- 1Department of Microbiology, University of Dhaka, Dhaka, Bangladesh
- 2Institute of National Analytical Research and Services (INARS), Bangladesh Council of Scientific and Industrial Research (BCSIR), Dhaka, Bangladesh
- 3Faculty of Dentistry, University of Malaya, Kuala Lumpur, Malaysia
The application of plant growth-promoting rhizobacteria (PGPR) as vital components for plant growth promotion against biotic and abiotic stresses could be a promising strategy to improve crop production in areas vulnerable to increasing salinity. Here, we isolated Seventy-five endophytic bacteria from roots of healthy Oryza sativa grown in a saline environment of the southern coastal region of Bangladesh. The endophytes in a culture of ~108 CFU/ml showed arrays of plant growth-promoting (PGP) activities: phytohormone (Indole acetic acid) production (1.20–60.13 μg/ ml), nutrient (phosphate) solubilization (0.02–1.81 μg/ml) and nitrogen fixation (70.24–198.70 μg/ml). Four genomically diverse groups were identified namely, Enterobacter, Achromobacter, Bacillus, and Stenotrophomonas using amplified ribosomal DNA restriction analysis followed by their respective 16S rDNA sequence analyses with that of the data available in NCBI GenBank. These four specific isolates showed tolerance to NaCl ranging from 1.37 to 2.57 mol/L in the nutrient agar medium. Under a 200 mmol/L salt stress in vitro, the bacteria in a culture of 108 CFU/ml exhibited competitive exopolysaccharide (EPS) production: Stenotrophomonas (65 μg/ml) and Bacillus (28 μg/ml), when compared to the positive control, Pseudomonas spp. (23.65 μg/ml), a phenomenon ably supported by their strong biofilm-producing abilities both in a microtiter plate assay, and in soil condition; and demonstrated by images of the scanning electron microscope (SEM). Overall, the isolated endophytic microorganisms revealed potential PGP activities that could be supported by their biofilm-forming ability under salinity stress, thereby building up a sustainable solution for ensuring food security in coastal agriculture under changing climate conditions.
Introduction
Soil salinity, one of the most vicious abiotic stresses of global magnitude is predominantly generated as a result of sea-level rise owing to climate change, and accountable for the loss of agricultural production and sustainability (Shahid et al., 2018). Constant salt deposition from intruding saltwater in agricultural fields interferes with soil physicochemical properties that led to permanent depletion in plant water absorption followed by dehydration and osmotic stress in plants (Kaushal and Wani, 2016). The accumulation of ions (Na+ and Cl−) beyond threshold limits badly affects plant metabolic machinery, transpiration system, photosynthesis, and most importantly, ionic balance and nutrient (N, Ca, K, P, Fe, Zn) uptake; thereby retarding overall growth, seed germination, reproductive development, and yield of crops (Gupta and Huang, 2014; Islam et al., 2015; Hashem et al., 2016; Egamberdieva et al., 2019; Albdaiwi et al., 2020). Scientific communities across the world are earnestly involved in finding novel solutions of plant stress tolerance strategies without compromising proper growth to fulfill food demands under limited resource availability. One such environment-friendly scheme includes the strengthening of plants' defense systems by employing beneficial microbes (Glick, 2014; Kasim et al., 2016; Sharma et al., 2016).
The plant kingdom is inhabited by a diverse group of endophytic bacteria which grow symptomless within plants as an integral part of host metabolism and function, bearing commensalism or mutualism relationship with plants (Haidar et al., 2018; Vinayarani and Prakash, 2018). These endophytic bacteria employ different mechanisms to stimulate plant growth, ensure nutrient availability of mineral assets such as phosphorus, iron; produce phytohormones, various secondary metabolites, volatile compounds, siderophores, lytic enzymes, and antibiotics to counter phytopathogens; and enhance stress tolerance in plants (Boddey and Dobereiner, 1988; Hallmann et al., 1997; Lazarovits and Nowak, 1997; Wakelin et al., 2004; Waqas et al., 2012). Some endophytes are well-reported to produce exopolysaccharide (EPS) outside of cell surfaces under inhospitable conditions which secure water holding capacity along with nutrient uptake around the plant roots, mitigating the effects of salinity and desiccation under salinity stress (Balsanelli et al., 2014; Ilangumaran and Smith, 2017; Ansari and Ahmad, 2018; Etesami and Beattie, 2018).
In the present study, an attempt was made to isolate endophytic plant growth-promoting rhizobacteria (PGPR) associated with Oryza sativa grown in saline soils in the southern coastal region of Bangladesh. The isolates were tested in vitro for their plant growth-promoting (PGP) abilities and exopolysaccharide production under different levels of salinity. In line with coastal agricultural development prioritizing the crop cultivation, this study can be considered as a climate-smart solution that harnessed an eco-friendly sustainable approach to enhance crop productivity as well as soil fertility with an insight of possible stress tolerance mechanism of endophytic PGPR providing to plants (Rima et al., 2018; Egamberdieva et al., 2019).
Materials and Methods
Sample Collection
During May 2017, roots of Oryza sativa samples were collected from six cultivated fields of three sub-districts of Patuakhali district namely Dumky (22°26′N, 90°22′E), Bauphal (22°25.8′N, 90°30.8′E), and Kalapara (21°59.2′N, 90°14.5′E) (Figure 1). Earlier, electrical conductivity (EC) of the soil samples from these regions were recorded as 7.88 ± 0.53 dS/m (Sultana et al., 2020), hence, the regions were considered saline since yields of many crops are restricted beyond the salinity threshold limit, 4.1–8.0 dS/m (Munns, 2005; Soil Resource Development Institute., 2010). Root samples were carried in a portable ice-box and transported back to the Fermentation and Enzyme Biotechnology laboratory, Department of Microbiology, University of Dhaka, Bangladesh and were stored at −20°C until further processing.
Isolation of Endophytic Bacteria
The roots of the collected samples were washed under running tap water for 5 min to remove adhering soil particles followed by washing serially in 75% ethanol, 99% ethanol, and finally, 10% H2O2 (5 min in each) to remove any kind of adhering microbes or epiphytes that were present on the root surfaces. Thereafter, surface-sterilized root tissues, resuspended at 10% in physiological saline (0.85% NaCl) were macerated with a sterilized mortar and pestle. The homogenized root samples were then used to screen endophytic PGPR through the spread plate technique. The processed root samples were not diluted to ensure not to lose a single root endophytic bacterium. Samples were spread plated onto two nitrogen-free media namely Jensen's agar media (10 g sucrose, 10 g glucose, 0.20 g MgSO4.7H2O, 0.15 g K2HPO4, 0.10 g CaSO4.2H2O, 0.05 g KH2PO4, 0.02 g CaCl2, 2 mg Na2MoO4, 1 mg FeCl3, 1 mg Na2Mo.2H2O, 15 g agar, pH 7.2 ± 0.2, in a 1 lit volume at 25°C) and Ashby's agar media (5 g sucrose, 5 g CaCO3, 0.2 g MgSO4.2H2O, 0.2 g NaCl, 0.2 g KH2PO4, 5 g benzoate, 10 mg FeSO4, 15 g agar, pH 7.2 ± 0.2, in a 1 lit volume at 25°C) working in the Class II Biosafety cabinet (ESCO, Singapore). Plates were incubated for 5–7 days at 30°C incubator (ESCO, Singapore) until the colonies were detected. Colonies with distinct morphological characteristics were selected and purified by sub-culturing two times on respective media before they were stored in a 20% glycerol solution at −80°C. Since the bacteria were collected exclusively from the root origin, the letter “R” was used as the source of collection, followed by either “J” or “A” representing “Jensen” and “Ashby” agar, respectively, while naming the isolates. For example, R2J6 would represent a bacterium recovered after homogenization of the second root sample, and isolated as the sixth isolate after growth in Jensen agar.
In vitro Assessment of Plant Growth Promoting (PGP) Traits
Indole Acetic Acid Production Assay
The amount of IAA produced by the isolated endophytes was determined by following the method described by Gordan and Weber (1950). Initially, the bacterial isolates were cultured in nutrient broth (NB) and 100 μl of bacterial inoculum (~108 CFU/ml) from each culture was taken afterward to continue the assay as described by Sultana et al. (2020).
Phosphate Solubilization Assay
Qualitative Assay
The inorganic phosphate solubilization ability of endophytes was observed by spot inoculation of bacterial isolates on Pikovskaya agar and National Botanical Research Institute's Phosphate (NBRIP) growth medium (Pikovskaya, 1948; Nautiyal, 1999). The formation of transparent halo zones around the bacterial colonies after 5–7 days of incubation at 30°C was considered as an indication of phosphate solubilizing activity.
Quantitative Assay
Phosphate solubilization by 100 μl of endophytic bacteria (~108 CFU/ml) was quantitated by the Molybdenum blue method in NBRIP broth, as described earlier (Sultana et al., 2020).
Nitrogen Fixation Assay
To determine the nitrogen-fixing capability of isolates, 100 μl of bacterial inoculum (~108 CFU/ml) for each selected isolates was inoculated into a test tube containing 5 ml nitrogen-free Jensen's broth and incubated for 5 days at 30°C at 120 r/min on a shaker. The amount of fixed atmospheric nitrogen was determined by the Kjeldahl method (Kjeldahl, 1883).
Genetic Diversity of Endophytic PGPR
Extraction of Total Genomic DNA
Genomic DNA of the endophytes was isolated by boiling method (Queipo-Ortuño et al., 2008). Isolated colonies were grown overnight in 5 ml nutrient broth in an orbital incubator (NEW BRUNSWICK™ 94 EXCELLA® E25/E25R, Germany) at 30°C. After 16 h incubation, 1 ml culture broth from each respective culture was transferred into an eppendorf tube and centrifuged at 15,000 × g for 5 min. The supernatant was eliminated and the pellet was resuspended in 200 μl nuclease-free water, subjected to boiling at 100°C in a water bath for 10 min, cooled on ice, and centrifuged at 15,000 × g for 10 min. The supernatant was taken into another fresh eppendorf tube and stored at −20°C. The DNA concentration was measured using a NanoDrop™ 8000 spectrophotometer (THERMO SCIENTIFIC, CALIFORNIA, USA).
ARDRA Analysis of 16S rDNA
In order to phylogenetically characterize the bacterial isolates prior to sequencing, Amplified Ribosomal DNA Restriction Analysis (ARDRA) was used to segregate them into clusters using 16S rDNA from the respective bacterial genome as a template. For the amplification of the 16S rDNA region, PCR was performed by 16S rDNA bacterial universal primers: forward (5′-AGAGTTTGATCMTGGCTCAG-3′) and reverse (5′- GGTTACCTTGTTACGACTT-3′) (Stevens and Van Elsas, 2010). PCR was carried out by using a thermal cycler (MODEL AERIS™, 96 WELLS, ESCO MICRO PTE. LTD., SINGAPORE) with the following amplification conditions: 94°C for 5 min for initial DNA denaturation, 35 cycles at 94°C for 1.5 min (denaturation), 56°C for 1 min (annealing) and 72°C for 1.5 min (extension), and a final elongation step at 72°C for 10 min. The amplified products were analyzed by gel electrophoresis in a 1.5% agarose gel (SIGMA, USA) with a 100 bp DNA ladder (PROMEGA, USA) and visualized by using a gel documentation system (ALPHALMAGER, USA).
In order to find the ARDRA patterns, the purified 16S rDNAs from bacterial isolates were subjected to restriction digestion with BsuRI (HaeIII) (THERMO SCIENTIFIC) restriction enzyme following the manufacturer's instructions. Ten units of restriction enzyme were added to 10 μl of the amplified DNA and incubated for 4 h at 37°C in a total volume of 30 μl. The digests were resolved by gel electrophoresis on a 1.5% (w/v) agarose gel (SIGMA, USA) and compared to a 100 bp DNA ladder (PROMEGA, USA) for size estimation. Using data from a freely available open-source website (http://insilico.ehu.es), a dendrogram was constructed by evaluating the restriction pattern of 16S rDNAs using Dice and UPGMA (Unweighted Pair Group Method with Arithmetic mean) analysis. The data were analyzed as discrete binary variables by “1” to represent the presence of bands and “0” for the absence.
16S rDNA Sequencing and Phylogenetic Analysis
16S rDNA PCR products were purified by FavorPrep™ GEL/PCR Purification Kit (FAVORGEN, TAIWAN) following the manufacturer's instructions. Based on PGP activities (in vitro) along with ARDRA analysis, four bacterial isolates were sent for automated DNA sequencing (1st Base, Malaysia). The sequences were further analyzed by using BLAST tools on the National Center of Biotechnology Information (NCBI) website. The 16S rDNA gene along with their closest homology sequences were aligned using multiple sequence alignment program CLUSAL W implemented in MEGA X software by using default parameters (Kumar et al., 2018). The phylogenetic tree was constructed by the neighbor-joining (NJ) method using MEGA X software and evolutionary distances were computed with the help of Kimura's 2 parameter models (Kimura, 1980; Saitou and Nei, 1987). The 16S rDNA gene sequences obtained in the present study were submitted to the GenBank nucleotide sequence database.
Halotolerance Assay
Followed by identification, the four selected endophytic isolates were then screened for salt-tolerance properties using nutrient agar (NA) media supplemented with various levels of NaCl (w/v) such as, 0.63% (107 mM), 1.25% (213 mM), 2.50% (427 mM), 5% (854 mM), 7.50% (1.30 M), 10% (1.71 M), and 15% (2.60 M). Control plate was maintained with 0.05% (8.54 mM) NaCl (w/v). The plates were inoculated with fresh culture following the streak plate method and incubated for 48 h at 30°C and the growth on the NaCl-supplemented plates was compared with the control plate (Albdaiwi et al., 2020).
Biofilm Detection Assay
Biofilm formation under varying salt concentration by selected bacterial isolates was determined using microtiter plate assay (Auger and Gohar, 2006; Qurashi and Sabri, 2012b). Bacterial cultures were inoculated into LB broth with varying salt concentrations (0, 100, and 200 mM NaCl) in a culture flask and incubated for 24 h at 30°C. After incubation, 200 μl cultures (~108 CFU/ml) were transferred to each of the 96 wells polyvinylchloride microtiter plate (Greiner BIO-ONE, Germany) for further incubation at 30°C for 48 h. Plates were washed twice with distilled water, left at room temperature for 30 min. Surface-bound cells were stained with 0.01% (w/v) crystal violet solution at room temperature for 20 min. The wells were then washed with phosphate-buffered saline three times and the dye was solubilized with 200 μl ethanol. The absorbance at 570 nm of the solubilized dye was subsequently determined.
Biofilm Detection in Soil
To evaluate the biofilm-forming ability of the isolates at different NaCl concentrations in soil, the method described in Qurashi and Sabri (2012b) was followed with some modifications. Briefly, garden soil was autoclaved at 121°C for 40 min and the sterile plastic pots were filled with sterile soil where a sterilized glass slide was placed into each pot. In control pots, neither salt nor an inoculum was used while in test pots; salt concentrations of 0, 100, and 200 mM NaCl in 20 ml solution with an initial bacterial inoculum of ~108 CFU/ml in 120 g soil were applied in each pot. In the control pot, the inoculum and salt volume were replaced with sterile distilled water. The pot was sealed and kept in the incubator (ESCO, Singapore) at 37°C for 15 days. After the incubation, the glass slide was removed from the pot and washed carefully with phosphate buffer saline (PBS) to remove the soil particles. The glass slide was put in a flask containing PBS placed on a shaker at 150 rpm for 30 min at 37°C. The bacterial count was estimated in CFU/ml by culturing them in nutrient agar plates through the drop plate method after serial dilution which revealed the biofilm-forming capability of the isolates in soil condition.
Quantitative Analysis of EPS Production
Production of EPS by the selected bacterial isolates was determined during the growth of a batch culture in particular salt concentrations (0, 100, and 200 mM NaCl) (Qurashi and Sabri, 2012b). Briefly, 250 ml flasks containing 100 ml of a medium (Verhoef et al., 2003) was inoculated with freshly grown bacterial culture (~108 CFU/ml) and incubated on a shaker at 160 rpm at 30°C for 48 h. Following centrifugation, the supernatant was collected and added with three volumes of pre-chilled absolute ethanol and placed at 4°C for 24 h to precipitate the EPS fraction. Following centrifugation at 15,000 rpm for 20 min, the pellet containing bacterial EPS was separated and dried at 58°C for 24 h. The dry weight of the collected EPS was measured afterward. All the experiments were performed in triplicate.
Glucose Quantification in the Produced EPS
Total carbohydrate content was further determined according to the phenol sulfuric acid method from the dried EPS as described earlier (Dubois et al., 1956).
Biofilm Qualitative Analysis by Scanning Electron Microscope (SEM)
Biofilm formation by bacterial isolates was observed by SEM as described earlier (Sultana et al., 2020). Briefly, isolates were grown in nutrient agar with 0, 100, and 200 mmol/L NaCl overnight, and a single colony was taken and dispersed in absolute ethanol (99.5%) dropped into a glass slide, and dried out under a UV lamp. The sample was observed after platinum coating at different magnification and resolution and the images were captured for the SEM study.
Results
Isolation of Endophytic Bacteria
Plating the homogenate of collected root samples of Oryza sativa on nitrogen-free media, Jensen's and Ashby's agar gave a selective advantage of isolating nitrogen-fixing bacteria from non-fixers. Seventy-five endophytic bacteria were isolated and were ID'd based on their origin and respective isolation media.
In vitro Assessment of Plant Growth Promoting (PGP) Traits
Indole Acetic Acid Production Assay
The bacterial isolates in their respective culture of ~108 CFU/ml were screened for their ability to produce IAA. The IAA production ranged from 1.20 to 60.13 μg/ml with R4A3 producing the maximum (60.13 μg/ml) (Supplementary Table 1).
Phosphate Solubilization Assay
The isolates were tested for their ability to solubilize inorganic phosphate from the media. In Pikovskaya's medium, satisfactory halo zones were not observed (data not shown) but in the NBRIP medium, 57 out of 75 (76%) isolates were able to solubilize phosphate and formed clear zones. Estimated solubilization was recorded as 0.02–1.81 μg/ml (Supplementary Table 1).
Nitrogen Fixation Assay
The isolated endophytes were supposed to fix atmospheric N2 as they could grow in nitrogen-free Jensen's and Ashby's medium. The amount of nitrogen fixation varied from 70.24 to 198.70 μg/ml. R4A6 was found as the most efficient one fixing atmospheric nitrogen (198.70 μg/ml) (Supplementary Table 1).
Thirty isolates out of 75 were short-listed for further study (Supplementary Table 1), thanks to their superior in vitro plant growth-promoting assays, their respective quantitative values are illustrated (Figure 2).
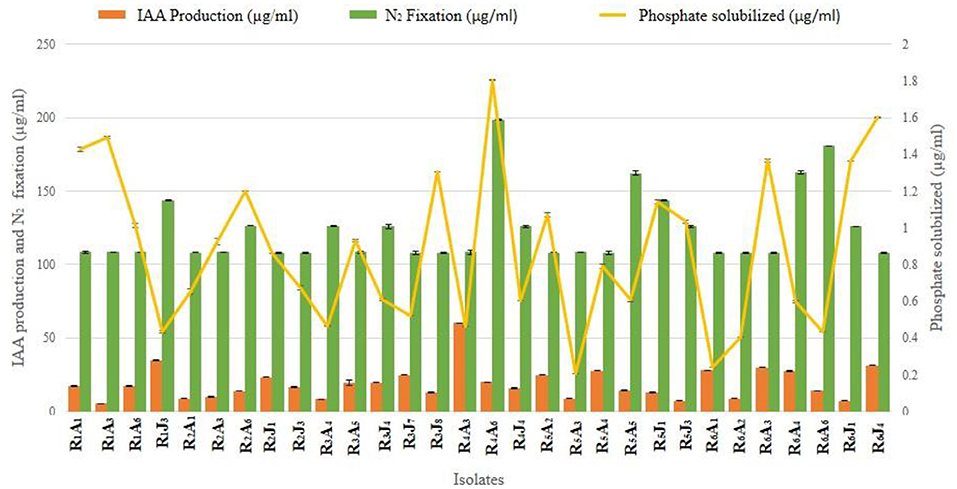
Figure 2. A representative illustration of in vitro plant growth-promoting traits of 30 endophytic PGPR isolates shortlisted from seventy-five, that showed an elevated level of productivity [Nitrogen fixation (108–198.7 μg/ml), Phosphate solubilization (0.2–1.8 μg/ml), and IAA production (5.4–60.1 μg/ml)]. Error bars represent the mean ± SD (n = 3).
ARDRA Analysis and Identification of the Isolates
For the restriction analysis of the amplified rDNA procedures, the 16S rDNAs were isolated from the short-listed thirty isolates by PCR using the universal primers that produced ~1,465 bp amplification products (Figure 3A) following the method described earlier (Sultana et al., 2020). Upon digestion by restriction enzyme, the 16S rDNAs amplicon of these isolates produced a pattern (Figure 3B) that could be grouped in four clusters, named A, B, C and D in the resulting dendrogram that included 5 (16.67%), 16 (53.33%), 4 (13.33%), and 5 (16.67%) isolates, respectively (Figure 3C). Based on overall PGP attributes (IAA production, Nitrogen fixation and Phosphate solubilization), one finest isolate from each cluster, named R5A4, R4A6, R6J4, and R6A1 representing clusters A, B, C, and D were chosen, respectively, with a view to sequencing their respective 16S rDNA genes after PCR amplification. The raw tracer files found from the sequencing were manually corrected and assembled by SeqMan for a homology search using the BLAST tool of NCBI and a phylogenetic tree was constructed (Figure 3D) where the percentage of replicate trees in which the associated taxa clustered together in the bootstrap test (1,000 replicates) are shown next to the branches (Felsenstein, 1985). Sequence analysis of the 16S rDNA gene revealed that isolates R4A6, R5A4, R6A1, and R6J4 were identified as Enterobacter cloacae GRF2, Stenotrophomonas pavanii BRF2, Achromobacter xylosoxidans DRF2, and Bacillus aryabhattai DRF1, respectively. These sequences were then submitted to GenBank [https://www.ncbi.nlm.nih.gov/nuccore/?term=MT768046:MT768049accn] that assigned the isolates R4A6, R5A4, R6A1, and R6J4 with accession numbers (Table 1) after necessary verification.
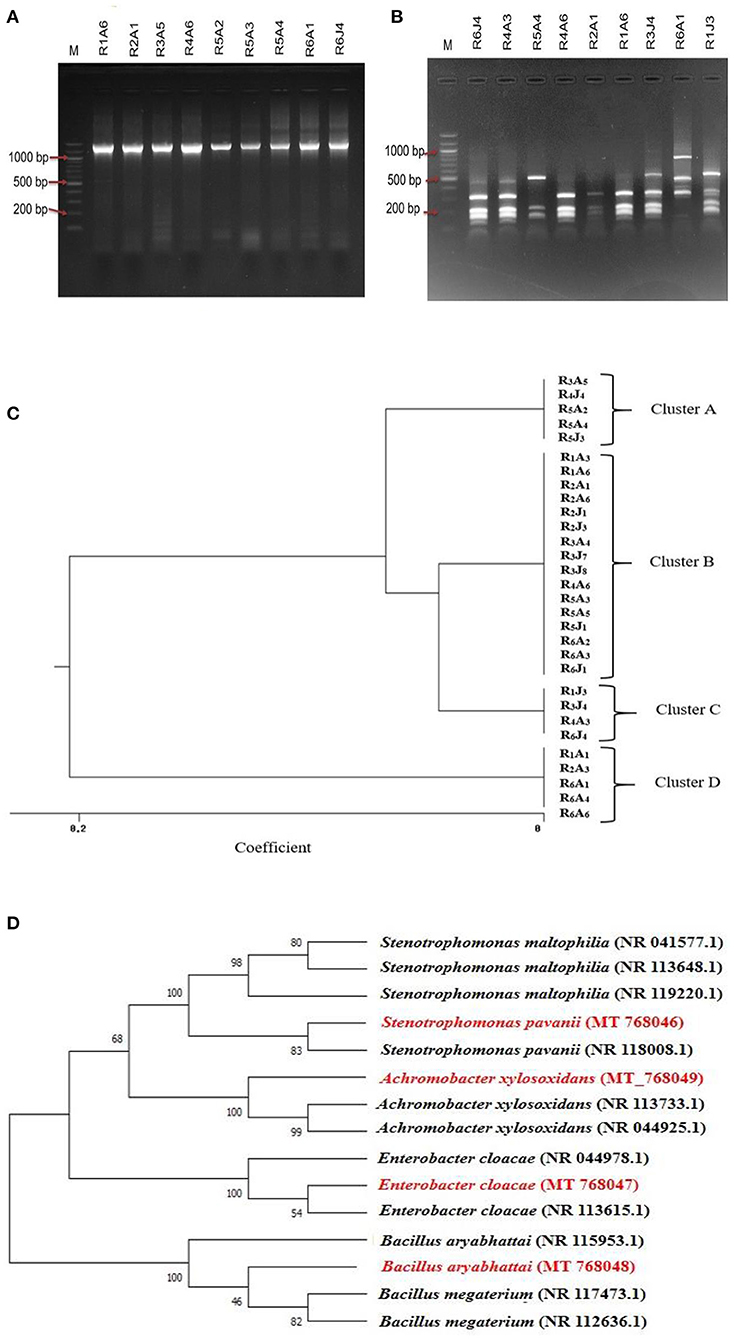
Figure 3. A representative illustration of 16S rDNA products amplified from the respective bacterial genome by PCR and electrophoresed on a 1.5% agarose gel (A) and ARDRA analysis of the 16S rDNA amplicon digested with the BsuRI (HaeIII) enzyme in a 1.5% (w/v) agarose gel; M: 100 bp ladder (B), and the resulting restriction pattern was used to produce a Dendrogram (C). The phylogenetic tree shows evolutionary distance among isolates based on 16S rDNA gene sequence using MEGA X. Bootstrap values are represented by numbers at the nodes based on 1,000 replications. The scale is the evolutionary distance value (D).

Table 1. Identity profile of 16S rDNA gene partial sequence of four isolates according to BLAST identification.
Halotolerance Assay
The four identified endophytes were tested for their salt tolerance abilities. All of them showed a great level of tolerance. The tolerance ranged from 1.37 mol/L, exhibited by A. xylosoxidans DRF2, to 2.05 mol/L by E. cloacae GRF2 and S. pavanii BRF2, up to 2.57 mol/L NaCl concentration, demonstrated by B. aryabhattai DRF1.
Biofilm Formation Assay
The biofilm formation of the isolates at different salt stress (0, 100, and 200 mM NaCl) was estimated in microtiter plates along with a positive control for biofilm formation, Pseudomonas spp, collected from the departmental repository. The test isolates exhibited a different pattern of biofilm formation with increasing salt concentrations. At non-saline conditions, although E. cloacae GRF2 showed the highest potential, S. pavanii BRF2 outcompeted the rest at 200 mM salt stress (P < 0.001). The other strains produced comparable biofilm formation as efficiently as the positive control under saline conditions (P = 0.11) (Figure 4A). Under soil condition, which is happened to be a natural medium, the efficacy of biofilm formation was evaluated by measuring changes of growth of the isolates (in CFU/ml) at different salt concentrations (Figure 4B). Isolate S. pavanii BRF2 was observed to produce prolific biofilm as compared to others (p < 0.001) under different stress conditions. It produced the highest amount of biofilm at 200 mM NaCl concentration which is 33.93% greater than in 100 mM NaCl concentration. The estimate was also found increased for B. aryabhattai DRF1 measured 26.32 and 57.89% in CFU/ml at 100 and 200 mM NaCl concentrations, respectively, compared to that of no added salt condition.
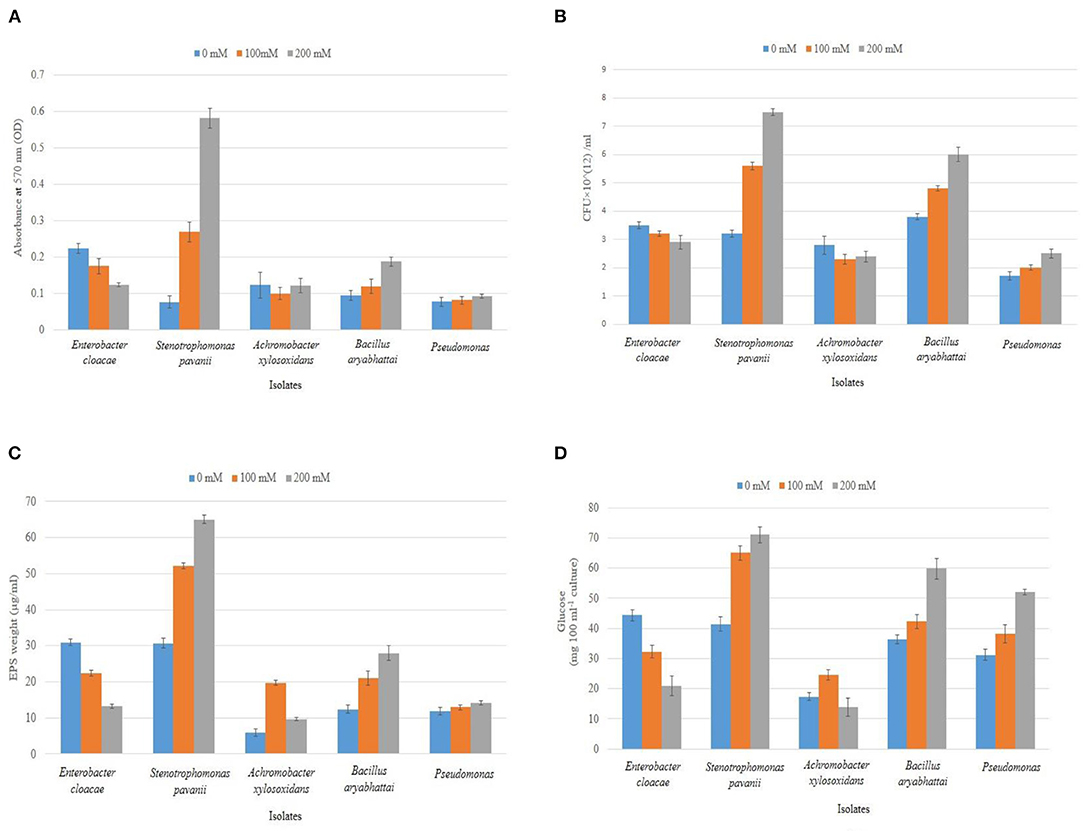
Figure 4. Biofilm formation of the identified isolates was studied in microtiter plates (A) and under soil condition (B), followed by the estimation of respective EPS materials in dry weight (C) and their corresponding Glucose content (D) at varying salt concentrations (0, 100, and 200 mM NaCl). Error bars represent the mean ± SD (n = 3).
The biofilms are mainly composed of EPS, a high molecular weight carbohydrate compound. We attempted to quantitate its amount in the biofilm materials of the isolates by taking the dry weight of EPS and measuring the glucose content of the EPS. As expected, S. pavanii BRF2 produced the highest amount (65 μg/ml) followed by B. aryabhattai DRF1 (28 μg/ml) at 200 mM NaCl concentration, and this production was found significant when compared to the positive isolate (p < 0.05). The other strains produced a comparable amount of EPS with the positive control (Figure 4C). The total carbohydrates in EPS showed an increase of 73.17% (71.06 mg 100 ml−1 culture) and 64.6% (59.77 mg 100 ml−1 culture) for S. pavanii BRF2 and B.aryabhattai DRF1, respectively, at 200 mM NaCl concentration compared to that of non-saline condition (p < 0.05 for both). On the contrary, the isolate E. cloacae GRF2 had a 52.9% (20.90 mg 100 ml−1 culture) decrease of EPS accumulation (Figure 4D).
Biofilm Qualitative Analysis by Scanning Electron Microscope (SEM)
An SEM for observation of bacterial biofilm formation revealed a clear capsule-like layer outside the cell surface, which was more evident when the salt concentration was gradually increased from 0 to 200 mM indicating the formation of biofilm was stress-driven. Here, the SEM of S. pavanii BRF2 is shown as a representative salt-tolerant isolate (Figure 5).
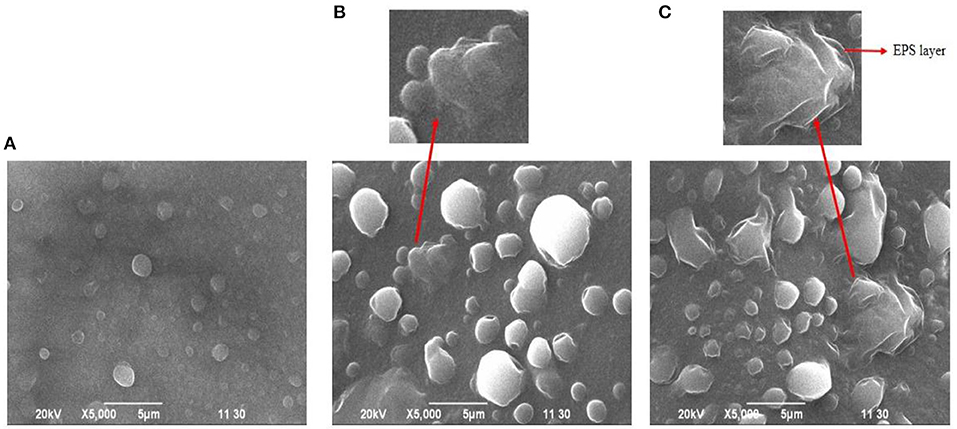
Figure 5. Biofilm formation by S. pavanii BRF2 at 0 (A), 100 (B), and 200 mM (C) NaCl concentrations under a scanning electron microscope.
Discussion
Today's agriculture sector is being stroked by drastic climate changing conditions and the consequent salt intrusion has shrunk coastal agricultural lands ending up creating food insecurity and unsustainability for the ever-increasing population worldwide (Nabti et al., 2015; Shrivastava and Kumar, 2015; Szabo et al., 2016; Ansari et al., 2019). Current approaches of different irrigation methods, traditional breeding, and genetic engineering of salt-tolerant transgenic plants are highly technical and labor -intensive, and thus difficult to implement in practice (Singh et al., 2015; Niu et al., 2018). In order to counter salinity stress with a view to improving crop yields in salinity-prone coastal agricultural lands, the application of PGPR in the form of bioinoculants/ biofertilizer has emerged as a part of climate -smart agricultural practice under the changing climate conditions (Nabti et al., 2015; Sharma et al., 2016; Ansari and Ahmad, 2018).
Many endophytes have been reported to be endowed with the inherent capability to cope with salt stress and stimulate stress resilience in plant growth under saline conditions when inoculated with plants (Egamberdieva et al., 2019; Kearl et al., 2019). With a view to creating such bio-bank, here we isolated 75 salt-tolerant endophytes associated with roots of Oryza sativa from the low-lying southern coastal region (pH > 7, EC > 7dS/m) of Bangladesh close to the Bay of Bengal, where periodic flushing from seawater has been reported to increase soil salinity. To mention, soil samples having EC > 4 dS/m can be referred to as saline soil (Bhat et al., 2020). One of the significant findings of this study is the screening of IAA producing, phosphate solubilizing, and nitrogen-fixing potential endophytes (analyzed in vitro) with an anticipation that they can stimulate the plant growth in the fields under saline condition if provided in the form of biofertilizer; a similar was experienced in our earlier study (Sultana et al., 2020).
IAA is the major auxin in plants, that maintains the growth and developmental stages of plants such as tissue elongation and cell division, responses to light, gravity, and pathogens, and so on (Glick, 2012). Experiments showed that elevated salt stress affects the concentration of IAA in both xylem and phloem in plants (Junghans et al., 2006). Furthermore, IAA plays a major role in plant-microbe interactions which could be destabilized during different abiotic stresses (e.g., salt stress) (Spaepen and Vanderleyden, 2011). Therefore, the higher IAA producing salt-tolerant endophytes could help the growth-cornered plants by providing additional IAA under salinity conditions as well as furnishing the rhizosphere. In our study, 11 isolates out of 75 were able to produce IAA in a range of 20–55 μg/ml (Supplementary Table 1), which is similar or even higher than other reported PGPR that promoted growth of many crops (Sharma et al., 2016). On the other hand, the poor level of phosphorus in saline-prone cultivable lands leaves no other option for the farmers but to use excess conventional phosphorus-containing fertilizers to improve agricultural productivity, which otherwise causes potential surface water pollution, eutrophication, and fertility depletion in soil. The Phosphate Solubilizing Microorganisms (PSM) can improve the growth and yield of crops by mineralizing insoluble soil phosphate to release soluble phosphorus and making it available to plants. Thus, inoculating crops with PSM which can play the same role under saline conditions is a promising strategy to improve world food production without causing any environmental hazard (Alori et al., 2017). In our study, 57 isolates out of 75 were able to solubilize insoluble phosphate in NBRIP media indicating their potentiality to promote the growth of plants under phosphate limited conditions. In quantitative analysis, 12 isolates could solubilize phosphate in a range of 1–2 μg/ml which is lower than the previously reported study on epiphytes (Albdaiwi et al., 2020; Sultana et al., 2020). Concurrently, intervention between salinity and nitrogen availability in soil is a very complex network disturbing almost all processes in plant metabolism and development. Many reports demonstrate that salt-tolerant bacteria associated with the rhizosphere have been found to show better survival, nodulation, nitrogen fixation, and profound nitrogen metabolism under saline conditions (Bala et al., 1990). Salinity adversely affects root nodulation and induces premature senescence of already formed nodules (Swaraj and Bishnoi, 1999). Thus, salt-tolerant endophytes with nitrogen-fixing ability are considered a potential resource for saline soil-based agriculture (Etesami and Beattie, 2018). Thirty-nine isolates found in our study were able to fix a significant amount of nitrogen (108–198.7 μg/ml), hence can be considered as a potential weapon for biological nitrogen fixation if supplied during cultivation.
Biofilm development by different bacterial species is an elicited adaptation mechanism of survival in a harsh environment. The role of biofilm developed by endophytic PGPR is now considered as an important trait for effectively surviving in soil and root surface through colonization which is even more significant under salt stress (Santoyo et al., 2016). It was previously reported that biofilm protected bacterial cells at elevated salt stress by forming exopolysaccharide (EPS), a high molecular weight carbohydrate compound attached to the outer surface of bacteria allowing them to attach with each other and to surfaces of plant roots and soil particles (Qurashi and Sabri, 2012a). Bacterial EPS were reported to form a water-absorbing sheath around the roots to support the root system in excessive Na+ contained soils (Ashraf and Harris, 2004; Rolli et al., 2015). Moreover, EPS synthesized by endophytic bacteria might bind cations including Na+, decreasing Na+ availability for plant uptake and consequently improving the salinity tolerance of the plant (Ashraf and Harris, 2004; Siddikee et al., 2011). Inoculation of EPS producing PGPR showed ameliorative effects on the uptake of K+, Na+ and Ca2+ in plants which resulted in stabilization of soil aggregates and regulation of nutrients and water flow across plant roots through biofilm formation (Grover et al., 2011; Kasim et al., 2016; Ilangumaran and Smith, 2017; Abbas et al., 2019; Egamberdieva et al., 2019). In this study, we report that the intrinsic ability of screened isolates to form biofilm in vitro and ‘in soil' condition was enhanced with increasing NaCl concentrations which is in accordance with previous reports (Qurashi and Sabri, 2012a,b; Kasim et al., 2016). The isolate, S. pavanii BRF2 demonstrated strong biofilm formation under saline conditions (Figure 4), and this was well-supported in SEM (Figure 5) images. Both the isolates S. pavanii BRF2 and B. aryabhattai DRF1 outcompeted others in forming biofilm and EPS under the additional saline concentrations (200 mM NaCl). Since the isolates were not challenged beyond 200 mM NaCl, the peak for the S. pavanii BRF2 and B. aryabhattai DRF1 could not be measured, however, it was well-understood for A. xylosoxidans DRF2 (100 mM NaCl).
Our previous study provided a documentary regarding salt-tolerant epiphytes and their demonstrated proven role to support the yield and overall growth of crops under saline conditions (Sultana et al., 2020). In addition to the reported IAA producing, phosphate solubilizing, and nitrogen fixing potentials, the PGPR isolate, B. aryabhattai MS9 was shown in another study to reveal its siderophore producing ability in an iron-poor condition under salinity stress (Sultana et al., 2021), making it a suitable candidate for composing biofertilizer. It is also notable that B. aryabhattai has been found as a common PGPR member (both epi- and endophytes) with profound PGP activities (Park et al., 2017; Shen et al., 2019). Here, we isolated some potential endophytes, collected from the roots of rice plants grown in saline soil with significant PGP attributes: IAA production, phosphate solubilization, and N2 fixation (Figure 2). Being the endophytes, these were already acclimatized in the plants' system biology, and that too under the stress condition, where they established a symbiotic relationship with plants, a phenomenon well-supported by other reports (Nautiyal et al., 2013; Khalifa et al., 2016; Abdel-Rahman et al., 2017; Singh and Jha, 2017). Furthermore, their salt-tolerance properties associated with the significant amount of EPS production suggest the mechanism behind their survivability under stress conditions. As endophytic microorganisms from plants of different ecosystems do not involve host specificity (Nair and Padmavathy, 2014), this report supports the notion of other reports about endophytes being a substantial alternative strategy to plants for alleviating abiotic stresses arising from changeful environmental conditions (Khalifa et al., 2016; Haidar et al., 2018; Niu et al., 2018). Overall, the findings from this report support the development of sustainable biotechnological approaches toward the application of endophytes in the improvement of crop yield under stressful conditions.
Conclusion
The present study demonstrated that biofilm-forming salt-tolerant endophytes with multifarious PGP attributes could be utilized as promising bioinoculants for salinity stress management. The positive effects exhibited by the four isolates need to be evaluated in vivo experiments under saline conditions for further practical exploitation in crop production. Utilization of this green biotechnology will have multi-faceted positive impacts and could be a savior for saline-prone areas. In the future, these salt-tolerant endophytic PGPR could improve crop production in an economically sustainable manner that would add value to the preparedness strategy for climate change.
Data Availability Statement
The datasets presented in this study can be found in online repositories. The names of the repository/repositories and accession number(s) can be found below: NCBI (Accession: MT768046-MT768049).
Author Contributions
MK conceptualized the idea. TJ and JR did the bench works. SS, TJ, and MK drafted the manuscript and worked on literature review. MR critically reviewed the manuscript. All authors approved the final manuscript.
Funding
We thankfully acknowledge the financial support provided by the University Grants Commission [6(75)/UGC/S&T/Bio-05/2018/2732, dated 27 March 2019], Government of the People's Republic of Bangladesh in conducting the research.
Conflict of Interest
The authors declare that the research was conducted in the absence of any commercial or financial relationships that could be construed as a potential conflict of interest.
Publisher's Note
All claims expressed in this article are solely those of the authors and do not necessarily represent those of their affiliated organizations, or those of the publisher, the editors and the reviewers. Any product that may be evaluated in this article, or claim that may be made by its manufacturer, is not guaranteed or endorsed by the publisher.
Supplementary Material
The Supplementary Material for this article can be found online at: https://www.frontiersin.org/articles/10.3389/fsufs.2021.687531/full#supplementary-material
References
Abbas, R., Rasul, S., Aslam, K., Baber, M., Shahid, M., Mubeen, F., et al. (2019). Halotolerant PGPR: a hope for cultivation of saline soils. J. King Saud Univer. Sci. 31, 1195–1201. doi: 10.1016/j.jksus.2019.02.019
Abdel-Rahman, H. M., Salem, A. A., Moustafa, M. M. A., and El-Garhy, H. A. S. (2017). A novice Achromobacter sp. EMCC1936 strain acts as a plant-growth-promoting agent. Acta Physiol. Plant. 39:61. doi: 10.1007/s11738-017-2360-6
Albdaiwi, R. N., Khyami-Horani, H., Ayad, J. Y., Alananbeh, K. M., and Al-Sayaydeh, R. (2020). Isolation and characterization of halotolerant plant growth promoting rhizobacteria from durum wheat (Triticum turgidum subsp. durum) cultivated in saline areas of the dead sea region. Oxidat. Med. Cell. Long. 10, 1–16. doi: 10.3389/fmicb.2019.01639
Alori, E. T., Glick, B. R., and Babalola, O. O. (2017). Microbial phosphorus solubilization and its potential for use in sustainable agriculture. Front. Microb. 8, 1–8. doi: 10.3389/fmicb.2017.00971
Ansari, F. A., and Ahmad, I. (2018). Biofilm development, plant growth promoting traits and rhizosphere colonization by <i>pseudomonas entomophila</i> FAP1: a promising PGPR. Adv. Microb. 08, 235–251. doi: 10.4236/aim.2018.83016
Ansari, F. A., Ahmad, I., and Pichtel, J. (2019). Growth stimulation and alleviation of salinity stress to wheat by the biofilm forming Bacillus pumilus strain FAB10. Appl. Soil Ecol. 143, 45–54. doi: 10.1016/j.apsoil.2019.05.023
Ashraf, M., and Harris, P. J. C. (2004). Potential biochemical indicators of salinity tolerance in plants. Plant Sci. 166, 3–16. doi: 10.1016/j.plantsci.2003.10.024
Auger, S., and Gohar, M. (2006). Autoinducer 2 affects biofilm formation by Bacillus cereus. Appl. Environ. Microbiol. 72, 937–941. doi: 10.1128/AEM.72.1.937
Bala, N., Sharma, P. K., and Lakshminarayana, K. (1990). Nodulation and nitrogen fixation by salinity-tolerant rhizobia in symbiosis with tree legumes. Agricult. Ecosyst. Environ. 33, 33–46. doi: 10.1016/0167-8809(90)90142-Z
Balsanelli, E., Vad, B., Fdo, P., Emd, S., and Monteiro, R. A. (2014). Exopolysaccharide biosynthesis enables mature biofilm formation on abiotic surfaces by Herbaspirillum seropedicae. PLoS ONE. 9:110392. doi: 10.1371/journal.pone.0110392
Bhat, M. A., Kumar, V., Bhat, M. A., Wani, I. A., Dar, F. L., Farooq, I., et al. (2020). Mechanistic insights of the interaction of plant growth-promoting rhizobacteria (PGPR) with plant roots toward enhancing plant productivity by alleviating salinity stress. Front. Microb. 11:1952. doi: 10.3389/fmicb.2020.01952
Boddey, R. M., and Dobereiner, J. (1988). Nitrogen fixation associated with grasses and cereals: recent results and perspectives for future research. Plant Soil 108, 53–65. doi: 10.1007/BF02370099
Dubois, M., Gilles, K. A., Hamilton, J. K., Rebers, P. A., and Smith, F. (1956). Colorimetric method for determination of sugars and related substances. Analyt. Chem. 28, 350–356. doi: 10.1021/ac60111a017
Egamberdieva, D., Wirth, S., Bellingrath-kimura, S. D., Mishra, J., and Arora, N. K. (2019). Salt-tolerant plant growth promoting rhizobacteria for enhancing crop productivity of saline soils. Front. Microbiol. 10, 2791. doi: 10.3389/fmicb.2019.02791
Etesami, H., and Beattie, G. A. (2018). Mining halophytes for plant growth-promoting halotolerant bacteria to enhance the salinity tolerance of non-halophytic crops. Front. Microb. 9:148. doi: 10.3389/fmicb.2018.00148
Felsenstein, J. (1985). Confidence limits on phylogenies: an approach using the bootstrap. Evol. 39, 783–791. doi: 10.2307/2408678
Glick, B. R. (2012). Plant growth-promoting bacteria: mechanisms and applications. Scientifica 2012:963401. doi: 10.6064/2012/963401
Glick, B. R. (2014). Bacteria with ACC deaminase can promote plant growth and help to feed the world. Microb. Res. 169, 30–39. doi: 10.1016/j.micres.2013.09.009
Gordan, S. A., and Weber, R. (1950). COLORIMETRIC ESTIMATION OF INDOLEACETIC ACID. Analyt. Biochem. 72, 134–138. doi: 10.1016/0003-2697(76)90514-5
Grover, M., Ali, S. Z., Sandhya, V., Rasul, A., and Venkateswarlu, B. (2011). Role of microorganisms in adaptation of agriculture crops to abiotic stresses. World J. Microb. Biotechn. 27, 1231–1240. doi: 10.1007/s11274-010-0572-7
Gupta, B., and Huang, B. (2014). Mechanism of salinity tolerance in plants: physiological, biochemical, and molecular characterization. Int. J. Genomics 2014:701596. doi: 10.1155/2014/701596
Haidar, B., Ferdous, M., Fatema, B., Ferdous, A. S., Islam, M. R., and Khan, H. (2018). Population diversity of bacterial endophytes from jute (Corchorus olitorius) and evaluation of their potential role as bioinoculants. Microbiol. Res. 208, 43–53. doi: 10.1016/j.micres.2018.01.008
Hallmann, J., Quadt-Hallmann, A., Mahaffee, W. F., Kloepper, J. W., Quadt-Hallmann,', A, and Kl~epper, J. W. ~. (1997). Bacterial endophytes in agricultural crops J. NRC Canada Can. J. Microbiol. 43, 895–914.
Hashem, A., Abd Allah, E. F., Alqarawi, A. A., Al-Huqail, A. A., and Shah, M. A. (2016). Induction of osmoregulation and modulation of salt stress in Acacia gerrardii Benth. by arbuscular mycorrhizal fungi and Bacillus subtilis (BERA 71). BioMed Res. Int. 2016:6294098. doi: 10.1155/2016/6294098
Ilangumaran, G., and Smith, D. L. (2017). Plant growth promoting rhizobacteria in amelioration of salinity stress: a systems biology perspective. Front. Plant Sci. 8, 1–14. doi: 10.3389/fpls.2017.01768
Islam, F., Yasmeen, T., Ali, S., Ali, B., Farooq, M. A., and Gill, R. A. (2015). Priming-induced antioxidative responses in two wheat cultivars under saline stress. Acta Physiol. Plant. 37:153. doi: 10.1007/s11738-015-1897-5
Junghans, U., Polle, A., Düchting, P., Weiler, E., Kuhlman, B., Gruber, F., et al. (2006). Adaptation to high salinity in poplar involves changes in xylem anatomy and auxin physiology. Plant, Cell Environ. 29, 1519–1531. doi: 10.1111/j.1365-3040.2006.01529.x
Kasim, W. A., Gaafar, R. M., Abou-Ali, R. M., Omar, M. N., and Hewait, H. M. (2016). Effect of biofilm forming plant growth promoting rhizobacteria on salinity tolerance in barley. Ann. Agricult. Sci. 61, 217–227. doi: 10.1016/j.aoas.2016.07.003
Kaushal, M., and Wani, S. P. (2016). Plant-growth-promoting rhizobacteria: drought stress alleviators to ameliorate crop production in drylands. Ann. Microb. 66, 35–42. doi: 10.1007/s13213-015-1112-3
Kearl, J., McNary, C., Lowman, J. S., Mei, C., Aanderud, Z. T., Smith, S. T., et al. (2019). Salt-tolerant halophyte rhizosphere bacteria stimulate growth of alfalfa in salty soil. Front. Microb. 10:1849. doi: 10.3389/fmicb.2019.01849
Khalifa, A. Y. Z., Alsyeeh, A. M., Almalki, M. A., and Saleh, F. A. (2016). Characterization of the plant growth promoting bacterium, Enterobacter cloacae MSR1, isolated from roots of non-nodulating Medicago sativa. Saudi J. Biol. Sci. 23, 79–86. doi: 10.1016/j.sjbs.2015.06.008
Kimura, M. (1980). A simple method for estimating evolutionary rates of base substitutions through comparative studies of nucleotide sequences. J Mol Evol. 16:111–120. doi: 10.1007/BF01731581
Kjeldahl, J. (1883). Neue Methode zur Bestimmung des Stickstoffs in organischen Körpern. Zeitschrift Für Analyt. Chem. 22, 366–382. doi: 10.1007/BF01338151
Kumar, S., Stecher, G., Li, M., Knyaz, C., and Tamura, K. (2018). MEGA X: molecular evolutionary genetics analysis across computing platforms. Mol. Biol. Evol. 35(6): 1547–1549. doi: 10.1093/molbev/msy096
Lazarovits, G., and Nowak, J. (1997). Rhizobacteria for improvement of plant growth and establishment. HortSci. 32(2): 188–192.
Munns, R. (2005). Genes and salt tolerance: bringing them together. N. Phytol. 167, 645–663. doi: 10.1111/j.1469-8137.2005.01487.x
Nabti, E., Schmid, M., and Hartmann, A. (2015). “Application of halotolerant bacteria to restore plant growth under salt stress,” in Halophiles. Sustainable Development and Biodiversity, Vol. 6, eds D. Maheshwari and M. Saraf (Cham: Springer). doi: 10.1007/978-3-319-14595-2_9
Nair, D., and Padmavathy, S. (2014). Impact of endophytic microorganisms on plants, environment and humans. Sci. World J. 2014, 1–11. doi: 10.1155/2014/250693
Nautiyal, C. S. (1999). An efficient microbiological growth medium for screening phosphate solubilizing microorganisms. FEMS Microb. Lett. 170, 265–270. doi: 10.1016/S0378-1097(98)00555-2
Nautiyal, C. S., Srivastava, S., Chauhan, P. S., Seem, K., Mishra, A., and Sopory, S. K. (2013). Plant growth-promoting bacteria Bacillus amyloliquefaciens NBRISN13 modulates gene expression profile of leaf and rhizosphere community in rice during salt stress. Plant Physiol. Biochem. 66, 1–9. doi: 10.1016/j.plaphy.2013.01.020
Niu, X., Song, L., Xiao, Y., and Ge, W. (2018). Drought-tolerant plant growth-promoting rhizobacteria associated with foxtail millet in a semi-arid and their potential in alleviating drought stress. Front. Microb. 8, 1–11. doi: 10.3389/fmicb.2017.02580
Park, Y. G., Mun, B. G., Kang, S. M., Hussain, A., Shahzad, R., Seo, C. W., et al. (2017). Bacillus aryabhattai SRB02 tolerates oxidative and nitrosative stress and promotes the growth of soybean by modulating the production of phytohormones. PLoS ONE. 12, 1–28. doi: 10.1371/journal.pone.0173203
Pikovskaya, R. I. (1948). Mobilization of phosphorus in soil connection with the vital activity of some microbial species. Microbiology. 17, 362–370.
Queipo-Ortuño, M. I., De Dios Colmenero, J., Macias, M., Bravo, M. J., and Morata, P. (2008). Preparation of bacterial DNA template by boiling and effect of immunoglobulin g as an inhibitor in real-time PCR for serum samples from patients with brucellosis. Clin. Vaccine Immun. 15, 293–296. doi: 10.1128/CVI.00270-07
Qurashi, A. W., and Sabri, A. N. (2012a). Bacterial exopolysaccharide and biofilm formation stimulate chickpea growth and soil aggregation under salt stress. Brazil. J. Microb. 43, 1183–1191. doi: 10.1590/S1517-83822012000300046
Qurashi, A. W., and Sabri, A. N. (2012b). Biofilm formation in moderately halophilic bacteria is influenced by varying salinity levels. J. Basic Microb. 52, 566–572. doi: 10.1002/jobm.201100253
Rima, F. S., Biswas, S., Sarker, P. K., Islam, M. R., and Seraj, Z. I. (2018). Bacteria endemic to saline coastal belt and their ability to mitigate the effects of salt stress on rice growth and yields. Ann. Microb. 68, 525–535. doi: 10.1007/s13213-018-1358-7
Rolli, E., Marasco, R., Vigani, G., Ettoumi, B., Mapelli, F., Deangelis, M. L., et al. (2015). Improved plant resistance to drought is promoted by the root-associated microbiome as a water stress-dependent trait. Environ. Microb. 17, 316–331. doi: 10.1111/1462-2920.12439
Saitou, N., and Nei, M. (1987). The neighbor-joining method: a new method for reconstructing phylogenetic trees'. Mol. Biol. Evol. 4, 406–425. doi: 10.1093/oxfordjournals.molbev.a040454
Santoyo, G., Moreno-Hagelsieb, G., del Carmen Orozco-Mosqueda, M., and Glick, B. R. (2016). Plant growth-promoting bacterial endophytes. Microb. Res. 183, 92–99. doi: 10.1016/j.micres.2015.11.008
Shahid, S. A., Zaman, M., and Heng, L. (2018). “Soil salinity: historical perspectives and a world overview of the problem,” in Guideline for Salinity Assessment, Mitigation and Adaptation Using Nuclear and Related Techniques (Cham: Springer). doi: 10.1007/978-3-319-96190-3_2
Sharma, S., Kulkarni, J., and Jha, B. (2016). Halotolerant rhizobacteria promote growth and enhance salinity tolerance in peanut. Front. Microbiol. 7:1600. doi: 10.3389/fmicb.2016.01600
Shen, F. T., Yen, J. H., Liao, C., Sen, C., hen, W. C., and Chao, Y. T. (2019). Screening of rice endophytic biofertilizers with fungicide tolerance and plant growth-promoting characteristics. Sustainability 11:1133. doi: 10.3390/su11041133
Shrivastava, P., and Kumar, R. (2015). Soil salinity: a serious environmental issue and plant growth promoting bacteria as one of the tools for its alleviation. Saudi J. Biol. Sci. 22, 123–131. doi: 10.1016/j.sjbs.2014.12.001
Siddikee, M. A., Glick, B. R., Chauhan, P. S., Yim, W., jong, and Sa, T. (2011). Enhancement of growth and salt tolerance of red pepper seedlings (Capsicum annuum L.) by regulating stress ethylene synthesis with halotolerant bacteria containing 1-aminocyclopropane-1-carboxylic acid deaminase activity. Plant Physiol. Biochem. 49, 427–434. doi: 10.1016/j.plaphy.2011.01.015
Singh, R., Jha, P., and Jha, P. N. (2015). The plant-growth-promoting bacterium Klebsiella sp. SBP-8 confers induced systemic tolerance in wheat (Triticum aestivum) under salt stress. J. Plant Phys. 184, 57–67. doi: 10.1016/j.jplph.2015.07.002
Singh, R. P., and Jha, P. N. (2017). The PGPR stenotrophomonas maltophilia SBP-9 augments resistance against biotic and abiotic stress in wheat plants. Front. Microb. 8:1945. doi: 10.3389/fmicb.2017.01945
Soil Resource Development Institute. (2010). Saline Soils of Bangladesh Surveyed and data Compiled by Soil Resource Development Institute. Retrieved from: http://srdi.portal.gov.bd/sites/default/files/files/srdi.portal.gov.bd/publications/bc598e7a_df21_49ee_882e_0302c974015f/Soil/salinity/report/Nov/2010.pdf (accessed July 03, 2021).
Spaepen, S., and Vanderleyden, J. (2011). Auxin and plant-microbe interactions. Cold Spring Harbor Perspect. Biol. 3:a001438. doi: 10.1101/cshperspect.a001438
Stevens, P., and Van Elsas, J. D. (2010). Genetic and phenotypic diversity of Ralstonia solanacearum biovar 2 strains obtained from Dutch waterways. Int. J. Gen. Mol. Microb. 97, 171–188. doi: 10.1007/s10482-009-9400-1
Sultana, S., Alam, S., and Karim, M. M. (2021). Screening of siderophore-producing salt-tolerant rhizobacteria suitable for supporting plant growth in saline soils with iron limitation. J. Agricult. Food Res. 4:100150. doi: 10.1016/j.jafr.2021.100150
Sultana, S., Paul, S. C., Parveen, S., Alam, S., Rahman, N., Jannat, B., et al. (2020). Isolation and identification of salt-tolerant plant-growth-promoting rhizobacteria and their application for rice cultivation under salt stress. Can. J. Microb. 66, 144–160. doi: 10.1139/cjm-2019-0323
Swaraj, K., and Bishnoi, N. R. (1999). Effect of salt stress on nodulation and nitrogen fixation in legumes. Indian J. Exp. Biol. 37, 843–848.
Szabo, S., Hossain, M. S., Adger, W. N., Matthews, Z., Ahmed, S., Lázár, A. N., et al. (2016). Soil salinity, household wealth and food insecurity in tropical deltas: evidence from south-west coast of Bangladesh. Susta. Sci. 11, 411–421. doi: 10.1007/s11625-015-0337-1
Verhoef, R., De Waard, P., Schols, H. A., Siika-aho, M., and Voragen, A. G. J. (2003). Methylobacterium sp. isolated from a Finnish paper machine produces highly pyruvated galactan exopolysaccharide. Carbohydr. Res. 338, 1851–1859. doi: 10.1016/S0008-6215(03)00261-1
Vinayarani, G., and Prakash, H. S. (2018). Growth promoting rhizospheric and endophytic bacteria from Curcuma longa L. As biocontrol agents against rhizome rot and leaf blight diseases. Plant Pathol. J. 34, 218–235. doi: 10.5423/PPJ.OA.11.2017.0225
Wakelin, S. A., Warren, R. A., Harvey, P. R., and Ryder, M. H. (2004). Phosphate solubilization by Penicillium spp. closely associated with wheat roots. Biol. Fertil. Soils 40, 36–43. doi: 10.1007/s00374-004-0750-6
Keywords: biofilm, endophytes, plant growth-promoting rhizobacteria, salt-tolerant, sustainable agricultural production
Citation: Jhuma TA, Rafeya J, Sultana S, Rahman MT and Karim MM (2021) Isolation of Endophytic Salt-Tolerant Plant Growth-Promoting Rhizobacteria From Oryza sativa and Evaluation of Their Plant Growth-Promoting Traits Under Salinity Stress Condition. Front. Sustain. Food Syst. 5:687531. doi: 10.3389/fsufs.2021.687531
Received: 29 March 2021; Accepted: 24 June 2021;
Published: 23 July 2021.
Edited by:
Everlon Cid Rigobelo, São Paulo State University, BrazilReviewed by:
Joyce Dória, Universidade Federal de Lavras, BrazilIfigeneia Mellidou, Hellenic Agricultural Organisation (HAO), Greece
Copyright © 2021 Jhuma, Rafeya, Sultana, Rahman and Karim. This is an open-access article distributed under the terms of the Creative Commons Attribution License (CC BY). The use, distribution or reproduction in other forums is permitted, provided the original author(s) and the copyright owner(s) are credited and that the original publication in this journal is cited, in accordance with accepted academic practice. No use, distribution or reproduction is permitted which does not comply with these terms.
*Correspondence: Muhammad Manjurul Karim, bWFuanVyQGR1LmFjLmJk; orcid.org/0000-0003-0476-8239