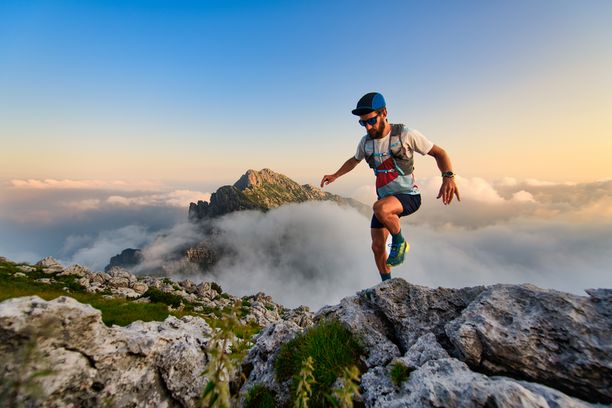
94% of researchers rate our articles as excellent or good
Learn more about the work of our research integrity team to safeguard the quality of each article we publish.
Find out more
REVIEW article
Front. Sustain. Food Syst., 11 October 2021
Sec. Agroecology and Ecosystem Services
Volume 5 - 2021 | https://doi.org/10.3389/fsufs.2021.706142
This article is part of the Research TopicEcological Nutrient Management as a Pathway to Zero HungerView all 13 articles
A continuously growing pressure to increase food, fiber, and fuel production to meet worldwide demand and achieve zero hunger has put severe pressure on soil resources. Abandoned, degraded, and marginal lands with significant agricultural constraints—many still used for agricultural production—result from inappropriately intensive management, insufficient attention to soil conservation, and climate change. Continued use for agricultural production will often require ever more external inputs such as fertilizers and herbicides, further exacerbating soil degradation and impeding nutrient recycling and retention. Growing evidence suggests that degraded lands have a large potential for restoration, perhaps most effectively via perennial cropping systems that can simultaneously provide additional ecosystem services. Here we synthesize the advantages of and potentials for using perennial vegetation to restore soil fertility on degraded croplands, by summarizing the principal mechanisms underpinning soil carbon stabilization and nitrogen and phosphorus availability and retention. We illustrate restoration potentials with example systems that deliver climate mitigation (cellulosic bioenergy), animal production (intensive rotational grazing), and biodiversity conservation (natural ecological succession). Perennialization has substantial promise for restoring fertility to degraded croplands, helping to meet future food security needs.
The continuously growing pressure on agricultural lands to increase food production has severely tested their capacity to produce agricultural products at an acceptable environmental cost. Estimates suggest that if current trajectories continue, 840 million people will be affected by hunger by 2030 (FAO et al., 2021). And some contend that by 2050 food production will need to double or more to meet the demands of a growing global population that is ever more affluent (Food Security Information Network, 2017). However, many lands that are already in use or have previously been used for food production are agronomically degraded. Decreased soil fertility and increased environmental sensitivity to farming due to poor soils or poor management or both have steadily reduced yields on these lands. Many once arable lands are now unsuitable for agriculture, and many have been abandoned from agriculture.
Degraded lands often result from reduced soil fertility stemming from intensive management, poor soil conservation measures, and climate change. Innumerable studies have documented the negative impacts of intensive annual crop production on the soil environment—indeed, the current resurgence of interest in regenerative agriculture (sensu Francis et al., 1986; Giller et al., 2021) has the restoration of soil health as a central tenet (Schreefel et al., 2020). Some specific aspects of intensive annual crop production that lead to soil degradation include frequent disturbance events such as tillage, the absence of continuous year-round plant cover, the lack of continuous deep rooting systems and crop functional diversity, and unbalanced nutrient budgets. Additionally, climate change—increased warming and changing precipitation dynamics worldwide—has accelerated or exacerbated soil degradation in regions where soils are increasingly subjected to flooding and drought (Intergovernmental Panel on Climate Change, 2019). Erosion losses have been particularly severe—up to 1% of topsoil is lost yearly in many places (Montgomery, 2007), the result of tillage, overgrazing, and the growing incidence of extreme climate events that accelerate both wind and water erosion.
The continued use of degraded lands for agricultural production requires ever-increasing management interventions to enable high-yielding food production. In this context, further land degradation represents an additional threat to agriculture's environmental integrity by exacerbating soil carbon (C), nitrogen (N), and phosphorus (P) losses. The loss of C and essential nutrients from the agricultural system results in land that struggles to produce nutritious food for human consumption (FAO, 2019), and losses will only increase as management intensifies to replace lost fertility, creating a positive, downward spiraling feedback loop.
Estimates of the extent of degraded lands worldwide differ markedly depending on the definition. Defined most commonly as lands with reduced productivity due to human activity (Oldeman et al., 1990) leaves wide latitude to estimates of its extent, which range globally from 0.5 to more than 6 billion ha (Gibbs and Salmon, 2015). Narrowing the definition to perhaps its most severe agricultural extent—former agricultural land now abandoned—yields a more restricted estimate of 864 to 951 million ha (Campbell et al., 2008), though still highly uncertain (Gibbs and Salmon, 2015). In the United States alone, estimates based on county land-use records (Campbell et al., 2013) and satellite observations (Cai et al., 2011) suggest a range of 74–99 million ha. We focus here on this narrower definition of degraded lands—croplands or pastures that might, with proper management, be restored and made productive again without long-term consequences to environmental health. Such management might include biologically based practices that promote soil health and recouple C, N, and P cycles through a systems-based approach, focused on improving nutrient retention and balancing nutrient budgets, rather than, for example, fertilizer additions intended to maintain high inorganic nutrient levels in soils (Drinkwater and Snapp, 2007; International Fertilizer Industry Association, 2009). Ecological nutrient management (Drinkwater et al., 2008) is intrinsic to organic, sustainable, and regenerative agriculture (Edwards et al., 1983; Robertson and Harwood, 2001; Giller et al., 2021) and is achieved principally by improving plant diversity, including the incorporation of perennials into long rotations.
We also consider restoring fertility for a newly recognized class of contemporary cropland—subfield areas with consistently low and unprofitable yields. Satellite-based yield stability analyses suggest that >20% of maize (Zea mays L.) and soybean (Glycine max L. Merr) fields in the US Midwest may fit this classification (Basso et al., 2019). Moreover, precision farming technologies (such as identifying under-performing subfield areas and converting them to perennials) create additional potential for restoring the productive capacity of these lands with perennial cropping strategies (Brandes et al., 2018). Subfield variability of this sort likely occurs worldwide.
The restoration of degraded soil fertility via natural perennialization is a longstanding farming practice in place for millennia. Shifting cultivation, known by different names in different regions of the world, and in widespread use worldwide until the Eighteenth century and in the pantropics into the Twentieth century, has as a central tenet the restoration of soil fertility during a natural fallow phase after intensive cropping (Nye and Greenland, 1960; Irvine, 1989; Robertson and Harwood, 2001; Sandor et al., 2007; Schmidt et al., 2021). The natural fallow provides an unmanaged period during which ecological succession restores soil fertility to a point where soil can again be “mined” for agriculture.
That ecological succession restores soil fertility—or, in the case of primary succession, creates soil fertility—is a longstanding ecological principle (Odum, 1969). In primary succession newly exposed parent material is successively colonized by lichens, grasses, forbs, shrubs, and eventually trees, together with a more and more complex soil ecological community that develops as soil organic matter accumulates and N, P, and other nutrients cycle quickly enough to support accelerating primary productivity (Gorham et al., 1979). Secondary succession follows a disturbance that resets the successional clock to some earlier time but does not remove soil and depending on the disturbance—be it fire, extreme weather, agriculture, or some other perturbation—a similar but faster sequence of recovery takes place, eventually, in the absence of continued disturbance, restoring the system to some pre-disturbance state. In one sense, annual cropping systems are caught in an early successional cycle, whereby the ecological clock is reset annually with crop harvest (Robertson and Paul, 1998; Crews et al., 2016). Essential nutrients are readily lost from early successional systems and tightly conserved later, when perennial biomass is rapidly accumulating (Vitousek and Reiners, 1975), which helps to explain the contribution of perennial vegetation to nutrient retention and system-wide nutrient use efficiency. Incorporating perennials into cropping systems to restore fertility and retain nutrients thus draws on ecological theory and a long history of worldwide practice.
Growing evidence suggests that degraded lands also have the potential for restoration while remaining productive (Asbjornsen et al., 2013; Bell et al., 2020). In almost all cases, perennialization—the incorporation of perennial crops and forages in long rotations—is key. Perennialization can be applied in many different systems to enhance the delivery of ecosystem services from agriculture (e.g., Syswerda and Robertson, 2014; Snapp et al., 2015; Schulte et al., 2017), including fertility restoration (Asbjornsen et al., 2013), soil C accretion (Bell et al., 2020; Ledo et al., 2020), N availability (Burke et al., 1995; Reeder et al., 1998; Tufekcioglu et al., 2003), and P retention (Patty et al., 1997; Crews and Brookes, 2014), all important components of ecological nutrient management.
Here we synthesize the advantages of and potentials for using perennial crops to restore soil fertility on degraded lands and their ecosystem functions (Figure 1). In particular, we identify the mechanisms whereby perennial crops enhance and restore C, N, and P cycling using a systems approach. Further, we illustrate alternative management strategies, barriers to adoption, and potential solutions to restore degraded lands via cropping system management might help to meet future food security needs (FAO, 2019).
Figure 1. Patterns of key agronomic and biogeochemical process changes during the restoration of soil fertility via perennialization of degraded cropland.
The central attributes of ecological nutrient management are more efficient nutrient cycling and greater retention of C, N, and P, which are particularly important for sustaining yields in agriculture. Soil C, N, and P stores are key indicators of soil health, and almost always associated with other aspects of soil quality—physical characteristics (including improved infiltration, soil structure, porosity, and aggregate stability), chemical characteristics (including nutrient availability and retention), and biological attributes (including soil food web complexity and pest and pathogen suppression).
There is perhaps no better metric to characterize soil fertility than soil organic matter or soil organic C (SOC) levels. Any activity that leads to SOC accrual benefits the system with increases in soil water holding capacity, nutrient storage and retention (N and P, among others), cation exchange capacity, soil porosity, erosion resistance, soil biota habitat, and any biologically mediated process dependent on C. Soil fertility restoration thus relies heavily on SOC accrual, with strategies to promote C accretion depending on crop type, agricultural management, and organic amendments.
Conventional agricultural practices tend to promote SOC loss. In particular, tillage stimulates the oxidation of soil organic matter, simplifies microbial populations (especially fungal; Helgason et al., 2010), and accelerates erosion, all leading to lower SOC pools, poor soil fertility, and land degradation. Additionally, annual crops contribute relatively little C belowground. In a typical annual cropping system, only a small proportion of total plant biomass is comprised of roots, ready to contribute to stable SOC through turnover and exudation. Root-to-shoot ratios of annual crops are typically <0.30 (Table 1) or <25% of total plant biomass. This is significant for SOC accretion because root derived-C appears to contribute more to SOC stabilization than does aboveground residue, whether the SOC is mineral-associated C (e.g., Kong and Six, 2010; Austin et al., 2017; King et al., 2020) or particulate organic C (e.g., Puget and Drinkwater, 2001; Cates et al., 2016).
Table 1. Root-to-shoot ratios of annual and perennial crops measured at the end of the growing season.
Conversely, perennial cropping systems tend to promote SOC accretion, which results from several attributes (Anderson-Teixeira et al., 2009; Agostini et al., 2015). First, the root-to-shoot ratios of perennial crops are high, typically much >1 and 3–20 times those of maize (Table 1; Ma et al., 2001; Frank et al., 2004; Bonifas et al., 2005; Dietzel et al., 2017). Perennial plants also tend to have longer growing seasons which contributes to more root biomass production (Dohleman and Long, 2009; Ferchaud et al., 2016). Relatively large and deep rooted systems correspond with greater root-associated C inputs (Rasse et al., 2005; Anderson-Teixeira et al., 2009; Agostini et al., 2015). In one synthesis, Anderson-Teixeira et al. (2013) found that a shift from annual conventional systems (e.g., maize-soybean rotations) to perennial crops increased belowground C allocation by >400%, associated with increases in root biomass of up to 2,500%.
Greater root biomass also implies greater rates of root exudation, known to increase and improve soil aggregation, which protects soil C from microbial attack. Thus, one can expect that more roots throughout the soil profile will increase aggregation at many different soil depths (Liebig et al., 2005; Kutsch et al., 2009; Stockmann et al., 2013; Cates et al., 2016). Aggregation not only protects soil C, assuring longer C residence times but also has positive implications for soil water holding capacity and water infiltration (Bharati et al., 2002; Hernandez-Santana et al., 2013; Huang et al., 2014; McGowan et al., 2019). Soils with high levels of aggregation are better able to withstand large precipitation events because water can more quickly infiltrate into deeper depths than soils with poor structure. Improved water infiltration thus reduces runoff of plant available water and essential nutrients, improving water availability over time and helping these systems to be more resilient to extreme weather events (Steward et al., 2011).
The absence of soil disturbance further contributes to SOC gains—in continuous perennial systems, tillage is used only in establishment years, such that afterwards permanent plant cover and better soil structure leads to reduced erosion, lowered decomposition rates, and greater aggregate stability. The use of perennial crops also has the potential to increase the amount, and the diversity of organic inputs returned to the soil when included in any given system. Longer growing periods and less biomass removals during harvest from perennial crops result in more ground cover and more biomass to be returned to the soil, resulting in more SOC.
Diversity per se can also boost SOC accrual, in perennial as in annual systems, leading to more diverse soil microbial communities (Tiemann et al., 2015; Sprunger et al., 2020) and more microbial biomass C (Spehn et al., 2000; Zak et al., 2003). More microbial diversity and biomass C can also enhance soil pore formation (Kravchenko et al., 2019) and aggregate stability facilitated by fungal hyphae and microbial extracellular compounds (Helgason et al., 2010; Tiemann et al., 2015). Further, microbial biomass and decomposition byproducts can stimulate gains in mineral-associated organic matter fractions (Carrington et al., 2012; Miltner et al., 2012) and thus stable C stores. Diversity can also promote soil C accrual through interspecific root C transfer, whereby systems with species that participate in such transfers gain stable C at faster rates (Kravchenko et al., 2021). In addition to impacts on SOC accrual, plant diversity can also enhance pest (herbivore, weed, and disease) suppression, pollination, and other ecosystem services (Gallandt et al., 1999; Abawi and Widmer, 2000; Robertson et al., 2014; Landis, 2017).
Nitrogen is one of the most important and dynamic elements that limit terrestrial plant growth (Lebauer and Treseder, 2008). Though N fertilizer is commonly added to agricultural ecosystems, it is energy-intensive and expensive to produce and typically results in large N losses that harm the environment and human health (Robertson and Vitousek, 2009): Less than half of the N fertilizer applied to agricultural lands globally is recovered at harvest (Lassaletta et al., 2014); the rest is lost to the environment, where it promotes the eutrophication of surface waters, causes marine dead zones, pollutes groundwater drinking supplies, suppresses biodiversity, and contributes to global warming, ultimately threatening long-term food security.
Soil N stocks tend to be severely depleted in degraded lands, making production on these lands even more dependent on external N sources. At the same time, production becomes less responsive to N inputs owing to other constraints on soil fertility, such as low soil organic matter (section Introduction). The net result is even lower N use efficiency, making these systems ever more leaky and environmentally harmful and exacerbating soil degradation in an unfortunate downward spiral.
Nitrogen conservation is thus a cornerstone of regenerative agriculture (Robertson and Harwood, 2001) and sustainable intensification (Pretty, 2018; Spiegal et al., 2018), is central to ecological nutrient management and can be readily evaluated by considering the balance of N inputs and outputs. Cropping systems with high N use efficiency—where N outputs other than harvest are low relative to inputs—will conserve N. Or, put another way, in N-conserving systems, most N inputs will become part of the harvest or be stored in soil organic matter, ready to supply N to a succeeding crop. This can be seen in side-by-side comparisons of fertilized perennial vs. annual cropping systems, where N use efficiency (the amount of N removed relative to fertilizer inputs) is substantially higher for perennial systems (Table 2). Perennial crops—whether harvested, grazed, or used for conservation plantings—have a naturally high potential for conserving N for a variety of reasons and additionally can have novel N acquisition strategies that can minimize their needs for fertilizer N.
Table 2. Crop yields, nitrogen removal, and nitrogen use efficiency (proportion of fertilizer removed at harvest) in alternative annual and perennial cropping systems at the Bioenergy Cropping Systems Experiment at the W. K. Kellogg Biological Station, Hickory Corners, Michigan in 2014.
Perennial crops are highly N use efficient due to a combination of harvest stoichiometry, translocation abilities, long growing seasons, and extensive root systems. First, with the exception of forage legumes and seed crops, relatively little N is removed in perennial harvests. This is because the N content of non-reproductive biomass is commonly several times lower than that of seeds and grain, with their high protein contents and low C:N ratios. This is especially true when harvest occurs post-senescence, when the N content of biomass can be well-under 1% due to N translocation to roots. In grazed systems, “harvests” occur in-season as forage is consumed, but most of the N in this biomass is immediately returned to the pasture as urine and manure. However, this is not as true for forage crops harvested during the growing season for later consumption, when substantially more N can be removed, especially by legumes such as alfalfa (Medicago sativa) that have especially high biomass N contents.
Low post-senescence N contents reflect the ability of perennial plants to translocate N from aboveground leaves and stems to belowground roots, rhizomes, and root crowns prior to senescence (Vergutz et al., 2012). The N stored will then be re-translocated aboveground for use during the next growing season (Yang and Udvardi, 2018), reducing the need for new N. Nitrogen resorption efficiencies for perennial grasses can be >75% (Vergutz et al., 2012) but can also vary substantially even within cultivars of the same species (Yang et al., 2009; Roley et al., 2020), as well as with stand age (Propheter and Staggenborg, 2010). Although N fertilization can increase resorption efficiency—more N gets translocated belowground even when there is no productivity response—it also can lead to higher post-senescence leaf N content, leading to less N conservation overall (Jach-Smith and Jackson, 2015).
Longer growing seasons for perennials also contribute to N conservation. Synchrony between N mineralized from soil organic matter, and plant N uptake is an important N conservation mechanism in most terrestrial ecosystems (Robertson, 1997). In perennial systems, plant growth typically starts earlier in the spring and persists longer into the fall, leading to a greater proportion of the growing season with active N uptake as compared to most annual crops (Culman et al., 2013). Since microbes are active throughout this period and more, in perennial systems, more of the N they mineralize will be immobilized by plants, leaving less to be lost to the environment (Sprunger et al., 2018).
Finally, as noted earlier, perennial plants tend to have deeper and more extensive rooting systems—about 3 to 8 times more extensive than annual crops (Anderson-Teixeira et al., 2013; Dietzel et al., 2017), with root:shoot ratios of 2 to 3 times higher than for annual crops (Table 1). This provides an enhanced potential to capture inorganic N, whether naturally mineralized from soil organic matter or added in fertilizer, before it leaches from the soil profile. Lower leaching rates for both nitrate (Syswerda et al., 2012; Smith et al., 2013; Hussain et al., 2019) and dissolved organic N (Hussain et al., 2020) have been documented in a variety of herbaceous crops and short-rotation trees relative to adjacent annual crops, as well as in conservation strips (Schulte et al., 2017).
All cropping systems must acquire N to replace that removed in harvest or lost to the environment via leaching, volatilization, or denitrification. Perennial cropping systems are no different than annual in this respect, although their losses to the environment are typically lower, as noted above. Nonetheless, to maintain productivity, lost N must be replaced through biological N fixation (BNF), atmospheric deposition, or fertilization. In non-harvested systems such as conservation plantings, losses can be extremely low in the absence of fire, and atmospheric deposition inputs on the order of a few kg per ha per year may be sufficient to meet most long-term N needs. But for harvested systems or unmanaged systems periodically burned, BNF or fertilization must make up for lost biomass N and must additionally be sufficient to provision accumulating soil organic matter, which might be 5% N at typical arable soil C:N ratios of 10:1. So degraded land regenerating soil fertility at a typical C accretion rate of 0.2 Mg C ha−1 yr−1 would sequester ~20 kg N ha−1 yr−1.
Long-term unfertilized perennial grasslands harvested for hay for >50 years (Jenkinson et al., 2004) show no declines in soil C and N stocks or yields, suggesting that N stasis is maintained largely through BNF. Likewise, that perennial herbaceous crops harvested for bioenergy are often unresponsive to N fertilizer suggests significant BNF inputs. At a site in the upper Midwest U.S., for example, Roley et al. (2018) found no response of switchgrass to N fertilizer in most years, and for a three-year period following two establishment years calculated an average minimum annual N deficit of 58 kg N ha−1 based on N mass balance. Biological N fixation—presumably associative N fixation—must have been at least this high to balance known losses from yield plus losses from leaching and denitrification and immobilization of N in soil organic matter, based on N mass balance.
Symbiotic BNF is well-known in legumes; agriculturally important perennials known to host rhizobia capable of N fixation include the herbaceous crops alfalfa and medic (Medicago spp.), clover (Trifolium spp.), vetch (Vicia spp.), and birdsfoot trefoil (Lotus corniculatus), as well as woody species like Leucaena, Gliricidia, and locust (Robinia spp.). In degraded soils, legumes can meet up to 90% of their N needs with atmospheric N2 (i.e., via BNF), and the inclusion of legumes in pastures is a well-known strategy for intensifying forage production.
Less is known about associative N fixation (Smercina et al., 2019; Roley, 2021), which appears as a casual and episodic association between plants and free-living diazotrophic bacteria. Associative N fixation appears to occur most commonly on or adjacent to root surfaces or, at least in sugarcane (Saccharum officinarum spp.), also within plant stems, where the bacteria have ready access to labile C. Nitrogenase, the enzyme complex responsible for transforming atmospheric N2 to a form that plants can use, is exquisitely sensitive to oxygen (Robertson and Groffman, 2021), making BNF difficult outside of the specialized nodules created by legumes and actinorhizal plants to exclude oxygen. Consequently, associative N fixation is slow, spatially discrete, perhaps episodic (Roley et al., 2019), and in any case hard to measure directly. Field measurements have detected major crops such as sugarcane and grasses that benefit from this type of association with N fixers (Boddey and Dobereiner, 1995; Peoples et al., 2001; Roley et al., 2018, 2019). Nevertheless, associative N fixation is being increasingly documented in a wide variety of ecosystems (Reed et al., 2011; Ladha et al., 2016) and attracting renewed attention as a low-cost source of N for perennial bioenergy crops. Although in some cases fertilizer N may still be needed to optimize production, including legumes in bioenergy species mixes would be an additional way to keep system-wide N use efficiency high. Annual crops that rely exclusively on BNF are known to have a higher system-wide N use efficiency (Córdova et al., 2019), and the potential for BNF's improving the N use efficiency of degraded lands through perennialization is equally promising.
Behind N, P is the second most limiting nutrient for plant growth. As an essential nutrient, P is crucial for the structure of DNA and RNA, enzyme production, and for ATP. Thus, P can co-limit plant productivity alongside N or even directly limit productivity in highly weathered soils where P supply is low (Elser et al., 2007). Unlike N, global stores of fertilizer P are limited and must be mined rather than synthesized from an unlimited atmospheric source. In addition to being in limited supply, around 50% of P losses are attributed to erosion, making P a strong environmental pollutant that contributes to poor water quality downstream (Alewell et al., 2020). Despite its importance, P cycling from a biological standpoint is under-studied, in part because P is more difficult to trace than C and N (Guignard et al., 2017). Yet, in degraded lands, P availability can be as severely compromised as C stores and N availability (Schneider et al., 2019).
Soil P is present in organic and inorganic forms, but only inorganic P is available for plant uptake. And like N, not all soil inorganic P is directly available to plants. Inorganic P is present in most soils as minerals such as apatite (a form of calcium phosphate), which must be weathered to an inorganic form available to plants. Additionally, P can be adsorbed to mineral surfaces such as clays containing iron and aluminum. This adsorbed P must undergo desorption reactions to become a soluble form available for plant uptake. Alternatively, organic P in plant residues, microbial biomass, and animal residues such as feces and urine can be mineralized to HPO4−2 for uptake. The organic P pool is dynamic and one of the most important sources of P availability in arable soils (Alewell et al., 2020), especially in organic, low-input, regenerative, and other systems that rely principally on biological nutrient sources.
Many degraded agricultural lands have abundant soil P but not in a form available to plants (Al-Abbas and Barber, 1964). Therefore, the processes that release plant-available P are important for sustainable P cycling. Factors that contribute to low plant-available P include fewer root exudates and microbial products that can aid the dissolution of P into plant-available forms (Graustein et al., 1977; Fox et al., 1990; Ingle and Padole, 2017), low pH, which can contribute to P adsorption by iron and aluminum, and high pH, which can contribute to P adsorption by calcium. Additionally, low levels of soil organic matter can reduce the amount of P available for dissolution insofar as soil organic matter also provides binding sites for soil inorganic P (Deb and Datta, 1967; Hue, 1991). Because the processes involved in P availability and retention have been largely ignored, degraded lands rely heavily on the addition of inorganic P fertilizers to meet plant demand and maintain plant productivity. However, much of the added P will not be returned to the soil as it will be harvested in plant biomass or lost to the system through erosion, runoff, and leaching (Bennett et al., 2001; Childers et al., 2011), further exacerbating the lack of available P in degraded systems.
Perennialization can improve P availability and recycling by reducing P losses and increasing the presence of plant-available P (Patty et al., 1997; Lehmann et al., 2001; Crews and Brookes, 2014). There are many different mechanisms by which this occurs. As previously noted, perennial crops increase soil organic matter, which can enhance P cycling by providing a source of P via decomposition or dissolution of adsorbed inorganic P (Kang et al., 2009; Gaxiola et al., 2011). Phosphatase enzymes produced by soil microbes can selectively cleave P esters from organic matter, allowing P to be immobilized in microbial biomass (van der Heijden et al., 2008; Richardson et al., 2009), which can lead to more P recycling when microbes die. The processes involved with the release of plant-available P are facilitated in perennial cropping systems through enhanced root biomass and rooting depths and through enhanced microbial biomass and activity.
Deeper and more diverse rooting systems, as well as enhanced microbial communities, can also contribute to more efficient P availability and recycling (Crews and Brookes, 2014). The release of P from minerals and organic matter can occur through root exudation of organic acids and through microbial activity. Organic acids produced by roots and microbes break down soil minerals and compete for organic matter adsorption sites to release plant-available P (Deb and Datta, 1967; Fox et al., 1990; Hue, 1991). There is a positive correlation between the amount of organic acids in soil and plant productivity due to P availability (Bolan et al., 1994). More roots at deeper soil depths increase the amount of root exudates and ultimately the amount of P available for plant growth. Microbes are also crucial for transforming P into plant-usable forms by exuding metabolites and organic acids that release adsorbed, unavailable P from minerals and organic matter (Graustein et al., 1977; Ingle and Padole, 2017). Mycorrhizae that are associated with plant roots are particularly important at facilitating this process (Malajczuk and Cromack, 1982; Lapeyrie, 1988), making P more available and enhancing plant uptake of P. Therefore, having an active, diverse microbial community will facilitate P dissolution and mobilization and ultimately the availability in soils.
Not only do perennial systems provide more plant-available P through an increase in root and microbial processes, but they also lead to P retention in the system. When P is not associated with minerals, organic matter, or biomass, it can easily be lost from the system through runoff and erosion (Bennett et al., 2001; Childers et al., 2011). For example, across the globe, agricultural crop fields lose ~15 million tons of P due to erosion (Smil, 2000). And Cordell et al. (2009) estimated that globally, 8 million Mg of P are lost from agricultural fields every year largely due to an imbalance of P fertilizer application rates and plant uptake rates, resulting in the overapplication of P in cropping systems. Patty et al. (1997) found that even small perennial grass buffer stirps were enough to reduce P runoff into water bodies by 89–100%, though it would be better if the P remained in the fields available for future uptake. Root and mycorrhizae uptake of P also helps ensure that the P is not lost from the system. The greater abundance of roots and mycorrhizae in perennial systems increases the probability that P uptake will occur, as root length, root surface area, and mycorrhizae strongly correlate with P uptake (Bolan, 1991; Pang et al., 2010). While P exports from harvested perennial biomass such as alfalfa can be large, less P is removed from the system compared to annual plants such as corn and soybean (Lehmann et al., 2001; Cadot et al., 2018; Cooney, 2019). Perennialization of degraded lands will keep more plant residues and canopy cover in place for longer periods of time. Thus, these lands will be less likely to lose P through erosion, leaching, or runoff.
To illustrate the mechanisms underpinning perennialization's impact on soil fertility restoration we describe below three potential management systems that differ in intensity, impact, and time to full restoration, broadly illustrating three overarching uses—climate change mitigation (bioenergy cropping systems), animal protein production (intensive rotational grazing), and biodiversity restoration (conservation plantings). All three provide complementary mixes of ecosystem services as diagrammed in Figure 2, and none are exclusive—these and other regenerative systems could be established in the same landscape—indeed, on the same farm or ranch—to provide the multifunctionality often sought in sustainable agriculture (Robertson and Harwood, 2001; Boody et al., 2005). Many other opportunities for restoration of soil fertility through perennialization are equally feasible. Some noteworthy examples include perennial grain systems (e.g., Glover et al., 2010; DeHaan et al., 2020), orchard and agroforestry systems (e.g., Subler and Uhl, 1990; Palm, 1995), and long cash crop rotations that include several years of perennial forage or cover crops. All have great potential to restore soil fertility on degraded lands.
Figure 2. Alternative combinations of ecosystem services that might be provided by different perennial systems as compared to degraded cropland.
Cellulosic bioenergy is central to all IPCC mitigation pathways capable of keeping end-of-century global temperature change below 1.5°C (Intergovernmental Panel on Climate Change, 2018), whether bioenergy is used for liquid fuel or electricity generation, or both. While some cellulosic feedstocks will come from agricultural and industrial by-products, a substantial fraction must come from purpose-grown biomass crops, mainly perennial grasses and short-rotation trees (Robertson et al., 2017). The amount of purpose-grown biomass required to meet C-negative energy demands is substantial—in the U.S. alone, at least a billion tons per year (U.S. Department of Energy, 2011) and globally much more (Nakada et al., 2014; Calvin et al., 2019).
Productive cropland cannot be used to sustainably meet much of this demand—converting lands now used to produce food to produce bioenergy will create pressure to convert other lands now unmanaged to food production in order to make up lost food crop productivity, negating much of the climate benefit of biomass crops on contemporary cropland. So-called indirect land-use change (ILUC) effects can only be avoided with unrealistic cropland productivity increases (to compensate for food production when arable cropland is planted to new bioenergy crops), or by avoiding altogether the use of current cropland for bioenergy (Robertson et al., 2017). On the other hand, the use of unproductive or degraded cropland for perennial bioenergy crops will have little ILUC impact on account of the additional climate benefit of removing these lands from annual crop production, and thus are also excellent candidate lands for perennial feedstock production.
Using degraded lands worldwide for cellulosic bioenergy production is thus attractive on two fronts. First, it avoids ILUC effects to allow the full climate benefits of cellulosic bioenergy to be applied toward climate mitigation without benefits' having to be discounted for the additional greenhouse gases produced when natural areas elsewhere are converted to food production. This also addresses targets from Sustainable Development goal 13, relating to climate action. Second, it provides a means whereby degraded lands can be restored to a more fertile state, allowing a greater productive capacity to progressively reduce the amount of land needed for bioenergy production, which in turn will allow these lands to return eventually to a more robust natural state to support global biodiversity goals (IPBES, 2019) or returned to food crop production to meet Sustainable Development goal 2 related to hunger. A growing proportion of bioenergy lands with restored soil fertility would be available for food production once global temperatures stabilized sometime after 2,100.
The restoration of soil fertility under perennial bioenergy crops draws on most of the mechanisms related to ecological nutrient management identified in Section Impacts of perennialization on fertility restoration: soil C accretion, N conservation, and more efficient P cycling. We know most about soil C accretion because of its importance to the climate benefit of bioenergy crops. N is important both because of its positive impact on biomass production but also its negative potential to further burden the biosphere's reactive N load and to discount the climate benefit of bioenergy production via fertilizer and nitrous oxide production. Phosphorus can also limit biomass production, especially in highly weathered tropical soils.
As noted earlier, soil C gains under perennial crops, whether grasses such as switchgrass (Panicum virgatum) or short-rotation trees such as hybrid poplar (Populus spp.), or even complex semi-natural communities such as restored prairie (Tilman et al., 2006; Gelfand et al., 2020), derived from root biomass and stabilized soil C. More specifically, these soil C gains are derived from root exudates and decomposition products in a soil physical environment conducive to the persistence of stable forms of soil C—in particular, C associated with aggregates (e.g., Tiemann and Grandy, 2015) and mineral surfaces (e.g., Garten and Wullschleger, 2000). Some have also advocated biochar additions to bioenergy crops, though the climate benefit will likely be less than were the biomass C instead fully converted to energy production in order to offset fossil fuel use (Paustian et al., 2016).
That soil C can accumulate under bioenergy crops even when all aboveground biomass is harvested illustrates the importance of roots as sources of stabilized soil C. Perennial legumes grown as forage crops have long been known to sequester soil C. In southwest Michigan USA, for example, Syswerda et al. (2011) showed that a continuous alfalfa stand harvested 3–4 times per growing season gained 1.0 Mg C ha−1 yr−1 in the Ap horizon its first 12 years, as others have documented (e.g., Kumar et al., 2018), and at almost three times this rate (2.9 Mg C ha−1 yr−1) when considering the whole profile to 1 m. Soil C also accumulates but often more slowly under grasses; Schmer et al. (2011), for example, documented rates of SOC gain between 1.4 and 3.3 Mg C ha yr−1 to 1.2 m depth in a 5 year study of harvested switchgrass fields on farms in Nebraska, USA. Others (e.g., Frank et al., 2004; Chimento et al., 2016) but not all (e.g., Sprunger and Robertson, 2018) have found gains of a similar magnitude soon after switchgrass establishment. Soil C also accumulates under short-rotation tree crops (Chimento et al., 2016), although post-harvest SOC losses can substantially discount soil C gains when the soil is exposed to erosion and moisture and temperature conditions that accelerate decomposition when the soil is exposed prior to canopy closure of the next crop (Syswerda et al., 2011).
The two greatest risks of large-scale bioenergy production—apart from the use of contemporary cropland now used for food—are further biodiversity loss upon conversion of inappropriate land covers and further N loading of the biosphere. The use of appropriate crops on degraded lands minimizes both risks. First, use of the 864–951 million ha of abandoned cropland worldwide (Campbell et al., 2008; see Introduction) avoids lands of conservation interest—wetlands, old-growth forests, and other natural areas important for biodiversity conservation (IPBES, 2019). Avoiding land with significant forest cover is particularly important in order to avoid long-term C debt that works against climate benefits (Robertson et al., 2017); lands undergoing reforestation are already contributing to climate change mitigation (Griscom et al., 2017). Finally, planting native grasses and short-rotation trees that are native to a region will improve the conservation value of most degraded lands, typically dominated by non-native invasive species with less biodiversity value. Native mixed-species plantings should have an even greater benefit (Werling et al., 2014).
Second, avoiding crops with high N requirements or low N use efficiencies will keep additional reactive N from the environment. Non-leguminous biomass crops such as perennial grasses and short-rotation trees have low N requirements and high N use efficiencies, and if harvested post-senescence, will remove relatively little N in harvest. Mechanisms in section Impacts of perennialization on fertility restoration—in particular, persistent roots that occupy a large proportion of the available soil volume, pre-harvest translocation of N from aboveground biomass to roots, and relatively little N allocated to reproductive biomass such as seeds, resulting in high C:N ratios at harvest—creates a relatively closed ecosystem N cycle. This is even more the case when planting species capable of acquiring most or all of their own N through BNF (see section Nitrogen conservation). Empirical evidence for low N losses from perennial biomass cropping systems is accumulating (e.g., Ruan et al., 2016; Hussain et al., 2019), as is the potential for associative N fixation (Roley et al., 2019, 2020). Moreover, planting perennial biomass crops for biodiversity conservation or bioenergy or both in low-performing, unprofitable subfield portions of existing cropland (Schulte et al., 2017; Brandes et al., 2018) could avoid a substantial amount of contemporary N loss from this cropland (Basso et al., 2019).
All told, then, growing perennial biofuel crops on degraded lands could provide substantial climate mitigation while restoring long-term soil fertility. Non-leguminous perennial crops are especially attractive: few management inputs, long-term soil organic matter accretion, and N and P conservation with biodiversity and other co-benefits. The growth of bioenergy markets—currently unrealized—could make such practices economically profitable as well, providing a well-rounded suite of ecosystem services (Figure 2).
Managing perennial forage as pastures for grazing provides a second major management system for restoring degraded lands. In contrast to current grazing practices that tend to maintain or further exacerbate degraded soils, often due to overgrazing, here we refer to grazing management that aims to maintain perennial forage production in a sustainable way by rotating animals across the landscape. Rotational grazing can take many different forms. Simple rotational grazing (RG) moves animals at low intensities with 2–14 pastures per herd (Briske et al., 2011; Roche et al., 2015). Management intensive grazing (MiG) moves animals at higher intensities with 16+ pastures per herd, and adaptive multi-paddock grazing (AMP) often includes 40+ pastures per herd (Teague et al., 2015; Barton et al., 2020; Mosier et al., 2021). Low intensity systems tend to have more fixed, planned animal movements, whereas more intensive systems are more flexible and tend to move the animals based on forage growth (Undersander et al., 2002). By rotating the animals, the soil and perennial vegetation are provided grazing-free periods that help to maintain and improve soil fertility and perennial productivity (Kemp et al., 2000).
Pastures containing diverse, perennial vegetation offer forage that can often be more productive (Minns et al., 2001; Moore et al., 2004; Skinner et al., 2004) and available for a greater proportion of the growing season (Ferchaud et al., 2016) compared to grazing systems that rely heavily on annually planted crops for year-round forage. Increased productivity of grazed perennial forage could also be due to the increased drought and stress tolerance of many perennial grasses (Tilman and Downing, 1994; Skinner et al., 2006). Additionally, in perennial pastures, there are no annual tillage or extreme harvest events, so more above- and belowground biomass remains after grazing events, especially in AMP grazing systems, which aim to leave 50% of forage uneaten (Teague et al., 2013). With higher productivity of perennial forage, these lands are able to support more animals with increased stocking rates without the negative effects of overgrazing (Jakoby et al., 2015; Teague et al., 2015). Perennialization also diversifies the vegetation available for grazing, often producing more nutritional forage and improving cattle health (Teague et al., 2016).
There are many examples of improved soil fertility from perennial pastures that are rotationally grazed. When the perennial pastures are grazed, the animals keep nutrient cycles more closed and thus conservative, and as well-inexpensive—manure generated on-site provides organic C, N, and P inputs that can improve nutrient retention and availability (Elser and Bennett, 2011; Mosier et al., 2021). In AMP grazing systems, the use of fertilizers and other inputs is minimized or even eliminated; for example, Mosier et al. (2021) found that unfertilized AMP pastures had more soil N than non-AMP pastures annually fertilized. Rotationally grazed perennial systems have also been shown to improve soil health across physical, chemical, and biological indicators (Teague et al., 2011; Byrnes et al., 2018), also resulting in improved water retention and infiltration (McCallum et al., 2004; Teague et al., 2011). The recovery of degraded land using perennial pastures that are rotationally grazed has been shown in both humid regions such as the southern U.S. (Machmuller et al., 2015; Mosier et al., 2021) as well as in semi-arid rangelands in Africa and the western United States (Teague and Dowhower, 2003; Badini et al., 2007; McDonald et al., 2019).
In addition to soil fertility benefits, rotational grazing in perennial pastures can also reduce some greenhouse gas emissions. Teague et al. (2016) found that grazed systems with year-long grass cover produced a smaller greenhouse gas footprint than croplands with periods of bare soil through increased soil C accrual. Further reductions in greenhouse gas emissions, specifically methane, can be achieved through AMP grazing. For example, Shrestha et al. (2020) found that AMP grazing systems increased methane uptake 1.5× compared to other grazing systems. Rotationally grazed perennial systems can also reduce the total amount of CO2 emissions associated with production as compared to other conventionally grazing systems through increased C sequestration and reduced external forage requirements (Bosch et al., 2008). However, some studies have found that N2O emissions were increased in these rotational systems due to higher stocking rates, though these emissions were offset by higher levels of soil C accretion (Bosch et al., 2008; Rowntree et al., 2020). Reductions in net greenhouse gas emissions will also address Sustainable Development Goals linked to climate action.
The costs associated with converting degraded croplands into perennial forage systems that are rotationally grazed, such as the need for more fencing, are relatively low and readily offset by increased productivity and economic returns (Teague et al., 2013; Jakoby et al., 2015; Wang et al., 2018), as demonstrated for areas in the Midwest United States (Riley et al., 1997). Additionally, with higher perennial productivity and longer growing seasons, animals in these systems become less reliant on external sources of forage (Wang et al., 2018), another cost savings. And particularly in dry environments, conversion from underperforming croplands to perennial pastures that are grazed is attractive, especially when ranchers consider forecasted increases in drought intensity (Wang et al., 2021).
All told perennial pasture systems that utilize intensive rotational grazing have the potential to deliver an improved suite of ecosystem services relative to degraded grazing lands and underperforming cropland (Figure 2) while at the same time restoring soil fertility to provide an increasingly productive land base to meet future food needs. Increased productivity will also proportionately alleviate pressures to convert natural ungrazed areas to pastureland, providing significant indirect biodiversity benefits.
At the low end of the perennial management intensity spectrum is the practice of converting degraded croplands into conservation plantings in order to restore soil fertility. Its simplest form is the fallow phase of bush-fallow or shifting cultivation agriculture—simply leaving the land to undergo ecological succession with its restoration of soil organic matter and nutrient availability, as noted earlier (section Introduction). In many cases today, however, soil degradation is past the point of rapid recovery, and the ubiquity of invasive plants means that the vegetation that recovers may bear little resemblance to the original native community with its associated biodiversity benefits. Often more direct conservation management is warranted, whereby specific species selections are made with the explicit goals of restoring ecosystem health or biodiversity or both.
An example of such a management program is the USDA Conservation Reserve Program (CRP) (Skold, 1989). Initiated as a set-aside program to create higher commodity prices—removing land from production creates higher prices by reducing supply—the program now targets environmentally vulnerable lands, otherwise subject to high erosion and nutrient losses, that can be converted to habitat for wildlife and provide other environmental benefits. In short, landowners are paid to take lands that are vulnerable to degradation out of production and to replace them with perennial systems that minimize soil erosion (U.S. Department of Agriculture, 2006) and improve soil health (Li et al., 2017), water quality, and wildlife habitat (Wu and Weber, 2012). An increasingly recognized benefit has been greenhouse gas mitigation (Farm Service Agency, 2011), achieved by increasing soil C sequestration and reducing the use of N fertilizers responsible for soil nitrous oxide emissions (Robertson, 2014), simultaneaously helping to reach Sustainable Development goal 13, which addresses climate change and its impacts.
By 2011 the CRP had reduced the use of N and P fertilizers by 275 and 55 million kg, respectively (Farm Service Agency, 2011), and between 1986 and 2014 resulted in avoidance of over 7 billion Mg of soil erosion (Stubbs, 2014). Earlier studies estimated that CRP enrollment had an average erosion savings of 38 Mg ha−1 (Ribaudo et al., 1990). Young and Osborn (1990) valued the reduction in wind erosion at up to a billion $US, and Ribaudo et al. (1990) valued downstream water quality improvements (from avoided water erosion and nutrient losses) at several times this amount.
Effects of converting cultivated land to conservation lands like CRP have increased soil C and N stocks appreciably. In the U.S., Burke et al. (1995) showed CRP improvements in soil stability and fertility that accompanied soil C and N accretion. Improvements can occur quickly, often after only 5 years, likely due to higher plant productivity from a diversity of seeded perennial grasses (Gebhart et al., 1994; Reeder et al., 1998; Baer et al., 2000). On the other hand, recovery rates can vary by physiochemical soil condition and as well-depend on past land use and disturbance history (Post and Kwon, 2000). In particular, recovery can be slower in climate regions with lower plant productivity, such as arid regions of the western United States (Robles and Burke, 1998).
Similar trends of soil C recovery have been reported for conservation plantings in Europe and Asia. In Germany, Breuer et al. (2006) showed greater soil C and N stores in sites converted to perennial grassland species than in sites under continuous cropland. In Russia and China, several authors have shown a high potential for soil C stock recovery in croplands returned to native grass vegetation. Soil C accretion rates for restored grasslands in Russia were nearly 50% higher than for forests in the same region (Shvidenko and Nilsson, 2003; Vuichard et al., 2008). Similarly, on the Chinese Loess Plateau, abandoned croplands converted to grasslands sequestered more soil C than did restored forested lands (Wei et al., 2012; Jin et al., 2014). Zhang and Shao (2018) also measured higher soil N, P, and overall soil fertility after maize and wheat croplands were converted to perennial grassland.
Conversion of degraded land to conservation plantings benefits multiple taxa, including those that constitute soil microbial communities. Matamala et al. (2008), for example, showed microbial community recovery in a restored prairie converted from cropland, although the recovery was at a much slower rate than soil C and N stocks. Similarly, Baer et al. (2000) showed a slow but consistent increase in microbial biomass on CRP lands planted to native perennial grasses. In China's Loess Plateau, Zhang et al. (2012) documented increases in microbial species richness and biomass, as well as enhanced microbial activity, after cropland conversion to perennial grassland. Most authors relate recovery to perennial rooting systems and associated increases in C inputs from root turnover and exudation.
With the exception of direct economic return or food production, the ecosystem services delivered by conservation plantings are considerable (Figure 2). Removing land from production and its associated inputs immediately eliminates many of the environmental costs of agriculture and initiates the restoration of supporting services such as biodiversity and soil fertility and regulating services such as flood control. However, it also eliminates most provisioning services, which means the direct economic returns derived from food, fiber, or fuel production. That said, with the potential for ecosystem service payments (Swinton et al., 2007) that may include payment for biodiversity benefits such as pollination and natural pest suppression (Asbjornsen et al., 2013; Landis and Gage, 2015), direct economic returns may become an additional ecosystem service provided by conservation plantings (Figure 2). Ultimately, of course, the repatriation of these lands to food production will benefit future food security as once-degraded lands regain their capacity to produce food crops.
To break the cycle of degradation—recovery must be a key part of any soil restoration initiative. It makes little sense to invest decades in management that restores the productive capacity of a system—whether by bioenergy production, intensive rotational grazing, conservation plantings, or any of a number of other practices—only to allow the system to degrade again when returned to food production. Thus, the production system implemented post-recovery must be sustainable. Sustainable Development Goal 2 embodies this challenge: to ensure sustainable food production systems and implement resilient agricultural practices that increase productivity and production; that help maintain ecosystems, strengthen the capacity for adaptation to climate change, extreme weather, drought, flooding, and other disasters; and that progressively improve land and soil quality.
This is a tall order consistent with the need to incorporate sustainable practices on lands that are not currently degraded (see other papers in this Collection). The principles outlined in section Impacts of perennialization on fertility restoration apply. To sustain fertility will require practices that incorporate ecological nutrient management, drawing on principles from organic and regenerative agriculture to maintain stable SOC stores and link C, N, and P cycles to provide nutrients with little environmental loss. First and most important is the need to diversify rotations. Complex rotations that include perennial crops for forage or fallow have, for reasons described earlier, nutrient cycles that are more efficient than simpler rotations. Agricultural systems export C, N, P, and other elements with harvest, so they will never have closed cycles, but high system-wide nutrient use efficiency is achievable with continuous green cover and crops that fix N and mobilize plant-available P. Within-crop diversity can also help to build and maintain greater stabilized C stores and improve nutrient use efficiency, both by intercropping and by using mixed-species cover crops and forages.
Other practices that will be important to engage include no-till to better maintain soil health; variable rate nutrient management and subfield conservation plantings to avoid over-fertilizing low yielding areas of a given field; minimal, precisely targeted pesticide use in order to keep soil food webs intact and fully functional; and animal integration via periodic grazing or manure return. If perennial grains become agronomically viable in the coming decades, a new path will open to incorporate perennial crops into cereal croplands. Incorporating perennials into post-recovery rotations is thus a key aspect of sustainable, regenerative soil management.
Farmers and landowners have numerous options for incorporating perennials into their production and land management strategies, ranging from more complex crop rotations that include perennial crops such as forage grasses and legumes (King and Blesh, 2018) to the establishment of perennial cropping systems such as those for cellulosic bioenergy, rotational grazing, and conservation plantings noted above. Why aren't these practices more widely adopted? Barriers are not, in general, related to knowledge gaps—we have the fundamental knowledge to deploy restorative perennial cropping systems today, and most farmers have the knowledge and skill to successfully establish and manage them. Rather, barriers are largely socioeconomic, related to global trade and national policies that reward the status quo. Three barriers, in particular, stand out.
First is the continued pressure of global food demand. Global food needs are expected to continue their upward trajectory; projections of 30–50% greater food needs by mid-century on account of population and income growth (Food Security Information Network, 2017) will exert continuous pressure on farmers to intensify and expand food production. To the extent that expanded production cannot be met by intensification, there will be pressure to utilize for food production degraded lands with their inherent production and nutrient conservation limitations.
Second are policies that reinforce and reward annual cropping on degraded farmland. In the United States, crop insurance incentivizes farming on even unprofitable cropland as farmers are compensated for poor annual yields—which occur ever more frequently on such lands, creating a downward spiral of positive feedbacks. Moreover, crop insurance in the U.S. and subsidies elsewhere do not incentivize ecological management and are in any case available for only a select few commodities, directly discouraging crop diversity (Archer et al., 2003) and instead rewarding low diversity, high input production systems. As noted by Pascual and Perrings (2007), there is no global market for soil fertility or cropland diversification, and with current policies creating financial roadblocks that discourage regenerative practices, land abandonment is too often the eventual result.
A final major barrier is the lack of markets for some of the most promising restorative perennial cropping systems. In the examples above, neither cellulosic bioenergy crops nor conservation plantings have contemporary markets, nor are regional markets for diverse grain crops sufficiently available—even in the U.S. Midwest, crops as common as canola (Brassica rapa) cannot be grown for lack of nearby processing plants. Thus, not only are there often disincentives for moving away from degraded farmland but there are also no immediate incentives—and indeed disincentives—for adopting regenerative practices.
Two solutions seem tenable, especially in more affluent countries: removing perverse incentives that motivate land degradation and paying farmers for the delivery of ecosystem services (Robertson et al., 2014). By expanding crop subsidy payments, whether direct or indirect, to include additional annual and perennial crops, producers could be rewarded for the management of crop diversity for ecological nutrient management and the accompanying soil restoration. Co-benefits of diversification include resilience to extreme weather (e.g., Bowles et al., 2020), a more stabilized food supply (e.g., Renard and Tilman, 2019), and avoiding yield penalties associated with continuous rotations (Seifert et al., 2017).
Second, payments for ecosystem services (e.g., Swinton et al., 2007) provide a means for society to directly compensate landowners and operators for agricultural practices they would otherwise not adopt. Long lists of candidate services have been generated, and nascent markets are paying farmers for soil C sequestration (Climate Action Reserve, 2020) and more precise N management (Millar et al., 2012), though payments are currently insufficient to motivate much adoption. But payments for climate mitigation practices have been recently proposed in the U.S. and may allow incentives for sequestering soil C using more diverse cropping practices that include perennial crops. Likewise, the USDA CRP program could be expanded to accelerate C accretion in soil and biomass, and markets for cellulosic bioenergy may be on the horizon (Robertson et al., 2017). Ensuring that perennial crops remain a principal target for the delivery of these services will allow the full restorative benefits of perennialization to be realized.
There is an abundance of degraded land in the world in need of soil fertility restoration to meet current and future food security needs. Using perennialization to restore lost fertility seems possible through practices that promote C accretion and the efficient use and conservation of N and P. Carbon accretion is central to fertility restoration, resulting from greater amounts and diversity of belowground C inputs, improved soil structure, and less soil disturbance. Nitrogen conservation can result from practices that improve system-wide N use efficiency, including perennials with their ability to capture N from deeper in the soil profile, translocate N to roots prior to senescence, and for some, fix atmospheric N. Improved P retention and recycling in perennials arises from enhanced inputs of root and microbial metabolites that make soil mineral and organic P available for plant uptake, and fewer losses via runoff, leaching, and erosion.
Barriers to using perennialization to restore degraded soils are surmountable with policies that can incentivize landowners, farmers, and ranchers to manage ecological processes for soil fertility and ecosystem services, perhaps by shifting incentives away from land degrading practices such as intensive short rotation grains toward more diverse rotations and other practices related to regenerative agriculture. Ultimately millions of ha of currently degraded crop and rangeland could be repatriated to restore and enhance ecosystem services, including those related to biodiversity, water, and nutrient conservation, and economic and societal well-being.
All authors listed have made a substantial, direct and intellectual contribution to the work, and approved it for publication.
Support for this research was provided by the Great Lakes Bioenergy Research Center, U.S. Department of Energy, Office of Science, Office of Biological and Environmental Research (Award DE-SC0018409), by the National Science Foundation Long-term Ecological Research Program (DEB 1832042) at the Kellogg Biological Station, by the USDA Long-term Agroecosystem Research (LTAR) Program, and by Michigan State University AgBioResearch.
The authors declare that the research was conducted in the absence of any commercial or financial relationships that could be construed as a potential conflict of interest.
All claims expressed in this article are solely those of the authors and do not necessarily represent those of their affiliated organizations, or those of the publisher, the editors and the reviewers. Any product that may be evaluated in this article, or claim that may be made by its manufacturer, is not guaranteed or endorsed by the publisher.
Abawi, G. S., and Widmer, T. L. (2000). Impact of soil health management practices and soil borne pathogens, nematodes and root diseases of vegetable crops. Agric. Ecosyst. Environ. Appl. Soil Ecol. 15, 37–47. doi: 10.1016/S0929-1393(00)00070-6
Agostini, F., Gregory, A. S., and Richter, G. M. (2015). Carbon sequestration by perennial energy crops: is the jury still out? Bioenergy Res. 8, 1057–1080. doi: 10.1007/s12155-014-9571-0
Al-Abbas, A. H., and Barber, S. A. (1964). A soil test for phosphorus based upon fractionation of soil phosphorus: I. Correlation of soil phosphorus fractions with plant-available phosphorus. Soil Sci. Soc. Am. J. 28, 218–221. doi: 10.2136/sssaj1964.03615995002800020027x
Alewell, C., Ringeval, B., Ballabio, C., Robinson, D. A., Panagos, P., and Borrelli, P. (2020). Global phosphorus shortage will be aggravated by soil erosion. Nat. Commun. 11:4546. doi: 10.1038/s41467-020-18326-7
Allmaras, R. R., Nelson, W. W., and Voorhees, W. B. (1975). Soybean and corn rooting in southwestern Minnesota. II. Root distributions and related water inflow. Soil Sci. Soc. Am. J. 39, 771–777. doi: 10.2136/sssaj1975.03615995003900040046x
Anderson-Teixeira, K. J., Davis, S. C., Masters, M. D., and Delucia, E. H. (2009). Changes in soil organic carbon under biofuel crops. Glob. Change Biol. Bioenergy 1, 75–96. doi: 10.1111/j.1757-1707.2008.01001.x
Anderson-Teixeira, K. J., Masters, M. D., Black, C. K., Zeri, M., Hussain, M. Z., Bernacchi, C. J., et al. (2013). Altered belowground carbon cycling following land-use change to perennial bioenergy crops. Ecosystems 16, 508–520. doi: 10.1007/s10021-012-9628-x
Archer, D. W., Pikul, J. L. J., and Riedell, W. E. (2003). “Analyzing risk and risk management in cropping systems,” in Proceedings of the Dynamic Cropping Systems: Principles, Processes and Challenges, eds. J. D. Hanson and J. M. Krupinsky (Bismark, ND), 155–164.
Asbjornsen, H., Hernandez-Santana, V., Liebman, M., Bayala, J., Chen, J., Helmers, M., et al. (2013). Targeting perennial vegetation in agricultural landscapes for enhancing ecosystem services. Renew. Agric. Food Syst. 29, 101–125. doi: 10.1017/S1742170512000385
Austin, E. E., Wickings, K., Mcdaniel, M., Robertson, G. P., and Grandy, A. S. (2017). Cover crop root contributions to soil carbon in a no-till corn bioenergy cropping system. Glob. Change Biol. Bioenergy 9, 1252–1263. doi: 10.1111/gcbb.12428
Badini, O., Stöckle, C. O., Jones, J. W., Nelson, R., Kodio, A., and Keita, M. (2007). A simulation-based analysis of productivity and soil carbon in response to time-controlled rotational grazing in the West African Sahel region. Agric. Syst. 94, 87–96. doi: 10.1016/j.agsy.2005.09.010
Baer, S. G., Rice, C. W., and Blair, J. M. (2000). Assessment of soil quality in fields with short-, and long-term enrollment in the CRP. J. Soil Water Conserv. 55, 142–146. Available online at: https://www.scopus.com/record/display.uri?eid=2-s2.0-0033887517&origin=inward&txGid=fb3321a635b0f292ed198632a889f888
Barton, E., Bennett, D. E., and Burnidge, W. (2020). Holistic perspectives—Understanding rancher experiences with holistic resource management to bridge the gap between rancher and researcher perspectives. Rangelands 42, 143–150. doi: 10.1016/j.rala.2020.05.003
Basso, B., Shuai, G., Zhang, J., and Robertson, G. P. (2019). Yield stability analysis reveals sources of large-scale nitrogen loss from the U.S. Midwest. Sci. Rep. 9:5774. doi: 10.1038/s41598-019-42271-1
Bell, S. M., Barriocanal, C., Terrer, C., and Rosell-Melé, A. (2020). Management opportunities for soil carbon sequestration following agricultural land abandonment. Environ. Sci. Policy 108, 104–111. doi: 10.1016/j.envsci.2020.03.018
Bennett, E. M., Carpenter, S. R., and Caraco, N. F. (2001). Human impact on erodable phosphorus and eutrophication: a global perspective. Bioscience 51, 227–234. doi: 10.1641/0006-3568(2001)051[0227:HIOEPA]2.0.CO;2
Bharati, L., Lee, K. H., Isenhart, T. M., and Schultz, R. C. (2002). Soil-water infiltration under crops, pasture, and established riparian buffer in Midwestern USA. Agrofor. Syst. 56, 249–257. doi: 10.1023/A:1021344807285
Boddey, R. M., and Dobereiner, J. (1995). Nitrogen fixation associated with grasses and cereals: recent progress and perspectives for the future. Fertil. Res. 42, 241–250. doi: 10.1007/978-94-009-1706-4_24
Bolan, N. S. (1991). A critical review on the role of mycorrhizal fungi in the uptake of phosphorus by plants. Plant Soil 134, 189–207. doi: 10.1007/BF00012037
Bolan, N. S., Naidu, R., Mahimairaja, S., and Baskaran, S. (1994). Influence of low-molecular-weight organic acids on the solubilization of phosphates. Biol. Fert. Soils 18, 311–319. doi: 10.1007/BF00570634
Bonifas, K. D., Walters, D. T., Cassman, K. G., and Lindquist, J. L. (2005). Nitrogen supply affects root:shoot ratio in corn and velvetleaf (Abutilon theophrasti). Weed Sci. 53, 670–675. doi: 10.1614/WS-05-002R.1
Boody, G., Vondracek, B., Andow, D. A., Krinke, M., Westra, J., Zimmerman, J., et al. (2005). Multifunctional agriculture in the United States. Bioscience 55, 27–38. doi: 10.1641/0006-3568(2005)055[0027:MAITUS]2.0.CO;2
Bosch, D. J., Stephenson, K., Groover, G., and Hutchins, B. (2008). Farm returns to carbon credit creation with intensive rotational grazing. J. Soil Water Conserv. 63:91. doi: 10.2489/jswc.63.2.91
Bowles, T. M., Mooshammer, M., Socolar, Y., Calderón, F., Cavigelli, M. A., Culman, S. W., et al. (2020). Long-term evidence shows crop rotation diversification increases agricultural resilience to adverse growing conditions in North America. One Earth 2, 284–293. doi: 10.1016/j.oneear.2020.02.007
Brandes, E., Mcnunn, G. S., Schulte, L. A., Muth, D. J., Vanloocke, A., and Heaton, E. A. (2018). Targeted subfield switchgrass integration could improve the farm economy, water quality, and bioenergy feedstock production. Glob. Change Biol. Bioenergy 10, 199–212. doi: 10.1111/gcbb.12481
Breuer, L., Huisman, J. A., Keller, T., and Frede, H. G. (2006). Impact of a conversion from cropland to grassland on C and N storage and related soil properties: analysis of a 60-year chronosequence. Geoderma 133, 6–18. doi: 10.1016/j.geoderma.2006.03.033
Briske, D. D., Sayre, N. F., Huntsinger, L., Fernandez-Gimenez, M., Budd, B., and Derner, J. D. (2011). Origin, persistence, and resolution of the rotational grazing debate: integrating human dimensions into rangeland research. Rangel. Ecol. Manag. 64, 325–334. doi: 10.2111/REM-D-10-00084.1
Burke, I. C., Lauenroth, W. K., and Coffin, D. P. (1995). Soil organic matter recovery in semiarid grasslands: implications for the conservation reserve program. Ecol. Appl. 5, 793–801. doi: 10.2307/1941987
Byrnes, R. C., Eastburn, D. J., Tate, K. W., and Roche, L. M. (2018). A global meta-analysis of grazing impacts on soil health indicators. J. Environ. Qual. 47, 758–765. doi: 10.2134/jeq2017.08.0313
Cadot, S., BElander, G., Ziadi, N., Morel, C., and Sina, S. (2018). Critical plant and soil phosphorus for wheat, maize, and rapeseed after 44 years of P fertilization. Nutr. Cycling Agroecosyst. 112, 417–433. doi: 10.1007/s10705-018-9956-0
Cai, X., Zhang, X., and Wang, D. (2011). Land availability for biofuel production. Environ. Sci. Technol. 45, 334–339. doi: 10.1021/es103338e
Calvin, K., Patel, P., Clarke, L., Asrar, G., Bond-Lamberty, B., Cui, R. Y., et al. (2019). GCAM v5.1: representing the linkages between energy, water, land, climate, and economic systems. Geosci. Model Dev. 12, 677–698. doi: 10.5194/gmd-12-677-2019
Campbell, J. E., Lobell, D. B., Genova, R. C., and Field, C. B. (2008). The global potential of bioenergy on abandoned agriculture lands. Environ. Sci. Technol. 42, 5791–5794. doi: 10.1021/es800052w
Campbell, J. E., Lobell, D. B., Genova, R. C., Zumkehr, A., and Field, C. B. (2013). Seasonal energy storage using bioenergy production from abandoned croplands. Environ. Res. Lett. 8:035012. doi: 10.1088/1748-9326/8/3/035012
Carrington, E. M., Hernes, P. J., Dyda, R. Y., Plante, A. F., and Six, J. (2012). Biochemical changes across a carbon saturation gradient: lignin, cutin, and suberin decomposition and stabilization in fractionated carbon pools. Soil Biol. Biochem. 47, 179–190. doi: 10.1016/j.soilbio.2011.12.024
Cates, A. M., Ruark, M. D., Hedtcke, J. L., and Posner, J. L. (2016). Long-term tillage, rotation and perennialization effects on particulate and aggregate soil organic matter. Soil Tillage Res. 155, 371–380. doi: 10.1016/j.still.2015.09.008
Childers, D. L., Corman, J., Edwards, M., and Elser, J. J. (2011). Sustainability challenges of phosphorus and food: solutions from closing the human phosphorus cycle. Bioscience 61, 117–124. doi: 10.1525/bio.2011.61.2.6
Chimento, C., Almagro, M., and Amaducci, S. (2016). Carbon sequestration potential in perennial bioenergy crops: the importance of organic matter inputs and its physical protection. Glob. Change Biol. Bioenergy 8, 111–121. doi: 10.1111/gcbb.12232
Climate Action Reserve (2020). Soil enrichment protocol. Version 1.0. Climate Action Reserve. Available online at: https://www.climateactionreserve.org/how/protocols/soil-enrichment/
Cooney, D. R. (2019). Nitrogen, Phosphorus and Potassium, Removal From Perennial Energy Grasses When Grown on Wet Marginal Land, Master's thesis. University of Illinois at Urbana-Champaign, Champaign, IL, United States.
Cordell, D., Drangert, J.-O., and White, S. (2009). The story of phosphorus: global food security and food for thought. Glob. Environ. Change 19, 292–305. doi: 10.1016/j.gloenvcha.2008.10.009
Córdova, S. C., Castellano, M. J., Dietzel, R., Licht, M. A., Togliatti, K., Martinez-Feria, R., et al. (2019). Soybean nitrogen fixation dynamics in Iowa, USA. Field Crops Res. 236, 165–176. doi: 10.1016/j.fcr.2019.03.018
Crews, T. E., Blesh, J., Culman, S. W., Hayes, R. C., Jensen, E. S., Mack, M. C., et al. (2016). Going where no grains have gone before: from early to mid-succession. Agric. Ecosyst. Environ. 223, 223–238. doi: 10.1016/j.agee.2016.03.012
Crews, T. E., and Brookes, P. C. (2014). Changes in soil phosphorus forms through time in perennial versus annual agroecosystems. Agric. Ecosyst. Environ. 184, 168–181. doi: 10.1016/j.agee.2013.11.022
Culman, S. W., Snapp, S. S., Ollenburger, M., Basso, B., and DeHaan, L. R. (2013). Soil and water quality rapidly responds to the perennial grain Kernza wheatgrass. Agron. J. 105, 725–744. doi: 10.2134/agronj2012.0273
Deb, D. L., and Datta, N. P. (1967). Effect of associating anions on phosphorus retention in soil. Plant Soil 26, 303–316. doi: 10.1007/BF01880180
DeHaan, L., Larson, S., Lopez-Marques, R. L., Wenkel, S., Gao, C., and Palmgren, M. (2020). Roadmap for accelerated domestication of an emerging perennial grain crop. Trends Plant Sci. 25, 525–537. doi: 10.1016/j.tplants.2020.02.004
Dietzel, R., Liebman, M., and Archontoulis, S. (2017). A deeper look at the relationship between root carbon pools and the vertical distribution of the soil carbon pool. Soil 3, 139–152. doi: 10.5194/soil-3-139-2017
Dohleman, F. G., and Long, S. P. (2009). More productive than maize in the Midwest: how does miscanthus do it? Plant Physiol. 150, 2104–2115. doi: 10.1104/pp.109.139162
Drinkwater, L. E., Schipanski, M., Snapp, S. S., and Jackson, L. E. (2008). Ecologically based nutrient management. agricultural systems: agroecology and rural innovation for development, eds S.S. Snapp, and B. Pound (Burlington, MA: Academic Press), 159–208.
Drinkwater, L. E., and Snapp, S. S. (2007). Nutrients in agroecosystems: rethinking the management paradigm. Adv. Agron. 92, 163–186. doi: 10.1016/S0065-2113(04)92003-2
Edwards, C. A., Grove, T. L., Harwood, R. R., and Pierce Colfer, C. J. (1983). The role of agroecology and integrated farming systems in agricultural sustainability. Agric. Ecosyst. Environ. 46, 99–121. doi: 10.1016/0167-8809(93)90017-J
Elser, J., and Bennett, E. (2011). A broken biogeochemical cycle. Nature 478, 29–31. doi: 10.1038/478029a
Elser, J. J., Bracken, M. E. S., Cleland, E. E., Gruner, D. S., Harpole, W. S., Hillebrand, H., et al. (2007). Global analysis of nitrogen and phosphorus limitation of primary production in freshwater, marine, and terrestrial ecosystems. Ecol. Lett. 10, 1135–1142. doi: 10.1111/j.1461-0248.2007.01113.x
FAO (2019). Climate-Smart Agriculture and the Sustainable Development Goals: Mapping Interlinkages, Synergies and Trade-Offs and Guidelines for Integrated Implementation. FAO: Rome.
FAO., IFAD., UNICEF., WFP., and WHO. (2021). The State of Food Security and Nutrition in the World 2021. Transforming Food Systems for Food Security, Improved Nutrition and Affordable Healthy Diets for all. Rome: FAO.
Farm Service Agency (2011). The Environmental Benefits of the Conservation Reserve Program- United States 2011. USDA. Available online at: //www.fsa. usda.gov/Assets/USDA-FSA-Public/usdafiles/EPAS/PDF/us_benefits_2011.pdf
Ferchaud, F., Vitte, G., and Mary, B. (2016). Changes in soil carbon stocks under perennial and annual bioenergy crops. Glob. Change Biol. Bioenergy 8, 290–306. doi: 10.1111/gcbb.12249
Food Security Information Network (2017). Global Report on Food Crises 2017. Available online at: https://www.wfp.org/publications/global-report-food-crisis-2017
Fox, T. R., Comerford, N. B., and Mcfee, W. W. (1990). Phosphorus and aluminum release from a spodic horizon mediated by organic acids. Soil Sci. Soc. Am. J. 54, 1763–1767. doi: 10.2136/sssaj1990.03615995005400060043x
Francis, C. A., Harwood, R. R., and Parr, J. F. (1986). The potential for regenerative agriculture in the developing world. Am. J. Altern. Agric. 1, 65–74. doi: 10.1017/S0889189300000904
Frank, A. B., Berdahl, J. D., Hanson, J. D., Liebig, M. A., and Johnson, H. A. (2004). Biomass and carbon partitioning in switchgrass. Crop Sci. 44, 1391–1396. doi: 10.2135/cropsci2004.1391
Gallandt, E. R., Liebman, M., and Huggins, D. R. (1999). Improving soil quality: implications for weed management. J.Crop Product. 2, 95–121. doi: 10.1300/J144v02n01_06
Garten, C. T., and Wullschleger, S. D. (2000). Soil carbon dynamics beneath switchgrass as indicated by stable isotope analysis. J. Environ. Qual. 29, 645–653. doi: 10.2134/jeq2000.00472425002900020036x
Gaxiola, R. A., Edwards, M., and Elser, J. J. (2011). A transgenic approach to enhance phosphorus use efficiency in crops as part of a comprehensive strategy for sustainable agriculture. Chemosphere 84, 840–845. doi: 10.1016/j.chemosphere.2011.01.062
Gebhart, D. L., Johnson, H. B., Mayeux, H. S., and Polley, H. W. (1994). The CRP increases soil organic carbon. J. Soil Water Conserv. 45, 488–492.
Gelfand, I., Hamilton, S. K., Kravchenko, A. N., Jackson, R. D., Thelen, K. D., and Robertson, G. P. (2020). Empirical evidence for the potential climate benefits of decarbonizing light vehicle transport in the U.S. with bioenergy from purpose-grown biomass with and without BECCS. Environ. Sci. Technol. 54, 2961–2974. doi: 10.1021/acs.est.9b07019
Gibbs, H. K., and Salmon, J. M. (2015). Mapping the world's degraded lands. Appl. Geogr. 57, 12–21. doi: 10.1016/j.apgeog.2014.11.024
Giller, K. E., Hijbeek, R., Andersson, J. A., and Sumberg, J. (2021). Regenerative agriculture: an agronomic perspective. Outlook Agric. 50, 13–25. doi: 10.1177/0030727021998063
Glover, J. D., Reganold, J. P., Bell, L. W., Borevitz, J., Brummer, E. C., et al. (2010). Increased food and ecosystem security via perennial grains. Science 328, 1638–1639. doi: 10.1126/science.1188761
Gorham, E., Vitousek, P. M., and Reiners, W. A. (1979). The regulation of chemical budgets over the course of terrestrial ecosystem succession. Ann. Rev. Ecol. Syst. 10, 53–88. doi: 10.1146/annurev.es.10.110179.000413
Graustein, W. C., Cromack, K., and Sollins, P. (1977). Calcium oxalate: occurrence in soils and effect on nutrient and geochemical cycles. Science 198, 1252–1254. doi: 10.1126/science.198.4323.1252
Griscom, B. W., Adams, J., Ellis, P. W., Houghton, R. A., Lomax, G., Miteva, D. A., et al. (2017). Natural climate solutions. PNAS 114, 11645–11650. doi: 10.1073/pnas.1710465114
Guignard, M. S., Leitch, A. R., Acquisti, C., Eizaguirre, C., Elser, J. J., Hessen, D. O., et al. (2017). Impacts of nitrogen and phosphorus: from genomes to natural ecosystems and agriculture. Front. Ecol. Evol. 5:70. doi: 10.3389/fevo.2017.00070
Helgason, B. L., Walley, F. L., and Germida, J. J. (2010). No-till soil management increases microbial biomass and alters community profiles in soil aggregates. Appl. Soil Ecol. 46, 390–397. doi: 10.1016/j.apsoil.2010.10.002
Hernandez-Santana, V., Zhou, X., Helmers, M. J., Asbjornsen, H., Kolka, R., and Tomer, M. (2013). Native prairie filter strips reduce runoff from hillslopes under annual row-crop systems in Iowa, USA. J. Hydrol. 477, 94–103. doi: 10.1016/j.jhydrol.2012.11.013
Huang, X.-F., Chaparro, J. M., Reardon, K. F., Zhang, R., Shen, Q., and Vivanco, J. M. (2014). Rhizosphere interactions: root exudates, microbes, and microbial communities. Botany 92, 267–275. doi: 10.1139/cjb-2013-0225
Hue, N. V. (1991). Effects of organic acids/anions on p sorption and phytoavailability in soils with different mineralogies. Soil Sci. 152, 463–471. doi: 10.1097/00010694-199112000-00009
Hussain, M. Z., Bhardwaj, A. K., Basso, B., Robertson, G. P., and Hamilton, S. K. (2019). Nitrate leaching from continuous corn, perennial grasses, and poplar in the US Midwest. J. Environ. Qual. 48, 1849–1855. doi: 10.2134/jeq2019.04.0156
Hussain, M. Z., Robertson, G. P., Basso, B., and Hamilton, S. K. (2020). Leaching losses of dissolved organic carbon and nitrogen from agricultural soils in the upper US Midwest. Sci. Total Environ. 734:139379. doi: 10.1016/j.scitotenv.2020.139379
Ingle, K. P., and Padole, D. A. (2017). Phosphate solublizing microbes: an overview. Int. J. Curr. Microbiol. Appl. Sci. 6, 844–852. doi: 10.20546/ijcmas.2017.601.099
Intergovernmental Panel on Climate Change (2018). Global Warming of 1.5°C. An IPCC Special Report on the Impacts of Global Warming of 1.5°C Above Pre-Industrial Levels and Related Global Greenhouse Gas Emission Pathways, in the Context of Strengthening the Global Response to the Threat of Climate Change, Sustainable Development, and Efforts to Eradicate Poverty, eds. V. Masson-Delmotte, P. Xhai, H. Pörtner, D. Roberts, J. Skea, P. Shukla, et al. Available online at: https://www.ipcc.ch/sr15/
Intergovernmental Panel on Climate Change (2019). Climate Change and Land, eds P. R. Shukla, J. Skea, E. Calvo Buendia, V. Masson-Delmotte, H.-O. Pörtner, D. C. Roberts, et al. Available online at: https://www.ipcc.ch/srccl/
International Fertilizer Industry Association (2009). The Global “4R” Nutrient Stewardship Framework: Developing Fertilizer Best Management Practices for Delivering Economic, Social and Environmental Benefits. Paris: IFA Task Force on Fertilizer Best Management Practices.
IPBES (2019). Global Assessment Report on Biodiversity and Ecosystem Services of the Intergovernamental Science-Policy Platform on Biodiversity and Ecosystem Services, eds E. S. Brondizio, J. Settele, S. Diaz, and H. T. Ngo (Bonn: IPBES secretariat). Available online at: https://ipbes.net/global-assessment
Irvine, D. (1989). Succession management and resource distribution in an Amazonian rain forest. Adv. Econ. Bot. 7, 223–237.
Jach-Smith, L. C., and Jackson, R. D. (2015). Nitrogen conservation decreases with fertilizer addition in two perennial grass cropping systems for bioenergy. Agric. Ecosyst. Environ. 204, 62–71. doi: 10.1016/j.agee.2015.02.006
Jakoby, O., Quaas, M. F., Baumgärtner, S., and Frank, K. (2015). Adapting livestock management to spatio-temporal heterogeneity in semi-arid rangelands. J. Environ. Manage. 162, 179–189. doi: 10.1016/j.jenvman.2015.07.047
Jenkinson, D. S., Poulton, P. R., Johnston, A. E., and Powlson, D. S. (2004). Turnover of nitrogen-15-1abled fertilizer in old grassland. Soil Sci. Soc. Am. J. 68, 865–875. doi: 10.2136/sssaj2004.8650
Jin, Z., Dong, Y., Wang, Y., Wei, X., Wang, Y., Cui, B., et al. (2014). Natural vegetation restoration is more beneficial to soil surface organic and inorganic carbon sequestration than tree plantation on the Loess Plateau of China. Sci. Total Environ. 485–486, 615–623. doi: 10.1016/j.scitotenv.2014.03.105
Kang, J., Hesterberg, D., and Osmond, D. L. (2009). Soil organic matter effects on phosphorus sorption: a path analysis. Soil Sci. Soc. Am. J. 73, 360–366. doi: 10.2136/sssaj2008.0113
Kemp, D. R., Michalk, D. L., and Virgona, J. M. (2000). Towards more sustainable pastures: lessons learnt. Aust. J. Exp. Agric. 40, 343–356. doi: 10.1071/EA99001
King, A. E., and Blesh, J. (2018). Crop rotations for increased soil carbon: perenniality as a guiding principle. Ecol. Appl. 28, 249–261. doi: 10.1002/eap.1648
King, A. E., Congreves, K. A., Deen, B., Dunfield, K. E., Simpson, M. J., Voroney, R. P., et al. (2020). Crop rotations differ in soil carbon stabilization efficiency, but the response to quality of structural plant inputs is ambiguous. Plant Soil 457, 207–224. doi: 10.1007/s11104-020-04728-5
Kong, A. Y. Y., and Six, J. (2010). Tracing root vs. residue carbon into soils from conventional and alternative cropping systems. Soil Sci. Soc. Am. J. 74, 1201–1210. doi: 10.2136/sssaj2009.0346
Kravchenko, A. N., Guber, A. K., Rasavi, B. S., Koestel, J., Quigley, M. Y., Robertson, G. P., et al. (2019). Microbial spatial footprint as a driver of soil carbon stabilization. Nat. Commun. 10:3121. doi: 10.1038/s41467-019-11057-4
Kravchenko, A. N., Zheng, H., Kuzyakov, Y., Robertson, G. P., and Guber, A. K. (2021). Belowground interplant carbon transfer promotes soil carbon gains in diverse plant communities. Soil Biol. Biochem. 159:108297. doi: 10.1016/j.soilbio.2021.108297
Kumar, S., Meena, R. S., Lal, R., Singh Yadav, G., Mitran, T., Meena, B. L., et al. (2018). “Role of legumes in soil carbon sequestration,” in Legumes for Soil Health and Sustainable Management, eds. R.S. Meena, A. Das, G.S. Yadav and R. Lal (Singapore: Springer Singapore), 109–138. doi: 10.1007/978-981-13-0253-4_4
Kutsch, W. L., Schimel, J., and Denef, K. (2009). “Measuring soil microbial parameters relevant for soil carbon fluxes,” in Soil Carbon Dynamics: An Integrated Methodology, eds W. L. Kutsch, M. Bahn, and A. Heinemeyer. (Cambridge: Cambridge University Press), 169–186. doi: 10.1017/CBO9780511711794.010
Ladha, J. K., Tirol-Padre, A., Reddy, C. K., Cassman, K. G., and Verma, S. (2016). Global nitrogen budgets in cereals: a 50-year assessment for maize, rice, and wheat production systems. Sci. Rep. 6:19355. doi: 10.1038/srep19355
Landis, D. A. (2017). Designing agricultural landscapes for biodiversity-based ecosystem services. Basic App. Ecol. 18, 1–12. doi: 10.1016/j.baae.2016.07.005
Landis, D. A., and Gage, S. H. (2015). “Arthropod diversity and pest suppression in agricultural landscapes,” in The Ecology of Agricultural Landscapes: Long-Term Research on the Path to Sustainability, eds S. K. Hamilton, J. E. Doll, and G. P. Robertson (New York, NY: Oxford University Press), 188–212.
Lapeyrie, F. (1988). Oxalate synthesis from soil bicarbonate by the mycorrhizal fungus Paxillus involutus. Plant Soil 110, 3–8. doi: 10.1007/BF02143532
Lassaletta, L., Billen, G., Grizzetti, B., Anglade, J., and Garnier, J. (2014). 50 year trends in nitrogen use efficiency of world cropping systems: the relationship between yield and nitrogen input to cropland. Environ. Res. Lett. 9:105011. doi: 10.1088/1748-9326/9/10/105011
Lebauer, D. S., and Treseder, K. K. (2008). Nitrogen limitation of net primary production in terrestrial ecosystems is globally distributed. Ecology 89, 371–379. doi: 10.1890/06-2057.1
Ledo, A., Smith, P., Zerihun, A., Whitaker, J., Vicente-Vicente, J. L., Qin, Z., et al. (2020). Changes in soil organic carbon under perennial crops. Glob. Change Biol. 26, 4158–4168. doi: 10.1111/gcb.15120
Lehmann, J., Cravo, M. D. S., Vasconselos De Macêdo, J. L., Moreira, A., and Schroth, G. (2001). Phosphorus management for perennial crops in central amazonian upland soils. Plant Soil 237, 309–319. doi: 10.1023/A:1013320721048
Li, C., Fultz, L. M., Moore-Kucera, J., Acosta-Martínez, V., Horita, J., Strauss, R., et al. (2017). Soil carbon sequestration potential in semi-arid grasslands in the conservation reserve program. Geoderma 294, 80–90. doi: 10.1016/j.geoderma.2017.01.032
Liebig, M. A., Johnson, H. A., Hanson, J. D., and Frank, A. B. (2005). Soil carbon under switchgrass stands and cultivated cropland. Biomass Bioenergy 28, 347–354. doi: 10.1016/j.biombioe.2004.11.004
Ma, Z., Wood, C. W., and Bransby, D. I. (2001). Impact of row spacing, nitrogen rate, and time on carbon partitioning of switchgrass. Biomass Bioenergy 20, 413–419. doi: 10.1016/S0961-9534(01)00008-3
Machmuller, M. B., Kramer, M. G., Cyle, T. K., Hill, N., Hancock, D., and Thompson, A. (2015). Emerging land use practices rapidly increase soil organic matter. Nat. Commun. 6:6995. doi: 10.1038/ncomms7995
Malajczuk, N., and Cromack, K. Jr. (1982). Accumulation of calcium oxalate in the mantle of ectomycorrhizal roots of Pinus radiata and Eucalyptus marginata. New Phytol. 92, 527–531. doi: 10.1111/j.1469-8137.1982.tb03411.x
Matamala, R., Jastrow, J. D., Miller, R. M., and Garten, C. T. (2008). Temporal changes in c and n stocks of restored prairie: implications for C sequestration strategies. Ecol. Appl. 18, 1470–1488. doi: 10.1890/07-1609.1
McCallum, M. H., Kirkegaard, J. A., Green, T. W., Cresswell, H. P., Davies, S. L., Angus, J. F., et al. (2004). Improved subsoil macroporosity following perennial pastures. Aust. J. Exp. Agric. 44, 299–307. doi: 10.1071/EA03076
McDonald, S. E., Reid, N., Smith, R., Waters, C. M., Hunter, J., and Rader, R. (2019). Rotational grazing management achieves similar plant diversity outcomes to areas managed for conservation in a semi-arid rangeland. Rangeland J. 41, 135–145. doi: 10.1071/RJ18090
McGowan, A. R., Nicoloso, R. S., Diop, H. E., Roozeboom, K. L., and Rice, C. W. (2019). Soil organic carbon, aggregation, and microbial community structure in annual and perennial biofuel crops. Agron. J. 111, 128–142. doi: 10.2134/agronj2018.04.0284
Millar, N., Robertson, G. P., Diamant, A., Gehl, R. J., Grace, P. R., and Hoben, J. P. (2012). Methodology for quantifying nitrous oxide (N2O) emissions reductions by reducing nitrogen fertilizer use on agricultural crops. Little Rock, AR: American Carbon Registry; Winrock International.
Miltner, A., Bombach, P., Schmidt-Brucken, B., and Kastner, M. (2012). SOM genesis: microbial biomass as a significant source. Biogeochemistry 111, 41–55. doi: 10.1007/s10533-011-9658-z
Minns, A., Finn, J., Hector, A., Caldeira, M., Joshi, J., Palmborg, C., et al. (2001). The functioning of European grassland ecosystems: potential benefits of biodiversity to agriculture. Outlook Agric. 30, 179–185. doi: 10.5367/000000001101293634
Montgomery, D. R. (2007). Soil erosion and agricultural sustainability. PNAS 104, 13268–13272. doi: 10.1073/pnas.0611508104
Moore, K. J., White, T. A., Hintz, R. L., Patrick, P. K., and Brummer, E. C. (2004). Sequential grazing of cool- and warm-season pastures. Agron. J. 96, 1103–1111. doi: 10.2134/agronj2004.1103
Mosier, S., Apfelbaum, S., Byck, P., Calderon, F., Teague, R., Thompson, R., et al. (2021). Adaptive multi-paddock grazing enhances soil carbon and nitrogen stocks and stabilization through mineral association in southeastern U.S. grazing lands. J. Environ. Manage. 288:112409. doi: 10.1016/j.jenvman.2021.112409
Nakada, S., Sayfin, D., and Gielen, D. (2014). Global Bioenergy Supply and Demand Projections: A Working Paper for REmap 2030. International Renewable Energy Agency. Available online at: https://www.irena.org/publications/2014/Sep/Global-Bioenergy-Supply-and-Demand-Projections-A-working-paper-for-REmap-2030
Nye, P. H., and Greenland, D. J. (1960). “The soil under shifting cultivation,” in Technical Communication of the Commonwealth Bureau of Soils, Harpenden (Bucks: Commonwealth Agricultural Bureaux).
Odum, E. P. (1969). The strategy of ecosystem development. Science 164, 262–270. doi: 10.1126/science.164.3877.262
Oldeman, L. R., Hakkeling, R. T. A., and Sombroek, W. G. (1990). World Map of the Status of Human-Induced Soil Degradation: An Explanatory Note. Wageningen: International Soil Reference and Information Centre.
Ordóñez, R. A., Archontoulis, S. V., Martinez-Feria, R., Hatfield, J. L., and Wright, E. E. (2020). Root to shoot and carbon to nitrogen ratios of maize and soybean crops in the US Midwest. Eur. J. Agron. 120:126130. doi: 10.1016/j.eja.2020.126130
Palm, C. A. (1995). Contribution of agroforestry trees to nutrient requirements of intercropped plants. Agrofor. Syst. 30, 105–124. doi: 10.1007/978-94-017-0681-0_5
Pang, J., Ryan, M. H., Tibbett, M., Cawthray, G. R., Siddique, K. H. M., Bolland, M. D. A., et al. (2010). Variation in morphological and physiological parameters in herbaceous perennial legumes in response to phosphorus supply. Plant Soil 331, 241–255. doi: 10.1007/s11104-009-0249-x
Pascual, U., and Perrings, C. (2007). Developing incentives and economic mechanisms for in situ biodiversity conservation in agricultural landscapes. Agric. Ecosyst. Environ. 121, 256–268. doi: 10.1016/j.agee.2006.12.025
Patty, L., Réal, B., and Joël Gril, J. (1997). The use of grassed buffer strips to remove pesticides, nitrate and soluble phosphorus compounds from runoff water. Pestic. Sci. 49, 243–251. doi: 10.1002/(SICI)1096-9063(199703)49:3<243::AID-PS510>3.0.CO;2-8
Paustian, K., Lehmann, J., Ogle, S., Reay, D., Robertson, G. P., and Smith, P. (2016). Climate-smart soils. Nature 532, 49–57. doi: 10.1038/nature17174
Peoples, M. B., Giller, K. E., Herridge, D., and Vessey, J. (2001). “Limitations to biological nitrogen fixation as a renewable source of nitrogen for agriculture,” in Nitrogen Fixation: Global Perspectives, eds T. Finan, M. O'Brian, D. Layzell, J. Vessay, W. Newton (New York, NY: CABI Publishing), 356–360. doi: 10.1079/9780851995915.0356
Post, W. M., and Kwon, K. C. (2000). Soil carbon sequestration and land use change: processes and potential. Glob. Change Biol. 6, 317–327. doi: 10.1046/j.1365-2486.2000.00308.x
Pretty, J. (2018). Intensification for redesigned and sustainable agricultural systems. Science 362:eaav0294. doi: 10.1126/science.aav0294
Propheter, J. L., and Staggenborg, S. (2010). Performance of annual and perennial biofuel crops: nutrient removal during the first two years. Agron. J. 102, 798–805. doi: 10.2134/agronj2009.0462
Puget, P., and Drinkwater, L. E. (2001). Short-term dynamics of root- and shoot-derived carbon from a leguminous green manure. Soil Sci. Soc. Am. J. 65, 771–779. doi: 10.2136/sssaj2001.653771x
Rasse, D. P., Rumpel, C., and Dignac, M.-F. (2005). Is soil carbon mostly root carbon? Mechanisms for a specific stabilisation. Plant Soil 269, 341–356. doi: 10.1007/s11104-004-0907-y
Reed, S. C., Cleveland, C. C., and Townsend, A. R. (2011). Functional ecology of free-living nitrogen fixation: a contemporary perspective. Annu. Rev. Ecol. Evol. Syst. 42, 489–512. doi: 10.1146/annurev-ecolsys-102710-145034
Reeder, J. D., Schuman, G. E., and Bowman, R. A. (1998). Soil C and N changes on conservation reserve program lands in the central Great Plains. Soil Tillage Res. 47, 339–349. doi: 10.1016/S0167-1987(98)00122-6
Renard, D., and Tilman, D. (2019). National food production stabilized by crop diversity. Nature 571, 257–260. doi: 10.1038/s41586-019-1316-y
Ribaudo, M. O., Colacicco, D., Langer, L. L., Piper, S., and Schaible, G. D. (1990). Natural Resources and Users Benefit From the Conservation Reserve Program. Washington, DC: Economic Research Service, USDA.
Richardson, A. E., Barea, J.-M., Mcneill, A. M., and Prigent-Combaret, C. (2009). Acquisition of phosphorus and nitrogen in the rhizosphere and plant growth promotion by microorganisms. Plant Soil 321, 305–339. doi: 10.1007/s11104-009-9895-2
Riley, B., Nelson, C., Strohbehn, D., Bredahl, R., Peterson, B., and Sprague, R. (1997). An Economic Comparison of Rotational Grazing Systems to Eight Crop Alternatives and the CRP Option for Highly Erodible Land in Southwest Iowa. Ames, IA: Iowa State University Animal Industry Report.
Robertson, G. P. (1997). “Nitrogen use efficiency in row crop agriculture: crop nitrogen use and soil nitrogen loss,” in Ecology in Agriculture, ed L. E. Jackson (New York, NY: Academic Press), 347–365. doi: 10.1016/B978-012378260-1/50011-7
Robertson, G. P. (2014). “Soil greenhouse gas emissions and their mitigation,” in Encyclopedia of Agriculture and Food Systems, ed N. Van Alfen (San Diego, CA: Elsevier), 185–196. doi: 10.1016/B978-0-444-52512-3.00097-8
Robertson, G. P., and Groffman, P. M. (2021). “Nitrogen transformations,” in Soil Microbiology, Ecology, and Biochemistry, 5th edn. eds E. A. Paul, and S. D. Frey (Burlington, MA: Elsevier).
Robertson, G. P., Gross, K. L., Hamilton, S. K., Landis, D. A., Schmidt, T. M., Snapp, S. S., et al. (2014). Farming for ecosystem services: an ecological approach to production agriculture. Bioscience 64, 404–415. doi: 10.1093/biosci/biu037
Robertson, G. P., Hamilton, S. K., Barham, B. L., Dale, B. E., Izaurralde, R. C., Jackson, R. D., et al. (2017). Cellulosic biofuel contributions to a sustainable energy future: choices and outcomes. Science 356:eaal2324d. doi: 10.1126/science.aal2324
Robertson, G. P., and Harwood, R. R. (2001). “Sustainable Agriculture,” in Encyclopedia of Biodiversity, ed S. A. Levin (New York, NY: Academic Press), 99–108. doi: 10.1016/B0-12-226865-2/00008-0
Robertson, G. P., and Paul, E. A. (1998). “Ecological research in agricultural ecosystems: contributions to ecosystem science and to the management of agronomic resources,” in Successes, Limitations, and Frontiers in Ecosystem Science, eds M. L. Pace and P. M. Groffman (New York, NY: Springer-Verlag), 142–164. doi: 10.1007/978-1-4612-1724-4_6
Robertson, G. P., and Vitousek, P. M. (2009). Nitrogen in agriculture: balancing the cost of an essential resource. Annu. Rev. Environ. Resour. 34, 97–125. doi: 10.1146/annurev.environ.032108.105046
Robles, M. D., and Burke, I. C. (1998). Soil organic matter recovery on conservation reserve program fields in southeastern Wyoming. Soil Sci. Soc. Am. J. 62, 725–730. doi: 10.2136/sssaj1998.03615995006200030026x
Roche, L. M., Cutts, B. B., Derner, J. D., Lubell, M. N., and Tate, K. W. (2015). On-ranch grazing strategies: context for the rotational grazing dilemma. Rangeland Ecol. Manage. 68, 248–256. doi: 10.1016/j.rama.2015.03.011
Roley, S. S. (2021). “Diazotrophic nitrogen fixation in the rhizosphere and endosphere,” in Rhizosphere Biology: Interactions Between Microbes and Plants, eds V. V. S. R. Gupta and A. K. Sharma (Singapore: Springer Nature), 93–108. doi: 10.1007/978-981-15-6125-2_4
Roley, S. S., Duncan, D. S., Liang, D., Garoutte, A., Jackson, R. D., Tiedje, J. M., et al. (2018). Associative nitrogen fixation (ANF) in switchgrass (Panicum virgatum) across a nitrogen input gradient. PLoS ONE 13:e0197320. doi: 10.1371/journal.pone.0197320
Roley, S. S., Ulbrich, T. C., and Robertson, G. P. (2020). Nitrogen fixation and resorption efficiency differences among twelve upland and lowland switchgrass cultivars. Phytobiomes J. 5, 97–107. doi: 10.1094/PBIOMES-11-19-0064-FI
Roley, S. S., Xue, C., Hamilton, S. K., Tiedje, J. M., and Robertson, G. P. (2019). Isotopic evidence for episodic nitrogen fixation in switchgrass (Panicum virgatum L.). Soil Biol. Biochem. 129, 90–98. doi: 10.1016/j.soilbio.2018.11.006
Rowntree, J. E., Stanley, P. L., Maciel, I. C., Thorbecke, M., Rosenzweig, S. T., Hancock, D. W., et al. (2020). Ecosystem impacts and productive capacity of a multi-species pastured livestock system. Front. Sustain. Food Syst. 113, 17–35. doi: 10.3389/fsufs.2020.544984
Ruan, L., Bhardwaj, A. K., Hamilton, S. K., and Robertson, G. P. (2016). Nitrogen fertilization challenges the climate benefit of cellulosic biofuels. Environ. Res. Lett. 11:064007. doi: 10.1088/1748-9326/11/6/064007
Sainju, U. M., Allen, B. L., Lenssen, A. W., and Ghimire, R. P. (2017). Root biomass, root/shoot ratio, and soil water content under perennial grasses with different nitrogen rates. Field Crops Res. 210, 183–191. doi: 10.1016/j.fcr.2017.05.029
Sandor, J. A., Norton, J. E., Hamburg, J. A., Muenchrath, D. A., White, C. S., Williams, S. E., et al. (2007). Biochemical studies of a North American runoff agroecosystem. Geoarchaeology 22, 359–386. doi: 10.1002/gea.20157
Schmer, M. R., Liebig, M. A., Vogel, K. P., and Mitchell, R. B. (2011). Field-scale soil property changes under switchgrass managed for bioenergy. Glob. Change Biol. Bioenergy 3, 439–448. doi: 10.1111/j.1757-1707.2011.01099.x
Schmidt, M., Ikpeng, Y. U., Kayabi, T., Sanchces, R. A., Ono, K. Y., and Adams, C. (2021). Indigenous knowledge and forest succession management in the Brazilian Amazon: contributions to reforestation of degraded areas. Front. For. Glob. Change 4:605925. doi: 10.3389/ffgc.2021.605925
Schneider, K. D., Thiessen Martens, J. R., Zvomuya, F., Reid, D. K., Fraser, T. D., Lynch, D. H., et al. (2019). Options for improved phosphorus cycling and use in agriculture at the field and regional scales. J. Environ. Qual. 48, 1247–1264. doi: 10.2134/jeq2019.02.0070
Schreefel, L., Schulte, R. P. O., De Boer, I. J. M., Schrijver, A. P., and Van Zanten, H. H. E. (2020). Regenerative agriculture – the soil is the base. Global Food Secur. 26:100404. doi: 10.1016/j.gfs.2020.100404
Schulte, L. A., Niemi, J., Helmers, M. J., Liebman, M., Arbuckle, J. G., James, D. E., et al. (2017). Prairie strips improve biodiversity and the delivery of multiple ecosystem services from corn–soybean croplands. PNAS 114, 11247–11252. doi: 10.1073/pnas.1620229114
Seifert, C. A., Roberts, M. J., and Lobell, D. B. (2017). Continuous corn and soybean yield penalties across hundreds of thousands of fields. Agron. J. 109, 541–548. doi: 10.2134/agronj2016.03.0134
Shrestha, B. M., Bork, E. W., Chang, S. X., Carlyle, C. N., Ma, Z., Döbert, T. F., et al. (2020). Adaptive multi-paddock grazing lowers soil greenhouse gas emission potential by altering extracellular enzyme activity. Agronomy 10:1781. doi: 10.3390/agronomy10111781
Shvidenko, A., and Nilsson, S. (2003). A synthesis of the impact of Russian forests on the global carbon budget for 1961–1998. Tellus 55B, 391–415. doi: 10.1034/j.1600-0889.2003.00046.x
Skinner, R. H., Gustine, D. L., and Sanderson, M. A. (2004). Growth, water relations, and nutritive value of pasture species mixtures under moisture stress. Crop Sci. 44, 1361–1369. doi: 10.2135/cropsci2004.1361
Skinner, R. H., Sanderson, M. A., Tracy, B. F., and Dell, C. J. (2006). Above- and belowground productivity and soil carbon dynamics of pasture mixtures. Agron. J. 98, 320–326. doi: 10.2134/agronj2005.0180a
Skold, M. D. (1989). Cropland retirement policies and their effects on land use in the great plains. J. Prod. Agric. 2, 197–201. doi: 10.2134/jpa1989.0197
Smercina, D. N., Evans, S. E., Friesen, M. L., and Tiemann, L. K. (2019). To fix or not to fix: Controls on free-living nitrogen fixation in the rhizosphere. Appl. Environ. Microbiol. 85, e02546–e02518. doi: 10.1128/AEM.02546-18
Smil, V. (2000). Phosphorus in the environment: natural flows and human interferences. Annu. Rev. Energ. Environ. 25, 53–88. doi: 10.1146/annurev.energy.25.1.53
Smith, C. M., David, M. B., Mitchell, C. A., Masters, M. D., Anderson-Teixeira, K. J., Bernacchi, C. J., et al. (2013). Reduced nitrogen losses after conversion of row crop agriculture to perennial biofuel crops. J. Environ. Qual. 42, 219–228. doi: 10.2134/jeq2012.0210
Snapp, S. S., Smith, R. G., and Robertson, G. P. (2015). “Designing cropping systems for ecosystem services,” in The Ecology of Agricultural Landscapes: Long-Term Research on the Path to Sustainability, eds S. K. Hamilton, J. E. Doll, and G. P. Robertson (New York, NY: Oxford University Press), 378–408.
Spehn, E. M., Joshi, J., Schmid, B., Alphei, J., and Körner, C. (2000). Plant diversity effects on soil heterotrophic activity in experimental grassland ecosystems. Plant Soil 224, 217–230. doi: 10.1023/A:1004891807664
Spiegal, S., Bestelmeyer, B. T., Archer, D. W., Augustine, D. J., Boughton, E. H., Boughton, R. K., et al. (2018). Evaluating strategies for sustainable intensification of US agriculture through the long-term agroecosystem research network. Environ. Res. Lett. 13:034031. doi: 10.1088/1748-9326/aaa779
Sprunger, C. D., Culman, S. W., Robertson, G. P., and Snapp, S. S. (2018). How does nitrogen and perenniality influence belowground biomass and nitrogen use efficiency in small grain cereals. Crop Sci. 58, 2110–2120. doi: 10.2135/cropsci2018.02.0123
Sprunger, C. D., Martin, T., and Mann, M. (2020). Systems with greater perenniality and crop diversity enhance soil biological health. Agric. Environ. Lett. 5:e20030. doi: 10.1002/ael2.20030
Sprunger, C. D., and Robertson, G. P. (2018). Early accumulation of active fraction soil carbon in newly established cellulosic biofuel systems. Geoderma 318, 42–51. doi: 10.1016/j.geoderma.2017.11.040
Steward, D. R., Yang, X., Lauwo, S. Y., Staggenborg, S. A., Macpherson, G. L., and Welch, S. M. (2011). From precipitation to groundwater baseflow in a native prairie ecosystem: a regional study of the Konza LTER in the flint hills of Kansas, USA. Hydrol. Earth Syst. Sci. 15, 3181–3194. doi: 10.5194/hess-15-3181-2011
Stockmann, U., Adams, M. A., Crawford, J. W., Field, D. J., Henakaarchchi, N., Jenkins, M., et al. (2013). The knowns, known unknowns, and unknowns of sequestration of soil organic carbon. Agric. Ecosyst. Environ. 164, 80–99. doi: 10.1016/j.agee.2012.10.001
Stubbs, M. (2014). Conservation Reserve Program (CRP): Status and Issues. Congressional Research Service. Available online at https://crsreports.congress.gov/product/details?prodcode=R42783
Subler, S. J., and Uhl, C. F. (1990). “Japanese agroforestry in Amazonia: a case study in Tome-Acu, Brazil,” in Alternatives to Deforestation: Steps Toward Sustainable Use of the Amazon Rain Forest. ed A. B. Anderson, editor (New York, N: Columbia University Press), 152–166.
Swinton, S. M., Lupi, F., Robertson, G. P., and Hamilton, S. K. (2007). Ecosystem services and agriculture: cultivating agricultural ecosystems for diverse benefits. Ecol. Econ. 64, 245–252. doi: 10.1016/j.ecolecon.2007.09.020
Syswerda, S. P., Basso, B., Hamilton, S. K., Tausig, J. B., and Robertson, G. P. (2012). Long-term nitrate loss along an agricultural intensity gradient in the Upper Midwest USA. Agric. Ecosyst. Environ. 149, 10–19. doi: 10.1016/j.agee.2011.12.007
Syswerda, S. P., Corbin, A. T., Mokma, D. L., Kravchenko, A. N., and Robertson, G. P. (2011). Agricultural management and soil carbon storage in surface vs. deep layers. Soil Sci. Soc. Am. J. 75, 92–101. doi: 10.2136/sssaj2009.0414
Syswerda, S. P., and Robertson, G. P. (2014). Ecosystem services along a management gradient in Michigan (USA) cropping systems. Agric. Ecosyst. Environ. 189, 28–35. doi: 10.1016/j.agee.2014.03.006
Teague, R., Grant, B., and Wang, H.-H. (2015). Assessing optimal configurations of multi-paddock grazing strategies in tallgrass prairie using a simulation model. J. Environ. Manage. 150, 262–273. doi: 10.1016/j.jenvman.2014.09.027
Teague, R., Provenza, F., Kreuter, U., Steffens, T., and Barnes, M. (2013). Multi-paddock grazing on rangelands: why the perceptual dichotomy between research results and rancher experience? J. Environ. Manage. 128, 699–717. doi: 10.1016/j.jenvman.2013.05.064
Teague, W. R., Apfelbaum, S., Lal, R., Kreuter, U. P., Rowntree, J., Davies, C. A., et al. (2016). The role of ruminants in reducing agriculture's carbon footprint in North America. J. Soil Water Conserv. 71:156. doi: 10.2489/jswc.71.2.156
Teague, W. R., and Dowhower, S. L. (2003). Patch dynamics under rotational and continuous grazing management in large, heterogeneous paddocks. J. Arid Environ. 53, 211–229. doi: 10.1006/jare.2002.1036
Teague, W. R., Dowhower, S. L., Baker, S. A., Haile, N., Delaune, P. B., and Conover, D. M. (2011). Grazing management impacts on vegetation, soil biota and soil chemical, physical and hydrological properties in tall grass prairie. Agric. Ecosyst. Environ. 141, 310–322. doi: 10.1016/j.agee.2011.03.009
Tiemann, L. K., and Grandy, A. S. (2015). Mechanisms of soil carbon accrual and storage in bioenergy cropping systems. Glob. Change Biol. Bioenergy 7, 161–174. doi: 10.1111/gcbb.12126
Tiemann, L. K., Grandy, A. S., Atkinson, E. E., Marin-Spiotta, E., and Mcdaniel, M. D. (2015). Crop rotational diversity enhances belowground communities and functions in an agroecosystem. Ecol. Lett. 18, 761–771. doi: 10.1111/ele.12453
Tilman, D., and Downing, J. A. (1994). Biodiversity and stability in grasslands. Nature 367, 363–365. doi: 10.1038/367363a0
Tilman, D., Hill, J., and Lehman, C. (2006). Carbon-negative biofuels from low-input high-diversity grassland biomass. Science 314, 1598–1600. doi: 10.1126/science.1133306
Tufekcioglu, A., Raich, J. W., Isenhart, T. M., and Schultz, R. C. (2003). Biomass, carbon and nitrogen dynamics of multi-species riparian buffers within an agricultural watershed in Iowa, USA. Agrofor. Syst. 57, 187–198. doi: 10.1023/A:1024898615284
U.S. Department of Agriculture (2006). Agriculture Conservation Reserve Protection Program (CRP). Washington, DC: USDA Farm Service Agency.
U.S. Department of Energy (2011). U.S. Billion-Ton Update: Biomass Supply for a Bioenergy and Bioproducts Industry, eds R. D. Perlack, and B. J. Stokes. Oak Ridge, TN: Oak Ridge National Laboratory.
Undersander, D., Albert, B., Cosgrove, D., Johnson, D., and Peterson, P. (2002). Pastures for Profit: A Guide to Rotational Grazing. Cooperative Extension Publishing: No. A3529. Madison, WI: University of Wisconsin Extension.
van der Heijden, M. G. A., Bardgett, R. D., and Van Straalen, N. M. (2008). The unseen majority: soil microbes as drivers of plant diversity and productivity in terrestrial ecosystems. Ecol. Lett. 11, 296–310. doi: 10.1111/j.1461-0248.2007.01139.x
Vergutz, L., Manzoni, S., Porporato, A., Novais, R. F., and Jackson, R. B. (2012). Global resorption efficiencies and concentrations of carbon and nutrients in leaves of terrestrial plants. Ecol. Monogr. 82, 205–220. doi: 10.1890/11-0416.1
Vitousek, P. M., and Reiners, W. A. (1975). Ecosystems succession and nutrient retention: a hypothesis. BioScience 25, 376–381. doi: 10.2307/1297148
Vuichard, N., Ciais, P., Belelli, L., Smith, P., and Valentini, R. (2008). Carbon sequestration due to the abandonment of agriculture in the former USSR since 1990. Global Biogeochem. Cycles 22:GB4018. doi: 10.1029/2008GB003212
Wang, T., Jin, H., Kreuter, U., and Teague, R. (2021). Expanding grass-based agriculture on marginal land in the U.S. Great Plains: The role of management intensive grazing. Land Use Policy 104:105155. doi: 10.1016/j.landusepol.2020.105155
Wang, T., Richard Teague, W., Park, S. C., and Bevers, S. (2018). Evaluating long-term economic and ecological consequences of continuous and multi-paddock grazing - a modeling approach. Agric. Syst. 165, 197–207. doi: 10.1016/j.agsy.2018.06.012
Wei, J., Cheng, J., Li, W., and Liu, W. (2012). Comparing the effect of naturally restored forest and grassland on carbon sequestration and its vertical distribution in the Chinese Loess Plateau. PLoS ONE 7:e40123. doi: 10.1371/journal.pone.0040123
Werling, B. P., Dickson, T. L., Isaacs, R., Gaines, H., Gratton, C., Gross, K. L., et al. (2014). Perennial grasslands enhance biodiversity and multiple ecosystem services in bioenergy landscapes. PNAS 111, 1652–1657. doi: 10.1073/pnas.1309492111
Wu, J., and Weber, B. (2012). Implications of a Reduced Conservation Reserve Program. Washington, DC: Council on Food, Agriculture & Resource Economics.
Yang, J., and Udvardi, M. (2018). Senescence and nitrogen use efficiency in perennial grasses for forage and biofuel production. J. Exp. Bot. 69, 855–865. doi: 10.1093/jxb/erx241
Yang, J., Worley, E., Wang, M., Lahner, B., Salt, D. E., Saha, M., et al. (2009). Natural variation for nutrient use and remobilization efficiencies in switchgrass. Bioenergy Res. 2, 257–266. doi: 10.1007/s12155-009-9055-9
Young, C. E., and Osborn, C. T. (1990). The Conservation Reserve Program: An Economic Assessment. Washington, DC: Economic Research Service, USDA.
Zak, D. R., Holmes, W. E., White, D. C., Peacock, A. D., and Tilman, D. (2003). Plant diversity, soil microbial communities, and ecosystem function: Are there any links? Ecology 84, 2042–2050. doi: 10.1890/02-0433
Zhang, C., Liu, G., Xue, S., and Zhang, C. (2012). Rhizosphere soil microbial properties on abandoned croplands in the Loess Plateau, China during vegetation succession. Eur. J. Soil Biol. 50, 127–136. doi: 10.1016/j.ejsobi.2012.01.002
Keywords: degraded lands, marginal lands, soil fertility, soil carbon, soil nitrogen, soil phosphorus, bioenergy, rotational grazing
Citation: Mosier S, Córdova SC and Robertson GP (2021) Restoring Soil Fertility on Degraded Lands to Meet Food, Fuel, and Climate Security Needs via Perennialization. Front. Sustain. Food Syst. 5:706142. doi: 10.3389/fsufs.2021.706142
Received: 06 May 2021; Accepted: 09 September 2021;
Published: 11 October 2021.
Edited by:
Jennifer Blesh, University of Michigan, United StatesReviewed by:
Timothy Crews, The Land Institute, United StatesCopyright © 2021 Mosier, Córdova and Robertson. This is an open-access article distributed under the terms of the Creative Commons Attribution License (CC BY). The use, distribution or reproduction in other forums is permitted, provided the original author(s) and the copyright owner(s) are credited and that the original publication in this journal is cited, in accordance with accepted academic practice. No use, distribution or reproduction is permitted which does not comply with these terms.
*Correspondence: Samantha Mosier, bW9zaWVyczFAbXN1LmVkdQ==
Disclaimer: All claims expressed in this article are solely those of the authors and do not necessarily represent those of their affiliated organizations, or those of the publisher, the editors and the reviewers. Any product that may be evaluated in this article or claim that may be made by its manufacturer is not guaranteed or endorsed by the publisher.
Research integrity at Frontiers
Learn more about the work of our research integrity team to safeguard the quality of each article we publish.