- 1Corporación Colombiana de Investigación Agropecuaria—Agrosavia, C.I. Tibaitatá, Mosquera, Colombia
- 2Corporación Colombiana de Investigación Agropecuaria—Agrosavia, C.I. Obonuco, Pasto, Colombia
- 3Departamento de Ciência do Solo, Universidade de São Paulo, Escola Superior de Agricultura “Luiz de Queiroz”, Piracicaba, Brazil
The intercropping of ryegrass and red clover constitutes a sustainable alternative to mitigate the adverse effects of intensive livestock production on grassland degradation by increasing forage yield and quality. The implementation of biofertilization technologies has been widely used to improve soil nutritional properties, and therefore has the potential to ensure the success of this multicrop system. To determine the impact of bioaugmentation on forage growth and quality, as well as the associate changes in the rhizosphere bacterial community, we evaluated the inoculation with two plant growth-promoting bacteria (PGPB) under reduced nitrogen usage. Overall, Herbaspirillum sp. AP21 had a larger effect than Azospirillum brasilense D7 on plant growth. Inoculation with Herbaspirillum sp. AP21 together with 50% of the required nitrogen rate increased shoot dry weight, crude protein, and shoot nitrogen content, and decreased the amount of neutral detergent fiber. PGPB inoculation changed the rhizosphere bacterial community structure, which associated with forage growth and quality. We conclude that PGPB inoculation has the potential to improve the growth of the ryegrass-red clover system, decreasing the requirements for nitrogen fertilization.
Introduction
The growing demand for livestock is progressively resulting in grassland degradation and environmental pollution. Therefore, the pressing challenge is to maintain or increase livestock production, while achieving greater environmental and economical sustainability. This sustainability can be achieved by introducing practices that result in the reduction of soil erosion, nutrient deficiency, weed encroachment, and desertification (Sattari et al., 2016). One of the potential solutions to mitigate the negative environmental impact of intensive livestock production is the implementation of intercropping systems, as the introduction of forage legume crops in grass pasture production systems not only improves soil fertility but also allows the efficient use of water and nutrients, especially under drought conditions (Bi et al., 2019). Grass-legume intercropping systems increase the productivity and persistence of pastures compared to grass monocultures (Elgersma and Søegaard, 2018). Nonetheless, the grass-legume mixture is severely affected by two environmental factors: drought and soil nutrient availability, especially nitrogen (N) and phosphorus (Yang et al., 2020).
The association of perennial ryegrass (Lolium perenne L.) and red clover (Trifolium pratense L.) represents an example of a successful grass—legume intercropping (Hammelehle et al., 2018). Red clover increases pasture yield, as well as N use efficiency by ruminants due to its nutritional composition (Eriksen et al., 2014). In addition, legumes can derive a portion of their N requirements from the atmosphere through symbiotic biological N fixation. This process influences N dynamics in soil that can be extended to grass through a process known as “N transfer” between the legume and the grass, relying on the N exudation rate from the legume and its absorption by the grass (McElroy et al., 2016). The benefits of legume-grass intercropping can be further optimized using efficient plant growth-promoting bacteria (PGPB), which allows reducing the application rates of N fertilizers (Liu and Ludewig, 2020).
The PGPB are a group of microorganisms that improve plant growth by a variety of mechanisms such as symbiotic and associative N fixation, nutrient solubilization and mineralization (e.g., phosphorus, potassium, zinc), synthesis of phytohormones (e.g., indoles, gibberellins, and cytokinins), production of 1-aminocyclopropane-1-carboxylic acid (ACC) deaminase, synthesis of volatile organic compounds, and induction of osmolyte accumulation, among others (Ramakrishna et al., 2019; Moreno-Galván et al., 2020; Romero-Perdomo et al., 2021). A well-known facultative endophytic bacterial genus with PGPB traits is Azospirillum (Cassán et al., 2020). Its inoculation on forage grasses, for instance, has shown to minimize soil degradation risks while improving mass forage production (Boddey et al., 2003). The use of Azospirillum allows reducing the amounts of N-fertilizers applied without compromising important yield components, such as dry matter and plant height (Pankievicz et al., 2015; Leite et al., 2019). Another genus of endophytic bacteria capable of asimbiotically fixing N and producing phytohormones is Herbaspirillum (Baldani et al., 2014). This genus has demonstrated biofertilizer potential on crops, such as rice, corn, and sugarcane (Afzal et al., 2019). Likewise, Herbaspirillum, can increase the biomass production of pastures in low-fertility soils without using N fertilization (Marques et al., 2017). Inoculation with Herbaspirillum also increased the biomass of Miscanthus sinensis and perennial ryegrass plants (Straub et al., 2013; Cortés-Patiño et al., 2021).
Previously, we demonstrated the PGPB potential of two endophytes to promote the growth of both perennial ryegrass and red clover under drought and phosphorus limitation (Cortés-Patiño et al., 2021; Santos-Torres et al., 2021). Here, we further explored the PGPB potential of these two bacterial endophytes to facilitate the growth of an intercropping system conformed by both perennial ryegrass and red clover. We assessed the impact of their inoculation on the growth and quality of the system, and their influence on the diversity of the rhizosphere bacterial community.
Materials and Methods
Bacterial Strains and Inoculants
The PGPB strains Herbaspirillum sp. AP21 (SAMN15498633) and Azospirillum brasilense D7 (SAMN16830199) were provided by the Microorganism Germoplasm Collection of Agrosavia, Colombia. These strains were previously identified by whole genome sequencing and were characterized as facultative endophytes (Cortés-Patiño et al., 2021). For routine experiments, these strains were grown in DYGS culture medium, incubated at 30°C, and agitated at 140 rpm for 48 h (Baldani et al., 2014). For the greenhouse experiment, each bacterial suspension was homogenized at OD600 = 0.200 and washed twice in a sterile saline solution (8.5 g L−1 NaCl) to obtain bacterial suspensions of ~109 CFU mL−1.
Evaluation of Plant Growth-Promoting Ability Under Reduced Rates of N Fertilizer
The greenhouse experiments were carried out at the C.I. Tibaitatá of Agrosavia in Mosquera, Cundinamarca, Colombia (4°41′43.6″ N latitude, 74°12′19.9″ W longitude; 2,600 m above sea level). A completely randomized experimental design was used in a factorial arrangement (2 × 4) with ten treatments, three replicates, and three independent experiments. The first factor evaluated was N fertilization (50% and 75%) using urea as the N source. The second factor was PGPB application: without inoculation, Herbaspirillum sp. AP21, Azospirillum brasilense D7, and Herbaspirillum sp. AP21 + Azospirillum brasilense D7. Additionally, two fertilization controls without PGPB inoculation were included: 0% N and 100% N, totalizing 10 treatments (Supplementary Table 1). Pots with 3 kg of non-sterilized soil were used for the experiment. This soil is classified as Vitric Haplustands-AMBc and belongs to the Andisol order (Soil Science Division Staff, 2017). Its attributes are the following: pH 5.90, organic matter 53.30 g kg−1, effective cation exchange coefficient 11.97 cmol kg−1, P 5.00 mg kg−1, Ca 8.71 cmol kg−1, Mg 1.65 cmol kg−1, K 1.38 cmol kg−1, and Na 0.24 cmol kg−1. Soil was maintained at field capacity of 80% during the experiment. Perennial ryegrass (Lolium perenne L) var. One 50 and red clover (Trifolium pratense L.) plants were sown in each pot with a planting rate of 60:40 grass-legume (Supplementary Figure 1). Based on the physicochemical characteristics of the soil and the conventional fertilization rates used in the field [urea (150 kg ha−1), diammonium phosphate (DAP) (100 kg ha−1), and KCl (180 kg ha−1)], 5 mL of a solution with the following composition was applied per pot: 31.30 mg L−1 of urea, 80 mg L−1 of DAP, and 25 mg L−1 of KCl. The DAP fertilization was carried out one day before sowing; urea and KCl were applied once the seeds germinated. The bacterial suspensions, or the sterile saline solution for the uninoculated control, were applied directly to the soil surrounding the plant (10 mL pot−1). Plant cutting (8 cm high) was performed every 40 days after sowing.
Plant Tissue Analysis
The experiment was carried out for 120 days. The intercropping system was inoculated on days 0 and 20 after sowing, and cuts were performed two times before collecting samples for analysis. Plant tissues were oven-dried at 60°C for 48 h and shoot dry weight was recorded. The plant material was then ground in a grinder (Wiley Mill, USA) equipped with a mesh wire of a 1 mm opening. The ground material was used to determine the concentration of crude protein, neutral detergent fiber (NDF), and N content as nutritional quality indicators. NDF and crude protein were determined as described by Silva et al. (2009). Nitrogen concentration in shoot tissue (shoot N content) was determined by acid digestion (sulfuric acid) using a semi micro-Kjeldahl distiller Kjeltec™ 8100 (FOSS, Denmark) (Silva et al., 2016).
High-Throughput Sequencing of the Soil Bacterial Community
Rhizosphere soil was sampled from each pot, mixed between replicates, and homogenized per treatment and experiment. Rhizosphere was defined as the soil strongly adhered to the root (around 2 mm) that remained attached after vigorous shaking and crumbling. Following harvesting, the sampled soil was mixed and homogenized in a clean plastic bag. A subsample of each treatment per independent experiment (3 replicates) was placed into 10 mL transfer tubes and stored to −80°C for further analysis. Total DNA extraction from soil was performed using the DNeasy PowerSoil® Kit (Qiagen, Germany) following the manufacturer's instructions. The quality and integrity of the DNA was verified on a 1% agarose gel, and its concentration and purity were quantified utilizing a NanoDrop™ 2000/2000c (ThermoScientific, USA). For construction of the genomic libraries, a first PCR of the V3-V4 region was performed using the primers 16S rRNA-515F and 16S rRNA-806R, modified with pre-adapters (Pacheco-Montealegre et al., 2020). Platinum ™Taq DNA Polymerase (Invitrogen, UK) was used for amplification following the PCR conditions: Buffer (1X), MgCl2 (1.5 mM), dNTPs (0.2 mM), primers (0.2 mM), and Taq (1U). The PCR program was performed in an Eppendorf® thermocycler (Eppendorf, Germany) as follows: 94°C for 120 s; denaturation: 94°C for 45 s; annealing: 50°C for 60 s, and extension: 72°C for 90 s for 30 cycles; final extension: 72°C for 10 min (Pacheco-Montealegre et al., 2020). The PCR products were purified using the Agentcourt® Ampure® XP (Beckman Coulter, USA). The second-round of amplification was performed to include an inline barcode and an Illumina adaptor. For each sample, 2 μL of first-round PCR were used, and the same conditions described above were used for PCR, but only for 12 cycles. The amplicon was again cleaned using Agentcourt® Ampure® XP (Beckman Coulter, USA) (Quail et al., 2009). All samples were mixed into a single batch of V3-V4 region-amplicon pools and quantified using a Qubit Fluorometer (Thermofisher, USA) employing the Quant-iT dsDNA HS Assay Kit (Thermofisher, USA) and sequenced in the Illumina MiSeq plataform at the Microbial Genomics Laboratory of the Molecular Genetics and Antimicrobial Resistance Unit of Universidad El Bosque, Bogota, Colombia. The sequencing data were deposited in the NCBI Archive under Bioproject PRJNA627728.
Data Analyses
ANOVA (p < 0.05) and Duncan's test were employed to analyze the plant data and the alfa diversity index. These analyses were performed using IBM SPSS Statistics 22.0.0.0 (IBM, USA) and GraphPad Prism 8 (GraphPad Software, USA).
For the analysis of the rhizosphere bacterial community, reads from 16s rRNA V3-V4 were analyzed using Qiime2 v2019-7 (Bolyen et al., 2019). Quality control and denoise were performed with DADA2 pipeline (Callahan et al., 2016), retaining reads with a length of at least 220 base pairs (forward and reverse reads). Analyses were performed determining the sampling depth as the maximum number of sequences per treatment, obtaining the rarefaction curves (Supplementary Figure 2), and using the median of the number of sequences in the treatments. An optimal number of representative or non-chimeric sequences obtained after cleaning and cutting were determined to normalize the readings for subsequent analyses. In this case, 3,197 operational taxonomic units were obtained. The taxonomic classification was performed using the qiime2 plugin q2-feature-classifier based on a pre-trained naive Bayes classifier and the reference database Greengenes version 13.8 (http://greengenes.lbl.gov).
Alpha and beta diversity analyses were estimated using the R packages: Phyloseq version 1.30 (McMurdie and Holmes, 2013), qiime2R version 0.99.13” (Bisanz, 2019), and dplyr version 1.0.3 (Wickham et al., 2020). For alpha diversity, the Shannon-Weiner index was calculated for observed sequences without rarefying microbial community data, and the interpretation was made according to McMurdie and Holmes (2014). The effect of inoculation and N application on the total structure of the soil bacterial community was assessed by a permutational multivariate analysis of variance (PERMANOVA) (Anderson, 2001) employing the Bray-Curtis distances with 9,999 permutations. A distance-based redundancy analysis (db-RDA) of the Bray-Curtis dissimilarity matrices using the “vegan” package was performed to identify the main plant parameters related to changes in bacterial community structure (Oksanen et al., 2019).
Results
Inoculation With the Endophytes Improves Forage Growth Under Reduced N Rates
We established a greenhouse experiment on non-sterile soil, using single and mixed microbial inoculations, as well as reduced N fertilization rates, to assess the potential of Herbaspirillum sp. AP21 and Azospirillum brasilense D7 to improve the growth and quality of the ryegrass—red clover intercropping system. The system was inoculated 0 and 20 days after sowing, and samples were collected after 120 days (Figure 1A). In this experimental setting, nodulation was observed in all treatments. Shoot dry weight ranged between ~32 and ~40 g pot−1 without inoculation, and plant biomass gradually increased with the fertilization rate (Figure 1B). At 50% N and 75% N, for instance, the plant biomass was ~34 g pot−1 and ~37 g pot−1, respectively, validating our experimental setup. We observed that at 50% N, inoculation had a positive effect on shoot biomass. At this concentration, the largest effect was observed with AP21 and AP21 + D7, followed by D7 and the uninoculated control (Figure 1B). The present data thus suggests that AP21 has a larger potential than D7 to facilitate the growth of the intercropping system. Notably, inoculation with AP21 and AP21 + D7 at 50% N fertilization resulted in comparable plant biomass to the 100% N control. This positive effect, however, largely disappeared when the rate of N fertilization used was 75%.
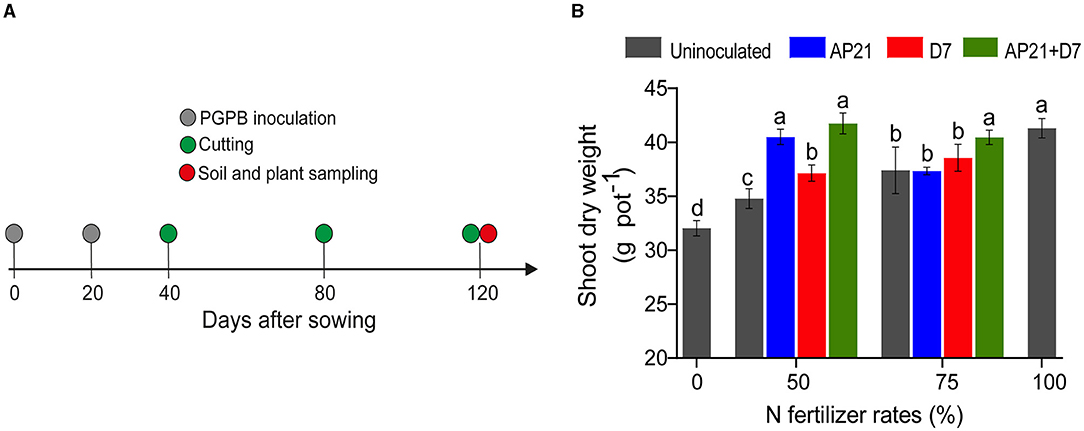
Figure 1. Timeline of the experiment (A). Effect of the inoculation of the strains Herbaspirillum sp. AP21, Azospirillum brasilense D7, and their co-inoculation with different nitrogen fertilization rates on plant biomass (B). An untreated treatment was included at all fertilization doses as a control for inoculation. Error bars represent ± standard deviation. Different letters indicate significant differences based on Duncan's test (p < 0.05). Each value is the mean of three blocks with three replicates per treatment.
Inoculation With Herbaspirillum sp. AP21 Results in Increased Forage Quality
We next explored the impact of both strains at the different N fertilization doses on forage quality. For this, three different parameters were assessed: crude protein, shoot N content, and neutral detergent fiber (NDF). Inoculation with AP21 led to a dramatic increase in protein content, which phenocopied the 100% N control (Figure 2A). By contrast, the effect of D7 was subtle, and no differences were seen compared with the co-inoculation. The effects due to bacterial inoculation fully disappeared when the N fertilization rate was increased to 75%. Overall, shoot N content and protein content exhibited a similar pattern. As seen before, a positive impact due to inoculation was only seen at 50% N fertilization (Figures 2A,B). The largest increase in shoot N content was also observed when AP21 was used at 50% N fertilization and resembled the N content of the 100% N control (Figure 2B). Finally, we assessed the content of NDF, which is associated with the digestibility of fiber. Smaller values of NDF indicate increased digestibility, and therefore superior forage quality. Noteworthy, the N fertilization rate did not affect NDF without inoculation (Figure 2C). At 50% N fertilization, inoculation with either AP21 or D7 resulted in a significant decrease in NDF; to our surprise, however, the co-inoculation led to opposite results. At 75% N fertilization, AP21 reduced NDF, while inoculation with D7 or AP21 + D7 only showed a slight increase compared to the control treatment.
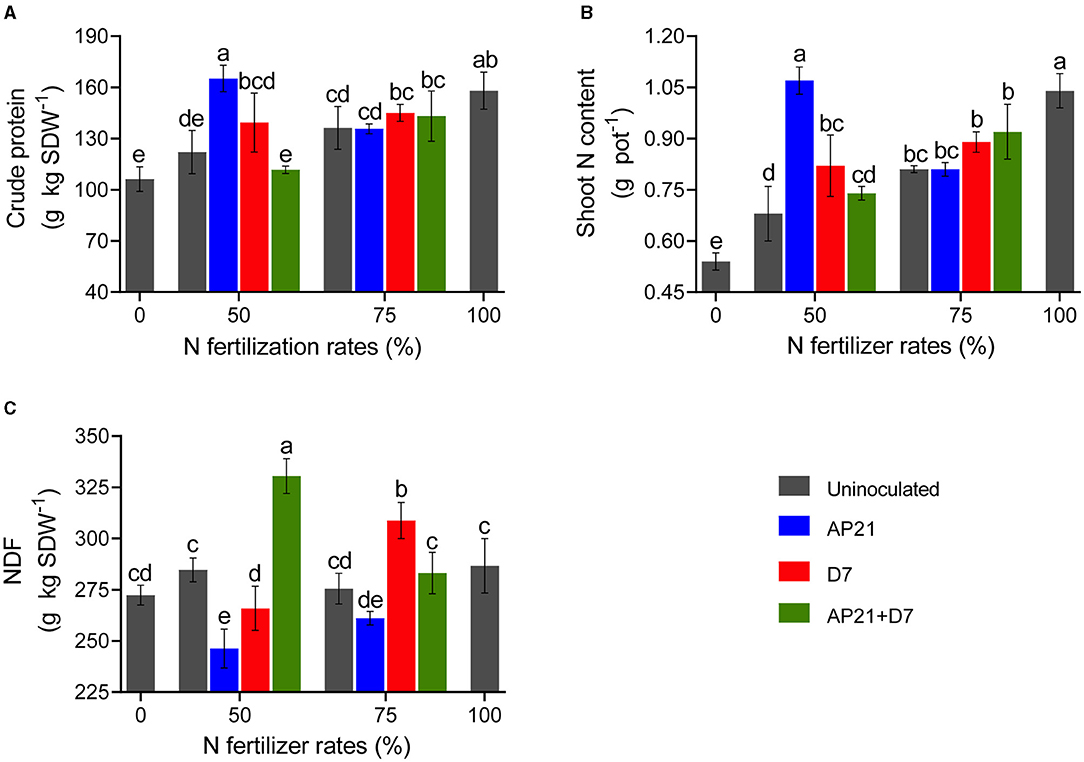
Figure 2. Effect of the inoculation on bromatological parameters: crude protein (A), shoot N content (B), neutral detergent fiber (NDF) (C). Error bars represent ± standard deviation. Different letters indicate significant differences based on Duncan's test (p < 0.05). Each value is the mean of three blocks with three replicates per treatment.
Inoculation With the Facultative Endophytes Impacts the Rhizosphere Bacterial Community
Next, we sought to determine how inoculation impacted the bacterial diversity of the plant's rhizosphere by using 16S rRNA metataxonomics. Results showed that the bacterial diversity (alpha-diversity), measured by the Shannon-Weiner diversity index, responded differently between the inoculated and uninoculated treatments. Inoculation slightly decreased the alpha diversity in comparison with the uninoculated treatments at 50% N fertilization rate. Likewise, at the 75% N dose, the treatments inoculated with D7 presented a lower alpha diversity (p < 0.05) compared with the uninoculated treatment. Altogether, these results suggest that the application of the PGPB alters the bacterial community associated with the rhizosphere, with an overall decrease in bacterial diversity (Figure 3A).
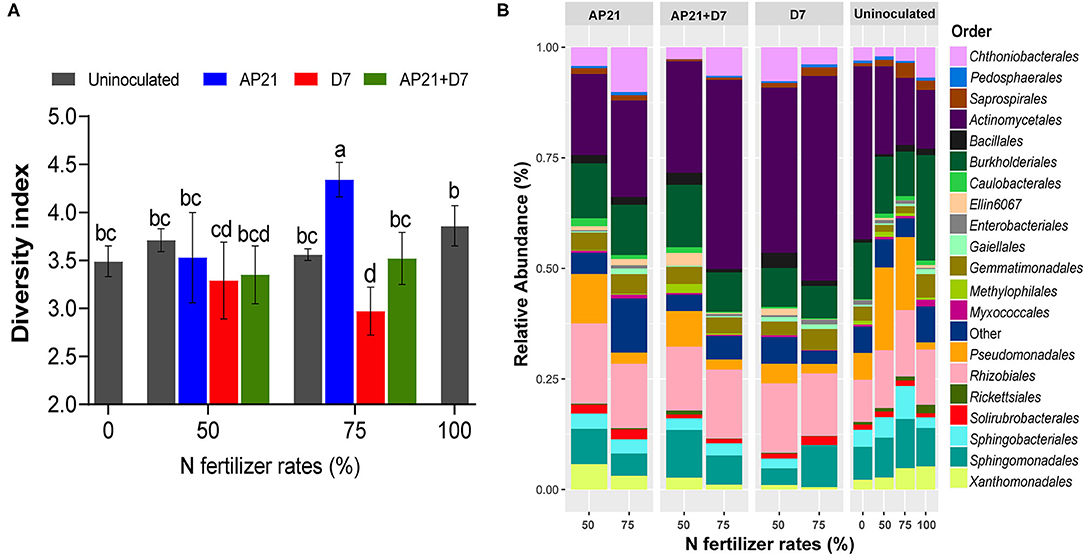
Figure 3. Variations in the Shannon-Weiner diversity index (A) and relative abundance of bacterial orders (B) in the rhizosphere for inoculation with Herbaspirillum sp. AP21, Azospirillum brasilense D7, and co-inoculation with Herbaspirillum sp. AP21 + Azospirillum brasilense D7 under different rates of nitrogen fertilization. Different letters indicate significant differences based on Duncan's test (p < 0.05). Each value is the mean of three replicates per treatment.
The distribution of the 20 most abundant orders was studied. In average, the predominant orders found in the treatments were: Actinomycetales (28.8%), Rhizobiales (13.5%), Burkholderiales (12.7%), Pseudomonadales (7.7%), Sphingomonadales (7.5%), Gemmatimonadales (3.5%), Sphingobacteriales (2.9%), and Xanthomonadales (2.9%) (Figure 3B). At the family level the most predominant families were Micrococcaceae (24.5%), Pseudomonadaceae (8.3%), Sphingomonadaceae (8,0%), Comamonadaceae (7.7%), Chthoniobacteraceae (6.0%), Oxalobacteraceae (5.8%), Hyphomicrobiaceae (5.3%), Bradyrhizobiaceae (5.1%), and Rhizobiaceae (3.1%) (Supplementary Figure 3). To evaluate the impact of the treatments on the structure of the rhizosphere bacterial community, a multivariate statistical analysis (PERMANOVA) was used (Table 1). Interestingly, the inoculation with AP21 and D7, regardless of the N rate, changed the structure of the bacterial community of the rhizosphere (p < 0.05). We did not observe significant changes in the bacterial community due to N fertilization.
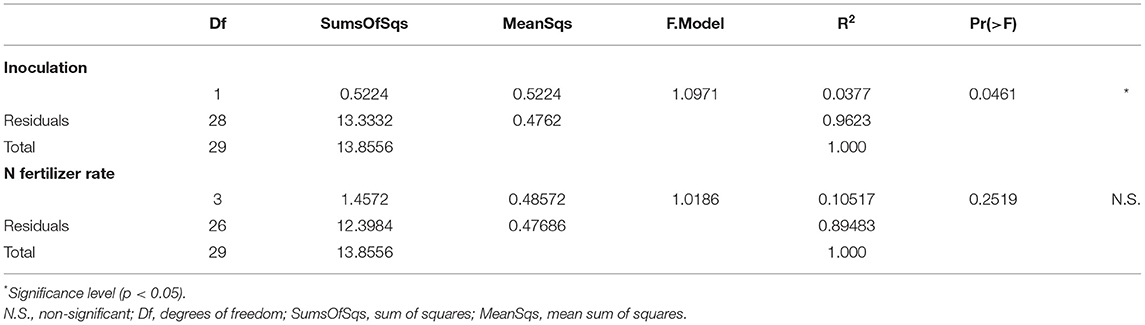
Table 1. Effects of PGPB inoculation and N fertilizer rate on the bacterial community structure expressed as R and F values obtained from a one-way PERMANOVA.
Relationship Between Rhizosphere Bacteriome, and Forage Growth and Quality
To understand how plant growth and quality correlated with the observed changes in bacterial diversity, we carried out a distance-based redundancy analysis. The db-RDA explained 53.33% of the diversity variation, where db-RDA1 accounted for 28.21% and db-RDA2 explained 25.12% of the total variance. The complete model illustrated the relationship between the variables associated with plant and bacterial diversity (p < 0.05). Besides, the angle in db-RDA included between the arrows pointing at two variables determined the correlation between the parameters: sharp angles defined positive correlations, orthogonal angles defined no correlations, and obtuse angles defined negative correlations (Figure 4; Carvalho-Estrada et al., 2020). Crude protein and shoot N content were the closest correlation parameters, followed by shoot N content, and shoot dry weight. Furthermore, we observed a negative correlation between NDF and crude protein parameters. The treatments inoculated with AP21, D7, and AP21 + D7 fertilized with 50 and 75% N formed a cluster with the positive control treatment fertilized with 100% N. This cluster was positively associated with crude protein, shoot N content, and shoot dry weight increments.
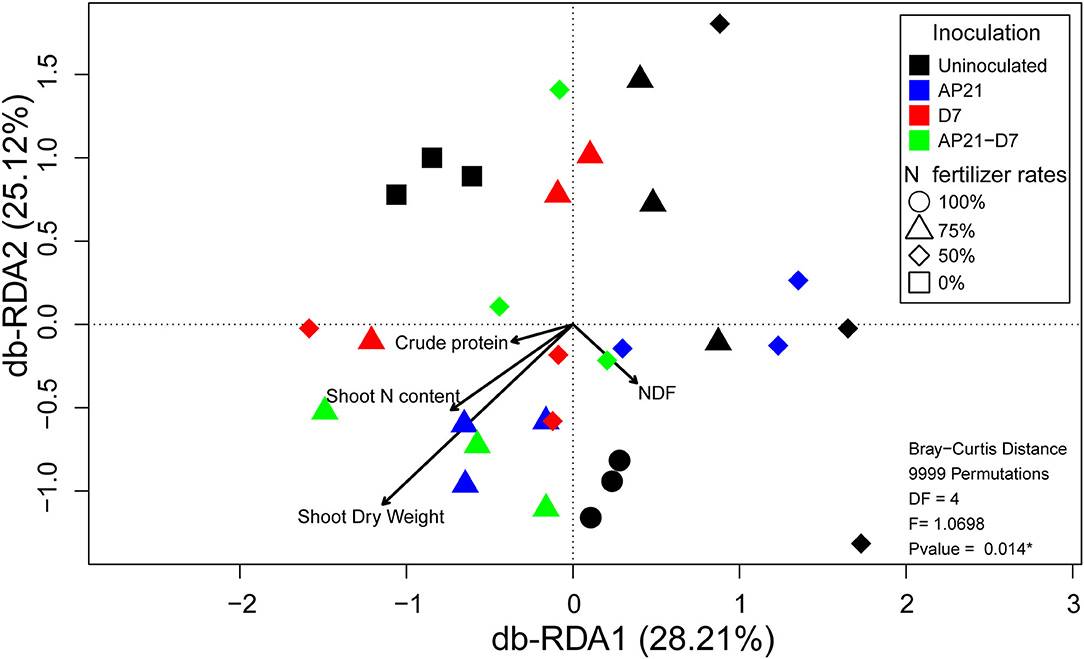
Figure 4. Distance-based redundancy analysis (db-RDA) plot illustrating the bacterial community structure of the rhizosphere in relation to plant parameters. The db-RDA was significant (p < 0.05).
Discussion
The implementation of technologies based on the use of PGPB has the potential to improve the environmental and economic sustainability of livestock, via an increase of forage biomass and quality (Chen et al., 2020; Santos-Torres et al., 2021). In this study, we described the potential of two endophytic strains to promote the growth and improve the quality of an intercropping system conformed by perennial ryegrass and red clover (Figure 5). Our rationale was that inoculation with the facultative endophytes had the potential to improve plant biomass and quality by directly stimulating plant growth or synergistically interacting with the native microbiota.
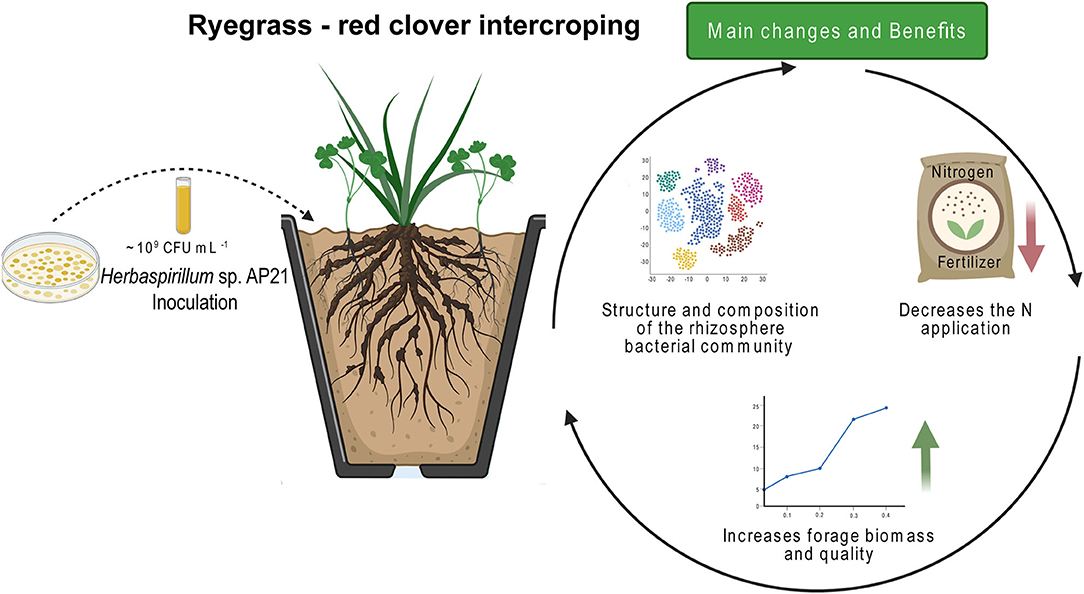
Figure 5. Inoculation of the intercropping system with Herbaspirillum sp. AP21 brought about changes in the microbial community, which positively correlated with an increase in plant growth and quality. The concomitant use of AP21 and 50% of the fertilization doses phenocopied the complete fertilization control. The use of this technology thus reduces the use of N fertilizers, improving the economical and environmental sustainability of the system.
The effect of microbial inoculation on plant growth was only seen when the N fertilization dose was 50%. Plant nutritional stress thus determines the potential of these microbes to promote growth. Since all plants were nodulated over the course of the experiment with native rhizobia, this implies that the incorporated microorganisms account for the observed differences. Yet, whether these inoculants improve the N fixation process itself or result in a better establishment of the symbiotic interaction legume-rhizobia remains to be determined. The positive effect of rhizosphere microorganisms to support plant growth under suboptimal growth conditions has been noted previously (Rojas-Tapias et al., 2012, 2014; Brisson et al., 2019). The ACC deaminase activity, for instance, has been strongly associated with the capacity of some microbes to facilitate plant growth under stressful conditions. The specific relation between plant nutritional stress and the plant growth potential of these microbes highlights the importance of this technology to improve forage growth and quality in environments that impose stressful conditions.
Inoculation with bacteria reduced the requirement of N and improved both biomass and quality. Inoculation with AP21 at 50% N, for instance, phenocopied the 100% N chemical control in terms of biomass, total N content, and crude protein. This positive effect of the inoculants is likely to be a direct consequence of microbial N fixation, as both microbes are diazotrophs, or an indirect effect due to their capacity to synthesize phytohormones or produce ACC deaminase activity (Cortés-Patiño et al., 2021). The positive effect of these microbes on plant growth and nutrition has been previously seen in other crops. Inoculation with Azospirillum on maize, for instance, has shown to increase grain yield compared with urea fertilization (Martins et al., 2018). In field conditions, Azospirillum inoculation increased corn and wheat yield by 27% and 31%, respectively, compared to the non-inoculated control (Hungria et al., 2010). Inoculation of rice plants with Herbaspirillum also showed the potential of this microbe to improve N acquisition under phosphorus limitation (Estrada et al., 2013). Likewise, Herbaspirillum inoculation with 50% of the N dose in maize crop produced yields comparable to the treatment with 100% N fertilization and increased grain quality (Alves et al., 2014).
NDF values were also affected by inoculation with bacteria. NDF is a quality parameter related to hemicellulose, cellulose, and lignin representing the entire fibrous part of the forage, which associates with increased digestibility (Raffrenato et al., 2017; Hristov et al., 2020). AP21 reduced the levels of NDF and increased the biomass and N content. Co-inoculation, to our surprise, resulted in reduced digestibility, but had a positive effect on growth. At this point, however, it is uncertain how inoculation affects NDF, since co-inoculation of AP21 and D7 resulted in opposite effects. The positive effect of various PGPB on the nutrient and dry matter content of different pastures (e.g., ryegrass, Brachiaria, and Mischantus) and legumes (e.g., white clover) has been reported (Zaman et al., 2016; Marques et al., 2017; Akinrinlola et al., 2018; Leite et al., 2019; Liu and Ludewig, 2020).
Facultative endophytes have the potential to modulate the growth and quality of the forage by acting within the inner plant tissue, and by acting on the rhizosphere (Duarte et al., 2020). This effect can be achieved by directly facilitating plant growth and development, or by modulating the microbial structure and diversity of soil (Wang et al., 2021). We observed a decrease in microbial diversity upon inoculation with both microbes. This effect is likely a direct result of the addition of AP21 and D7 as inoculants. This shift indeed correlated with an improvement in the growth and quality of the intercropping system, as seen in other studies (Wang et al., 2018; Zhong et al., 2019; Estrada-Bonilla et al., 2021). The capacity of these microbes to colonize the plant tissue (Cortés-Patiño et al., 2021), potentially ensures that the mutualist relationship between microbes and forage takes place, regardless of those changes. Moreover, the fertilization doses had no effect on the diversity of the bacterial community, highlighting the importance of the plant genotype on the composition of the plant microbiome (Chen et al., 2019).
Collectively, inoculation with Herbaspirillum sp. AP21, and Azospirillum brasilense D7 at a minor extent, reduced the requirements for N fertilization and improved plant quality, which concomitantly occurred with changes in the rhizosphere bacterial community. Inoculation with AP21 at 50% N fertilization, for instance, phenocopied the complete fertilization control, thereby suggesting that an equivalent to 75 kg of the N-fertilizer ha−1 can potentially be substituted in this system by the implementation of this sustainable technology. Interestingly, the microbial inoculants had no effect on plant growth when higher doses of N fertilization were used, suggesting that stressed plants are more responsive to the positive effects of microbial inoculation. Localization and functional studies are therefore needed to understand in detail the mechanisms involved. The implementation of these ecofriendly technologies has thus the potential to reduce the implementation of N fertilizers and can potentially be used as a tool for the restoration of deteriorated agricultural soils.
Data Availability Statement
The datasets presented in this study can be found in online repositories. The names of the repository/repositories and accession number(s) can be found below: https://www.ncbi.nlm.nih.gov/bioproject/?term=SAMN15498633, https://www.ncbi.nlm.nih.gov/bioproject/?term=SAMN16830199, https://www.ncbi.nlm.nih.gov/bioproject/PRJNA627728.
Author Contributions
All authors have made a direct and intellectual contribution to the manuscript. FR-P, JM-L, and SP-D performed the greenhouse experiment, sample collection and processing, and data analysis. EC-R and GE-B carried out the experimental design. SP-D, AS, and DD-D analyzed the metagenomic data. GE-B, DR-T, EC, and FR-P wrote the article. All authors contributed to the article and approved the submitted version.
Funding
This work was supported by Sistema General de Regalías de Nariño (Grant No. 2013000100279) and Ministerio de Ciencia Tecnología e Innovación (Grant agreement No. 1948-63331).
Conflict of Interest
The authors declare that the research was conducted in the absence of any commercial or financial relationships that could be construed as a potential conflict of interest.
Publisher's Note
All claims expressed in this article are solely those of the authors and do not necessarily represent those of their affiliated organizations, or those of the publisher, the editors and the reviewers. Any product that may be evaluated in this article, or claim that may be made by its manufacturer, is not guaranteed or endorsed by the publisher.
Acknowledgments
All authors are grateful to Mauricio Barón, Marilyn Santos-Torres, and Angélica Y. Gutiérrez, for their support during the greenhouse experiments, and to Lady Molano for carrying out the administrative management of this study. EC and AS would like to thank the São Paulo Research Foundation (FAPESP) (project No. 2016/18944-3, and the Ph.D. scholarship No. 2019/13436-8). The authors want to thank the Agencia Presidencial de Cooperación Internacional de Colombia (APC-Colombia) and the Agência Brasileira de Cooperação (ABC) for allowing the technical cooperation among Agrosavia and ESALQ-USP through the project “Microbial consortia and sustainable recovery of degraded soil in the southwest of Brazil and Cundinamarca-state Colombia”.
Supplementary Material
The Supplementary Material for this article can be found online at: https://www.frontiersin.org/articles/10.3389/fsufs.2021.715270/full#supplementary-material
References
Afzal, I., Shinwari, Z. K., Sikandar, S., and Shahzad, S. (2019). Plant beneficial endophytic bacteria: Mechanisms, diversity, host range and genetic determinants. Microbiol. Res. 221, 36–49. doi: 10.1016/j.micres.2019.02.001
Akinrinlola, R. J., Yuen, G. Y., Drijber, R. A., and Adesemoye, A. O. (2018). Evaluation of Bacillus strains for plant growth promotion and predictability of efficacy by in vitro physiological traits. Int. J. Microbiol. 2018:5686874. doi: 10.1155/2018/5686874
Alves, G. C., Videira, S. S., Urquiaga, S., and Reis, V. M. (2014). Differential plant growth promotion and nitrogen fixation in two genotypes of maize by several Herbaspirillum inoculants. Plant Soil 387, 307–321. doi: 10.1007/s11104-014-2295-2
Anderson, M. J. (2001). A new method for non-parametric multivariate analysis of variance. Aust. Ecol. 26, 32–46. doi: 10.1111/j.1442-9993.2001.01070.pp.x
Baldani, J. I., Reis, V. M., Videira, S. S., Boddey, L. H., and Baldani, V. L. D. (2014). The art of isolating nitrogen-fixing bacteria from non-leguminous plants using N-free semi-solid media: a practical guide for microbiologists. Plant Soil 384, 413–431. doi: 10.1007/s11104-014-2186-6
Bi, Y., Zhou, P., Li, S., Wei, Y., Xiong, X., Shi, Y., et al. (2019). Interspecific interactions contribute to higher forage yield and are affected by phosphorus application in a fully-mixed perennial legume and grass intercropping system. F. Crop. Res. 244, 107636. doi: 10.1016/j.fcr.2019.107636
Bisanz, J. E. (2019). Tutorial : Integrating QIIME2 and R for Data Visualization and Analysis Using. Available online at: https://forum.qiime2.org/t/tutorial-integrating-qiime2-and-r-for-data-visualization-and-analysis-using-qiime2r/4121 (accessed March, 2021).
Boddey, R. M., Xavier, D. F., Alves, B. J. R., and Urquiaga, S. (2003). Brazilian agriculture: The transition to sustainability. J. Crop Prod. 9, 593–621. doi: 10.1300/J144v09n01_10
Bolyen, E., Rideout, J. R., Dillon, M. R., Bokulich, N. A., Abnet, C. C., Al-Ghalith, G. A., et al. (2019). Reproducible, interactive, scalable and extensible microbiome data science using QIIME 2. Nat. Biotechnol. 37, 852–857. doi: 10.1038/s41587-019-0209-9
Brisson, V. L., Schmidt, J. E., Northen, T. R., Vogel, J. P., and Gaudin, A. C. M. (2019). Impacts of maize domestication and breeding on rhizosphere microbial community recruitment from a nutrient depleteda agricultural soil. Sci. Rep. 9:15611. doi: 10.1038/s41598-019-52148-y
Callahan, B. J., McMurdie, P. J., Rosen, M. J., Han, A. W., Johnson, A. J. A., and Holmes, S. P. (2016). DADA2: High-resolution sample inference from Illumina amplicon data. Nat. Methods 13, 581–583. doi: 10.1038/nmeth.3869
Carvalho-Estrada, P., Fernandes, J., da Silva, É. B., Tizioto, P., de Fátima Paziani, S., Duarte, A. P., et al. (2020). Effects of hybrid, kernel maturity, and storage period on the bacterial community in high-moisture and rehydrated corn grain silages. Syst. Appl. Microbiol. 43:126131. doi: 10.1016/j.syapm.2020.126131
Cassán, F., Coniglio, A., López, G., Molina, R., Nievas, S., de Carlan, C. L. N., et al. (2020). Everything you must know about Azospirillum and its impact on agriculture and beyond. Biol. Fertil. Soils 56, 461–479. doi: 10.1007/s00374-020-01463-y
Chen, L., Hao, Z., Li, K., Sha, Y., Wang, E., Sui, X., et al. (2020). Effects of growth-promoting rhizobacteria on maize growth and rhizosphere microbial community under conservation tillage in Northeast China. Microb. Biotechnol. 14, 535–550. doi: 10.1111/1751-7915.13693
Chen, S., Waghmode, T. R., Sun, R., Kuramae, E. E., Hu, C., and Liu, B. (2019). Root-associated microbiomes of wheat under the combined effect of plant development and nitrogen fertilization. Microbiome 7:136. doi: 10.1186/s40168-019-0750-2
Cortés-Patiño, S., Vargas, C., Álvarez-Flórez, F., Bonilla, R., and Estrada-Bonilla, G. (2021). Potential of Herbaspirillum and Azospirillum consortium to promote growth of perennial ryegrass under water deficit. Microorganisms 9:91. doi: 10.3390/microorganisms9010091
Duarte, C. F. D., Cecato, U., Hungria, M., Fernandes, H. J., Biserra, T. T., Galbeiro, S., et al. (2020). Morphogenetic and structural characteristics of Urochloa species under inoculation with plant-growth-promoting bacteria and nitrogen fertilisation. Crop Pasture Sci. 71:82. doi: 10.1071/CP18455
Elgersma, A., and Søegaard, K. (2018). Changes in nutritive value and herbage yield during extended growth intervals in grass-legume mixtures: effects of species, maturity at harvest, and relationships between productivity and components of feed quality. Grass Forage Sci. 73, 78–93. doi: 10.1111/gfs.12287
Eriksen, J., Askegaard, M., and Søegaard, K. (2014). Complementary effects of red clover inclusion in ryegrass-white clover swards for grazing and cutting. Grass Forage Sci. 69, 241–250. doi: 10.1111/gfs.12025
Estrada, G. A., Baldani, V. L. D., de Oliveira, D. M., Urquiaga, S., and Baldani, J. I. (2013). Selection of phosphate-solubilizing diazotrophic Herbaspirillum and Burkholderia strains and their effect on rice crop yield and nutrient uptake. Plant Soil 369, 115–129. doi: 10.1007/s11104-012-1550-7
Estrada-Bonilla, G. A., Durrer, A., and Cardoso, E. J. B. N. (2021). Use of compost and phosphate-solubilizing bacteria affect sugarcane mineral nutrition, phosphorus availability, and the soil bacterial community. Appl. Soil Ecol. 157:103760. doi: 10.1016/j.apsoil.2020.103760
Hammelehle, A., Oberson, A., Lüscher, A., Mäder, P., and Mayer, J. (2018). Above- and belowground nitrogen distribution of a red clover-perennial ryegrass sward along a soil nutrient availability gradient established by organic and conventional cropping systems. Plant Soil 425, 507–525. doi: 10.1007/s11104-018-3559-z
Hristov, A. N., Harper, M. T., Roth, G., Canale, C., Huhtanen, P., Richard, T. L., et al. (2020). Effects of ensiling time on corn silage neutral detergent fiber degradability and relationship between laboratory fiber analyses and in vivo digestibility. J. Dairy Sci. 103, 2333–2346. doi: 10.3168/jds.2019-16917
Hungria, M., Campo, R. J., Souza, E. M., and Pedrosa, F. O. (2010). Inoculation with selected strains of Azospirillum brasilense and A. lipoferum improves yields of maize and wheat in Brazil. Plant Soil 331, 413–425. doi: 10.1007/s11104-009-0262-0
Leite, R. D. C., dos Santos, J. G. D., Silva, E. L., Alves, C. R. C. R., Hungria, M., Leite, R. D. C., et al. (2019). Productivity increase, reduction of nitrogen fertiliser use and drought-stress mitigation by inoculation of Marandu grass (Urochloa brizantha) with Azospirillum brasilense. Crop Pasture Sci. 70:61. doi: 10.1071/CP18105
Liu, Y., and Ludewig, U. (2020). Improved establishment of Miscanthus × giganteus stem propagation by Herbaspirillum inoculation. Ind. Crops Prod. 150:112339. doi: 10.1016/j.indcrop.2020.112339
Marques, A. C. R., Oliveira, L. B., de, Nicoloso, F. T., Jacques, R. J. S., Giacomini, S. J., and Quadros, F. L. F. de (2017). Biological nitrogen fixation in C4 grasses of different growth strategies of South America natural grasslands. Appl. Soil Ecol. 113, 54–62. doi: 10.1016/j.apsoil.2017.01.011
Martins, M. R., Jantalia, C. P., Reis, V. M., Döwich, I., Polidoro, J. C., Alves, B. J. R., et al. (2018). Impact of plant growth-promoting bacteria on grain yield, protein content, and urea-15 N recovery by maize in a Cerrado Oxisol. Plant Soil 422, 239–250. doi: 10.1007/s11104-017-3193-1
McElroy, M., Papadopoulos, Y. A., Glover, K. E., Dong, Z., Fillmore, S. A. E., and Johnston, M. O. (2016). Interactions between cultivars of legumes species (Trifolium pratense L., Medicago sativa L.) and grasses (Phleum pratense L., Lolium perenne L.) under different nitrogen levels. Can. J. Plant Sci. 97:CJPS-2016-0130. doi: 10.1139/CJPS-2016-0130
McMurdie, P. J., and Holmes, S. (2013). phyloseq: an R package for reproducible interactive analysis and graphics of microbiome census data. PLoS ONE 8:e61217. doi: 10.1371/journal.pone.0061217
McMurdie, P. J., and Holmes, S. (2014). Waste not, want not: Why rarefying microbiome data is inadmissible. PLoS Comput. Biol. 10:e1003531. doi: 10.1371/journal.pcbi.1003531
Moreno-Galván, A., Romero-Perdomo, F. A., Estrada-Bonilla, G., Meneses, C. H. S. G., and Bonilla, R. R. (2020). Dry-Caribbean Bacillus spp. strains ameliorate drought stress in maize by a strain-specific antioxidant response modulation. Microorganisms 8:823. doi: 10.3390/microorganisms8060823
Oksanen, J., Blanchet, F. G., Friendly, M., Kindt, R., Legendre, P., Mcglinn, D., et al. (2019). Package “vegan” Title community ecology package. Community Ecol. Packag. 2, 1–297. Available online at: https://cran.r-project.org/web/packages/vegan/vegan.pdf (accessed March 2021).
Pacheco-Montealegre, M. E., Dávila-Mora, L. L., Botero-Rute, L. M., Reyes, A., and Caro-Quintero, A. (2020). Fine resolution analysis of microbial communities provides insights into the variability of cocoa bean fermentation. Front. Microbiol. 11:650. doi: 10.3389/fmicb.2020.00650
Pankievicz, V. C. S., do Amaral, F. P., Santos, K. F. D. N., Agtuca, B., Xu, Y., Schueller, M. J., et al. (2015). Robust biological nitrogen fixation in a model grass-bacterial association. Plant J. 81, 907–919. doi: 10.1111/tpj.12777
Quail, M. A., Swerdlow, H., and Turner, D. J. (2009). Improved protocols for the Illumina genome analyzer sequencing system. Curr. Protoc. Hum. Genet. 62:2. doi: 10.1002/0471142905.hg1802s62
Raffrenato, E., Fievisohn, R., Cotanch, K. W., Grant, R. J., Chase, L. E., and Van Amburgh, M. E. (2017). Effect of lignin linkages with other plant cell wall components on in vitro and in vivo neutral detergent fiber digestibility and rate of digestion of grass forages. J. Dairy Sci. 100, 8119–8131. doi: 10.3168/jds.2016-12364
Ramakrishna, W., Yadav, R., and Li, K. (2019). Plant growth promoting bacteria in agriculture: two sides of a coin. Appl. Soil Ecol. 138, 10–18. doi: 10.1016/j.apsoil.2019.02.019
Rojas-Tapias, D., Moreno-Galván, A., Pardo-Díaz, S., Obando, M., Rivera, D., and Bonilla, R. (2012). Effect of inoculation with plant growth-promoting bacteria (PGPB) on amelioration of saline stress in maize (Zea mays). Appl. Soil Ecol. 61, 264–272. doi: 10.1016/j.apsoil.2012.01.006
Rojas-Tapias, D. F., Bonilla, R., and Dussán, J. (2014). Effect of inoculation and co-inoculation of Acinetobacter sp. RG30 and Pseudomonas putida GN04 on growth, fitness, and copper accumulation of maize (Zea mays). Water Air Soil Pollut. 225:2232. doi: 10.1007/s11270-014-2232-2
Romero-Perdomo, F., Beltrán, I., Mendoza-Labrador, J., Estrada-Bonilla, G., and Bonilla, R. (2021). phosphorus nutrition and growth of cotton plants inoculated with growth-promoting bacteria under low phosphate availability. Front. Sustain. Food Syst. 4:618425. doi: 10.3389/fsufs.2020.618425
Santos-Torres, M., Romero-Perdomo, F., Mendoza-Labrador, J., Gutiérrez, A. Y., Vargas, C., Castro-Rincon, E., et al. (2021). Genomic and phenotypic analysis of rock phosphate-solubilizing rhizobacteria. Rhizosphere 17:100290. doi: 10.1016/j.rhisph.2020.100290
Sattari, S. Z., Bouwman, A. F., Martinez Rodríguez, R., Beusen, A. H. W., and van Ittersum, M. K. (2016). Negative global phosphorus budgets challenge sustainable intensification of grasslands. Nat. Commun. 7:10696. doi: 10.1038/ncomms10696
Silva, C. V., da, Lana, R., de, P., Campos, J. M. de, S., Queiroz, A. C. de, Leão, M. I., and Abreu, D. C., de (2009). Consumo, digestibilidade aparente dos nutrientes e desempenho de vacas leiteiras em pastejo com dietas com diversos níveis de concentrado e proteína bruta. Rev. Bras. Zootec. 38, 1372–1380. doi: 10.1590/S1516-35982009000700029
Silva, V. M., Buzetti, S., Dupas, E., Teixeira Filho, M. C. M., and Galindo, F. S. (2016). Sources and rates of residual phosphorus for Marandu palisadegrass grown in Western São Paulo. Científica 44:615. doi: 10.15361/1984-5529.2016v44n4p615-622
Soil Science Division Staff (2017). Soil Survey Manual: USDA Handbook No. 18. Washington, D.C: Government Printing Office.
Straub, D., Yang, H., Liu, Y., Tsap, T., and Ludewig, U. (2013). Root ethylene signalling is involved in Miscanthus sinensis growth promotion by the bacterial endophyte Herbaspirillum frisingense GSF30T. J. Exp. Bot. 64, 4603–4615. doi: 10.1093/jxb/ert276
Wang, C., Lu, X., Mori, T., Mao, Q., Zhou, K., Zhou, G., et al. (2018). Responses of soil microbial community to continuous experimental nitrogen additions for 13 years in a nitrogen-rich tropical forest. Soil Biol. Biochem. 121, 103–112. doi: 10.1016/j.soilbio.2018.03.009
Wang, Z., Chen, Z., Kowalchuk, G. A., Xu, Z., Fu, X., and Kuramae, E. E. (2021). Succession of the resident soil microbial community in response to periodic inoculations. Appl. Environ. Microbiol. 87, 1–16. doi: 10.1128/AEM.00046-21
Wickham, H., François, R., Henry, L., and Müller, K. (2020). dplyr: A Grammar of Data Manipulation. Available online at: https://cran.r-project.org/web/packages/dplyr/dplyr.pdf (accessed March 2021).
Yang, X., Ni, K., Shi, Y., Yi, X., Ji, L., Ma, L., et al. (2020). Heavy nitrogen application increases soil nitrification through ammonia-oxidizing bacteria rather than archaea in acidic tea (Camellia sinensis L.) plantation soil. Sci. Total Environ. 717:137248. doi: 10.1016/j.scitotenv.2020.137248
Zaman, M., Kurepin, L. V., Catto, W., and Pharis, R. P. (2016). Evaluating the use of plant hormones and biostimulators in forage pastures to enhance shoot dry biomass production by perennial ryegrass (Lolium perenne L.). J. Sci. Food Agric. 96, 715–726. doi: 10.1002/jsfa.7238
Keywords: perennial ryegrass, red clover, forage, fertilization, Herbaspirillum, Azospirillum
Citation: Pardo-Díaz S, Romero-Perdomo F, Mendoza-Labrador J, Delgadillo-Duran D, Castro-Rincon E, Silva AMM, Rojas-Tapias DF, Cardoso EJBN and Estrada-Bonilla GA (2021) Endophytic PGPB Improves Plant Growth and Quality, and Modulates the Bacterial Community of an Intercropping System. Front. Sustain. Food Syst. 5:715270. doi: 10.3389/fsufs.2021.715270
Received: 26 May 2021; Accepted: 08 September 2021;
Published: 01 October 2021.
Edited by:
Diego Cunha Zied, São Paulo State University, BrazilReviewed by:
Ifigeneia Mellidou, Hellenic Agricultural Organisation (HAO), GreeceAdriana M. Garcia-Lemos, International Flavors and Fragrances (IFF)—Nutrition and Biosciences, Denmark
Copyright © 2021 Pardo-Díaz, Romero-Perdomo, Mendoza-Labrador, Delgadillo-Duran, Castro-Rincon, Silva, Rojas-Tapias, Cardoso and Estrada-Bonilla. This is an open-access article distributed under the terms of the Creative Commons Attribution License (CC BY). The use, distribution or reproduction in other forums is permitted, provided the original author(s) and the copyright owner(s) are credited and that the original publication in this journal is cited, in accordance with accepted academic practice. No use, distribution or reproduction is permitted which does not comply with these terms.
*Correspondence: German A. Estrada-Bonilla, Z2Flc3RyYWRhJiN4MDAwNDA7YWdyb3NhdmlhLmNv; Z2VybWFuZXN0cmEmI3gwMDA0MDtnbWFpbC5jb20=