- 1Department of Agriculture and Animal Health, Florida Science Campus, University of South Africa, Roodepoort, South Africa
- 2Department of Plant and Food Sciences, School of Agricultural and Food Sciences, Jaramogi Oginga Odinga University of Science and Technology, Bondo, Kenya
- 3Department of Environmental Science, Florida Science Campus, University of South Africa, Roodepoort, South Africa
- 4Faculty of Natural and Agricultural Sciences, North West University, Potchefstroom, South Africa
Environmental degradation related to mining-generated acid mine drainage (AMD) is a major global concern, contaminating surface and groundwater sources, including agricultural land. In the last two decades, many developing countries are expanding agricultural productivity in mine-impacted soils to meet food demand for their rapidly growing population. Further, the practice of AMD water (treated or untreated) irrigated agriculture is on the increase, particularly in water-stressed nations around the world. For sustainable agricultural production systems, optimal microbial diversity, and functioning is critical for soil health and plant productivity. Thus, this review presents up-to-date knowledge on the microbial structure and functional dynamics of AMD habitats and AMD-impacted agricultural soils. The long-term effects of AMD water such as soil acidification, heavy metals (HM), iron and sulfate pollution, greatly reduces microbial biomass, richness, and diversity, impairing soil health plant growth and productivity, and impacts food safety negatively. Despite these drawbacks, AMD-impacted habitats are unique ecological niches for novel acidophilic, HM, and sulfate-adapted microbial phylotypes that might be beneficial to optimal plant growth and productivity and bioremediation of polluted agricultural soils. This review has also highlighted the impact active and passive treatment technologies on AMD microbial diversity, further extending the discussion on the interrelated microbial diversity, and beneficial functions such as metal bioremediation, acidity neutralization, symbiotic rhizomicrobiome assembly, and plant growth promotion, sulfates/iron reduction, and biogeochemical N and C recycling under AMD-impacted environment. The significance of sulfur-reducing bacteria (SRB), iron-oxidizing bacteria (FeOB), and plant growth promoting rhizobacteria (PGPRs) as key players in many passive and active systems dedicated to bioremediation and microbe-assisted phytoremediation is also elucidated and discussed. Finally, new perspectives on the need for future studies, integrating meta-omics and process engineering on AMD-impacted microbiomes, key to designing and optimizing of robust active and passive bioremediation of AMD-water before application to agricultural production is proposed.
Introduction
Globally, large-scale commercial mining operations and other related industries generate various waste streams, that constitute one of the major anthropogenic sources of environmental pollution. Mining is associated with significant production of large volumes of toxic acidic water with elevated heavy metals (HM) and sulfates known as acid mine drainage (AMD) (Rambabu et al., 2020). Acid mine drainage has become a serious global issue in recent times owing to its hazardous impact on the environment and living organisms (Kefeni et al., 2017; Grande et al., 2018; Steyn et al., 2019; Rezaie and Anderson, 2020). In the mining regions of Australia (Lei et al., 2010), Brazil (Galhardi and Bonotto, 2016), Canada (Sracek et al., 2004), England, Wales, Spain, Norway (Hallberg, 2010; Romero et al., 2011), Morocco (Boularbah et al., 2006), South Africa (Ochieng et al., 2010), China (Wu et al., 2009; Wang et al., 2021b), and United States of America (Blowes et al., 2005; Acharya and Kharel, 2020) concern over AMD pollution has been reported, with inactive and abandoned mines generating huge quantities of AMD that contaminates both terrestrial and aquatic systems including agricultural soils, ground-, and surface-water sources (Rezaie and Anderson, 2020). The negative effects of phytotoxicity-related AMD acidity and HM contamination of agricultural soil and crops have been documented (Netto et al., 2013; Liao et al., 2016), the HM entering the food chain through the food crop uptake posing a potential health risk to human population (Kumar et al., 2020; Massányi et al., 2020).
With an increased public health concern and ecological awareness, stricter legislative control on mine water/waste management and remediation strategies are being implemented in many countries to counter the environmental hazards posed by AMD. Furthermore, the increased interest in the beneficial use of mine-impacted water for irrigation, especially in water-stressed regions in the world, imply remediation to meet the set water quality regulatory standard is key for long term sustainable application of the AMD wastewater in agricultural production (Annandale et al., 2006). Toward this, various treatment methods that cut across active and passive treatment methods have been adopted by many countries in the treatment of AMD. These range from conventional active treatment methods employing various physical and chemical approaches such as neutralization, adsorption, ion exchange, membrane technology, and electrochemical approaches. The active treatment of AMD is based on the principle of increasing the pH to precipitation of HM and sulfates before discharging into environment. In contrast, passive treatment involves biological treatment under natural or constructed wetlands through the aid of both plants, aerobic and anaerobic microorganisms, algae strains, and other macroorganisms (Bwapwa et al., 2017; Park et al., 2019; Rambabu et al., 2020) to accelerate conversion HM and sulfates to an acceptable form with least or null environmental impact.
Detailed reports of AMD origin and different processes contributing to AMD generation, including the role of iron- and/or sulfur-oxidizing microbes are available in literature (Baker and Banfield, 2003; Hallberg, 2010; Chen et al., 2016). In many AMD sites, oxidation is primarily catalyzed by naturally occurring bacteria called Acidithiobacillus ferrooxidans (Chen et al., 2015; Mesa et al., 2017; Bomberg et al., 2019; Wang et al., 2019b) and these bacteria accelerate the oxidation reactions for sulfides of most HM (Akcil and Koldas, 2006). According to Edwards et al. (2000), about 75% of AMD produced results from microbial activity. Under the extremophilic AMD environment, acidophilic microbial communities belonging to Bacteria, Archaea, and Eukarya domains dominates, with members of phylum Proteobacteria, Nitrospira, Actinobacteria, Firmicutes, and Acidobacteria being the dominant bacterial taxa (Mesa et al., 2017; Lukhele et al., 2019; Distaso et al., 2020), as well as iron/sulfur-oxidizing microbes such Leptospirillum ferrooxidans, Ferrovum, Acidothiobacillus occurring in an environmentally dependent biogeographic pattern (Kuang et al., 2013, 2016; Wang et al., 2019b). In addition, a large number of rare taxa constituting a “rare biosphere” in the microbial community that may perform crucial function in AMD ecosystems have been reported (Luo et al., 2020b). Collectively, AMD environments are unique ecological niches with significantly reduced species richness and diversity, but enriched with sulfate-, acid-, and toxic-metals-adapted microorganisms. However, remediation treatments of AMD targeting increasing pH, HM, and sulfate removal is associated with shifts in microbial diversity and structure from acidophilic microbial communities to a more metabolically diverse set of taxa and from a less sulfur-reducing bacteria (SRB) to a more SRB.
In the last few decades significant efforts have been made to increase agricultural productivity to meet food security for the ever increasing global population. In water stressed mining nations, where the pressures on agricultural lands and water resources are extremely great amid rapid population growth, the use of AMD water and AMD-affected streams for irrigated agriculture and expansion of agriculture on mining impacted land is common (Jovanovic et al., 1998; Lin et al., 2005; Vicente-Beckett et al., 2016; Musvoto and de Lange, 2019). This implies that the application of AMD water for irrigated agriculture have the potential to alter the soil microbiome which could negatively impact soil health, plant growth and productivity of the crops. This review provides an overview of the microbial community structure and diversity of AMD wastewater, highlighting the potential positive and negative roles associated microbiome may play under AMD-irrigated agricultural production. Furthermore, the understanding of the enriched microbial groups in the treated AMD that could give an insight into their roles and potential application in the bioremediation of polluted agricultural land and possibly enhance sustainable agricultural productivity are discussed.
Environmental and Health Impacts of AMD Pollution
Acid mine drainage (AMD) is a global environmental issue negatively impacting many mining-associated ecological system, including prokaryotic and eukaryotic life. From the standpoint of global food security, mining activities, and its associated AMD pollution is causing massive disturbances of aquatic resources and land-use changes that are significantly altering agricultural productivity and food quality (Choudhury et al., 2017). Generally, AMD threat to ecological systems, agricultural land, and human health is attributable to its high acidity and non-biodegradable HM contamination in living organisms and food chains. For example, small-scale mining site in Ghana caused Pb, Hg, Cd, and K pollution of rivers used in crop irrigations (Nukpezah et al., 2017). In Portugal, AMD contributes to higher levels of , Fe, Al, as well as Cu, Pb, Zn, Cd, and As pollution of irrigation water sources in Lousal area (Luís et al., 2009). Downstream water sources and agricultural land laden with high concentration of Pb, Cu, and Zn as impacted by AMD has also been reported in China (Wu et al., 2009; Cao et al., 2019; Luo et al., 2020a), India (Sahoo et al., 2017; Mohanty et al., 2018; Dutta et al., 2020), and USA (Acharya and Kharel, 2020).
Acid mine drainage also severely affects aquatic and terrestrial life ecosystem. Specifically, contaminations of the aquatic systems by HM can stimulate the production of reactive oxygen species (ROS) that can damage fishes, aquatic macroinvertebrates, and other organisms (Jennings et al., 2008; Gomez Isaza et al., 2020). Furthermore, acidification affects the structure and functioning of ecosystems (Carlson, 2013; Bonilla et al., 2018; Simate, 2021). Bioaccumulation and biotransformation of toxic HM and metalloids (e.g., As, Pb, Cr, Cd, and Hg) along the food chains that may constitute a serious public health risk associated with AMD pollution have also been reported in literature (Emmanuel et al., 2018; Darko et al., 2019; Gwenzi, 2020; Chan et al., 2021; Yang et al., 2021). The main effects of HM on human health range from bronchitis, skin and bladder cancer, liver and kidney failure, to mental retardation in children (Rambabu et al., 2020).
In the latter subsections, we focus on the prokaryotic structure and functioning providing a comprehensive assessment of the main implications and challenges of using untreated AMD wastewater for irrigated agricultural production. The discussion is further extended to the impact of active and passive treatment systems on the microbial community composition and function that may beneficial in the use AMD wastewater for agricultural production and bioremediation.
Microbial Community Diversity Under AMD Habitats
In the last three decades, advances in 16S rRNA gene and meta-omics based molecular analyses in combination with culture-dependent approaches has drastically enlarged knowledge on the microbial diversity and functioning of AMD microenvironments as shaped by environmental factors such as very low pH, high concentrations of dissolved HM and other solutes, low total organic carbon (TOC), and dissolved oxygen (DO). There are excellent articles that has thoroughly reviewed AMD ecosystems as extreme habitats that provides multiple niches for distinct bacterial, archaeal, and eukaryotic microbiomes (Baker and Banfield, 2003; Méndez-García et al., 2015; Chen et al., 2016; Mesa et al., 2017; Lukhele et al., 2020). In these reports, the dominance of members of Eukarya domain, including fungal and algal taxa has been reported in diverse AMD sites across the globe (Baker et al., 2009). For example, members of Stramenopiles, Alveolata, Rhizaria, and Opisthokonta was also observed as the dominating microbes in abandoned mercury mine at Los Rueldos (NW Spain) (Mesa et al., 2017). Acidea extrema, Acidiella bohemica, Acidiella uranophila, Acidomyces acidophilus, Acidomyces acidothermus, Acidothrix acidophila, Coniochaeta fodinicola, Hortaea acidophila, and Soosiella minima are fungal species has reported to be enriched in acidic habitat (Selbmann et al., 2008; Vázquez-Campos et al., 2014). Most recently, Kalu et al. (2021) revealed Ascomycota and Basidiomycota as the dominant phyla in two gold mining sites in Gauteng Province of South Africa using high-throughput sequencing technology. Prasanna et al. (2011) also reported the enrichment of AMD with algal communities such as Euglena sp. and Ulothrix sp. as well as unicellular microalgae such as Chlorella, Cylindrocystis, Botryococcus, and Navicula and several filamentous forms identified as Microspora, Cladophora, and Binuclearia. These studies indicates that diversity of organisms within the Eukarya domain is dependent on the AMD from different mining sites.
Similarly, different studies have unveiled the diversity of bacterial and archaeal communities that are enriched in AMD sites. Collated information of the bacterial communities dominating diverse AMD polluted environment show that Proteobacteria, Nitrospirae, Acidobacteria, Chloroflexi, and Actinobacteria are the dominant phyla (Kuang et al., 2013; Méndez-García et al., 2015; Lukhele et al., 2019; Wang et al., 2019b). Among the key dominant bacteria taxa, acidophilic proteobacterial taxa such as Acidithiobacillus spp, Leptospirillum spp., Acidiphilum spp., and Ferrovum spp, play important role chemolithotrophic metabolism of iron and sulfur under AMD environment (Kuang et al., 2013; Méndez-García et al., 2015; Wang et al., 2019b). However, there is evidence of uniqueness of the mines with respect to the bacterial diversities and functioning capabilities of autochthonous taxa related to biogeochemical recycling of iron, sulfur, nitrogen, carbon, and oxygen. In Yunfu sulfide mine in Guangdong province, China, Nitrospira, Aphaproteobacteria, Betaproteobacteria, and Gammaroteobacteria bacterial families as well as Acidithiobacillus and Gallionella genera were identified as the major dominating bacterial communities (He et al., 2007). Acidiphilium spp. in pyrite mine tailings and AMD lake in Anhui Province, China (Hao et al., 2010; Xin et al., 2021), Proteobacteria, Firmicutes, and Planctomycetes including Marinobacteria spp. and Anabaena spp. in deep mines, tailings and mine-water from AMD dams in South Africa (Keshri et al., 2015; Lukhele et al., 2019; Sibanda et al., 2021). Novel species belonging to Leptospirillum spp., Acidiphilum spp., Acidithiobacillus spp., and Ferrovum spp., including other members of Alphaproteobacteria, Euryarchaeota, Gammaproteobacteria, and Nitrospira in AMD sites across Southeast China has been reported (Kuang et al., 2013, 2016). The dominance of Acidithiobacillus ferrivorans, Acidobacteria, Actinobacteria, and Chloroflexi in Svalbard (García-Moyano et al., 2015). In contrast, members of phylum Crenarchaeota and Thaumarchaeota are the important archaeal taxa with Thermoplasmatales and Ferroplasma playing key roles in metabolism and element cycling in a number of AMD systems. For example, Thermoplasmatales dominates AMD from Dabaoshan Mine (Guangdong Province, China) (Lu et al., 2010). Distaso et al. (2020) and Korzhenkov et al. (2019) also recently reported higher representation of archaea Thermoplasmatales across all depths in oxic and low-pH sediment layers underlying an acidic stream in Parys Mountain (Mynydd Parys) AMD habitat in the UK, indicating contribution of these organisms to carbon, and probably to iron and sulfur cycles in this ecosystem. Gupta et al. (2021) also reported the dominant archaeal communities in AMD sediment of Malanjkhand Copper Project, India as Thermoplasmata, Nitrososphaeria, Bathyarchaeia, Hydrothermarchaeota, Methanomassiliicoccales, Methanobacteriaceae, Methanocellaceae, Haloferaceae, Methanosaetaceae, and Methanoregulaceae. In this study, members of the Thermoplasmata present in Malanjkhand AMD were reported to be mostly involved in chemoheterotrophy, Fe/S redox cycling, and with HM resistance, while the Nitrososphaeria members participate in ammonia oxidation and fixation at low pH and oligotrophic environment and subsequently play an important role in nitrification process in AMD sediment. Table 1 summarizes various microbial communities associated with diverse AMD systems in different countries.
AMD Remediation Technologies and Microbial Community Structure
Due to its detrimental toxicological impact to environments, several AMD remediation technologies have been proposed or are being used for remediation of AMD water and AMD-polluted soils. This includes an assortment of passive treatment technologies involving processes that combine geochemical and biological activities in the remediation of AMD wastewater as well as mine waters to improve the water quality are being applied across the globe (Rambabu et al., 2020). In contrast, active treatment processes involving the use alkaline reagents to precipitate metals, adsorption, ion exchange, and systems with membranes in constructed plant to remediate contaminated AMD water (RoyChowdhury et al., 2015; Rodríguez-Galán et al., 2019). Comparatively, a high quality of treated effluent can be considered as a great advantage of active treatment over passive treatments, however, passive treatment technologies have found wider use due to low operational and labor costs, easy process design and control, and with better sulfate and metal recovery (Rambabu et al., 2020).
The key goal for both remediation systems is to remove HM and sulfates from the acidic AMD and to increase pH level. To date, some of the used methods range from limestone, lime, flyash neutralization (Watten et al., 2005; Tolonen et al., 2014; Iakovleva et al., 2015), phytoremediation and use of wetlands (RoyChowdhury et al., 2015; Ding and Sun, 2021), adsorption (Gitari, 2014; Kim et al., 2021), permeable reactive barriers (Obiri-Nyarko et al., 2014) sulfidogenic bioreactors (Panda et al., 2016; Aoyagi et al., 2018), among others. Each of these technologies are characterized by pros and cons based on running cost, efficiencies, and generation of effluent sludge. Furthermore, the effectiveness of these technologies/systems are influenced by the types of pollution, amounts of pollutants, and activities of microorganisms and the aquatic plants that present in the AMD sites. More importantly, removal of HM and sulfates and neutralization of acidity by the treatment technologies may be associated with perturbations of the autochthonous AMD microbial structural diversity and functioning dependent on the type of treatment method applied. Despite limited data on the impacts of AMD treatment systems on the microbial communities, accumulating evidence indicates a general shift from acidophilic and less sulfur-reducing microbial communities upon treatment to a more metabolically diverse and SRB and iron-reducing (FeOB) bacteria set of taxa being reported (Müller et al., 2015; Aoyagi et al., 2018; Dutta et al., 2020). Table 2 below summarizes the microbial diversity of AMD sites and agricultural soils treated using passive and active technologies.
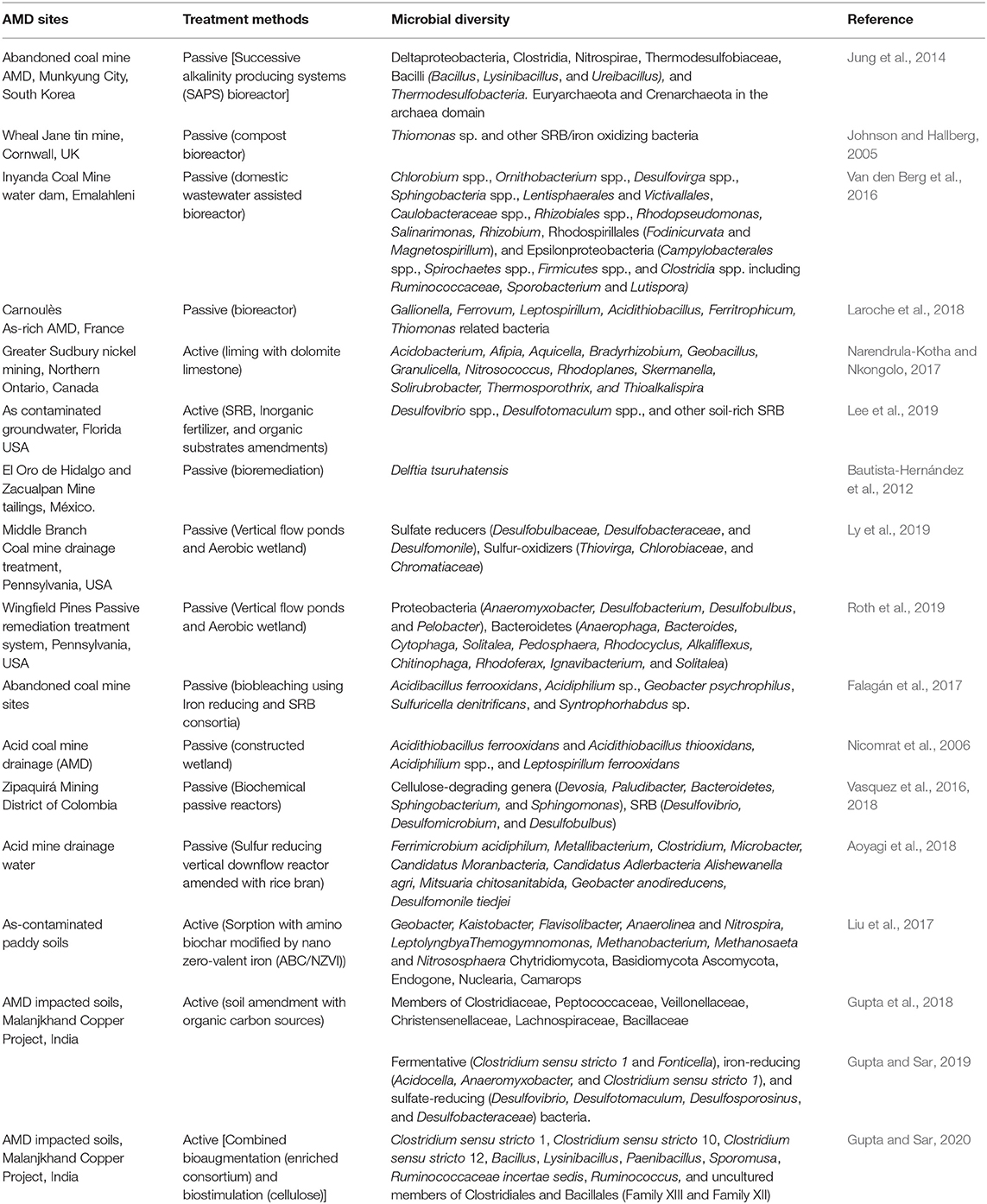
Table 2. Summary of the microbial diversity in selected passive and active treated mining AMD sites and agricultural soils.
In passive successive alkalinity-producing system (SAPS) AMD treatment, enrichment of Dsr-AB type dissimilatory reductase based bacteria comprising Clostridia and Deltaproteobacteria as well as Bacilli, Nitrospirae, and Thermodesulfobacteria, with higher levels acidity and metals SAPS bioreactor having a more diverse (i.e., higher Shannon, Shannon, and inverse Simpson indices), and evenly distributed bacterial community (i.e., higher ACE and Chao indices) (Jung et al., 2014). Passive treatment of As-rich AMD via iron biological oxidation showed that the biogenic precipitate formed is dominated by iron-oxidizing bacteria (FeOB) such as Gallionella, Ferrovum, Leptospirillum, Acidithiobacillus, and Ferritrophicum, and arsenite-oxidizing Thiomonas spp. (Laroche et al., 2018). Similarly, passive bioremediation system enriched with Ignavibacterium, Pelotomaculum, and Petrimonas and species known to catalyse the dissimilatory reduction of ferric iron (Geobacter psychrophilus), oxidation of sulfur (Polaromonas hydrogenivorans, Flavobacterium johnsoniae, Dechloromonas aromatica, Novosphingobium sediminicola, Clostridium saccharobutylicum, and Pseudomonas extremaustralis), and reduction of nitrate (Sulfuricella denitrificans, A. ferrooxidans, and Acidithiobacillus thiooxidans), methylotrophs (methane/methanol-oxidizers), and anaerobic aromatic compound-degraders (Syntrophorhabdus sp.) are enriched at the end of the remediation process (Nicomrat et al., 2006; Falagán et al., 2017; Vasquez et al., 2018; Roth et al., 2019). A down flow structured bed bioreactor (DFSBR) treatment of synthetic AMD treatment using sugar vinasse as an electron donor for SRB, resulted in efficient metal sulfide precipitation attributed to high microbial diversity and syntrophism of sulfate and metal reducers Geobacter and Desulfovibrio and fermenters such as Parabacteroides and Sulfurovum (Nogueira et al., 2021).
Comparatively, there is limited number of studies on the impact of active treatment technologies on the microbial community structure of AMD-polluted habitats and water. Currently, bulk of work done focuses mainly on the impact of the active treatment technologies on physiochemical components such pH, and HM components of the treated AMD (Othman et al., 2017), as well as their influence in promoting the growth of crops (Choudhury et al., 2017; Nkongolo et al., 2017; Madiseng, 2018; Narendrula-Kotha et al., 2019). However, available literature indicate that active treatments technologies also exert variable influence on microbial diversity of AMD wastewater and contaminated soils. For example, Pang et al. (2019) observed increased species richness and the enrichment of members of Acidobacteria and Chloroflexi (Bacteria domain) and symbiotrophs fungal communities (Eukarya domain) upon liming treatment of sugarcane farm impacted by AMD. In this study, consecutive liming was associated improvement on microbial community richness and evenness, their functions, soil nutrient status, and crop yield in a sugarcane cropping system. Similarly, Narendrula-Kotha and Nkongolo (2017) also detected dominance of bacterial phyla such as Actinobacteria, Acidobacteria, Chloroflexi, Firmicutes, and Proteobacteria and fungal phyla Basidiomycota in limed than unlimed mining-impacted soils. The authors observed higher microbial diversity and biomass in the limed than the unlimed sites, with Bradyrhizobiaceae family including the nitrogen fixing Bradyrhizobium genus was more abundant (representing 50% relative abundance) in limed sites compared to unlimed areas. However, Ascomycota was the most predominant fungal phylum in unlimed soils (46%) while Basidiomycota phylum represented 86% of all fungi in the limed areas. Quicklime treatment (at rate 0.75 g.kg−1) (pH <5) impact positively the bacterial and fungal diversity of strongly acidic soils of tobacco plants, enrichment of bacterial genera Rhodanobacter, Gaiellales, Streptomyces, and Terrabacter. Mortierella, Penicillium, Trichoderma, Aspergillus, Talaromyces, and Fusarium were the abundant fungal genera after 50th day treatment (Liang et al., 2021). Several studies have also reported increase in the bacterial and fungal community diversity in acid/metal-contaminated soils after treatment with fly ash (Garcia-Sánchez et al., 2015; Leclercq-Dransart et al., 2019).
Microbial Diversity Changes in AMD-Contaminated Agricultural Soil
Many studies have demonstrated that irrigation AMD water could result in the shift of soil basic characteristics that may influence microbial diversities that play pivotal ecological roles related to bulk and rhizospheric soil health (Wang et al., 2018, 2019a; Narendrula-Kotha et al., 2019; Wu et al., 2019; Li et al., 2020; Xin et al., 2021). The changes in microbial diversity are influenced by the AMD-induced changes, with the bacterial and archaeal community diversity majorly dependent on soil pH and HM content, respectively (Wang et al., 2018; Li et al., 2020). Heavy metals toxicity may manifest through alteration of physiological and biochemical properties of microorganisms through various mechanisms such as nucleic acid structure damage leading to functional disturbance, disruption of cell membranes, enzymatic activity inhibition, and oxidative phosphorylation, increased ROS inducing oxidative damage, and impaired ion regulation (Jacob et al., 2018; Medfu Tarekegn et al., 2020). Overall, richness and alpha diversity of AMD associated microbial communities vary widely across the type of ecosystem, with communities inhabiting the AMD water being less diverse than those from impacted soils, sediments, or bioreactors from AMD treatment (Villegas-Plazas et al., 2019). Interestingly, community-level functional acclimatization occurs in response to the AMD perturbation despite the extreme condition of the acidity and high concentrations of toxic metal and sulfate.
From sustainable agricultural production and biotechnological standpoint, the enrichment of soil acidophilic, HM, and sulfate-adapted microbial taxa contributed by both AMD water and autochthonous soil microbiota that might play beneficial interrelated functions key to optimal plant growth and productivity and bioremediation of AMD-polluted agricultural soils. For example, AMD irrigation of paddy rice considerably enriched the bacterial phylum Acidobacteria and the archaeal phylum Crenarchaeota correlating to AMD-related environmental factors of pH and HM (Cu, Pb, and Zn) (Wang et al., 2018). Notably, the dominance of Acidobacterial genera Candidatus Solibacter and Candidatus Koribacter in the highly contaminated sites were key to stabilization of the energy metabolic processes related to C/N related pathways under AMD contamination. Similarly, the increased of metabolic activities that generate alkalinity and the abundance and the diversity of SRB and iron-reducing bacteria in the long-term AMD-irrigated paddy soil has also been reported (Wang et al., 2016, 2021a; Ding et al., 2017). In summary, the key beneficial and interrelated microbial functions under AMD pollution can largely be grouped into: (i) metal bioremediation; (ii) plant growth promotion; and (iii) sulfates/iron reduction and biogeochemical N and C recycling. Figure 1 provides the schematic representation of the diverse microbial diversity and functions key to plant growth and productivity under AMD-contaminated agricultural soils.
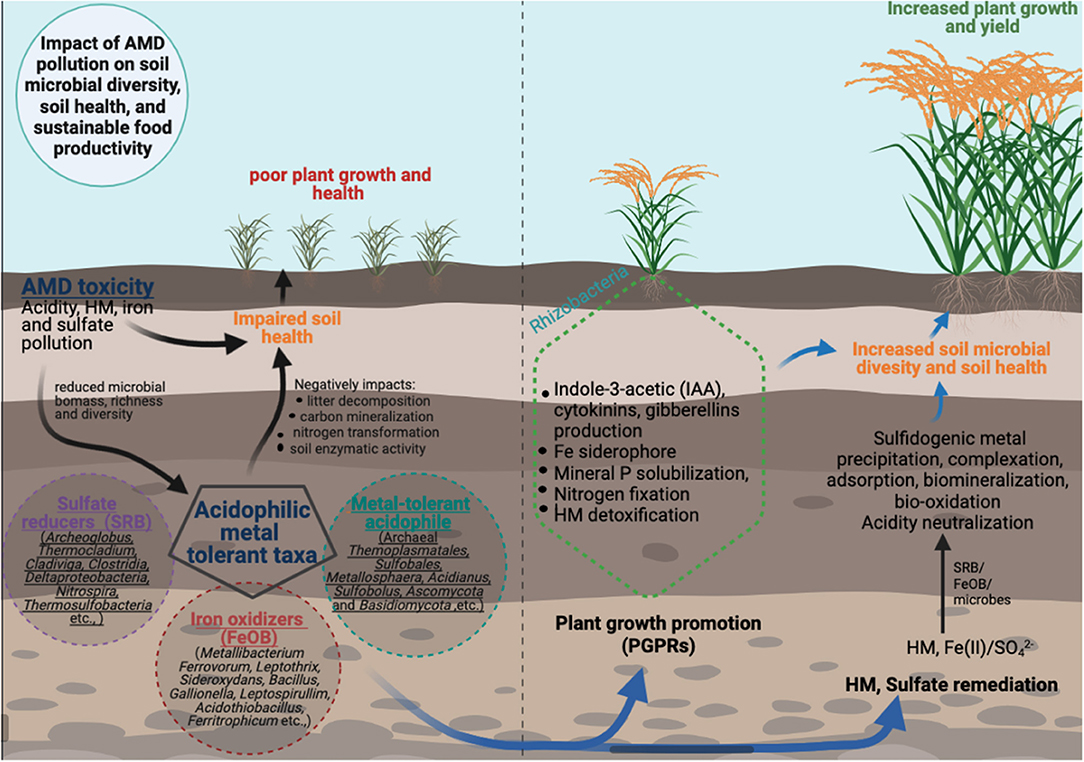
Figure 1. Long term effects of AMD pollution on agricultural soil health, microbial diversity/functions, and plant growth productivity. The potential beneficial functions/applications of adapted autochthonous microbial taxa in improving AMD-impacted soils health and microbial diversity for sustainable agricultural production systems is also illustrated (SRB,sulfate reducing bacteria; FeOB, iron oxidizing bacteria).
HM Bioremediation and Plant Growth Promoting Activity
Soil microbiota, particularly rhizomicrobime, play a critical role in metal bioremediation contaminated soils due to their ability to degrade, detoxify, and even accumulate HM, with metal-microbe interactions important in reducing HM stress in plants. In AMD-polluted habitats and agricultural soils, selection, and enrichment of microbes having high metabolic capacity supported by molecular machinery to adapt and perform in the presence of high concentration of HM is common feature. The adaptability of these microorganisms indicates their potential bioremediation application for rehabilitation of AMD-irrigated paddy soil and mined sites. The inherent metal-microbe and plant–microbe interactions are important to protect the plant from HM stress as well as help in natural attenuation of HM in soil (Mishra et al., 2017; Henao and Ghneim-Herrera, 2021). Generally, these microorganisms utilize various mechanisms ranging from biosorption (including precipitation, chemical adsorption, and ion exchange, surface precipitation, complexation to organic ligands, and redox reactions), biomineralization (bioleaching, dissolution, or complexation), to bio-oxidation (Jin et al., 2018; Hou et al., 2020).
Both bulk soil and plant-associated microbes play critical role in bioremediation and microbe-assisted phytoremediation of HM under AMD conditions. Notably, higher abundance of members of bacterial phyla Actinobacteria, Proteobacteria, Firmicutes, and Bacteroidetes and archaeal phyla Euryarchaeota and Crenarchaeota in the rhizosphere of plants growing in AMD-polluted and other extreme environments has attracted great attention in the last three decades. Specifically, the potential application of metal-tolerant plant growth promoting rhizobacteria (PGPRs) has become attractive as an efficient bio-inoculants for metal remediation for sustainable agriculture (Backer et al., 2018). The PGPRs generally play key roles in nutrient acquisition and assimilation, toxic HM bioremediation, improved soil texture, secreting, and modulating extracellular molecules such as hormones, secondary metabolites, antibiotics, and various signal compounds, these attributes collectively contributing to enhancement of plant adaptability to metal stress and productivity (Mishra et al., 2017). Li and Ramakrishna (2011) reported improved growth and copper uptake by maize (Zea mays) and sunflower (Helianthus annuus) under HM pollution in presence of copper resistant Pseudomonas strains exhibiting higher Zn and Pb bioaccumulation and plant growth promoting indole-3-acetic acid (IAA), iron chelating siderophore, and solubilization of mineral phosphate and metals ability. The use of copper-tolerant rhizobacteria Brevundimonas diminuta MYS6 and Pseudomonas vermicola to efficiently remove Cu improving plant growth, biomass and chlorophyll under Cu stress in sunflower (Rathi and Yogalakshmi, 2021) and lentils (Islam et al., 2016), respectively, has also been reported. Wu et al. (2019) also isolated indigenous HM-resistant plant growth-promoting bacteria (PGPRs) genera Burkholderia, Paraburkholderia, Cupriavidus, Pseudomonas, and Ralstonia AMD-impacted farmlands in Le'an River basin, China. Two bacterial strains Burkholderia sp. strain S6-1 and Pseudomonas sp. strain S2-3 possessed both greater PGP activities and metal-resistant characteristics significantly increased the height, dry weight and N uptake of sorghum (Sorghum bicolor L.). The importance of PGPR-mediated metal phytoremediation by various plant species under wetland and agricultural ecosystems impacted by AMD have also been documented (Checcucci et al., 2017; Mishra et al., 2017; Song et al., 2020). These examples illustrate AMD-impacted soils PGPRs as potential tools for sustainable agriculture.
Sulfate-Reducing and Iron-Reducing Activities
Acid mine drainage-impacted habitats and agricultural soils is also rich in Fe- and S-metabolizing bacteria that may play key role in bioremediation. Generally, SRB oxidize simple organic molecules using the sulfate ion as an electron acceptor producing hydrogen sulfide (H2S) and the bicarbonate ions () that are effective in reducing high AMD sulfate concentration and neutralizing its acidity. The sulfates are removed as H2S while the HM ions can be precipitated as insoluble metal sulfides by the biogenic H2S produced due to sulfate reduction by SRB. Sulfur-reducing bacteria can be classified into several bacterial phylotypes belonging to class Deltaproteobacteria, Clostridia, Nitrospira, and Thermosulfobacteria, as well as archaeal genera Archaeoglobus, Thermocladium, and Caldivirga (Xu and Chen, 2020). Currently, active and passive technologies utilizing sulfidogenic bioreactors involving SRB has become attractive for treatment and recovery of HM in acidic sulfate-containing wastewater such as AMD. The performance including the microbial communities of sulfidogenic reactors treating AMD comprising fermentative-, acetogenic-, and SRB as well as methanogenic archaea has been documented (Sánchez-Andrea et al., 2014; Aoyagi et al., 2018; Ayangbenro et al., 2018; Xu and Chen, 2020; Nogueira et al., 2021). In addition, innate activity of diverse FeOB ranging from members of genera Metallibacterium, Ferrovum, Leptothrix, Sideroxydans, Bacillus, Gallionella, Leptospirillum, Acidithiobacillus, and Ferritrophicum are important for robust oxidative precipitation of Fe from AMD, suggesting their biotechnological relevance in the treatment of AMD impacted environment (Brantner et al., 2014).
In agricultural soils, long-term AMD irrigation of paddy soil has been associated with increased abundance of SRB and bacterial lineages such as Desulfobacca, Desulfovibrio, Syntrophobacter, Desulforhopalus, Desulfarculus, and Desulfobulbus mainly correlating to soil pH, the organic matter and the sulfate than the HMs (Wang et al., 2016). Furthermore, the effects of root associated FeOB in increasing phosphate nutrition and influencing root to shoot partitioning of iron in tolerant plant Typha angustifolia under AMD conditions have been reported (Ghosh et al., 2014). Similarly, the application of As-resistant FeOB strains (Bacillus sp. T2, Pseudomonas sp. Yangling I4, and Bacillus sp. TF1-3) in decreasing As in brown rice growing in mine polluted soils through promotion of root Fe plaque formation that sequesters As and reduces As(III) influx has been reported (Xiao et al., 2020). These few examples illustrate the potential biotechnological application SRB and FeOB in improving agricultural productivity and bioremediation of agricultural soils.
Nutrient (N and C) Recycling
Heavy metal concentration in the soil significantly influences its chemical properties such pH, carbon (C), and nitrogen (N) content. Under the oligotrophic and metal-rich AMD environments, organic C, and bioavailable N content can be growth limiting, with AMD contamination documented to impact the both carbon and nitrogen cycle in several ways (Méndez-García et al., 2015). Metabolic processes related to carbon-fixation, nitrogen fixation, and denitrification by in situ microbiome are important. Acid mine drainage contamination influence on the distribution of both autrotrophic carbon-fixing phylotypes (such as Leptospirillum and Metallibacterium) and heterotrophs/facultative autotrophs (such as Bradyrhizobium, Rhizobium, and Burkholderia) dependent of TOC level (Wang et al., 2016; Sun et al., 2020). Whereas, Metallibacterium are chemolithoautotrophs participating in carbon fixation and sulfur oxidation, Leptospirrulum are iron-oxidizers capable of dissimilatory sulfate reduction. Nitrogen-fixing bacteria (Bradyrhizobium, Rhizobium, and Burkholderia) and Leptospirrulum performs the dual function of heterotrophic carbon-fixation and providing bioavailable N to the harsh environments and help sustain the function of the ecosystem. However, higher AMD contamination significantly decreases the number of soil nitrogen bacterial species and species richness, with a consequence of impairing both the nitrogen fixation and dissimilatory nitrate reduction (DNRA) activity, altered uptake, and utilization of nutrients (Wang et al., 2019a). This may explain the importance of organic and inorganic amendments to boost both N and C content to efficiently treat AMD in passive bioreactor-based technologies.
Conclusion
The objective of the present review was to provide a comprehensive assessment on the impact of AMD pollution on microbial diversity and functioning, highlighting the main implications and challenges of long-term AMD-irrigated agriculture on soil health, and sustainable agricultural productivity. Overall, HM toxicity and highly acidic conditions is associated with reduced microbial biomass, richness, and diversity, negatively impacting on soil microbial functions and processes such as litter decomposition, carbon mineralization, nitrogen transformations, and soil enzymatic activity, that may detrimental to soil health and plant productivity in agricultural soils. The dual drawbacks on food safety (potential biomagnification of toxic HM along food chain) and detrimental effect on agricultural productivity coupled with environmental degradation, imply that untreated AMD water is unsuitable for irrigated agriculture. Currently, there are numerous technologies for passive or active treatment of AMD wastewater and contaminated agricultural soils, differentiated by their cost effectiveness, and efficiency in HM removal. Interestingly, AMD impacted habitats are unique ecological niches for novel acidophilic, HM, and sulfate-adapted microbial phylotypes that might be beneficial to optimal plant growth and productivity and bioremediation of AMD-polluted agricultural soils. This review has highlighted the impact active and passive treatment technologies on AMD microbial diversity. The discussion is further extended to the interrelated microbial diversity and beneficial functions such as metal bioremediation, acidity neutralization, symbiotic rhizomicrobiome assembly and plant growth promotion, sulfates/iron reduction and biogeochemical N and C recycling under AMD-impacted environment, elucidating and discussing the significance of SRB, FeOB, and PGPRs passive and active systems dedicated to bioremediation and microbe-assisted phytoremediation. Despite bioremediation being attractive alternative for AMD management, current understanding on the link between microbial community dynamics, process stability, and functions in engineered active and passive systems for bioremediation is still limited. Furthermore, bioremediation is a constantly-evolving multidisciplinary endeavor, thus, future studies integrating meta-omics and process engineering on AMD impacted microbiomes will be important in the design and optimization of robust approaches to improve bioremediation processes.
Author Contributions
RM wrote the manuscript. HO and DM critically edited the manuscript. All authors contributed to the article and approved the submitted version.
Funding
HO acknowledges University of South Africa (UniSA) Research Support and Commercialization Directorate for the funding of Visiting Researcher Fellowship.
Conflict of Interest
The authors declare that the research was conducted in the absence of any commercial or financial relationships that could be construed as a potential conflict of interest.
Publisher's Note
All claims expressed in this article are solely those of the authors and do not necessarily represent those of their affiliated organizations, or those of the publisher, the editors and the reviewers. Any product that may be evaluated in this article, or claim that may be made by its manufacturer, is not guaranteed or endorsed by the publisher.
Acknowledgments
The authors wish to thank the University of South Africa for financial support.
References
Acharya, B. S., and Kharel, G. (2020). Acid mine drainage from coal mining in the United States–An overview. J. Hydrol. 588:125061. doi: 10.1016/j.jhydrol.2020.125061
Akcil, A., and Koldas, S. (2006). Acid Mine Drainage (AMD): causes, treatment and case studies. J. Clean. Prod. 14, 1139–1145. doi: 10.1016/j.jclepro.2004.09.006
Annandale, J. G., Jovanovic, N. Z., Hodgson, F. D. I., Usher, B. H., Aken, M. E., Van Der Westhuizen, A. M., et al. (2006). Prediction of the environmental impact and sustainability of large-scale irrigation with gypsiferous mine-water on groundwater resources. Water SA 32, 21–28. doi: 10.4314/wsa.v32i1.5235
Aoyagi, T., Hamai, T., Hori, T., Sato, Y., Kobayashi, M., Sato, Y., et al. (2018). Microbial community analysis of sulfate-reducing passive bioreactor for treating acid mine drainage under failure conditions after long-term continuous operation. J. Environ. Chem. Eng. 6, 5795–5800. doi: 10.1016/j.jece.2018.09.003
Ayangbenro, A. S., Olanrewaju, O. S., and Babalola, O. O. (2018). Sulfate-reducing bacteria as an effective tool for sustainable acid mine bioremediation. Front. Microbiol. 9:1986. doi: 10.3389/fmicb.2018.01986
Backer, R., Rokem, J. S., Ilangumaran, G., Lamont, J., Praslickova, D., Ricci, E., et al. (2018). Plant growth-promoting rhizobacteria: context, mechanisms of action, and roadmap to commercialization of biostimulants for sustainable agriculture. Front. Plant Sci. 9:1473. doi: 10.3389/fpls.2018.01473
Baker, B. J., and Banfield, J. F. (2003). Microbial communities in acid mine drainage. FEMS Microbiol. Ecol. 44, 139–152. doi: 10.1016/S0168-6496(03)00028-X
Baker, B. J., Lutz, M. A., Dawson, S. C., Bond, P. L., and Banfield, J. F. (2004). Metabolically active eukaryotic communities in extremely acidic mine drainage. Appl. Environ. Microbiol. 70, 6264–6271. doi: 10.1128/AEM.70.10.6264-6271.2004
Baker, B. J., Tyson, G. W., Goosherst, L., and Banfield, J. F. (2009). Insights into the diversity of eukaryotes in acid mine drainage biofilm communities. Appl. Environ. Microbiol. 75, 2192–2199. doi: 10.1128/AEM.02500-08
Bao, Y., Jin, X., Guo, C., Lu, G., and Dang, Z. (2021). Sulfate-reducing bacterial community shifts in response to acid mine drainage in the sediment of the Hengshi watershed, South China. Environ. Sci. Pollut. Res. 28, 2822–2834. doi: 10.1007/s11356-020-10248-7
Bautista-Hernández, D., Ramírez-Burgos, L., Duran-Páramo, E., and Fernández-Linares, L. (2012). Zinc and lead biosorption by delftia tsuruhatensis: a bacterial strain resistant to metals isolated from mine tailings. J. Water Resource Prot. 4, 207–216. doi: 10.4236/jwarp.2012.44023
Bernardez, L. A., de Oliveira, L. E. L., and de Andrade Lima, L. R. P. (2021). Acid mine drainage at the Bahia Gold Belt (Brazil): microbial isolation and characterization. Environ. Monit. Assess. 193, 60. doi: 10.1007/s10661-021-08844-2
Blowes, D., Ptacek, C., Jambor, J., and Weisener, C. (2005). The geochemistry of acid mine. Environ. Geochem 9, 149–204. doi: 10.1016/B0-08-043751-6/09137-4
Bomberg, M., Mäkinen, J., Salo, M., and Kinnunen, P. (2019). High diversity in iron cycling microbial communities in acidic, iron-rich water of the Pyhäsalmi mine, Finland. Geofluids 2019:7401304. doi: 10.1155/2019/7401304
Bonilla, J. O., Kurth, D. G., Cid, F. D., Ulacco, J. H., Gil, R. A., and Villegas, L. B. (2018). Prokaryotic and eukaryotic community structure affected by the presence of an acid mine drainage from an abandoned gold mine. Extremophiles 22, 699–711. doi: 10.1007/s00792-018-1030-y
Boularbah, A., Schwartz, C., Bitton, G., Aboudrar, W., Ouhammou, A., and Morel, J. L. (2006). Heavy metal contamination from mining sites in South Morocco: 2. Assessment of metal accumulation and toxicity in plants. Chemosphere 63, 811–817. doi: 10.1016/j.chemosphere.2005.07.076
Brantner, J. S., Haake, Z. J., Burwick, J. E., Menge, C. M., Hotchkiss, S. T., and Senko, J. M. (2014). Depth-dependent geochemical and microbiological gradients in Fe(III) deposits resulting from coal mine-derived acid mine drainage. Front. Microbiol. 5:215. doi: 10.3389/fmicb.2014.00215
Bwapwa, J. K., Jaiyeola, A. T., and Chetty, R. (2017). Bioremediation of acid mine drainage using algae strains: a review. South Afr. J. Chem. Eng. 24, 62–70. doi: 10.1016/j.sajce.2017.06.005
Cao, X., Zhou, S., Xie, F., Rong, R., and Wu, P. (2019). The distribution of rare earth elements and sources in Maoshitou reservoir affected by acid mine drainage, Southwest China. J. Geochem. Explor. 202, 92–99. doi: 10.1016/j.gexplo.2019.03.019
Carlier, J. D., Ettamimi, S., Cox, C. J., Hammani, K., Ghazal, H., and Costa, M. C. (2020). Prokaryotic diversity in stream sediments affected by acid mine drainage. Extremophiles 24, 809–819. doi: 10.1007/s00792-020-01196-8
Carlson, B. (2013). Water Chemistry and Benthic Macroinvertebrate Ecology in Response to Acid Mine Drainage. Graduate Theses, Dissertations, and Problem Reports. 7304. West Virginia University.
Chan, W. S., Routh, J., Luo, C., Dario, M., Miao, Y., Luo, D., et al. (2021). Metal accumulations in aquatic organisms and health risks in an acid mine-affected site in South China. Environ. Geochem. Health. doi: 10.1007/s10653-021-00923-0
Checcucci, A., Bazzicalupo, M., and Mengoni, A. (2017). “Exploiting nitrogen-fixing rhizobial symbionts genetic resources for improving phytoremediation of contaminated soils,” in Enhancing Cleanup of Environmental Pollutants, eds N. A. Anjum, S. S. Gill and N. Tuteja (Springer), 275–288. doi: 10.1007/978-3-319-55426-6_13
Chen, L., Hu, M., Huang, L., Hua, Z., Kuang, J., Li, S., et al. (2015). Comparative metagenomic and metatranscriptomic analyses of microbial communities in acid mine drainage. ISME J. 9, 1579–1592. doi: 10.1038/ismej.2014.245
Chen, L., Huang, L., Méndez-García, C., Kuang, J., Hua, Z., Liu, J., et al. (2016). Microbial communities, processes and functions in acid mine drainage ecosystems. Curr. Opin. Biotechnol. 38, 150–158. doi: 10.1016/j.copbio.2016.01.013
Choudhury, B. U., Malang, A., Webster, R., Mohapatra, K. P., Verma, B. C., Kumar, M., et al. (2017). Acid drainage from coal mining: effect on paddy soil and productivity of rice. Sci. Total Environ. 583, 344–351. doi: 10.1016/j.scitotenv.2017.01.074
Darko, G., Boakye, K. O., Nkansah, M. A., Gyamfi, O., Ansah, E., Yevugah, L. L., et al. (2019). Human health risk and bioaccessibility of toxic metals in topsoils from gbani mining community in Ghana. J Health Pollut. 9:190602. doi: 10.5696/2156-9614-9.22.190602
Ding, Z., and Sun, Q. (2021). Effects of flooding depth on metal(loid) absorption and physiological characteristics of Phragmites australis in acid mine drainage phytoremediation. Environ. Technol. Innov. 22:101512. doi: 10.1016/j.eti.2021.101512
Ding, Z., Wu, J., You, A., Huang, B., and Cao, C. (2017). Effects of heavy metals on soil microbial community structure and diversity in the rice (Oryza sativa L. subsp. Japonica, Food Crops Institute of Jiangsu Academy of Agricultural Sciences) rhizosphere. Soil Sci Plant Nutr. 63, 75–83. doi: 10.1080/00380768.2016.1247385
Distaso, M. A., Bargiela, R., Brailsford, F., Williams, G. B., Wright, S., Lunev, E. A., et al. (2020). High representation of archaea across all depths in oxic and low-pH sediment layers underlying an acidic stream. Front. Microbiol. 11:2871. doi: 10.3389/fmicb.2020.576520
Dutta, M., Islam, N., Rabha, S., Narzary, B., Bordoloi, M., Saikia, D., et al. (2020). Acid mine drainage in an Indian high-sulfur coal mining area: cytotoxicity assay and remediation study. J. Hazard. Mater. 389:121851. doi: 10.1016/j.jhazmat.2019.121851
Edwards, K. J., Bond, P. L., Gihring, T. M., and Banfield, J. F. (2000). An archaeal iron-oxidizing extreme acidophile important in acid mine drainage. Science 287, 1796–1799. doi: 10.1126/science.287.5459.1796
Emmanuel, A. Y., Jerry, C. S., and Dzigbodi, D. A. (2018). Review of environmental and health impacts of mining in Ghana. J Health Pollut 8, 43–52. doi: 10.5696/2156-9614-8.17.43
Falagán, C., Grail, B. M., and Johnson, D. B. (2017). New approaches for extracting and recovering metals from mine tailings. Miner. Eng. 106, 71–78. doi: 10.1016/j.mineng.2016.10.008
Galhardi, J. A., and Bonotto, D. M. (2016). Hydrogeochemical features of surface water and groundwater contaminated with acid mine drainage (AMD) in coal mining areas: a case study in southern Brazil. Environ. Sci. Pollut. Res. 23, 18911–18927. doi: 10.1007/s11356-016-7077-3
García-Moyano, A., Austnes, A. E., Lanzén, A., González-Toril, E., Aguilera, Á., and Øvreås, L. (2015). Novel and unexpected microbial diversity in acid mine drainage in Svalbard (78° N), revealed by culture-independent approaches. Microorganisms 3, 667–694. doi: 10.3390/microorganisms3040667
García-Moyano, A., González-Toril, E., Aguilera, A., and Amils, R. (2007). Prokaryotic community composition and ecology of floating macroscopic filaments from an extreme acidic environment, Río Tinto (SW, Spain). Syst. Appl. Microbiol. 30, 601–614. doi: 10.1016/j.syapm.2007.08.002
Garcia-Sánchez, M., Garcia-Romera, I., Cajthaml, T., Tlustoš, P., and Száková, J. (2015). Changes in soil microbial community functionality and structure in a metal-polluted site: the effect of digestate and fly ash applications. J. Environ. Manage. 162, 63–73. doi: 10.1016/j.jenvman.2015.07.042
Ghosh, U. D., Saha, C., Maiti, M., Lahiri, S., Ghosh, S., Seal, A., et al. (2014). Root associated iron oxidizing bacteria increase phosphate nutrition and influence root to shoot partitioning of iron in tolerant plant Typha angustifolia. Plant Soil 381, 279–295. doi: 10.1007/s11104-014-2085-x
Gitari, W. M. (2014). Attenuation of metal species in acidic solutions using bentonite clay: implications for acid mine drainage remediation. Toxicol. Environ. Sci. 96, 201–217. doi: 10.1080/02772248.2014.923426
Gomez Isaza, D. F., Cramp, R. L., and Franklin, C. E. (2020). Simultaneous exposure to nitrate and low pH reduces the blood oxygen-carrying capacity and functional performance of a freshwater fish. Conserv. Physiol. 8:coz092. doi: 10.1093/conphys/coz092
Grande, J., Santisteban, M., de la Torre, M., Dávila, J., and Pérez-Ostal,é, E. (2018). Map of impact by acid mine drainage in the river network of The Iberian Pyrite Belt (Sw Spain). Chemosphere 199, 269–277. doi: 10.1016/j.chemosphere.2018.02.047
Gupta, A., Dutta, A., Sarkar, J., Panigrahi, M. K., and Sar, P. (2018). Low-abundance members of the firmicutes facilitate bioremediation of soil impacted by highly acidic mine drainage from the Malanjkhand Copper Project, India. Front. Microbiol. 9:2882. doi: 10.3389/fmicb.2018.02882
Gupta, A., Saha, A., and Sar, P. (2021). Thermoplasmata and Nitrososphaeria as dominant archaeal members in acid mine drainage sediment of Malanjkhand Copper Project, India. Arch. Microbiol. 203, 1833–1841. doi: 10.1007/s00203-020-02130-4
Gupta, A., and Sar, P. (2019). Role of cost-effective organic carbon substrates in bioremediation of acid mine drainage–impacted soil of Malanjkhand Copper Project, India: a biostimulant for autochthonous microbial populations. Environ. Sci. Pollut. Res. Int. 27, 27407–27421. doi: 10.1007/s11356-019-06293-6
Gupta, A., and Sar, P. (2020). Characterization and application of an anaerobic, iron and sulfate reducing bacterial culture in enhanced bioremediation of acid mine drainage impacted soil. J. Environ. Sci. Health A 55, 464–482. doi: 10.1080/10934529.2019.1709362
Gwenzi, W. (2020). Occurrence, behaviour, and human exposure pathways and health risks of toxic geogenic contaminants in serpentinitic ultramafic geological environments (SUGEs): a medical geology perspective. Sci. Total Environ. 700:134622. doi: 10.1016/j.scitotenv.2019.134622
Hallberg, K. (2010). New perspectives in acid mine drainage microbiology. Hydrometallurgy 104, 448–453. doi: 10.1016/j.hydromet.2009.12.013
Hao, C., Wang, L., Gao, Y., Zhang, L., and Dong, H. (2010). Microbial diversity in acid mine drainage of Xiang Mountain sulfide mine, Anhui Province, China. Extremophiles 14, 465–474. doi: 10.1007/s00792-010-0324-5
He, Z., Xiao, S., Xie, X., Zhong, H., Hu, Y., Li, Q., et al. (2007). Molecular diversity of microbial community in acid mine drainages of Yunfu sulfide mine. Extremophiles 11, 305–314. doi: 10.1007/s00792-006-0044-z
Henao, S. G., and Ghneim-Herrera, T. (2021). Heavy metals in soils and the remediation potential of bacteria associated with the plant microbiome. Front. Environ. Sci. 9:15. doi: 10.3389/fenvs.2021.604216
Hou, D., O'Connor, D., Igalavithana, A. D., Alessi, D. S., Luo, J., Tsang, D. C., et al. (2020). Metal contamination and bioremediation of agricultural soils for food safety and sustainability. Nat. Rev. Earth Environ. 1, 366–381. doi: 10.1038/s43017-020-0061-y
Iakovleva, E., Mäkilä, E., Salonen, J., Sitarz, M., Wang, S., and Sillanpää, M. (2015). Acid mine drainage (AMD) treatment: neutralization and toxic elements removal with unmodified and modified limestone. Ecol. Eng. 81, 30–40. doi: 10.1016/j.ecoleng.2015.04.046
Islam, F., Yasmeen, T., Ali, Q., Mubin, M., Ali, S., Arif, M. S., et al. (2016). Copper-resistant bacteria reduces oxidative stress and uptake of copper in lentil plants: potential for bacterial bioremediation. Environ. Sci. Pollut. Res. 23, 220–233. doi: 10.1007/s11356-015-5354-1
Jacob, J. M., Karthik, C., Saratale, R. G., Kumar, S. S., Prabakar, D., Kadirvelu, K., et al. (2018). Biological approaches to tackle heavy metal pollution: a survey of literature. J. Environ. Manage. 217, 56–70. doi: 10.1016/j.jenvman.2018.03.077
Jennings, S. R., Blicker, P. S., and Neuman, D. R. (2008). Acid Mine Drainage and Effects on Fish Health and Ecology: A Review. Reclamation Research Group.
Jin, Y., Luan, Y., Ning, Y., and Wang, L. (2018). Effects and mechanisms of microbial remediation of heavy metals in soil: a critical review. Appl. Sci. 8:1336. doi: 10.3390/app8081336
Johnson, D. B., and Hallberg, K. B. (2005). Biogeochemistry of the compost bioreactor components of a composite acid mine drainage passive remediation system. Sci. Total Environ. 338, 81–93. doi: 10.1016/j.scitotenv.2004.09.008
Jovanovic, N., Barnard, R., Rethman, N., and Annandale, J. (1998). Crops can be irrigated with lime-treated acid mine drainage. Water Pretor. 24, 113–122.
Jung, S. P., Cheong, Y., Yim, G., Ji, S., and Kang, H. (2014). Performance and bacterial communities of successive alkalinity-producing systems (SAPSs) in passive treatment processes treating mine drainages differing in acidity and metal levels. Environ. Sci. Pollut. Res. 21, 3722–3732. doi: 10.1007/s11356-013-2366-6
Justice, N. B., Pan, C., Mueller, R., Spaulding, S. E., Shah, V., Sun, C. L., et al. (2012). Heterotrophic Archaea contribute to carbon cycling in low-pH, Suboxic biofilm communities. Appl. Environ. Microbiol. 78, 8321–8330. doi: 10.1128/AEM.01938-12
Kalu, C. M., Ogola, H. J. O., Selvarajan, R., Tekere, M., and Ntushelo, K. (2021). Fungal and metabolome diversity of the rhizosphere and endosphere of Phragmites australis in an AMD-polluted environment. Heliyon 7:e06399. doi: 10.1016/j.heliyon.2021.e06399
Kefeni, K. K., Msagati, T. A., and Mamba, B. B. (2017). Acid mine drainage: prevention, treatment options, and resource recovery: a review. J. Clean. Prod. 151, 475–493. doi: 10.1016/j.jclepro.2017.03.082
Keshri, J., Mankazana, B. B. J., and Momba, M. N. B. (2015). Profile of bacterial communities in South African mine-water samples using Illumina next-generation sequencing platform. Appl. Microbiol. Biotechnol. 99, 3233–3242. doi: 10.1007/s00253-014-6213-6
Kim, D., Ren, Y., Cui, M., Lee, Y., Kim, J., Kwon, O., et al. (2021). Arsenic adsorption on two types of powdered and beaded coal mine drainage sludge adsorbent. Chemosphere 272:129560. doi: 10.1016/j.chemosphere.2021.129560
Kimura, S., Bryan, C. G., Hallberg, K. B., and Johnson, D. B. (2011). Biodiversity and geochemistry of an extremely acidic, low-temperature subterranean environment sustained by chemolithotrophy. Environ. Microbiol. 13, 2092–2104. doi: 10.1111/j.1462-2920.2011.02434.x
Korzhenkov, A. A., Toshchakov, S. V., Bargiela, R., Gibbard, H., Ferrer, M., Teplyuk, A. V., et al. (2019). Archaea dominate the microbial community in an ecosystem with low-to-moderate temperature and extreme acidity. Microbiome 7:11. doi: 10.1186/s40168-019-0623-8
Kuang, J., Huang, L., He, Z., Chen, L., Hua, Z., Jia, P., et al. (2016). Predicting taxonomic and functional structure of microbial communities in acid mine drainage. ISME J. 10, 1527–1539. doi: 10.1038/ismej.2015.201
Kuang, J.-L., Huang, L.-N., Chen, L.-X., Hua, Z.-S., Li, S.-J., Hu, M., et al. (2013). Contemporary environmental variation determines microbial diversity patterns in acid mine drainage. ISME J. 7, 1038–1050. doi: 10.1038/ismej.2012.139
Kumar, A., MMS, C.-P., Chaturvedi, A. K., Shabnam, A. A., Subrahmanyam, G., Mondal, R., et al. (2020). Lead toxicity: health hazards, influence on food chain, and sustainable remediation approaches. Int. J. Environ. Res. Public Health 17:2179. doi: 10.3390/ijerph17072179
Laroche, E., Casiot, C., Fernandez-Rojo, L., Desoeuvre, A., Tardy, V., Bruneel, O., et al. (2018). Dynamics of bacterial communities mediating the treatment of an as-rich acid mine drainage in a field pilot. Front. Microbiol. 9:3169. doi: 10.3389/fmicb.2018.03169
Leclercq-Dransart, J., Demuynck, S., Bidar, G., Douay, F., Grumiaux, F., Louvel, B., et al. (2019). Does adding fly ash to metal-contaminated soils play a role in soil functionality regarding metal availability, litter quality, microbial activity and the community structure of Diptera larvae? Appl. Soil Ecol. 138, 99–111. doi: 10.1016/j.apsoil.2019.02.027
Lee, M.-K., Saunders, J. A., Wilson, T., Levitt, E., Saffari Ghandehari, S., Dhakal, P., et al. (2019). Field-scale bioremediation of arsenic-contaminated groundwater using sulfate-reducing bacteria and biogenic pyrite. Bioremediat. J. 23, 1–21. doi: 10.1080/10889868.2018.1516617
Lei, L.-Q., Song, C., Xie, X.-L., Li, Y., and Fei, W. (2010). Acid mine drainage and heavy metal contamination in groundwater of metal sulfide mine at arid territory (BS mine, Western Australia). Trans. Nonferr. Met. Soc. China 20, 1488–1493. doi: 10.1016/S1003-6326(09)60326-5
Li, K., and Ramakrishna, W. (2011). Effect of multiple metal resistant bacteria from contaminated lake sediments on metal accumulation and plant growth. J. Hazard. Mater. 189, 531–539. doi: 10.1016/j.jhazmat.2011.02.075
Li, Y., Yuan, L., Xue, S., Liu, B., and Jin, G. (2020). The recruitment of bacterial communities by the plant root system changed by acid mine drainage pollution in soils. FEMS Microbiol. Lett. 367:fnaa117. doi: 10.1093/femsle/fnaa117
Liang, J., Yu, X., Cao, Y., Zhang, J., Yan, N., Xue, L., et al. (2021). Effect of quicklime on microbial community in strong acidic soil. J. Soil Sci. Plant Nutr. doi: 10.1007/s42729-021-00478-0
Liao, J., Wen, Z., Ru, X., Chen, J., Wu, H., and Wei, C. (2016). Distribution and migration of heavy metals in soil and crops affected by acid mine drainage: public health implications in Guangdong Province, China. Ecotoxicol. Environ. Saf. 124, 460–469. doi: 10.1016/j.ecoenv.2015.11.023
Lin, C., Lu, W., and Wu, Y. (2005). Agricultural soils irrigated with acidic mine water: acidity, heavy metals, and crop contamination. Soil Res. 43, 819–826. doi: 10.1071/SR04148
Liu, S., Lu, Y., Yang, C., Liu, C., Ma, L., and Dang, Z. (2017). Effects of modified biochar on rhizosphere microecology of rice (Oryza sativa L.) grown in As-contaminated soil. Environ. Sci. Pollut. Res. 24, 23815–23824. doi: 10.1007/s11356-017-9994-1
Lu, S., Gischkat, S., Reiche, M., Akob, D. M., Hallberg, K. B., and Küsel, K. (2010). Ecophysiology of Fe-cycling bacteria in acidic sediments. Appl. Environ. Microbiol. 76, 8174–8183. doi: 10.1128/AEM.01931-10
Luís, A., Teixeira, P., Almeida, S., Ector, L., Matos, J., and Da Silva, E. F. (2009). Impact of acid mine drainage (AMD) on water quality, stream sediments and periphytic diatom communities in the surrounding streams of Aljustrel mining area (Portugal). Water Air Soil Pollut. 200, 147–167. doi: 10.1007/s11270-008-9900-z
Lukhele, T., Selvarajan, R., Nyoni, H., Mamba, B. B., and Msagati, T. A. (2020). Acid mine drainage as habitats for distinct microbiomes: current knowledge in the era of molecular and omic technologies. Curr. Microbiol. 77, 657–674. doi: 10.1007/s00284-019-01771-z
Lukhele, T., Selvarajan, R., Nyoni, H., Mamba, B. B., and Msagati, T. A. M. (2019). Diversity and functional profile of bacterial communities at Lancaster acid mine drainage dam, South Africa as revealed by 16S rRNA gene high-throughput sequencing analysis. Extremophiles 23, 719–734. doi: 10.1007/s00792-019-01130-7
Luo, C., Routh, J., Dario, M., Sarkar, S., Wei, L., Luo, D., et al. (2020a). Distribution and mobilization of heavy metals at an acid mine drainage affected region in South China, a post-remediation study. Sci. Total Environ. 724:138122. doi: 10.1016/j.scitotenv.2020.138122
Luo, Z.-H., Li, Q., Lai, Y., Chen, H., Liao, B., and Huang, L. (2020b). Diversity and genomic characterization of a novel parvarchaeota family in acid mine drainage sediments. Front. Microbiol. 11:3313. doi: 10.3389/fmicb.2020.612257
Ly, T., Wright, J. R., Weit, N., McLimans, C. J., Ulrich, N., Tokarev, V., et al. (2019). Microbial communities associated with passive acidic abandoned coal mine remediation. Front. Microbiol. 10:1955. doi: 10.3389/fmicb.2019.01955
Madiseng, L. (2018). Crop Response to Irrigation With Acidic and Neutralized, Saline Mine Water. MSc Dissertation, University of Pretoria.
Massányi, P., Massányi, M., Madeddu, R., Stawarz, R., and Lukáč, N. (2020). Effects of cadmium, lead, and mercury on the structure and function of reproductive organs. Toxics 8:94. doi: 10.3390/toxics8040094
Medfu Tarekegn, M., Zewdu Salilih, F., and Ishetu, A. I. (2020). Microbes used as a tool for bioremediation of heavy metal from the environment. Cogent Food Agric. 6:1783174. doi: 10.1080/23311932.2020.1783174
Méndez-García, C., Peláez, A. I., Mesa, V., Sánchez, J., Golyshina, O. V., and Ferrer, M. (2015). Microbial diversity and metabolic networks in acid mine drainage habitats. Front. Microbiol. 6:475. doi: 10.3389/fmicb.2015.00475
Mesa, V., Gallego, J. L., González-Gil, R., Lauga, B., Sánchez, J., Méndez-García, C., et al. (2017). Bacterial, archaeal, and eukaryotic diversity across distinct microhabitats in an acid mine drainage. Front. Microbiol. 8:1756. doi: 10.3389/fmicb.2017.01756
Mishra, J., Singh, R., and Arora, N. K. (2017). Alleviation of heavy metal stress in plants and remediation of soil by rhizosphere microorganisms. Front. Microbiol. 8:1706. doi: 10.3389/fmicb.2017.01706
Mohanty, A. K., Lingaswamy, M., Rao, V. G., and Sankaran, S. (2018). Impact of acid mine drainage and hydrogeochemical studies in a part of Rajrappa coal mining area of Ramgarh District, Jharkhand State of India. Groundwater Sustain. Dev. 7, 164–175. doi: 10.1016/j.gsd.2018.05.005
Müller, A. L., Kjeldsen, K. U., Rattei, T., Pester, M., and Loy, A. (2015). Phylogenetic and environmental diversity of DsrAB-type dissimilatory (bi)sulfite reductases. ISME J. 9, 1152–1165. doi: 10.1038/ismej.2014.208
Musvoto, C., and de Lange, W. (2019). A framework for selecting crops for irrigation using mining contaminated water: an example from the Olifants basin of South Africa. J. Environ. Manage. 231, 49–58. doi: 10.1016/j.jenvman.2018.09.058
Narendrula-Kotha, R., and Nkongolo, K. K. (2017). Microbial response to soil liming of damaged ecosystems revealed by pyrosequencing and phospholipid fatty acid analyses. PLoS ONE 12:e0168497. doi: 10.1371/journal.pone.0168497
Narendrula-Kotha, R., Theriault, G., Mehes-Smith, M., Kalubi, K., and Nkongolo, K. (2019). Metal toxicity and resistance in plants and microorganisms in terrestrial ecosystems. Rev. Environ. Contamin. Toxicol. 249, 1–27. doi: 10.1007/398_2018_22
Netto, E., Madeira, R., Silveira, F., Fiori, M., Angioleto, E., Pich, C., et al. (2013). Evaluation of the toxic and genotoxic potential of acid mine drainage using physicochemical parameters and bioassays. Environ. Toxicol. Pharmacol. 35, 511–516. doi: 10.1016/j.etap.2013.02.007
Nicomrat, D., Dick, W. A., and Tuovinen, O. H. (2006). Microbial populations identified by fluorescence in situ hybridization in a constructed wetland treating acid coal mine drainage. J. Environ. Qual. 35, 1329–1337. doi: 10.2134/jeq2005.0325
Nkongolo, K., Narendrula-Kotha, R., Kalubi, K., Rainville, S., and Michael, P. (2017). High level of nickel tolerance and metal exclusion identified in silver maple (Acer saccharinum). Chem. Ecol. 33, 795–806. doi: 10.1080/02757540.2017.1376664
Nogueira, E. W., Gouvêa de Godoi, L. A., Marques Yabuki, L. N., Brucha, G., and Zamariolli Damianovic, M. H. R. (2021). Sulfate and metal removal from acid mine drainage using sugarcane vinasse as electron donor: performance and microbial community of the down-flow structured-bed bioreactor. Bioresour. Technol. 330:124968. doi: 10.1016/j.biortech.2021.124968
Nukpezah, D., Rahman, F. A., and Koranteng, S. (2017). The impact of small scale mining on irrigation water quality in Asante Akim Central Municipality of Ghana. West Afr. J. Appl. Ecol. 25, 49–67.
Nural Yaman, B., Aytar Çelik, P., Mutlu, M. B., and Çabuk, A. (2020). A combinational analysis of acidophilic bacterial diversity of an iron-rich environment. Geomicrobiol J. 37, 877–889. doi: 10.1080/01490451.2020.1795320
Obiri-Nyarko, F., Grajales-Mesa, S. J., and Malina, G. (2014). An overview of permeable reactive barriers for in situ sustainable groundwater remediation. Chemosphere 111, 243–259. doi: 10.1016/j.chemosphere.2014.03.112
Ochieng, G. M., Seanego, E. S., and Nkwonta, O. I. (2010). Impacts of mining on water resources in South Africa: a review. Scient. Res. Essays 5, 3351–3357.
Othman, A., Sulaiman, A., and Sulaiman, S. K. (2017). The use of hydrated lime in acid mine drainage treatment. AIP Conf. Proc. 1847:040001. doi: 10.1063/1.4983897
Panda, S., Mishra, S., and Akcil, A. (2016). Bioremediation of acidic mine effluents and the role of sulfidogenic biosystems: a mini-review. Euro Mediterr. J. Environ. Integr. 1:8. doi: 10.1007/s41207-016-0008-3
Pang, Z., Tayyab, M., Kong, C., Hu, C., Zhu, Z., Wei, X., et al. (2019). Liming positively modulates microbial community composition and function of sugarcane fields. Agronomy 9:808. doi: 10.3390/agronomy9120808
Park, I., Tabelin, C. B., Jeon, S., Li, X., Seno, K., Ito, M., et al. (2019). A review of recent strategies for acid mine drainage prevention and mine tailings recycling. Chemosphere 219, 588–606. doi: 10.1016/j.chemosphere.2018.11.053
Prasanna, R., Ratha, S. K., Rojas, C., and Bruns, M. A. (2011). Algal diversity in flowing waters at an acidic mine drainage “barrens” in central Pennsylvania, USA. Folia Microbiol. 56, 491–496. doi: 10.1007/s12223-011-0073-6
Rambabu, K., Banat, F., Pham, Q. M., Ho, S.-H., Ren, N.-Q., and Show, P. L. (2020). Biological remediation of acid mine drainage: Review of past trends and current outlook. Environ. Sci. Ecotechnol. 2:100024. doi: 10.1016/j.ese.2020.100024
Rathi, M., and Yogalakshmi, K. N. (2021). Brevundimonas diminuta MYS6 associated Helianthus annuus L. for enhanced copper phytoremediation. Chemosphere 263:128195. doi: 10.1016/j.chemosphere.2020.128195
Rezaie, B., and Anderson, A. (2020). Sustainable resolutions for environmental threat of the acid mine drainage. Sci. Total Environ. 717:137211. doi: 10.1016/j.scitotenv.2020.137211
Rodríguez-Galán, M., Baena-Moreno, F. M., Vázquez, S., Arroyo-Torralvo, F., Vilches, L. F., and Zhang, Z. (2019). Remediation of acid mine drainage. Environ. Chem. Lett. 17, 1529–1538. doi: 10.1007/s10311-019-00894-w
Romero, A., González, I., and Galán, E. (2011). Stream water geochemistry from mine wastes in Peña de Hierro, Riotinto area, SW Spain: a case of extreme acid mine drainage. Environ. Earth Sci. 62, 645–656. doi: 10.1007/s12665-010-0554-y
Roth, H., Gallo, S., Badger, P., and Hillwig, M. (2019). Changes in microbial communities of a passive coal mine drainage bioremediation system. Can. J. Microbiol. 65, 775–782. doi: 10.1139/cjm-2018-0612
RoyChowdhury, A., Sarkar, D., and Datta, R. (2015). Remediation of acid mine drainage-impacted water. Curr. Pollut. Rep. 1, 131–141. doi: 10.1007/s40726-015-0011-3
Sahoo, P., Tripathy, S., Panigrahi, M., and Equeenuddin, S. M. (2017). Anthropogenic contamination and risk assessment of heavy metals in stream sediments influenced by acid mine drainage from a northeast coalfield, India. Bull. Eng. Geol. Environ. 76, 537–552. doi: 10.1007/s10064-016-0975-2
Sánchez-Andrea, I., Sanz, J. L., Bijmans, M. F. M., and Stams, A. J. M. (2014). Sulfate reduction at low pH to remediate acid mine drainage. J. Hazard. Mater. 269, 98–109. doi: 10.1016/j.jhazmat.2013.12.032
Santofimia, E., González-Toril, E., López-Pamo, E., Gomariz, M., Amils, R., and Aguilera, Á. (2013). Microbial diversity and its relationship to physicochemical characteristics of the water in two extreme acidic pit lakes from the Iberian Pyrite Belt (SW Spain). PLoS ONE 8:e66746. doi: 10.1371/journal.pone.0066746
Selbmann, L., De Hoog, G., Zucconi, L., Isola, D., Ruisi, S., van den Ende, A. G., et al. (2008). Drought meets acid: three new genera in a dothidealean clade of extremotolerant fungi. Stud. Mycol. 61, 1–20. doi: 10.3114/sim.2008.61.01
Sibanda, T., Selvarajan, R., Msagati, T., Venkatachalam, S., and Meddows-Taylor, S. (2019). Defunct gold mine tailings are natural reservoir for unique bacterial communities revealed by high-throughput sequencing analysis. Sci. Total Environ. 650, 2199–2209. doi: 10.1016/j.scitotenv.2018.09.380
Sibanda, T., Selvarajan, R., Ogola, H. J., Obieze, C. C., and Tekere, M. (2021). Distribution and comparison of bacterial communities in HVAC systems of two university buildings: Implications for indoor air quality and public health. Environ. Monit. Assess. 193, 1–15.
Simate, G. S. (2021). “Environmental and health effects of acid mine drainage,” in Acid Mine Drainage (Boca Raton, FL: CRC Press) 97–114. doi: 10.1201/9780429401985-6
Song, C., Sarpong, C. K., He, J., Shen, F., Zhang, J., Yang, G., et al. (2020). Accelerating phytoremediation of degraded agricultural soils utilizing rhizobacteria and endophytes: a review. Environ. Rev. 28, 115–127. doi: 10.1139/er-2019-0020
Sracek, O., Choquette, M., Gélinas, P., Lefebvre, R., and Nicholson, R. V. (2004). Geochemical characterization of acid mine drainage from a waste rock pile, Mine Doyon, Quebec, Canada. J. Contam. Hydrol. 69, 45–71. doi: 10.1016/S0169-7722(03)00150-5
Steyn, M., Oberholster, P., Botha, A., Genthe, B., Van den Heever-Kriek, P., and Weyers, C. (2019). Treated acid mine drainage and stream recovery: downstream impacts on benthic macroinvertebrate communities in relation to multispecies toxicity bioassays. J. Environ. Manage. 235, 377–388. doi: 10.1016/j.jenvman.2019.01.051
Sun, W., Sun, X., Li, B., Xu, R., Young, L. Y., Dong, Y., et al. (2020). Bacterial response to sharp geochemical gradients caused by acid mine drainage intrusion in a terrace: relevance of C, N, and S cycling and metal resistance. Environ. Int. 138:105601. doi: 10.1016/j.envint.2020.105601
Tolonen, E.-T., Sarpola, A., Hu, T., Rämö, J., and Lassi, U. (2014). Acid mine drainage treatment using by-products from quicklime manufacturing as neutralization chemicals. Chemosphere 117, 419–424. doi: 10.1016/j.chemosphere.2014.07.090
Van den Berg, M. F., Botes, M., Slabbert, E., and Cloete, T. E. (2016). Evaluating sulphate removal and identifying the bacterial community present in acid mine drainage treated with synthetic domestic wastewater sludge. Water SA 42, 475–482. doi: 10.4314/wsa.v42i3.13
Vasquez, Y., Escobar, M. C., Neculita, C. M., Arbeli, Z., and Roldan, F. (2016). Biochemical passive reactors for treatment of acid mine drainage: effect of hydraulic retention time on changes in efficiency, composition of reactive mixture, and microbial activity. Chemosphere 153, 244–253. doi: 10.1016/j.chemosphere.2016.03.052
Vasquez, Y., Escobar, M. C., Saenz, J. S., Quiceno-Vallejo, M. F., Neculita, C. M., Arbeli, Z., et al. (2018). Effect of hydraulic retention time on microbial community in biochemical passive reactors during treatment of acid mine drainage. Bioresour. Technol. 247, 624–632. doi: 10.1016/j.biortech.2017.09.144
Vázquez-Campos, X., Kinsela, A. S., Waite, T. D., Collins, R. N., and Neilan, B. A. (2014). Fodinomyces uranophilus gen. nov. sp. nov. and Coniochaeta fodinicola sp. nov., two uranium mine-inhabiting Ascomycota fungi from northern Australia. Mycologia 106, 1073–1089. doi: 10.3852/14-013
Vicente-Beckett, V. A., McCauley, G. J. T., and Duivenvoorden, L. J. (2016). Metals in agricultural produce associated with acid-mine drainage in Mount Morgan (Queensland, Australia). J. Environ. Sci. Health A 51, 561–570. doi: 10.1080/10934529.2016.1141622
Villegas-Plazas, M., Sanabria, J., and Junca, H. (2019). A composite taxonomical and functional framework of microbiomes under acid mine drainage bioremediation systems. J. Environ. Manage. 251:109581. doi: 10.1016/j.jenvman.2019.109581
Wang, C., Wu, B., Jiang, K., Wei, M., and Wang, S. (2019a). Effects of different concentrations and types of Cu and Pb on soil N-fixing bacterial communities in the wheat rhizosphere. Appl. Soil Ecol. 144, 51–59. doi: 10.1016/j.apsoil.2019.07.008
Wang, H., Guo, C. L., Yang, C. F., Lu, G. N., Chen, M. Q., and Dang, Z. (2016). Distribution and diversity of bacterial communities and sulphate-reducing bacteria in a paddy soil irrigated with acid mine drainage. J. Appl. Microbiol. 121, 196–206. doi: 10.1111/jam.13143
Wang, H., Zeng, Y., Guo, C., Bao, Y., Lu, G., Reinfelder, J. R., et al. (2018). Bacterial, archaeal, and fungal community responses to acid mine drainage-laden pollution in a rice paddy soil ecosystem. Sci. Total Environ. 616–617:107–116. doi: 10.1016/j.scitotenv.2017.10.224
Wang, H., Zeng, Y., Guo, C., Zheng, X., Ding, C., Lu, G., et al. (2021a). Soil rehabilitation shaped different patterns of bacterial and archaeal community in AMD-irrigated paddy soil. Chemosphere 263:128259. doi: 10.1016/j.chemosphere.2020.128259
Wang, R., Lin, J.-Q., Liu, X.-M., Pang, X., Zhang, C.-J., Yang, C.-L., et al. (2019b). Sulfur oxidation in the acidophilic autotrophic Acidithiobacillus spp. Front. Microbiol. 9:3290. doi: 10.3389/fmicb.2018.03290
Wang, Z., Xu, Y., Zhang, Z., and Zhang, Y. (2021b). Acid Mine Drainage (AMD) in abandoned coal mines of Shanxi, China. Water 13:8. doi: 10.3390/w13010008
Watten, B. J., Sibrell, P. L., and Schwartz, M. F. (2005). Acid neutralization within limestone sand reactors receiving coal mine drainage. Environ. Pollut. 137, 295–304. doi: 10.1016/j.envpol.2005.01.026
Wu, P., Tang, C., Liu, C., Zhu, L., Pei, T., and Feng, L. (2009). Geochemical distribution and removal of As, Fe, Mn and Al in a surface water system affected by acid mine drainage at a coalfield in Southwestern China. Environ. Geol. 57, 1457–1467. doi: 10.1007/s00254-008-1423-9
Wu, Z., Kong, Z., Lu, S., Huang, C., Huang, S., He, Y., et al. (2019). Isolation, characterization and the effect of indigenous heavy metal-resistant plant growth-promoting bacteria on sorghum grown in acid mine drainage polluted soils. J. Gen. Appl. Microbiol. 65, 254–264. doi: 10.2323/jgam.2018.11.004
Xiao, A., Li, W. C., and Ye, Z. (2020). Effects of Fe-oxidizing bacteria (FeOB) on iron plaque formation, As concentrations and speciation in rice (Oryza sativa L.). Ecotoxicol. Environ. Saf. 190:110136. doi: 10.1016/j.ecoenv.2019.110136
Xin, R., Banda, J. F., Hao, C., Dong, H., Pei, L., Guo, D., et al. (2021). Contrasting seasonal variations of geochemistry and microbial community in two adjacent acid mine drainage lakes in Anhui Province, China. Environ. Pollut. 268:115826. doi: 10.1016/j.envpol.2020.115826
Xu, Y.-N., and Chen, Y. (2020). Advances in heavy metal removal by sulfate-reducing bacteria. Water Sci. Technol. 81, 1797–1827. doi: 10.2166/wst.2020.227
Yang, M., Xu, Y., Ke, H., and Chen, H. (2021). Cumulative effect and content variation of toxic trace elements in human hair around Xiaoqinling gold mining area, Northwestern China. Int. J. Environ. Res. Public Health 18:2074. doi: 10.3390/ijerph18042074
Keywords: acid mine drainage, heavy metal bioremediation, active and passive technologies, plant growth promoting rhizobacteria, sulfate-reducing bacteria, agricultural sustainability
Citation: Munyai R, Ogola HJO and Modise DM (2021) Microbial Community Diversity Dynamics in Acid Mine Drainage and Acid Mine Drainage-Polluted Soils: Implication on Mining Water Irrigation Agricultural Sustainability. Front. Sustain. Food Syst. 5:701870. doi: 10.3389/fsufs.2021.701870
Received: 28 April 2021; Accepted: 13 August 2021;
Published: 21 September 2021.
Edited by:
José Martinez, Institut National de Recherche pour l'Agriculture, l'Alimentation et l'Environnement (INRAE), FranceReviewed by:
Francisco Jesus Fernandez Morales, University of Castilla-La Mancha, SpainMingJiang Zhang, Independent Researcher, Beijing, China
Copyright © 2021 Munyai, Ogola and Modise. This is an open-access article distributed under the terms of the Creative Commons Attribution License (CC BY). The use, distribution or reproduction in other forums is permitted, provided the original author(s) and the copyright owner(s) are credited and that the original publication in this journal is cited, in accordance with accepted academic practice. No use, distribution or reproduction is permitted which does not comply with these terms.
*Correspondence: Rabelani Munyai, bXVueWFyJiN4MDAwNDA7dW5pc2EuYWMuemE=