. Bot. 50, 1123–1128. doi: 10.1093/jexbot/50.336.1123
Konieczny, R., Swierczyńska, J., Czaplicki, A. Z., and Bohdanowicz, J. (2007). Distribution of pectin and arabinogalactan protein epitopes during organogenesis from androgenic callus of wheat. Plant Cell Rep. 26, 355–363. doi: 10.1007/s00299-006-0222-6
Konopka, J. B. (2012). N-acetylglucosamine (GlcNAc) functions in cell signaling. Scientifica 2012:489208. doi: 10.6064/2012/489208
Krzewska, M., Czyczyło-Mysza, I., Dubas, E., Gołebiowska-Pikania, G., Golemiec, E., Stojałowski, S., et al. (2012). Quantitative trait loci associated with androgenic responsiveness in triticale (xTriticosecale Wittm.) anther culture. Plant Cell Rep. 31, 2099–2108. doi: 10.1007/s00299-012-1320-2
Krzewska, M., Gołebiowska-Pikania, G., Dubas, E., Gawin, M., and Zur, I. (2017). Identification of proteins related to microspore embryogenesis responsiveness in anther cultures of winter triticale (x Triticosecale Wittm.). Euphytica 213:192. doi: 10.1007/s10681-017-1978-1
Kuwabara, C., and Imai, R. (2009). Molecular basis of disease resistance acquired through cold acclimation in overwintering plants. J. Plant Biol. 52, 19–26. doi: 10.1007/s12374-008-9006-6
Lacerda, A. F., Vasconcelos, E. A. R., Pelegrini, P. B., and Grossi de Sa, M. F. (2014). Antifungal defensins and their role inplant defense. Front. Microbiol. 5:116. doi: 10.3389/fmicb.2014.00116
Lamport, D. T. A., and Várnai, P. (2013). Periplasmic arabinogalactan glycoproteins act as a calcium capacitor that regulates plant growth and development. New Phytol. 197, 58–64 doi: 10.1111/nph.12005
Lee, S. C., Hwang, I. S., Choi, H. W., and Hwang, B. K. (2008). Involvement of the pepper antimicrobial protein CaAMP1 gene in broad spectrum disease resistance. Plant Physiol. 148, 1004–1020. doi: 10.1104/pp.108.123836
Lei, R., Li, X., Ma, Z., Lv, Y., Hu, Y., and Yu, D. (2017). Arabidopsis WRKY2 and WRKY34 transcription factors interact with VQ20 protein to modulate pollen development and function. Plant J. 91, 962–976. doi: 10.1111/tpj.13619
Leljak-Levanić, D., Mihaljević, S., and Bauer, N. (2015). Somatic and zygotic embryos share common developmental features at the onset of plant embryogenesis. Acta Physiol. Plant. 37:127. doi: 10.1007/s11738-015-1875-y
Leszczuk, A., Pieczywek, P. M., Gryta, A., Frac, M., and Zdunek, A. (2019). Immunocytochemical studies on the distribution of arabinogalactan proteins (AGPs) as a response to fungal infection in Malus x domestica fruit. Sci. Rep. 9:17428. doi: 10.1038/s41598-019-54022-3
Letarte, J., Simion, E., Miner, M., and Kasha, K. J. (2006). Arabinogalactans and arabinogalactan-proteins induce embryogenesis in wheat (Triticum aestivum L.) microspore culture. Plant Cell Rep. 24, 691–698. doi: 10.1007/s00299-005-0013-5
Leubner-Metzger, G., and Meins, F. (1999). “Functions and regulation of plant β-1,3-glucanases (PR-2),” in Pathogenesis-related proteins in plants, eds S. K. Datta, and S. Muthukrishnan (Florida: CRC Press), 49-76. doi: 10.1201/9781420049299.ch3
Levy, A., Erlanger, M., Rosenthal, M., and Epel, B. L. (2007). A plasmodesmata-associated beta-1,3-glucanase in Arabidopsis. Plant J. 49, 669–682. doi: 10.1111/j.1365-313X.2006.02986.x
Li, B., Wang, W., Zong, Y., Qin, G., and Tian, S. (2012). Exploring pathogenic mechanisms of Botrytis cinerea secretome under different ambient pH based on comparative proteomic analysis. J. Proteome Res. 11, 4249–4260. doi: 10.1021/pr300365f
Li, G., Wang, L., Yang, J., He, H., Jin, H., Li, X., et al. (2021). A high-quality genome assembly highlights rye genomic characteristics and agronomically important genes. Nat. Genet. 53, 574-584. doi: 10.1038/s41588-021-00808-z
Li, H., Soriano, M., Cordewener, J., Muino, J. M., Riksen, T., Fukuoka, H., et al. (2014). The histone deacetylase inhibitor trichostatin a promotes totipotency in the male gametophyte. Plant Cell 26, 195–209. doi: 10.1105/tpc.113.116491
Li, J.-Y., Wang, J., and Zeigler, R. S. (2014). The 3,000 rice genomes project: new opportunities and challenges for future rice research. Gigascience 3:8. doi: 10.1186/2047-217X-3-8
Li, M., Lopato, S., Hrmova, M., Pickering, M., Shirley, N., Koltunow, A. M., et al. (2014). Expression patterns and protein structure of a lipid transfer protein END1 from Arabidopsis. Planta 240, 1319–1334. doi: 10.1007/s00425-014-2155-6
Li, W. L., Liu, Y., and Douglas, C. J. (2017). Role of glycosyltransferases in pollen wall primexine formation and exine patterning. Plant Physiol. 173, 167–182. doi: 10.1104/pp.16.00471
Liu, F., Zhang, X., Lu, C., Zeng, X., Li, Y., Fu, D., et al. (2015). Non-specific lipid transfer proteins in plants: presenting new advances and an integrated functional analysis. J. Exp. Bot. 66, 5663–5681. doi: 10.1093/jxb/erv313
Liu, J., Gaj, T., Patterson, J. T., Sirk, S. J., and Barbas, C. F. III. (2014). Cell-penetrating peptide-mediated delivery of TALEN proteins via bioconjugation for genome engineering. PLoS ONE 9:e85755. doi: 10.1371/journal.pone.0085755
Liu, K. H., and Lin, T. Y. (2003). Cloning and characterization of two novel lipid transfer protein I genes in Vigna radiata. DNA Seq.4, 420–426. doi: 10.1080/1042517032000160198
Liu, S., Kracher, B., Ziegler, J., Birkenbihl, R. P., and Somssich, I. E. (2015). Negative regulation of ABA signaling by WRKY33 is critical for Arabidopsis immunity toward Botrytis cinerea 2100. Elife 4:e07295. doi: 10.7554/eLife.07295.033
Locato, V., Cimini, S., and De Gara, L. (2018). ROS and redox balance as multifaceted players of cross-tolerance: epigenetic and retrograde control of gene expression. J. Exp. Bot. 69, 3373–3391. doi: 10.1093/jxb/ery168
Locato, V., Uzal, E. N., Cimini, S., Zonno, M. C., Evidente, A., Micera, A., et al. (2015). Low concentrations of the toxin ophiobolin a lead to an arrest of the cell cycle and alter the intracellular partitioning of glutathione between the nuclei and cytoplasm. J. Exp. Bot. 66, 2991–3000. doi: 10.1093/jxb/erv110
Lyapina, I., Filippova, A., and Fesenko, I. (2019). The role of peptide signals hidden in the structure of functional proteins in plant immune responses. Int. J. Mol. Sci. 20:4343. doi: 10.3390/ijms20184343
Malaga, S., Janeczko, A., Janowiak, F., Waligórski, P., Oklestkova, J., Dubas, E., et al. (2020). Involvement of homocastasterone, salicylic and abscisic acids in the regulation of drought and freezing tolerance in doubled haploid lines of winter barley. Plant Growth Regul. 90, 173–188. doi: 10.1007/s10725-019-00544-9
Maleki, F., Ovens, K. L., Hogan, D. J., Rezaei, E., Rosenberg, A. M., and Kusalik, A. J. (2019). Measuring consistency among gene set analysis methods: a systematic study. J. Bioinform. Comput. Biol. 17:1940010. doi: 10.1142/S0219720019400109
Malik, M. R., Wang, F., Dirpaul, J. M., Zhou, N., Polowick, P. L., Ferrie, A. M. R., et al. (2007). Transcript profiling and identification of molecular markers for early microspore embryogenesis in Brassica napus. Plant Physiol. 144, 134–154. doi: 10.1104/pp.106.092932
Maluszynski, M., Szarejko, I., and Sigurbjörnsson, B. (1996). “Haploidy and mutation techniques,” in In vitro Haploid Production in Higher Plants, eds S. M. Jain, S. K. Sopory, and R. E. Veilleux (Dordrecht: Academic Publishers), 67–93. doi: 10.1007/978-94-017-1860-8_5
Maraschin, S. de. F., Caspers, M., Potokina, E., Wuelfert, F., Graner, A., Spaink, H. P., et al. (2006). CDNA array analysis of stress-induced gene expression in barley androgenesis. Physiol. Plant. 127, 535–550. doi: 10.1111/j.1399-3054.2006.00673.x
Maraschin, S. F., de Priester, W., Spaink, H. P., and Wang, M. (2005). Androgenic switch: an example of plant embryogenesis from the male gametophyte perspective. J. Exp. Bot. 56, 1711–1726. doi: 10.1093/jxb/eri190
Marathi, B., Guleria, S., Mohapatra, T., Parsad, R., Mariappan, N., Kurungara, V. K., et al. (2012). QTL analysis of novel genomic regions associated with yield and yield related traits in new plant type based recombinant inbred lines of rice (Oryza sativa L.). BMC Plant Biol. 12:137. doi: 10.1186/1471-2229-12-137
Mareri, L., Romi, M., and Cai, G. (2018). Arabinogalactan proteins: actors or spectators during abiotic and biotic stress in plants? Plant Biosyst. 153, 173–185. doi: 10.1080/11263504.2018.1473525
Maróti, G., Downie, J. A., and Kondorosi, É. (2015). Plant cysteine-rich peptides that inhibit pathogen growth and control rhizobial differentiation in legume nodules. Curr. Opin. Plant Biol. 26, 57–63. doi: 10.1016/j.pbi.2015.05.031
Marty, L., Siala, W., Schwarzländer, M., Fricker, M. D., Wirtz, M., Sweetlove, L. J., et al. (2009). The NADPH-dependent thioredoxin system constitutes a functional backup for cytosolic glutathione reductase in Arabidopsis. Proc. Natl. Acad. Sci. U. S. A. 106, 9109–9114. doi: 10.1073/pnas.0900206106
Mascher, M., Gundlach, H., Himmelbach, A., Beier, S., Twardziok, S. O., Wicker, T., et al. (2017). A chromosome conformation capture ordered sequence of the barley genome. Nature 544, 427-433. doi: 10.1038/nature22043
Mashiguchi, K., Yamaguchi, I., and Suzuki, Y. (2004). Isolation and identification of glycosylphosphatidylinositol-anchored arabinogalactan proteins and novel beta-glucosyl Yariv-reactive proteins from seeds of rice (Oryza sativa). Plant Cell Physiol. 45, 1817–1829. doi: 10.1093/pcp/pch208
Matsubayashi, Y. (2014). Post-translationally modified small-peptide signals in plants. Annu. Rev. Plant Biol. 65, 385–413. doi: 10.1146/annurev-arplant-050312-120122
Matthys-Rochon, E. (2005). Secreted molecules and their role in embryo formation in plants: a mini-review. Acta Biol. Cracov. Bot. 47, 23–29. Available online at: https://hal.archives-ouvertes.fr/hal-00188834
Maughan, S., and Foyer, C. H. (2006). Engineering and genetic approaches to modulating the glutathione network in plants. Physiol. Plant. 126, 382–397. doi: 10.1111/j.1399-3054.2006.00684.x
Maynard, J. C., Burlingame, A. L., and Medzihradszky, K. F. (2016). Cysteine S-linked N-acetylglucosamine (S-GlcNAcylation), a new post-translational modification in mammals. Mol. Cell. Proteom. 15, 3405–3411. doi: 10.1074/mcp.M116.061549
Meena, M., Prasad, V., Zehra, A., Gupta, V. K., and Upadhyay, R. S. (2015). Mannitol metabolism during pathogenic fungal–host interactions under stressed conditions. Front. Microbiol. 6:1019. doi: 10.3389/fmicb.2015.01019
Melo, F. R., Rigden, D. J., Franco, O. L., Mello, L. V., Ary, M. B., de Sa, M. F. G., et al. (2002). Inhibition of trypsin by cowpea thionin: characterization, molecular modeling, and docking. Proteins 48, 311–319. doi: 10.1002/prot.10142
Mészáros, B., Simon, I., and Dosztányiet, Z. (2011). The expanding view of protein-protein interactions: complexes involving intrinsically disordered proteins. Phys. Biol. 8:035003. doi: 10.1088/1478-3975/8/3/035003
Michalko, J., Renner, T., Mészáros, P., Socha, P., Moravčíková, J., Blehová, A., et al. (2017). Molecular characterization and evolution of carnivorous sundew (Drosera rotundifolia L.) class V β-1,3-glucanase. Planta 245, 77–91. doi: 10.1007/s00425-016-2592-5
Minic, Z. (2008). Physiological roles of plant glycoside hydrolases. Planta 227, 723–740. doi: 10.1007/s00425-007-0668-y
Monat, C., Padmarasu, S., Lux, T., Wicker, T., Gundlach, H., Himmelbach, A., et al. (2019). TRITEX: chromosome-scale sequence assembly of Triticeae genomes with open-source tools. Genome Biol. 20:284. doi: 10.1186/s13059-019-1899-5
Moravčíková, J., Matušíková, I., and Libantová, J. (2004). Expression of a cucumber class III chitinase and Nicotiana plumbaginifolia class I glucanase genes in transgenic potato plants. Plant Cell Tiss. Organ Cult. 79, 161–168. doi: 10.1007/s11240-004-0656-x
Morimoto, K., and van der Hoorn, R. A. L. (2019). Plant biology: proteolytic release of damage signals. Curr. Biol. 29, 378–380. doi: 10.1016/j.cub.2019.04.014
Muñoz-Amatriaín, M., Svensson, J. T., Castillo, A.-M., Cistue, L., Close, T. J., and Valles, M.-P. (2006). Transcriptome analysis of barley anthers: effect of mannitol treatment on microspore embryogenesis. Physiol. Plant. 127, 551–560. doi: 10.1111/j.1399-3054.2006.00729.x
Muñoz-Amatriaín, M., Svensson, J. T., Castillo, A. M., Close, T. J., and Vallés, M. P. (2009). Microspore embryogenesis: assignment of genes to embryo formation and green vs. albino plant production. Funct. Integr. Genomics 9, 311–323. doi: 10.1007/s10142-009-0113-3
Murphy, E., Smith, S., and De Smet, I. (2012). Small signaling peptides in Arabidopsis development: how cells communicate over a short distance. Plant Cell 24, 3198–3217. doi: 10.1105/tpc.112.099010
Naseem, S., Parrino, S. M., Buenten, D. M., and Konopka, J. B. (2012). Novel roles for GlcNAc in cell signaling. Commun. Integr. Biol. 5, 156–159. doi: 10.4161/cib.19034
Nawrot, R., Barylski, J., Nowicki, G., Broniarczyk, J., Buchwald, W., and Gozdzicka-Józefiak, A. (2014). Plant antimicrobial peptides. Folia Microbiol. (Praha) 59, 181–196 doi: 10.1007/s12223-013-0280-4
Nielsen, K. A., and Hansen, I. B. (1992). Appearance of extracellular proteins associated with somatic embryogenesis in suspension cultures of barley (Hordeum vulgare L.). J. Plant Physiol. 139, 489–497. doi: 10.1016/S0176-1617(11)80500-6
Nieuwland, J., Feron, R., Huisman, B. A. H., Fasolino, A., Hilbers, C. W., Derksen, J., et al. (2005). Lipid transfer proteins enhance cell wall extension in tobacco. Plant Cell 17, 2009–2019. doi: 10.1105/tpc.105.032094
Nikte, S., Gahankari, A., Mulla, J., Sengupta, D., Joshi, M., and Tamhane, V. (2020). In vitro and in silico studies on membrane interactions of diverse Capsicum annuum flower γ-thionin peptides. Proteins 88, 227–236. doi: 10.1002/prot.25791
Noctor, G., Mhamdi, A., Chaouch, S., Han, Y., Neukermans, J., Marquez-Garcia, B., et al. (2012). Glutathione in plants: an integrated overview. Plant Cell Environ. 35, 454–484. doi: 10.1111/j.1365-3040.2011.02400.x
Nowicka, A., Juzoń, K., Krzewska, M., Dziurka, M., Dubas, E., Kopeć, P., et al. (2019). Chemically-induced DNA de-methylation alters the effectiveness of microspore embryogenesis in triticale. Plant Sci. 287:110189. doi: 10.1016/j.plantsci.2019.110189
Olszewski, N. E., West, C. M., Sassi, S. O., and Hartweck, L. M. (2010). O-GlcNAc protein modification in plants: evolution and function. Biochim. Biophys. Acta Gen. Subj. 1800, 49–56. doi: 10.1016/j.bbagen.2009.11.016
Paire, A., Devaux, P., Lafitte, C., Dumas, C., and Matthys-Rochon, E. (2003). Proteins produced by barley microspores and their derived androgenic structures promote in vitro zygotic maize embryo formation. Plant Cell Tiss. Org. 73, 167–176. doi: 10.1023/A:1022805623167
Park, A. R., Cho, S. K., Yun, U. J., Jin, M. Y., Lee, S. H., Sachetto-Martins, G., et al. (2001). Interaction of the Arabidopsis receptor protein kinase Wak1 with a glycine-rich protein, AtGRP-3. J. Biol. Chem. 276, 26688–26693. doi: 10.1074/jbc.M101283200
Parra-Vega, V., Corral-Martinez, P., Rivas-Sendra, A., and Seguí-Simarro, J. M. (2015). Induction of embryogenesis in Brassica napus microspores produces a callosic subintinal layer and abnormal cell walls with altered levels of callose and cellulose. Front. Plant Sci. 6:1018. doi: 10.3389/fpls.2015.01018
Pauls, K. P., Chan, J., Woronuk, G., Schulze, D., and Brazolot, J. (2006). When microspores decide to become embryos–Cellular and molecular changes. Can. J. Bot. 84, 668–678. doi: 10.1139/b06-064
Paxson-Sowders, D. M., Owen, H. A., and Makaroff, C. A. (1997). A comparative ultrastructural analysis of exine pattern development in wild-type Arabidopsis and a mutant defective in pattern formation. Protoplasma 198, 53–65. doi: 10.1007/BF01282131
Pearce, G., Moura, D. S., Stratmann, J., and Ryan, C. A. (2001). RALF, a 5-kDa ubiquitous polypeptide in plants, arrests root growth and development. PNAS U.S.A. 98, 12843–12847. doi: 10.1073/pnas.201416998
Pennell, R. I., Janniche, L., Kjellbom, P., Scofield, G. N., Peart, J. M., and Roberts, K. (1991). Developmental regulation of a plasma membrane arabinogalactan protein epitope in oilseed rape flowers. The Plant Cell 3, 1317–1326. doi: 10.1105/tpc.3.12.1317
Pereira, A. M., Lopes, A. L., and Coimbra, S. (2016). Arabinogalactan proteins as interactors along the crosstalk between the pollen tube and the female tissues. Front. Plant Sci. 7:1895. doi: 10.3389/fpls.2016.01895
Pérez-Clemente, R. M., and Gómez-Cadenas, A. (2012). “In vitro tissue culture, a tool for the study and breeding of plants subjected to abiotic stress conditions,” in Recent Advances in Plant in vitro Culture, eds A. Leva, and L. M. R. Rinaldi. IntechOpen. doi: 10.5772/50671
Petrovská, B., Salaj, T., Moravčíková, J., Libantová, J., and Salaj, J. (2010). Development of embryo-like structures in the suspension cultures of flax coincides with secretion of chitinase-like proteins. Acta Physiol. Plant. 32, 651–656. doi: 10.1007/s11738-009-0442-9
Pfeifer, L., Shafee, T., Johnson, K. L., Bacic, A., and Classen, B. (2020). Arabinogalactan-proteins of Zostera marina L. contain unique glycan structures and provide insight into adaption processes to saline environments. Sci. Rep. 10:8232. doi: 10.1038/s41598-020-65135-5
Phyo, P., Gu, Y., and Hong, M. (2018). Impact of acidic pH on plant cell wall polysaccharide structure and dynamics: insights into the mechanism of acid growth in plants from solid-state NMR. Cellulose 26, 291–304. doi: 10.1007/s10570-018-2094-7
Plattner, S., Gruber, C., Stadlmann, J., Widmann, S., Gruber, C. W., Altmann, F., et al. (2015). Isolation and characterization of a thionin proprotein-processing enzyme from barley. J. Biol. Chem. 290, 18056–18067. doi: 10.1074/jbc.M115.647859
Poole, L. B. (2015). The basics of thiols and cysteines in redox biology and chemistry. Free Radic. Biol. Med. 80, 148–157. doi: 10.1016/j.freeradbiomed.2014.11.013
Potocka, I., Baldwin, T. C., and Kurczyńska, E. U. (2012). Distribution of lipid transfer protein 1 (LTP1) epitopes associated with morphogenic events during somatic embryogenesis of Arabidopsis thaliana. Plant Cell Rep. 31, 2031–2045. doi: 10.1007/s00299-012-1314-0
Pourmohammad, A., Moieni, A., Dehghani, H., and Rashidi-Monfared, S. (2021). Field-grown donor plants and arabinogalactan proteins improve microspore embryogenesis in sweet pepper (Capsicum annuum L.). In Vitro Cell. Dev. Biol. Plant 57, 510–518. doi: 10.1007/s11627-020-10152-2
Qin, Y., Chen, D., and Zhao, J. (2007). Localization of arabinogalactan proteins in anther, pollen, and pollen tube of Nicotiana tabacum L. Protoplasma 231, 43–53. doi: 10.1007/s00709-007-0245-z
Queval, G., and Foyer, C. H. (2012). Redox regulation of photosynthetic gene expression. Philos. Trans. R Soc. Lond. B Biol. Sci. 367, 3475–3485. doi: 10.1098/rstb.2012.0068
Queval, G., Jaillard, D., Zechmann, B., and Noctor, G. (2011). Increased intracellular H2O2 availability preferentially drives glutathione accumulation in vacuoles and chloroplasts. Plant Cell Environ. 34, 21–32. doi: 10.1111/j.1365-3040.2010.02222.x
Quilichini, T. D., Grienenberger, E., and Douglas, C. J. (2015). The biosynthesis, composition and assembly of the outer pollen wall: a tough case to crack. Phytochemistry 113, 170–182. doi: 10.1016/j.phytochem.2014.05.002
Rabanus-Wallace, M. T., Hackauf, B., Mascher, M., Lux, T., Wicker, T., Gundlach, H., et al. (2021). Chromosome-scale genome assembly provides insights into rye biology, evolution and agronomic potential. Nat. Genet. 53, 564–573. doi: 10.1038/s41588-021-00807-0
Rahantaniaina, M.-S., Tuzet, A., Mhamdi, A., and Noctor, G. (2013). Missing links in understanding redox signaling via thiol/disulfide modulation: how is glutathione oxidized in plants? Front. Plant Sci. 4:477. doi: 10.3389/fpls.2013.00477
Rai, M. K., Kalia, R. K., Singh, R., Gangola, M. P., and Dhawan, A. K. (2011). Developing stress tolerant plants through in vitro selection—an overview of the recent progress. Environ. Exp. Bot. 71, 89–98. doi: 10.1016/j.envexpbot.2010.10.021
Rasheed, A., and Xia, X. (2019). From markers to genome-based breeding in wheat. Theor. Appl. Genet. 132, 767–784. doi: 10.1007/s00122-019-03286-4
Razzaq, A., Saleem, F., Kanwal, M., Mustafa, G., Yousaf, S., Arshad, H. M. I., et al. (2019). Modern trends in plant genome editing: an inclusive review of the CRISPR/Cas9 Toolbox. Int. J. Mol. Sci. 20:4045. doi: 10.3390/ijms20164045
Ren, J., Wu, P., Trampe, B., Tian, X., Lübberstedt, T., and Chen, S. (2017). Novel technologies in doubled haploid line development. Plant Biotechnol. J. 15, 1361–1370. doi: 10.1111/pbi.12805
Rivas-Sendra, A., Corral-Martinez, P., Porcel, R., Camacho-Fernandez, C., Calabuig-Serna, A., and Seguí-Simarro, J. M. (2019). Embryogenic competence of microspores is associated with their ability to form a callosic, osmoprotective subintinal layer. J. Exp. Bot. 70, 1267–1281. doi: 10.1093/jxb/ery458
Roeder, S., Dreschler, K., Wirtz, M., Cristescu, S. M., van Harren, F. J. M., Hell, R., et al. (2009). SAM levels, gene expression of SAM synthetase, methionine synthase and ACC oxidase, and ethylene emission from N. suaveolens flowers. Plant Mol. Biol. 70, 535–546. doi: 10.1007/s11103-009-9490-1
Sahu, P. K., Sao, R., Mondal, S., Vishwakarma, G., Gupta, S. K., Kumar, V., et al. (2020). Next generation sequencing based forward genetic approaches for identification and mapping of causal mutations in crop plants: a comprehensive review. Plant 9:1355. doi: 10.3390/plants9101355
Sahu, P. P., Pandey, G., Sharma, N., Puranik, S., Muthamilarasan, M., and Prasad, M. (2013). Epigenetic mechanisms of plant stress responses and adaptation. Plant Cell Rep. 32, 1151–1159. doi: 10.1007/s00299-013-1462-x
Saito, M., Mukai, Y., Komazaki, T., Oh, K. B., Nishizawa, Y., Tomiyama, M., et al. (2003). Expression of rice chitinase gene triggered by the direct injection of Ca2+. J. Biotechnol. 105, 41–49. doi: 10.1016/S0168-1656(03)00184-6
Salas, C. E., Badillo-Corona, J. A., Ramírez-Sotelo, G., and Oliver-Salvador, C. (2015). Biologically active and antimicrobial peptides from plants. Bio. Med. Res. Int. 2015:102129. doi: 10.1155/2015/102129
Salminen, T. A., Blomqvist, K., and Edqvist, J. (2016). Lipid transfer proteins: classification, nomenclature, structure, and function. Planta 244, 971–997. doi: 10.1007/s00425-016-2585-4
Šamaj, J., Bobák, M., and Erdelský, K. (1990). Histological-anatomical studies of the structure of the organogenic callus in Papaver somniferum L. Biol. Plant. 32, 14–18. doi: 10.1007/BF02897337
Sánchez-Díaz, R. A., Castillo, A. M., and Vallés, M. P. (2013). Microspore embryogenesis in wheat: new marker genes for early, middle and late stages of embryo development. Plant Reprod. 26, 287–296. doi: 10.1007/s00497-013-0225-8
Sato, K. (2020). History and future perspectives of barley genomics. DNA Res. 27:dsaa023. doi: 10.1093/dnares/dsaa023
Schnable, P. S., Ware, D., Fulton, R. S., Stein, J. C., Wei, F., Pasternak, S., et al. (2009). The B73 maize genome: complexity, diversity, and dynamics. Science 326, 1112–1115. doi: 10.1126/science.1178534
Schnaubelt, D., Queval, G., Dong, Y., Diaz-Vivancos, P., Makgopa, M. E., Howell, G., et al. (2015). Low glutathione regulates gene expression and the redox potentials of the nucleus and cytosol in Arabidopsis thaliana. Plant Cell Environ. 38, 266–279. doi: 10.1111/pce.12252
Schneider-Müller, S., Kurosaki, F., and Nishi, A. (1994). Role of salicylic acid and intracellular Ca2+ in the induction of chitinase activity in carrot suspension culture. Physiol. Mol. Plant Pathol. 45, 101–109. doi: 10.1016/S0885-5765(05)80069-4
Schwarzländer, M., Fricker, M. D., Müller, C., Marty, L., Brach, T., Novak, J., et al. (2008). Confocal imaging of glutathione redox potential in living plant cells. J. Microsc. 231, 299–316. doi: 10.1111/j.1365-2818.2008.02030.x
Seguí-Simarro, J. M., Corral-Martínez, P., Parra-Vega, V., and González-García, B. (2011). Androgenesis in recalcitrant solanaceous crops. Plant Cell Rep. 30, 765–778. doi: 10.1007/s00299-010-0984-8
Seguí-Simarro, J. M., and Nuez, F. (2008). How microspores transform into haploid embryos: changes associated with embryogenesis induction and microspore-derived embryogenesis. Physiol. Plant. 134, 1–12. doi: 10.1111/j.1399-3054.2008.01113.x
Seifert, F., Boessow, S., Kumlehn, J., Gnad, H., and Scholten, S. (2016). Analysis of wheat microspore embryogenesis induction by transcriptome and small RNA sequencing using the highly responsive cultivar “Svilena.” BMC Plant Biol. 16:97. doi: 10.1186/s12870-016-0782-8
Seifert, G., and Roberts, K. (2007). The biology of arabinogalactan proteins. Annu. Rev. Plant Biol. 58, 137–161. doi: 10.1146/annurev.arplant.58.032806.103801
Shahmir, F., and Pauls, K. P. (2021). Identification, gene structure, and expression of BnMicEmUP: a gene upregulated in embryogenic Brassica napus microspores. Front. Plant Sci. 11:576008. doi: 10.3389/fpls.2020.576008
Shan, X., Chang, Y., and Lin, C.-L. G. (2007). Messenger RNA oxidation is an early event preceding cell death and causes reduced protein expression. Faseb J. 21, 2753–2764. doi: 10.1096/fj.07-8200com
Shchukina, L. V., Pshenichnikova, T. A., Khlestkina, E. K., Misheva, S., Kartseva, T., Abugalieva, A., et al. (2018). Chromosomal location and mapping of quantitative trait locus determining technological parameters of grain and flour in strong-flour bread wheat cultivar saratovskaya 29. Cereal Res. Commun. 46, 628–638. doi: 10.1556/0806.46.2018.047
Shi, Y. G., Lian, Y., Shi, H. W., Wang, S. G., Fan, H., Sun, D. Z., et al. (2019). Dynamic analysis of QTLs for green leaf area duration and green leaf number of main stem in wheat. Cereal Res. Commun. 47, 250–263. doi: 10.1556/0806.47.2019.06
Showalter, A. M. (2001). Arabinogalactan-proteins: structure, expression and function. Cell Mol. Life Sci. 58, 1399–1417. doi: 10.1007/PL00000784
Shu, H., Xu, L., Li, Z., Li, J., Jin, Z., and Chang, S. (2014). Tobacco arabinogalactan protein NtEPc can promote banana (Musa AAA) somatic embryogenesis. Appl. Biochem. Biotechnol. 174, 2818–2826. doi: 10.1007/s12010-014-1228-0
Simon, R., and Dresselhaus, T. (2015). Peptides take centre stage in plant signalling. J. Exp. Bot. 66, 5135–5138. doi: 10.1093/jxb/erv376
Solís, M.-T., El-Tantawy, A.-A., Cano, V., Risueno, M. C., and Testillano, P. S. (2015). 5-azacytidine promotes microspore embryogenesis initiation by decreasing global DNA methylation, but prevents subsequent embryo development in rapeseed and barley. Front. Plant Sci. 6:472. doi: 10.3389/fpls.2015.00472
Song, X.-F., Ren, S.-C., and Liu, C.-M. (2017). “Peptide hormones,” in Hormone Metabolism and Signaling in Plants, eds J. Li, C. Li, and S. M. Smith (London: Academic Press), 361–404. doi: 10.1016/B978-0-12-811562-6.00011-6
Soriano, M., Li, H., and Boutilier, K. (2013). Microspore embryogenesis: establishment of embryo identity and pattern in culture. Plant Reprod. 26, 181–196. doi: 10.1007/s00497-013-0226-7
Soriano, M., Li, H., Jacquard, C., Angenent, G. C., Krochko, J., Offringa, R., et al. (2014). Plasticity in cell division patterns and auxin transport dependency during in vitro embryogenesis in Brassica napus. Plant Cell 26, 2568–2581. doi: 10.1105/tpc.114.126300
Stacey, N. J., Roberts, K., and Knox, J. P. (1990). Patterns of expression of the JIM4 arabinogalactan-protein epitope in cell cultures and during somatic embryogenesis in Daucus carota L. Planta 180, 285–292. doi: 10.1007/BF00194009
Stasolla, C. (2010). Glutathione redox regulation of in vitro embryogenesis. Plant Physiol. Biochem. 48, 319–327. doi: 10.1016/j.plaphy.2009.10.007
Stavolone, L., and Lionetti, V. (2017). Extracellular matrix in plants and animals: hooks and locks for viruses. Front. Microbiol. 8:760. doi: 10.3389/fmicb.2017.01760
Stegmann, M., Monaghan, J., Smakowska-Luzan, E., Rovenich, H., Lehner, A., Holton, N., et al. (2017). The receptor kinase FER is a RALF-regulated scaffold controlling plant immune signaling. Science 355, 287–289. doi: 10.1126/science.aal2541
Sterk, P., Booij, H., Schellekens, G. A., Van Kammen, A., and De Vries, S. C. (1991). Cell-specific expression of the carrot EP2 lipid transfer protein gene. Plant Cell 3, 907–921. doi: 10.1105/tpc.3.9.907
Stressmann, M., Kitao, S., Griffith, M., Moresoli, C., Bravo, L. A., and Marangoni, A. G. (2004). Calcium interacts with antifreeze proteins and chitinase from cold-acclimated winter rye. Plant Physiol. 135, 364–376. doi: 10.1104/pp.103.038158
Sugano, S. S., Shimada, T., Imai, Y., Okawa, K., Tamai, A., Mori, M., et al. (2010). Stomagen positively regulates stomatal density in Arabidopsis. Nature 463, 241–244. doi: 10.1038/nature08682
Szalai, G., Kelloes, T., Galiba, G., and Kocsy, G. (2009). Glutathione as an antioxidant and regulatory molecule in plants under abiotic stress conditions. J. Plant Growth Regul. 28, 66–80. doi: 10.1007/s00344-008-9075-2
Szarejko, I., and Forster, B. P. (2007). Doubled haploidy and induced mutation. Euphytica 158, 359–370. doi: 10.1007/s10681-006-9241-1
Tam, J. P., Wang, S., Wong, K. H., and Tan, W. L. (2015). Antimicrobial peptides from plants. Pharmaceuticals (Basel, Switzerland) 8, 711–757. doi: 10.3390/ph8040711
Tang, X.-C., He, Y.-Q., Wang, Y., and Sun, M.-X. (2006). The role of arabinogalactan proteins binding to Yariv reagents in the initiation, cell developmental fate, and maintenance of microspore embryogenesis in Brassica napus L. cv. Topas. J. Exp. Bot. 57, 2639–2650. doi: 10.1093/jxb/erl027
Tavormina, P., De Coninck, B., Nikonorova, N., De Smet, I., and Cammue, B. P. A. (2015). The plant peptidome: an expanding repertoire of structural features and biological functions. Plant Cell 27, 2095–2118. doi: 10.1105/tpc.15.00440
Telmer, C. A., Newcomb, W., and Simmonds, D. H. (1995). Cellular changes during heat shock induction and embryo development of cultured microspores of Brassica napus cv. Topas. Protoplasma 185, 106–112. doi: 10.1007/BF01272758
Testillano, P. S. (2019). Microspore embryogenesis: targeting the determinant factors of stress-induced cell reprogramming for crop improvement. J. Exp. Bot. 70, 2965-2978. doi: 10.1093/jxb/ery464
Touraev, A., Pfosser, M., and Heberle-Bors, E. (2001). The microspore: a haploid multipurpose cell. Adv. Bot. Res. 35, 53–109. doi: 10.1016/S0065-2296(01)35004-8
Tsuwamoto, R., Fukuoka, H., and Takahata, Y. (2007). Identification and characterization of genes expressed in early embryogenesis from microspores of Brassica napus. Planta 225, 641–652. doi: 10.1007/s00425-006-0388-8
Tuzet, A., Rahantaniaina, M.-S., and Noctor, G. (2019). Analyzing the function of catalase and the ascorbate–glutathione pathway in H2O2 processing: insights from an experimentally constrained kinetic model. Antioxid. Redox Signal. 30, 1238–1268. doi: 10.1089/ars.2018.7601
Tyrka, M., Oleszczuk, S., Rabiza-Swider, J., Wos, H., Wedzony, M., Zimny, J., et al. (2018). Populations of doubled haploids for genetic mapping in hexaploid winter triticale. Mol. Breed. 38:46. doi: 10.1007/s11032-018-0804-3
Ueda, M., Zhang, Z., and Laux, T. (2011). Transcriptional activation of Arabidopsis axis patterning genes WOX8/9 links zygote polarity to embryo development. Dev. Cell 20, 264–270. doi: 10.1016/j.devcel.2011.01.009
van der Weerden, N. L., Bleackley, M. R., and Anderson, M. A. (2013). Properties and mechanisms of action of naturally occurring antifungal peptides. Cell Mol. Life Sci. 70, 3545-3570. doi: 10.1007/s00018-013-1260-1
van Hengel, A. J., Guzzo, F., van Kammen, A., and de Vries, S. C. (1998). Expression pattern of the carrot EP3 endochitinase genes in suspension cultures and in developing seeds. Plant Physiol. 117, 43–53. doi: 10.1104/pp.117.1.43
van Hengel, A. J., and Roberts, K. (2002). Fucosylated arabinogalactan-proteins are required for full root cell elongation in arabidopsis. Plant J. 32, 105–113. doi: 10.1046/j.1365-313X.2002.01406.x
van Hengel, A. J., Tadesse, Z., Immerzeel, P., Schols, H., van Kammen, A., and de Vries, S. C. (2001). N-acetylglucosamine and glucosamine-containing arabinogalactan proteins control somatic embryogenesis. Plant Physiol. 125, 1880–1890. doi: 10.1104/pp.125.4.1880
Vanderauwera, S., Suzuki, N., Miller, G., van de Cotte, B., Morsa, S., Ravanat, J.-L., et al. (2011). Extranuclear protection of chromosomal DNA from oxidative stress. PNAS U.S.A. 108, 1711–1716. doi: 10.1073/pnas.1018359108
Vrinten, P. L., Nakamura, T., and Kasha, K. J. (1999). Characterization of cDNAs expressed in the early stages of microspore embryogenesis in barley (Hordeum vulgare L.) Plant Mol. Biol. 41, 455–463. doi: 10.1023/A:1006383724443
Wajdzik, K., Gołebiowska, G., Dyda, M., Gawrońska, K., Rapacz, M., and Wedzony, M. (2019). The QTL mapping of the important breeding traits in winter triticale (× Triticosecale Wittm.). Cereal Res. Comm. 47:3. doi: 10.1556/0806.47.2019.24
Wang, X., Li, Q., Yuan, W., Cao, Z., Qi, B., Kumar, S., et al. (2016). The cytosolic Fe-S cluster assembly component MET18 is required for the full enzymatic activity of ROS1 in active DNA demethylation. Sci. Rep. 6:26443. doi: 10.1038/srep26443
Wang, Z., Xie, W. Q., Chi, F., and Li, C. F. (2005). Identification of non-specific lipid transfer protein-1 as a calmodulin-binding protein in Arabidopsis. FEBS Lett. 579, 1683–1687. doi: 10.1016/j.febslet.2005.02.024
Wang, Y., Li, X., Fan, B., Zhu, C., and Chen, Z. (2021). Regulation and function of defense-related callose deposition in plants. Intl. J. Mol. Sci. 22:2393. doi: 10.3390/ijms22052393
Wedzony, M., Forster, B. P., Zur, I., Golemiec, E., Szechyńska-Hebda, M., Dubas, E., et al. (2009). “Progress in doubled haploid technology in higher plants,” in Advances in Haploid Production in Higher Plants, eds A. Touraev, B. P. Forster, and S. M. Jain (Berlin: Springer Science + Business Media), 1-33. doi: 10.1007/978-1-4020-8854-4_1
Wedzony, M., Szechyńska-Hebda, M., Zur, I., Dubas, E., and Krzewska, M. (2014). “Tissue culture and regeneration: a prerequisite for alien gene transfer,” in Alien Gene Transfer in Crop Plants, Vol. 1, eds A. Pratap, and J. Kumar (New York, NY: Springer), 43–75. doi: 10.1007/978-1-4614-8585-8_3
Wojtasik, W., Boba, A., Preisner, M., Kostyn, K., Szopa, J., and Kulma, A. (2019). DNA methylation profile of β-1, 3-glucanase and chitinase genes in flax shows specificity toward Fusarium oxysporum strains differing in pathogenicity. Microorganisms 7:589. doi: 10.3390/microorganisms7120589
Wu, S.-W., Kumar, R., Bagus, A., Iswanto, B., and Kim, J.-Y. (2018). Callose balancing at plasmodesmata. J. Exp. Bot. 69, 5325–5339. doi: 10.1093/jxb/ery317
Yamaguchi, Y., Pearce, G., and Huffaker, A. (2013). “Defense signal peptides,” in Handbook of Biologically Active Peptides, ed A. J. Kastin (San Diego: Academic Press), 5-14. doi: 10.1016/B978-0-12-385095-9.00002-6
Yamakawa, S., Matsubayashi, Y., Sakagami, Y., Kamada, H., and Satoh, S. (1998). Promotion by a peptidyl growth factor, phytosulfokine, of chlorophyll formation in etiolated cotyledon of cucumber. Biosci. Biotechnol. Biochem. 62, 2441–2443. doi: 10.1271/bbb.62.2441
Yamakawa, S., Matsubayashi, Y., Sakagami, Y., Kamada, H., and Satoh, S. (1999). Promotive effects of the peptidyl plant growth factor, phytosulfokine-alpha, on the growth and chlorophyll content of Arabidopsis seedlings under high night-time temperature conditions. Biosci. Biotechnol. Biochem. 63, 2240–2243. doi: 10.1271/bbb.63.2240
Yamuna, S., Kumar, V., Subramaniam, D., and Kumaravel, K. (2019). Antimicrobial peptides from plants and their mode of action. Am. Int. J. Res. Sci. Technol. Eng. Math. 105, 265–269. Special issue of National Conference on Innovations in Bio, Chemical and Food Technology (IBCFT-2019).
Yeats, T. H., and Rose, J. K. C. (2009). The biochemistry and biology of extracellular plant lipid-transfer proteins (LTPs). Protein Sci. 17, 191–198. doi: 10.1110/ps.073300108
Yeung, E. C., Rahman, M. H., and Thorpe, T. A. (1996). Comparative development of zygotic and microspore-derived embryos in Brassica napus L cv Topas.1. Histodifferentiation. Int. J. Plant Sci. 157, 27–39. doi: 10.1086/297317
Zechmann, B. (2020). Subcellular roles of glutathione in mediating plant defense during biotic stress. Plants Basel 9:1067. doi: 10.3390/plants9091067
Zechmann, B., Koffler, B. E., and Russell, S. D. (2011). Glutathione synthesis is essential for pollen germination in vitro. BMC Plant Biol. 11:54. doi: 10.1186/1471-2229-11-54
Zechmann, B., Mauch, F., Sticher, L., and Muller, M. (2008). Subcellular immunocytochemical analysis detects the highest concentrations of glutathione in mitochondria and not in plastids. J. Exp. Bot. 59, 4017–4027. doi: 10.1093/jxb/ern243
Zechmann, B., and Müller, M. (2010). Subcellular compartmentation of glutathione in dicotyledonous plants. Protoplasma 246, 15–24. doi: 10.1007/s00709-010-0111-2
Zeng, A., Song, L., Cui, Y., and Yan, J. (2017). Reduced ascorbate and reduced glutathione improve embryogenesis in broccoli microspore culture. S. Afr. J. Bot. 109, 275–280. doi: 10.1016/j.sajb.2017.01.005
Zhang, L., Cai, X., Wu, J., Liu, M., Grob, S., Cheng, F., et al. (2018). Improved Brassica rapa reference genome by single-molecule sequencing and chromosome conformation capture technologies. Hortic. Res. 5:50. doi: 10.1038/s41438-018-0071-9
Zhang, X., Zhang, Z., and Chen, X.-L. (2021). The redox proteome of thiol proteins in the rice blast fungus Magnaporthe oryzae. Front. Microbiol. 12:278. doi: 10.3389/fmicb.2021.648894
Zhou, H., Finkemeier, I., Guan, W., Tossounian, M.-A., Wei, B., Young, D., et al. (2018). Oxidative stress-triggered interactions between the succinyl-and acetyl-proteomes of rice leaves. Plant Cell Environ. 41, 1139–1153. doi: 10.1111/pce.13100
Zieliński, K., Dubas, E., Gerši, Z., Krzewska, M., Janas, A., Nowicka, A., et al. (2021). β-1,3-Glucanases and chitinases participate in the stress-related defence mechanisms that are possibly connected with modulation of arabinogalactan proteins (AGP) required for the androgenesis initiation in rye (Secale cereale L.). Plant Sci. 302:110700. doi: 10.1016/j.plantsci.2020.110700
Zieliński, K., Krzewska, M., Nowicka, A., Zur, I., Fodor, J.E., et al. (2018). “Redox regulation of androgenesis in rye (Secale cereale L.),” in Proceedings of the 11th International Conference “Plant Functioning under Environmental Stress,” eds M. T. Grzesiak, and T. Hura (Krakow), 82.
Zieliński, K., Krzewska, M., Zur, I., Juzoń, K., Kopeć, P., Nowicka, A., et al. (2020). The effect of glutathione and mannitol on androgenesis in anther and isolated microspore cultures of rye (Secale cereale L.). Plant Cell Tiss. Org. 140, 577–592. doi: 10.1007/s11240-019-01754-9
Zieliński, K., Zur, I., Moravčíková, J., and Dubas, E. (2019). “The most important molecular markers related to somatic embryogenesis (SE) with the special focus on β-1, 3-glucanases and chitinases,” in Plant Functioning Under Environmental Stress, eds M. Grzesiak, A. Rzepka, T. Hura, and S. Grzesiak (Krakow: The Franciszek Górski Institute of Plant Physiology Polish Academy of Sciences), 70–75.
Żur, I., Dubas, E., Golemiec, E., Szechyńska-Hebda, M., Gołebiowska, G., and Wedzony, M. (2009). Stress-related variation in antioxidative enzymes activity and cell metabolism efficiency associated with embryogenesis induction in isolated microspore culture of triticale (x Triticosecale Wittm.). Plant Cell Rep. 28, 1279–1287. doi: 10.1007/s00299-009-0730-2
Żur, I., Dubas, E., Golemiec, E., Szechyńska-Hebda, M., Janowiak, F., and Wedzony, M. (2008). Stress-induced changes important for effective androgenic induction in isolated microspore culture of triticale (× Triticosecale Wittm.). Plant Cell Tiss. Org. Cult. 94, 319–328. doi: 10.1007/s11240-008-9360-6
Żur, I., Dubas, E., Krzewska, M., and Janowiak, F. (2015b). Current insights into hormonal regulation of microspore embryogenesis. Front. Plant Sci. 6:424. doi: 10.3389/fpls.2015.00424
Żur, I., Dubas, E., Krzewska, M., Janowiak, F., Hura, K., Pociecha, E., et al. (2014a). Antioxidant activity and ROS tolerance in triticale (x Triticosecale Wittm.) anthers affect the efficiency of microspore embryogenesis. Plant Cell Tiss. Org. Cult. 119, 79–94. doi: 10.1007/s11240-014-0515-3
Żur, I., Dubas, E., Krzewska, M., Kopeć, P., Nowicka, A., Surówka, E., et al. (2021). Triticale and barley microspore embryogenesis induction requires both reactive oxygen species generation and efficient system of antioxidative defence. Plant Cell Tiss. Org. Cult. 145, 347–366. doi: 10.1007/s11240-021-02012-7
Żur, I., Dubas, E., Krzewska, M., Sanchez-Diaz, R. A., Castillo, A. M., and Valles, M. P. (2014b). Changes in gene expression patterns associated with microspore embryogenesis in hexaploid triticale (x Triticosecale Wittm.). Plant Cell Tiss. Org. Cult. 116, 261–267. doi: 10.1007/s11240-013-0399-7
Żur, I., Dubas, E., Krzewska, M., Waligórski, P., Dziurka, M., and Janowiak, F. (2015a). Hormonal requirements for effective induction of microspore embryogenesis in triticale (x Triticosecale Wittm.) anther cultures. Plant Cell Rep. 34, 47–62. doi: 10.1007/s00299-014-1686-4
Żur, I., Dubas, E., Krzewska, M., Zieliński, K., Fodor, J., and Janowiak, F. (2019). Glutathione provides antioxidative defence and promotes microspore-derived embryo development in isolated microspore cultures of triticale (x Triticosecale Wittm.). Plant Cell Rep. 38, 195–209. doi: 10.1007/s00299-018-2362-x
Żur, I., Gołebiowska, G., Dubas, E., Golemiec, E., Matušíková, I., Libantová, J., et al. (2013). β-1,3-glucanase and chitinase activities in winter triticales during cold hardening and subsequent infection by Microdochium nivale. Biologia 68, 241–248. doi: 10.2478/s11756-013-0001-0
Żur, I., Krzewska, M., Dubas, E., Gołebiowska-Pikania, G., Janowiak, F., and Stojałowski, S. (2012). Molecular mapping of loci associated with abscisic acid accumulation in triticale (x Triticosecale Wittm.) anthers in response to low temperature stress inducing androgenic development. Plant Growth Regul. 68, 483–492. doi: 10.1007/s10725-012-9738-7
Keywords: double haploids, microspore embryogenesis (ME), pathogenesis-related protein (PR), small signaling peptides, stress
Citation: Dubas E, Żur I, Moravčiková J, Fodor J, Krzewska M, Surówka E, Nowicka A and Gerši Z (2021) Proteins, Small Peptides and Other Signaling Molecules Identified as Inconspicuous but Possibly Important Players in Microspores Reprogramming Toward Embryogenesis. Front. Sustain. Food Syst. 5:745865. doi: 10.3389/fsufs.2021.745865
Received: 22 July 2021; Accepted: 17 September 2021;
Published: 21 October 2021.
Edited by:
Ravinder K. Goyal, Agriculture and Agri-Food Canada (AAFC), CanadaReviewed by:
John Laurie, Agriculture and Agri-Food Canada, CanadaPatricia Corral-Martínez, Polytechnic University of Valencia, Spain
Copyright © 2021 Dubas, Żur, Moravčiková, Fodor, Krzewska, Surówka, Nowicka and Gerši. This is an open-access article distributed under the terms of the Creative Commons Attribution License (CC BY). The use, distribution or reproduction in other forums is permitted, provided the original author(s) and the copyright owner(s) are credited and that the original publication in this journal is cited, in accordance with accepted academic practice. No use, distribution or reproduction is permitted which does not comply with these terms.
*Correspondence: Ewa Dubas, ZS5kdWJhcyYjeDAwMDQwO2lmci1wYW4uZWR1LnBs; Iwona Żur, aS56dXImI3gwMDA0MDtpZnItcGFuLmVkdS5wbA==