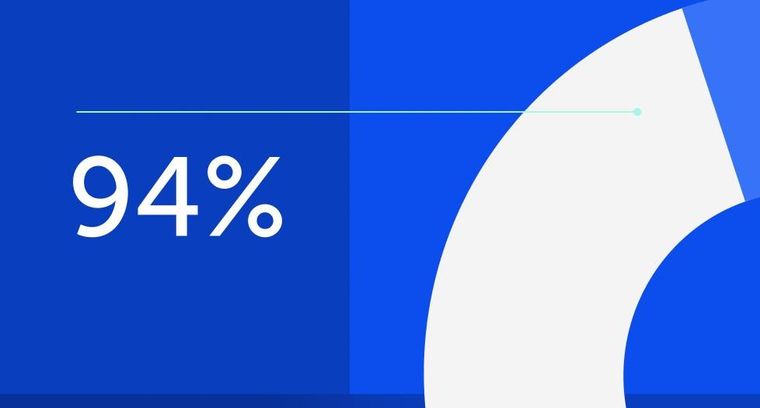
94% of researchers rate our articles as excellent or good
Learn more about the work of our research integrity team to safeguard the quality of each article we publish.
Find out more
ORIGINAL RESEARCH article
Front. Sustain. Food Syst., 28 July 2021
Sec. Crop Biology and Sustainability
Volume 5 - 2021 | https://doi.org/10.3389/fsufs.2021.676207
This article is part of the Research TopicCoffee: From the Field to the CupView all 14 articles
Increasing atmospheric [CO2] is thought to contribute to changes in precipitation patterns, increasing heatwaves and severe drought scenarios. However, how the combination of elevated [CO2] and progressive drought affect plant metabolism is poorly understood. Aiming to investigate the effects of this environmental condition on photosynthesis and specialized metabolites in leaves of Coffea arabica during the early growth, plants fertilized with ambient (a[CO2]-400 ppm) and elevated (e[CO2]-800 ppm) [CO2] were exposed to well-watered (WW) or water-deficit (WD) regimes for 40 days. Over the 40-day-water-withdrawal, soil moisture, and leaf water potential decreased compared to WW-condition. Elevated [CO2] stimulates CO2 assimilation (A) and intrinsic water use efficiency (iWUE) even under WD. Drought condition slightly changed stomatal conductance, transpiration rate and maximum quantum efficiency of photosystem II (PSII) regardless of [CO2] compared to WW-plants. Total soluble amino acid concentration did not change significantly, while total phenolic compounds concentration decreased under e[CO2] regardless of water regimes. The combination of e[CO2]+WD increased the 5-O-caffeoylquinic acid (5-CQA) and caffeine amounts by 40-day when compared to a[CO2]+WD plants. Altogether, these results suggest that e[CO2] buffers mild-drought stress in young C. arabica by increasing A, iWUE and stimulating changes in the leaf contents of 5-CQA and caffeine.
The concentration of carbon dioxide ([CO2]) in the atmosphere is one of the environmental factors of the greatest influence on plant development. CO2 emissions have gradually increased in recent decades as a consequence of anthropogenic activities, with a current concentration around 415 ppm (NOAA, 2020). The increase in atmospheric [CO2] contributes to the greenhouse effect and changes in the precipitation pattern, which could favor flood and more frequent drought events depending on the location (IPCC, 2014). Water deficit represents the main abiotic stress to affect crop production worldwide. To cope with drought conditions, plant metabolism is adjusted including a range of morphological, physiological, biochemical and molecular changes (Seleiman et al., 2021). The initial effects of drought on plants are related to stomatal closure, decrease in leaf area, acceleration of leaf senescence and abscission aiming to control water status. However, these responses reduce the availability of CO2 in the chloroplasts inhibiting photosynthesis and modifying carbohydrate supply for metabolism (McDowell et al., 2008). As drought severity increase biochemical restrictions and oxidative stress impair plant development, which can later cause plant death (Seleiman et al., 2021).
The isolated effect of elevated [CO2] (e[CO2]) on plant growth and development has been studied in a variety of species, especially in annual crops and soybean (Ainsworth and Rogers, 2007; Gamage et al., 2018). These works report a species-specific response, but in general e[CO2] stimulates photosynthesis by increasing [CO2] in the vicinity of Rubisco (ribulose-1,5-bisphosphate carboxylase/oxygenase), and reduces stomatal conductance and water loss. Additionally, plant growth enhancement is often observed, since photorespiration and reactive oxygen species (ROS) synthesis are inhibited in those conditions (DaMatta et al., 2016). Therefore, it is hypothesized that e[CO2] could mitigate the negative effects of drought stress on plants.
Although few studies have involved woody plants, some of them show a promising beneficial effect of e[CO2] when exposed to abiotic stresses (Ramalho et al., 2013; Xu et al., 2015; Sobuj et al., 2018). In Coffea arabica L., an important commodity worldwide, it was recently demonstrated that e[CO2] promoted higher carbon assimilation rate, water use efficiency and biomass under drought conditions when compared to plants exposed to drought and ambient [CO2] in a greenhouse (Sanches et al., 2017). These responses were also related to lower photorespiration rates and higher hydraulic conductance by the increase of aquaporins transcripts, which contributed to a better performance of these plants under water deficit conditions (Avila et al., 2020a,b). In C. arabica and C. canephora, e[CO2] improved photosynthetic efficiency by reducing energy dissipation and PSII activity inhibition of plants exposed to moderate and severe water deficit (Semedo et al., 2021). The mitigating role of e[CO2] was similarly observed in C. arabica trees under field conditions exposed to seasonal water deficit (Sanches et al., 2020). The authors assigned this positive effect to the increased levels of soluble carbohydrates, organic acids and amino acids in leaves of plants subjected to e[CO2].
Despite these important findings, the mechanisms underlying these responses, as well as the role of specialized metabolites, are poorly understood. The coffee tree has a complex phytochemical composition, including caffeine and chlorogenic acids (CGAs) which are both products of plant specialized metabolism that act in plant protection against biotic and abiotic stresses (Frischknecht et al., 1986; Mondolot et al., 2006; Leitão et al., 2008; Gebeyehu and Bikila, 2015). Among the purine alkaloids, caffeine is the most abundant, being found in all organs of coffee plants, with greater abundance in flowers and especially in young leaves, in which a great biosynthetic rate and concentration are noticed (Ashihara, 2006; Abrahão et al., 2010; Perrois et al., 2014; Sunarharum et al., 2014; Da Silva et al., 2018). Concerning phenolic compounds, the 5-O-caffeoylquinic acid (5-CQA) is the most abundant among the chlorogenic acids (CGAs) present in coffee plants, which is considered the main component of the phenolic fraction. 5-CQA is obtained by the esterification between quinic acid (esterification formed on the hydroxyl group at carbon 5) and caffeic acid (Campa et al., 2008). These substances are mainly involved with plant resistance and defense due to their antioxidant properties, acting particularly in ROS scavenging and, consequently, reducing the effects of oxidative stress. Chlorogenic acids seem to be involved in different abiotic stress responses, as observed by Ramakrishna and Ravishankar (2011). Furthermore, the amount of caffeine and chlorogenic acids are economically relevant due to their contribution to the coffee drink quality (Monteiro and Farah, 2012; Cheng et al., 2016).
Recently, the effect of increasing [CO2] on caffeine concentration was investigated in leaves of C. arabica and C. canephora grown under field conditions (Vega et al., 2020). In this work, caffeine amount was negatively correlated with e[CO2] in C. canephora (cv. Robusta), but no interaction was found between [CO2] and caffeine in C. arabica. In coffee beans, it was reported higher concentration of chlorogenic acids and kahweol when plants were exposed to water deficit and e[CO2] (Marcheafave et al., 2020). These two studies clearly showed that somehow e[CO2] and drought stress will affect synthesis/degradation of specialized metabolites in coffee plants. Nevertheless, the mechanisms underlying this process remain unclear, as is whether or not these compounds will be relevant to the acclimation of drought-stressed coffee plants. Therefore, the present study aimed to investigate the combined effect of e[CO2] and progressive water deficit on water relations, gas exchange and specific specialized metabolites in leaves of Coffea arabica L. in an early growth stage. Results related to photosynthesis, water status and specialized metabolites are discussed in an integrative way.
Seedlings of Coffea arabica cv. Catuaí Vermelho IAC-144 (3–4-month-old) obtained in a coffee nursery and having a similar growth pattern were transplanted into 7-liter pots containing Plantmax Café® (DDL Agroindustria Ltda) as substrate, with one plant per pot. After 20-day of acclimation in a greenhouse, plants were transferred to open-top chambers (OTC) installed inside a greenhouse at the Department of Physiology and Biochemistry of the Institute of Botany São Paulo, Brazil, for CO2 treatment acclimation (ambient atmospheric [CO2] – a[CO2] = 400 ppm or elevated atmospheric [CO2] – e[CO2] = 800 ppm), as described by Sanches et al. (2017). The gas injection was applied through a compressed CO2 cylinder attached to the system. During this [CO2] acclimation period, plants were daily watered with tap water and supplied with Hoagland and Arnon's nutrient solution (Hoagland and Arnon, 1950) once a week. After 30 days of [CO2] acclimation period (plants about 7–8 expanded leaf pairs), half of the plants grown in a[CO2] and e[CO2] were subjected to water deficit (WD) by total water withholding, while the other half was maintained on a daily watering regime (WW) for 40 consecutive days, featuring the following treatments: a[CO2]+WW, a[CO2]+WD, e[CO2]+WW and e[CO2]+WD. The experiment was performed from October to December 2018 and only [CO2] inside the OTCs was controlled. The air temperature and relative humidity (HMP45C-L, Campbell Sci.) and photosynthetic active radiation (Li-190R, LiCor) were monitored inside the OTCs over the experiment period. The environmental conditions inside the OTCs during the experimental period were as follows: photosynthetic photon flux density (PPFD) of 302 ± 36 μmol photons m−2 s−1, temperature (day/night) of 27.7 ± 0.6°C/20.9 ± 0.5°C, relative humidity (day/night) of 63.9 ± 1.2%/82.9 ± 0.7%, a[CO2] of 380 ± 41 ppm, e[CO2] of 780 ± 28 ppm, and 12-h of photoperiod (Supplementary Figures 1, 2). During the experimental period, measurements of gas exchange, chlorophyll a fluorescence, water potential (Ψw), soil moisture and sampling of leaf material for further analysis were performed on day 0, 20, and 40 after water deficit imposition. All instantaneous physiological analysis took place in the morning from 06:00 am to 11:30 a.m.
The soil moisture was measured by the Time Domain Reflectometry (TDR) method, using a ML2-x Delta-T Devices model sensor (Theta-Probe, Cambridge, UK). Water potential (Ψw) was measured from 06-07 am using a Scholander pressure pump (model 1000, PMS Instrument Co) in fully expanded leaves of the third to the fourth pair from the apex immediately opposite to the leaves that were used for instantaneous photosynthetic measurements.
The instantaneous net CO2 assimilation rate (A), stomatal conductance (gs), leaf transpiration rate (E), and intercellular CO2 partial pressure (Ci) were determined on fully expanded leaves of the third to fourth pair (from the apex and at the opposite side that Ψw was measured) using a portable infrared gas analyzer (IRGA model LC-SD Pro, ADC Bioscentific), under [CO2] of 400 ppm and 800 ppm, according to plant [CO2] condition, and at photosynthetic saturation light (PPFD of 600 μmol photons m−2 s−1) (Sanches et al., 2017). The intrinsic water use efficiency (iWUE) was calculated as A/gs. The chlorophyll a fluorescence emission was measured on dark-adapted (30 min) leaves according to the saturation pulse (Schreiber, 2004) using a portable fluorometer (OS5p Opti-Sciences, Hudson, NH, USA). The intensity and duration of the saturation pulse were 3,000 μmol m−2 s−1 and 1 s, respectively. The following parameters were assessed: minimum fluorescence (F0), maximum fluorescence (Fm) and maximum quantum efficiency of photosystem II (PSII) [Fv/Fm = (Fm-F0)/Fm)].
Mature leaves (third to fourth pairs from the apex) were taken in each sampling time (day 0, 20, and 40 after water deficit imposition) after the photosynthetic measurements and rapidly snap-frozen in liquid nitrogen followed by storage at −80°C until analysis. Later, the extract for biochemical analysis was performed as follows: leaf samples were freeze-dried, ground in a mill, incubated in 80% ethanol (v/v) for 48 h and constantly mixed. Afterwards, the solution was filtered to obtain the ethanolic extract and it was used in the subsequent analysis.
The total soluble amino acids (TSA) was determined according to Yemm et al. (1955), using L-leucine (Fluka®) as a standard. The absorbance reading was performed on a spectrophotometer (Bel SPECTRO S05) at 570 nm. The concentration of total phenolic compounds (TPC) was quantified according to the Folin-Ciocalteau method (Singleton and Rossi, 1965), modified by Marinova et al. (2005), using gallic acid (Sigma) as a standard. Subsequently, TPC levels were determined in an ELISA microplate reader (KC4, Biotek Instruments) at 750 nm.
The quantification of caffeine and 5-O-caffeoylquinic acid (5-CQA) in the ethanol extracts obtained as described above was performed with samples after drying in a speed-vac system, solubilized in 1 mL of MeOH (Merck), filtered (Millipore filter 0.45 μm), stored in vials for analysis on an Agilent 1260 Liquid Chromatograph equipped with a 60 mm flow cell and photodiode array scanning spectrum detector (HPLC-UV/DAD), according to Tamayose et al. (2019). All analyzes were performed on a C18 reverse-phase column Zorbax Eclipse Plus (150 × 4.6 mm and 3.5 μm particle diameter) as a stationary phase. The mobile phase was composed of a mixture of eluents using a gradient system, started with a mixture of 90% H2O acidified with 0.1% acetic acid and 10% acetonitrile (ACN) up to 6 min, 6–7 min, 10–15% ACN, and 7–20 min 15% ACN with a flow rate of 1.0 mL min−1 and injection volume of 1 μL of each sample. Chromatographic profiles were recorded at 254 nm, 280 nm, 325 nm and 352 nm. Standard curves were obtained with 3 μL of stock solutions of authentic standards of caffeine and 5-CQA in methanol, at concentrations of 1, 5, 10, 20, 40, 60, 100, 120, 150, 200, 300, and 400 μg mL−1). The chromatographic profiles of the crude extracts, the ultraviolet spectra and commercial standards (Sigma) allowed identifying two metabolites present in the crude extracts as 5-CQA (tR ~3.84 min) and caffeine (tR ~5.23 min). Quantification of compounds was carried out by external standard method.
The experiment was arranged in a completely randomized design, 2 × 2 × 3 factorial (two atmospheric [CO2] concentrations—a[CO2] and e[CO2], two water regimes—well-watered and water-deficit and three sampling times—day 0, 20, and 40 after water withholding onset) with 5–6 replicates per treatment, each one represented by one plant per pot. The data obtained were submitted to analysis of variance (ANOVA) and the contrast between averages was further evaluated by Tukey's test at 5% of probability (P ≤ 0.05). Figures were created in R (version 4.0.2) and RStudio (version 1.3.959). Box and whiskers plots were prepared using ggplot2 package; boxes show medians and first and third quartiles (25th and 75th percentiles), and whiskers extend from the hinge to the largest or smallest value, no further than 1.5 times. The statistical analyses showed in the box plots were performed using SigmaPlot 12.0 (Systat Software, San Jose, USA). The multivariate approach by principal component analysis (PCA) was performed using the R FactoMineR and factoextra packages (Kassambara and Mundt, 2017; Husson et al., 2020) in order to verify the behavior of samples and variables. The decompose of additive time-series was performed in R (version 4.0.2) and RStudio (version 1.3.959) using the commands “ts()” and “decompose()” from the preinstalled stats package.
Soil moisture was measured every 10 days after water withdrawal imposition (Supplementary Figure 3). In the first 10 days, the water content in the substrate of the water-deficit (WD) pots did not change compared to the well-watered (WW) ones. After that, soil moisture progressively reduced from day 20 to 40 (~58 and 89%, respectively) regardless [CO2], compared to WW pots. The impact of WD on leaf water potential was observed only on day 40 after WD imposition, which decreased from −0.4 at WW conditions to −0.7 in a[CO2]+WD plants and −0.9 in e[CO2]+WD plants (Figure 1).
Figure 1. Leaf water potential of C. arabica plants exposed to ambient [CO2] under well-watered and water deficit (a[CO2]+WW and a[CO2]+WD, respectively) and elevated [CO2] under well-watered and water deficit (e[CO2]+WW and e[CO2]+WD, respectively) conditions on day 0, 20, and 40 after water deficit imposition in the OTC. Boxes represent the median and first and third quartiles, symbols represent individual samples (n = 6–5 biological replicates). Different capital letters represent significant differences between sampling day in the same water and [CO2] condition, whereas different lowercase letters represent significant differences between water and [CO2] conditions in the same sampling day according to Tukey's test at 5% of probability (P ≤ 0.05).
On day 0 (i.e., 30 days after e[CO2] acclimation and before water restriction), CO2 assimilation and intrinsic water use efficiency increased 3- and 3.9-fold, respectively, and remained higher in WW condition relative to a[CO2] plants (Figures 2A,D). Water deficit reduced these parameters in e[CO2]+WD plants on day 20 and 40 after the stress onset, relative to e[CO2]+WW, but they were still higher than a[CO2]+WD, especially on day 20 (Figures 2A,D). Stomatal conductance and transpiration rate were affected by WD only on day 40 after stress imposition irrespective [CO2], which reduced by around 60 and 51%, respectively, compared to WW plants (Figures 2B,C). The gas exchange measurements of a[CO2] plants was not affected by WD relative to WW plants (Figure 2). The stomatal conductance of all plants on day 20 was higher than day 0 and 40, probably as a consequence of lower temperature and higher air relative humidity on this day (Supplementary Figures 1, 2). The intercellular CO2 partial pressure (Ci) increased over the experiment in e[CO2]-enriched plants when compared to a[CO2] ones and it was not affected by WD (Supplementary Figure 4).
Figure 2. Gas exchange of C. arabica plants exposed to ambient [CO2] under well-watered and water deficit (a[CO2]+WW and a[CO2]+WD, respectively) and elevated [CO2] under well-watered and water deficit (e[CO2]+WW and e[CO2]+WD, respectively) conditions on day 0, 20, and 40 after water deficit imposition in the OTC. (A) Net CO2 assimilation, (B) Stomatal conductance, (C) Transpiration rate, and (D) Intrinsic water use efficiency. Boxes represent the median and first and third quartiles, symbols represent individual samples (n = 6–5 biological replicates). Different capital letters represent significant differences between sampling day in the same water and [CO2] condition, whereas different lowercase letters represent significant differences between water and [CO2] conditions in the same sampling day according to Tukey's test at 5% of probability (P ≤ 0.05).
The chlorophyll a minimum and maximum fluorescence decreased similarly in plants acclimated to e[CO2] on day 0 of water shortage, mainly under WD, irrespective to [CO2], when compared to a[CO2]+WW plants (Figures 3A,B). On day 20, the levels of these parameters in all treatments returned to the levels found in a[CO2]+WW on day 0, but decreased equally in all treatments on day 40, possibly as an effect of the environmental condition variations (Figures 3A,B; Supplementary Figures 1, 2). Despite no statistical difference between treatments, on day 40 it was observed that F0 was quite variable in e[CO2]+WD plants. The maximum quantum efficiency of PSII did not change in the first two sampling times and slightly decreased (18%) in e[CO2]+WD plants compared to and e[CO2]+WW plants (Figure 3C).
Figure 3. Photochemical parameters of C. arabica plants exposed to ambient [CO2] under well-watered and water deficit (a[CO2]+WW and a[CO2]+WD, respectively) and elevated [CO2] under well-watered and water deficit (e[CO2]+WW and e[CO2]+WD, respectively) conditions on day 0, 20, and 40 after water deficit imposition in the OTC. (A) Chlorophyll a minimum fluorescence, (B) Maximum fluorescence, and (C) Maximum quantum efficiency of PSII. Boxes represent the median and first and third quartiles, symbols represent individual samples (n = 6–5 biological replicates). Different capital letters represent significant differences between sampling day in the same water and [CO2] condition, whereas different lowercase letters represent significant differences between water and [CO2] conditions in the same sampling day according to Tukey's test at 5% of probability (P ≤ 0.05).
The concentration of total soluble amino acids was variable and did not change between treatments over the experimental period, despite the tendency to increase in e[CO2]+WD plants on day 20 and 40 (Figure 4A). The amount of total phenolic compounds (TPC) increased over time in a[CO2]+WW plants (Figure 4B). While e[CO2] did not change the concentration of TPC on day 0, it was equally decreased by e[CO2] and water deficit on day 20, when compared to a[CO2]+WW plants (Figure 4B). On day 40, this response remained, especially in e[CO2] plants, regarding a[CO2]+WW plants (Figure 4B).
Figure 4. (A) Content of total soluble amino acids and (B) Total phenolic compounds in leaves of C. arabica plants exposed to ambient [CO2] under well-watered and water deficit (a[CO2]+WW and a[CO2]+WD, respectively) and elevated [CO2] under well-watered and water deficit (e[CO2]+WW and e[CO2]+WD, respectively) conditions on day 0, 20, and 40 after water deficit imposition in the OTC. Boxes represent the median and first and third quartiles, symbols represent individual samples (n = 6–5 biological replicates). Different capital letters represent significant differences between sampling day in the same water and [CO2] condition, whereas different lowercase letters represent significant differences between water and [CO2] conditions in the same sampling day according to Tukey's test at 5% of probability (P ≤ 0.05).
Elevated [CO2] did not change the amount of 5-O-caffeoylquinic acid (5-CQA) and caffeine on day 0 and 20, despite the large variation between treatments (Figure 5). On day 40, the combination of e[CO2] and WD increased the concentration of 5-CQA, when compared to e[CO2]+WW and a[CO2]+WD plants (Figure 5A). Moreover, e[CO2] and WD treatment stimulated the accumulation of caffeine regarding e[CO2]+WW plants, but it was similar to a[CO2] plants irrespective of water regime, on day 40 (Figure 5B).
Figure 5. (A) Content of 5-O-caffeoylquinic acid and (B) Caffeine in leaves of C. arabica plants exposed to ambient [CO2] under well-watered and water deficit (a[CO2]+WW and a[CO2]+WD, respectively) and elevated [CO2] under well-watered and water deficit (e[CO2]+WW and e[CO2]+WD, respectively) conditions on day 0, 20, and 40 after water deficit imposition in the OTC. Boxes represent the median and first and third quartiles, symbols represent individual samples (n = 6–5 biological replicates). Different capital letters represent significant differences between sampling day in the same water and [CO2] condition, whereas different lowercase letters represent significant differences between water and [CO2] conditions in the same sampling day according to Tukey's test at 5% of probability (P ≤ 0.05).
A multivariate approach was performed using PCA analysis aiming to better understand the data set variation. Figure 6 shows the distribution of variables determined in this study, including all treatments and sampling times. This analysis indicates that leaf water potential, Fm, soil moisture, intrinsic water use efficiency, stomatal conductance and transpiration rate were the main relevant variables for principal component 1 and 2 (Supplementary Figure 5). Additionally, 5-CQA and caffeine showed a distinct behavior when compared with other physiological and biochemical variables, such as photosynthetic rate and chlorophyll a fluorescence, and total soluble amino acids (Figure 6).
Figure 6. Principal component analysis of physiological and biochemical variables of C. arabica plants exposed to ambient [CO2] under well-watered and water deficit (a[CO2]+WW and a[CO2]+WD, respectively) and elevated [CO2] under well-watered and water deficit (e[CO2]+WW and e[CO2]+WD, respectively) conditions on day 0, 20, and 40 after water deficit imposition in the OTC. Numbers in parentheses give the per cent variation explained by the first and second principal component. Variables abbreviation: CQA, 5-CQA; Phenol, Total phenolic compounds; WPot, Leaf water potential; Fv.Fm, Fv/Fm; SoilHm, Soil moisture.
The same analysis was carried out in each sampling point to verify the distribution of treatments and variables (Figure 7). On day 0 and 20, there was no clear distribution pattern between treatments, but samples from e[CO2] tend to behave differently from a[CO2] samples (Figures 7A,B). On day 40, plants under water deficit were separated from well-watered plants (Figure 7C). Despite no clear separation between a[CO2]+WD and e[CO2]+WD groups was found, there was a tendency of different distribution supported by a similar behave of 5-CQA and caffeine in e[CO2]+WD plants, whereas amino acids content seems to be important to a[CO2]+WD plants (Figure 7C).
Figure 7. Principal component analysis of physiological and biochemical variables and treatments of C. arabica plants exposed to ambient [CO2] under well-watered and water deficit (a[CO2]+WW and a[CO2]+WD, respectively) and elevated [CO2] under well-watered and water deficit (e[CO2]+WW and e[CO2]+WD, respectively) conditions on day (A) 0, (B) 20, and (C) 40 after water deficit imposition in the OTC. Numbers in parentheses give the per cent variation explained by the first and second principal component. The color and distribution of each variable depending on their contribution to each principal component. Variables abbreviation: CQA, 5-CQA; Phenol, Total phenolic compounds; WPot, Leaf water potential; Fv.Fm, Fv/Fm; SoilHm, Soil moisture.
Coffee plants cultivated under natural conditions are constantly challenged by abiotic stresses (DaMatta and Ramalho, 2006; DaMatta et al., 2018; Sanches et al., 2020). However, how the predictable environmental conditions related to high [CO2] and the frequent drought events will affect coffee plants metabolism are not fully understood. In this study, we assessed whether elevated [CO2] will ameliorate drought stress and how the combination of these environmental conditions will affect the metabolism of specialized metabolites in young C. arabica plants. The effects of water deficit in plants will depend on the stress level (duration, intensity, and frequency), other environmental conditions (temperature, relative humidity and vapor pressure deficit) and interaction with other stresses, soil characteristics, plant stage and ability to tolerate the stress (Seleiman et al., 2021). In this study, water deficit drastically decreased soil moisture, but leaf water potential decreased only to about −1 MPa after 40 days of water withdrawal (Supplementary Figure 3; Figure 1). This water potential level represents mild to moderate drought stress to coffee, which has a narrow stomatal conductance and an efficient water status control (Martins et al., 2019; Semedo et al., 2021). Despite the very dry soil condition, the environmental conditions related to air temperature and relative humidity and the light intensity inside the OTCs were optimum to coffee plants and did not aggravate the stress. Indeed, this stress condition slightly affected gas exchange with no severe impacts on the PSII apparatus (Figures 2, 3).
Furthermore, elevated [CO2] stimulated CO2 assimilation, as a consequence of higher Ci (Supplementary Figure 4), and water use efficiency with no influence on stomatal conductance and transpiration rate, as reported by previous works (DaMatta et al., 2016; Sanches et al., 2017; Avila et al., 2020a). This response supports the hypothesis that the current atmospheric [CO2] is limiting for coffee plants photosynthesis since they have very low stomata aperture and insensitivity to [CO2] (DaMatta et al., 2016; Rodrigues et al., 2016). The gas exchange performance of plants exposed to e[CO2] and WD were not significantly different from plants exposed to ambient [CO2] and WD. However, higher medians were noted for these parameters on plants subjected to e[CO2] than a[CO2] under drought. These results suggest that coffee plants, even during the early growth stages, have an efficient water status control to keep a basal photosynthetic rate and when combined with drought, e[CO2] has the potential to buffer the negative effect of this stress on gas exchange.
Recently, it was demonstrated that coffee plants fertilized with e[CO2] have higher photosynthesis than ambient [CO2] under water deficit conditions in both greenhouse or field environments (Sanches et al., 2017, 2020). In this short-term experiment, considering the mild water deficit reached after 40 days in the WD plants (−1.0 MPa), the same pattern in gas exchange of coffee plants was observed. To better understand this behavior, we are carrying additional experiments subjecting the plants to a more intense water deficit associated with transcriptomic and phenotypic analysis. This approach aims to understand the implications of the intensity and duration of drought on the positive effect of e[CO2] on plant photosynthesis, carbon metabolism and ultimately on coffee plant growth.
The influence of e[CO2] in the presence or not of water deficit on PSII integrity was little (Figure 3). The slight decrease in Fv/Fm of e[CO2]+WD plants on day 40 could be assigned to the variations of F0, which was not statistically different from the other treatments. This response suggests that young coffee plants have an efficient capacity to dissipate excess energy in response to the imposed stress. Similar results were observed previously (Cavatte et al., 2012; Peloso et al., 2017; Semedo et al., 2021), where coffee plants submitted to different levels of water deficit did not show major changes in Fv/Fm, indicating that coffee plants exposed to that drought conditions may not have undergone photoinhibition. In fact, coffee plants seem to have an efficient protective thermal dissipation by activating the non-photochemical quenching, and a ROS scavenging system in the thylakoid membranes (Fortunato et al., 2010; Pompelli et al., 2010; Semedo et al., 2021). Hence, as it was observed in this study, a slight effect can be expected in the PSII activity of coffee plants exposed to mild water deficit and sub-saturating light conditions.
This study shows that e[CO2] probably increase the availability of CO2 next to Rubisco active sites, stimulating photosynthesis and buffer the negative effects of drought by increasing water use efficiency, in accordance with previous works (Avila et al., 2020b; Semedo et al., 2021). As mentioned above, C. arabica plants are drought resistant due to their low stomatal aperture and efficient water status control. This later can be improved by e[CO2], since it was observed that e[CO2]-fertilized plants kept hydraulic conductance and higher expression of aquaporin genes (Avila et al., 2020a). Moreover, we observed here that water deficit decreased the beneficial effect of e[CO2] in the gas exchange plant performance, which can be possibly related to biochemical limitations once stomatal and photochemical restrictions did not happen.
Our results also showed no statistically significant difference in total soluble amino acids (TSA) between a[CO2] and e[CO2] plants, but these later presented higher medians mainly under water deficit conditions (Figure 4). Therefore, it is possible to infer that the synthesis of TSA may not be the main sink of photoassimilates in e[CO2]-fertilized plants of C. arabica. Moreover, other solutes besides TSA may contribute to the osmotic adjustment of these plants under drought (Paixão et al., 2014; Avila et al., 2020a; Sanches et al., 2020).
In coffee, specialized metabolites are diverse and their amount varies according to plant development stage and tissue. These compounds are classified as specialized metabolites and act in many metabolic pathways. Besides, the role of these compounds is mainly related to antioxidant and plant defense responses, important under biotic and abiotic stresses (Baumann, 2006; Naikoo et al., 2019). The effect of elevated [CO2] under well-watered and water-deficit conditions on the metabolism of the three major specialized metabolites in coffee was investigated here (Figures 4, 5). The concentration of phenolic compounds increased over time in plants under normal conditions (a[CO2]+WW), corroborating with previous studies that showed higher accumulation of these compounds in older leaves of C. arabica plants (Kristiningrum et al., 2016). In plants subjected to e[CO2], despite water availability, the amount of TPC (total phenolic compounds) did not undergo major changes over time, but it was lower than a[CO2] plants on day 40. It suggests that the carbon skeletons synthesized by the higher photosynthetic rates under e[CO2] were probably mobilized to sustain other pathways, such as carbohydrate and amino acid synthesis and plant growth, as it was already demonstrated in coffee plants (Sanches et al., 2017; Avila et al., 2020b).
In fact, in an early development stage, rapid tissue growth is supported by a high synthesis/degradation of primary metabolites (Aharoni and Galili, 2011). However, the production and accumulation of specialized compounds are also noticed, especially in young leaves and beans from the upper layers, which are more exposed to intense light (Mondolot et al., 2006; Rakocevic et al., 2021). This response seems to be stimulated by e[CO2] exposure and it is species-specific (Sallas et al., 2001, 2003; Matros et al., 2006; Rakocevic et al., 2021). In needles scots pine seedling, e[CO2] did not affect TPC whereas in needles of Norway spruce seedlings it was reduced by e[CO2] (Sallas et al., 2003). The authors related that this response may be involved with the higher growth rates in Norway spruce needles. Moreover, it was also demonstrated that e[CO2] trend to increase specialized metabolites, but it can be restricted to particular compounds depend on plant species (Peñuelas and Estiarte, 1998; Sallas et al., 2003). Bearing in mind the growth stage and treatments imposed in this study, it is worth highlighting the tendency of increasing the concentration of 5-CQA throughout the experiment, especially in e[CO2]+WD treatment. In that case, it can be proposed that the fertilizing effect of the additional [CO2] observed in photosynthesis, even under WD, may favor vegetative growth and explain the gradual increase in 5-CQA levels under these conditions. In C. canephora plants, Mondolot et al. (2006) also observed an accumulation of 5-CQA, especially in young leaves.
A previous study showed that moderate drought alters caffeine content in C. canephora leaves (Kumar et al., 2015), which is decreased and the genes involved in its biosynthesis are possibly downregulated. On the other hand, a tendency toward an increase in the caffeine content in the treatment e[CO2]+WD, indicates a positive effect of e[CO2] on the concentrations of this compound in leaves. Recently, an experiment with arabica coffee trees subjected to four CO2 concentrations (300, 400, 500 and 600 ppm) under non-stressful conditions, showed that e[CO2] did not alter significantly the caffeine accumulation in leaves (Perrois et al., 2014).
In our study, the multivariate analysis indicate that specialized metabolites show distinct behavior comparing the physiological responses (Figure 6). As pointed earlier it might be associated with the use the additional carbon for specialized molecules synthesis. Surprisingly, after 40 days of treatments, the effect of water deficit appears as the main factor to distinguish the samples (Figure 7C), explaining one-third of the total variation (PC1). From these observations, we can conclude that the period of experiment was enough to allow the accumulation of the specialized metabolite by water deficit imposition but too short to allow the compensation of elevated [CO2], considering these variables. Further studies including a large-scale analysis of transcripts and metabolites, as well as more intense drought stress, will bring insightful contributions of future climatic scenarios in coffee production.
Collectively, our results demonstrated that e[CO2] had a positive effect on photosynthetic rates and intrinsic water use efficiency, buffering the extension of negative effects of mild drought stress. Moreover, water deficit and e[CO2] influenced the increased leaf concentration of 5-CQA and caffeine throughout the experimental period, which could play an important role in the vegetative phase of young coffee plants under this environmental condition. This work adds new insights about the short-term combination of e[CO2] and progressive water deficit affect the leaf concentration of these two specialized metabolites during the early growth stage of Coffea arabica plants. However, more studies are necessary especially involving long-term exposure to e[CO2] and drought and large scale analyses of transcripts and metabolomics to better understand the metabolism regulation and how coffee plants will cope with the predicted environmental scenario.
The original contributions presented in the study are included in the article/Supplementary Material, further inquiries can be directed to the corresponding author.
ES and LT designed the study. ES and DD supervised and acquired funds for the research. IC and GM conducted the experiments. MF and DC performed formal analysis. AL performed data curation. IC and AL wrote the manuscript with the contribution of all authors. All authors have read and agreed to the published version of the manuscript.
This research was funded by São Paulo State Research Support Foundation (Fundação de Amparo à Pesquisa do Estado de São Paulo—FAPESP), National Council for Scientifc and Technological Development (Conselho Nacional de Desenvolvimento Científico e Tecnológico—CNPq), and Coordination for the Improvement of Higher Education Personnel (Coordenação de Aperfeiçoamento de Pessoal de Nível Superior–CAPES). FAPESP grant number #2018/08042-8 and fellowship number #2019/26850-7 to AL. Research productivity fellowship from CNPq, number #312823/2019-3 and #308952/2020-0 to DD and MF, respectively. CAPES academic scholarship to IC.
The authors declare that the research was conducted in the absence of any commercial or financial relationships that could be construed as a potential conflict of interest.
All claims expressed in this article are solely those of the authors and do not necessarily represent those of their affiliated organizations, or those of the publisher, the editors and the reviewers. Any product that may be evaluated in this article, or claim that may be made by its manufacturer, is not guaranteed or endorsed by the publisher.
The authors were grateful to the Institute of Botany and its Post-Graduation Program in Plant Biodiversity and Environment for administrative and technical support and Mr. Paulo Venturini from Venturini Coffee Nursery for coffee seedlings donation.
The Supplementary Material for this article can be found online at: https://www.frontiersin.org/articles/10.3389/fsufs.2021.676207/full#supplementary-material
Abrahão, S. A., Pereira, R. G. F. A., Duarte, S. M., da, S., Lima, A. R., Alvarenga, D. J., et al. (2010). Compostos bioativos e atividade antioxidante do café (Coffea arabica L.). Ciênc. Agrotecnol. 34, 414–420. doi: 10.1590/S1413-70542010000200020
Aharoni, A., and Galili, G. (2011). Metabolic engineering of the plant primary-secondary metabolism interface. Curr. Opin. Biotechnol. 22, 239–244. doi: 10.1016/j.copbio.2010.11.004
Ainsworth, E. A., and Rogers, A. (2007). The response of photosynthesis and stomatal conductance to rising [CO2]: mechanisms and environmental interactions. Plant Cell Environ. 30, 258–270. doi: 10.1111/j.1365-3040.2007.01641.x
Ashihara, H. (2006). Metabolism of alkaloids in coffee plants. Brazilian J. Plant Physiol. 18, 1–8. doi: 10.1590/S1677-04202006000100001
Avila, R. T., Cardoso, A. A., de Almeida, W. L., Costa, L. C., Machado, K. L. G., Barbosa, M. L., et al. (2020a). Coffee plants respond to drought and elevated [CO2] through changes in stomatal function, plant hydraulic conductance, and aquaporin expression. Environ. Exp. Bot. 177:104148. doi: 10.1016/j.envexpbot.2020.104148
Avila, R. T., de Almeida, W. L., Costa, L. C., Machado, K. L. G., Barbosa, M. L., de Souza, R. P. B., et al. (2020b). Elevated air [CO2] improves photosynthetic performance and alters biomass accumulation and partitioning in drought-stressed coffee plants. Environ. Exp. Bot. 177:104137. doi: 10.1016/j.envexpbot.2020.104137
Baumann, T. W. (2006). Some thoughts on the physiology of caffeine in coffee: and a glimpse of metabolite profiling. Brazilian J. Plant Physiol. 18, 243–251. doi: 10.1590/S1677-04202006000100017
Campa, C., Rakotomalal, J.-J. R., de Kochko, A., and Hamon, S. (2008). Chlorogenic acids: diversity in green beans of wild coffee species. Adv. Plant Physiol. 10, 421–437.
Cavatte, P. C., Oliveira, Á. A. G., Morais, L. E., Martins, S. C. V., Sanglard, L. M. V. P., and DaMatta, F. M. (2012). Could shading reduce the negative impacts of drought on coffee? a morphophysiological analysis. Physiol. Plant. 144, 111–122. doi: 10.1111/j.1399-3054.2011.01525.x
Cheng, B., Furtado, A., Smyth, H. E., and Henry, R. J. (2016). Influence of genotype and environment on coffee quality. Trends Food Sci. Technol. 57, 20–30. doi: 10.1016/j.tifs.2016.09.003
Da Silva, C. J. R. S., Benjamim, C. J. R., Carvalho, L. B., Rocha, E. M. B., and Mori, E. (2018). Determinação do teor de cafeína em diferentes tipos de cafés. DEMETRA Aliment. Nutr. Saúde 13, 477–484. doi: 10.12957/demetra.2018.30653
DaMatta, F. M., Avila, R. T., Cardoso, A. A., Martins, S. C. V., and Ramalho, J. C. (2018). Physiological and agronomic performance of the coffee crop in the context of climate change and global warming: a review. J. Agric. Food Chem. 66, 5264–5274. doi: 10.1021/acs.jafc.7b04537
DaMatta, F. M., Godoy, A. G., Menezes-Silva, P. E., Martins, S. C. V., Sanglard, L. M. V. P., Morais, L. E., et al. (2016). Sustained enhancement of photosynthesis in coffee trees grown under free-air CO2 enrichment conditions: disentangling the contributions of stomatal, mesophyll, and biochemical limitations. J. Exp. Bot. 67, 341–352. doi: 10.1093/jxb/erv463
DaMatta, F. M., and Ramalho, J. D. C. (2006). Impacts of drought and temperature stress on coffee. Braz. J. Plant Physiol. 18, 55–81. doi: 10.1590/S1677-04202006000100006
Fortunato, A., Lidon, F. C., Batista-Santos, P., Leitão, A. E., Pais, I. P., Ribeiro, A. I., et al. (2010). Biochemical and molecular characterization of the antioxidative system of Coffea spp. under cold conditions in genotypes with contrasting tolerance. J. Plant Physiol. 167, 333–342. doi: 10.1016/j.jplph.2009.10.013
Frischknecht, P. M., Ulmer-Dufek, J., and Baumann, T. W. (1986). Purine alkaloid formation in buds and developing leaflets of Coffea arabica: expression of an optimal defence strategy? Phytochemistry 25, 613–616. doi: 10.1016/0031-9422(86)88009-8
Gamage, D., Thompson, M., Sutherland, M., Hirotsu, N., Makino, A., and Seneweera, S. (2018). New insights into the cellular mechanisms of plant growth at elevated atmospheric carbon dioxide concentrations. Plant Cell Environ. 41, 1233–1246. doi: 10.1111/pce.13206
Gebeyehu, B. T., and Bikila, S. L. (2015). Determination of caffeine content andantioxidant activity of coffee. Am. J. Appl. Chem. 3, 69–76. doi: 10.11648/j.ajac.20150302.16
Hoagland, D. R., and Arnon, D. I. (1950). The water-culture method for growing plants without soil. Calif. Agric. Exp. Stn. Circ. 347, 1–32.
Husson, F., Josse, J., Le, S., and Mazet, J. (2020). Package “FactoMineR”. Multivariate exploratory data analysis and data mining. Available online at: https://rdrr.io/cran/FactoMineR/ (accessed March 01, 2021).
IPCC (2014). Contribuição do Grupo de Trabalho II para o Quinto Relatório de Avaliação do Painel Intergovernamental sobre Alterações Climáticas. Geneva: IPCC.
Kassambara, A., and Mundt, F. (2017). Package “factoextra.” Extract and visualize the results of multivariate data analyses. Available online at: https://rpkgs.datanovia.com/factoextra/ (accessed March 01, 2021).
Kristiningrum, N., Cahyanti, Y. N., and Wulandari, L. (2016). “Determination of total phenolic content and antioxidant activity in methanolic extract of robusta and aarabica coffee leaves,” in Proceeding ICHMHS, University of Jember, Jember, Indonesia. 96–99.
Kumar, A., Naik, G. K., Simmi, P. S., and Giridhar, P. (2015). Salinity and drought response alleviate caffeine content of young leaves of Coffea canephora var. Robusta cv. S274. J. Appl. Biol. Biotechnol. 3, 50–60. doi: 10.7324/JABB.2015.3310
Leitão, S., Guerra-Guimarães, L., Bronze, M. R., Vilas Boas, L., Sá, M., Almeida, M., et al. (2008). “Chlorogenic acid content in coffee leaves : possible role in coffee leaf rust resistance,” in ASIC (Bali), 743–747.
Marcheafave, G. G., Pauli, E. D., Tormena, C. D., Ortiz, M. C. V., de Almeida, A. G., Rakocevic, M., et al. (2020). Factorial design fingerprint discrimination of Coffea arabica beans under elevated carbon dioxide and limited water conditions. Talanta 209:120591. doi: 10.1016/j.talanta.2019.120591
Marinova, D., Ribarova, F., and Atanassova, M. (2005). Total phenolics and total flavonoids in bulgarian fruits and vegetables. J. Univ. Chem. Technol. Metallurgy 40, 255–260.
Martins, S. C. V., Sanglard, M. L., Morais, L. E., Menezes-Silva, P. E., Mauri, R., Avila, R. T., et al. (2019). How do coffee trees deal with severe natural droughts? An analysis of hydraulic, diffusive and biochemical components at the leaf level. Trees Struct. Funct. 33, 1679–1693. doi: 10.1007/s00468-019-01889-4
Matros, A., Amme, S., Kettig, B., Buck-Sorlin, G. H., Sonnewald, U., and Mock, H. P. (2006). Growth at elevated CO2 concentrations leads to modified profiles of secondary metabolites in tobacco cv. SamsunNN and to increased resistance against infection with potato virus Y. Plant Cell Environ. 29, 126–137. doi: 10.1111/j.1365-3040.2005.01406.x
McDowell, N., Pockman, W. T., Allen, C. D., Breshears, D. D., Cobb, N., Kolb, T., et al. (2008). Mechanisms of plant survival and mortality during drought: why do some plants survive while others succumb to drought? New Phytol. 178, 719–739. doi: 10.1111/j.1469-8137.2008.02436.x
Mondolot, L., La Fisca, P., Buatois, B., Talansier, E., De Kochko, A., and Campa, C. (2006). Evolution in caffeoylquinic acid content and histolocalization during Coffea canephora leaf development. Ann. Bot. 98, 33–40. doi: 10.1093/aob/mcl080
Monteiro, M. C., and Farah, A. (2012). Chlorogenic acids in Brazilian Coffea arabica cultivars from various consecutive crops. Food Chem. 134, 611–614. doi: 10.1016/j.foodchem.2012.02.118
Naikoo, M. I., Dar, M. I., Raghib, F., Jaleel, H., Ahmad, B., Raina, A., et al. (2019). Role and regulation of plants phenolics in abiotic stress tolerance: an overview. Plant Signal. Mol. 2019, 157–168. doi: 10.1016/B978-0-12-816451-8.00009-5
NOAA (2020). National Oceanic and Atmospheric Administration. Silver Spring, MD: National Oceanic and Atmospheric Administration. Available online at: https://research.noaa.gov/article/ArtMID/587/ArticleID/2759/NOAA-index-tracks-how-greenhouse-gas-pollution-amplified-global-warming-in-2020
Paixão, C. L., Jesus, D. S., Costa, D. P., Pereira, P. P. A., and Neto, A. D. A. (2014). Caracterização fisiológica e bioquímica de genótipos de girassol com tolerância diferenciada ao estresse hídrico. Enciclopédia Biosf. 10, 2011–2022.
Peloso, A., de, F., Tatagiba, S. D., dos Reis, E. F., Pezzopane, J. E. M., and do Amaral, J. F. T. (2017). Photosynthetic limitations in leaves of arabic coffee promoted by the water deficit. Coffee Sci. 12, 389–399. doi: 10.25186/cs.v12i3.1314
Peñuelas J. and Estiarte, M. (1998). Can elevated CO2 affect secondary metabolism and ecosystem function? Trends Ecol. Evol. 13, 20–24. doi: 10.1016/S0169-5347(97)01235-4
Perrois, C., Strickler, S. R., Mathieu, G., Lepelley, M., Bedon, L., Michaux, S., et al. (2014). Differential regulation of caffeine metabolism in Coffea arabica (Arabica) and Coffea canephora (Robusta). Planta 241, 179–191. doi: 10.1007/s00425-014-2170-7
Pompelli, M. F., Martins, S. C. V., Antunes, W. C., Chaves, A. R. M., and DaMatta, F. M. (2010). Photosynthesis and photoprotection in coffee leaves is affected by nitrogen and light availabilities in winter conditions. J. Plant Physiol. 167, 1052–1060. doi: 10.1016/j.jplph.2010.03.001
Rakocevic, M., Batista, E. R., Pazianotto, R. A. A., Scholz, M. B. S., Souza, G. A. R., Campostrini, E., et al. (2021). Leaf gas exchange and bean quality fluctuations over the whole canopy vertical profile of Arabic coffee cultivated under elevated CO2. Funct. Plant Biol. 48, 469–482. doi: 10.1071/FP20298
Ramakrishna, A., and Ravishankar, G. A. (2011). Influence of abiotic stress signals on secondary metabolites in plants. Plant Signal. Behav. 6, 1720–1731. doi: 10.4161/psb.6.11.17613
Ramalho, J. C., Rodrigues, A. P., Semedo, J. N., Pais, I. P., Martins, L. D., Simões-Costa, M. C., et al. (2013). Sustained photosynthetic performance of Coffea spp. under long-term enhanced [CO2]. PLoS ONE 8:e82712. doi: 10.1371/journal.pone.0082712
Rodrigues, W. P., Martins, M. Q., Fortunato, A. S., Rodrigues, A. P., Semedo, J. N., Simões-Costa, M. C., et al. (2016). Long-term elevated air [CO2] strengthens photosynthetic functioning and mitigates the impact of supra-optimal temperatures in tropical Coffea arabica and C. canephora species. Glob. Change Biol. 22, 415–431. doi: 10.1111/gcb.13088
Sallas, L., Kainulainen, P., Utriainen, J., Holopainen, T., and Holopainen, J. K. (2001). The influence of elevated O3 and CO2 concentrations on secondary metabolites of Scots pine (Pinus sylvestris L.) seedlings. Glob. Change Biol. 7, 303–311. doi: 10.1046/j.1365-2486.2001.00408.x
Sallas, L., Luomala, E. M., Utriainen, J., Kainulainen, P., and Holopainen, J. K. (2003). Contrasting effects of elevated carbon dioxide concentration and temperature on Rubisco activity, chlorophyll fluorescence, needle ultrastructure, and secondary metabolites in conifer seedlings. Tree Physiol. 23, 97–108. doi: 10.1093/treephys/23.2.97
Sanches, R. F. E., Catarino, I. C. A., Braga, M. R., and Silva, E. A. (2017). Influência da alta concentração atmosférica de CO2 (↑[CO2]atm) × disponibilidade hídrica nas relações hídricas, trocas gasosas e acúmulo de carboidratos em Coffea arabica L. Hoehnea 44, 635–643. doi: 10.1590/2236-8906-33/2017
Sanches, R. F. E., Centeno, D. C., Braga, M. R., and Silva, E. A. (2020). Impact of high atmospheric CO2 concentrations on the seasonality of water-related processes, gas exchange, and carbohydrate metabolism in coffee trees under field conditions. Clim. Change 162, 1231–1248. doi: 10.1007/s10584-020-02741-2
Schreiber, U. (2004). “Pulse-amplitude- modulation (PAM) fluorometry and saturation pulse method: an overview,” in Chlorophyll a Fluorescence: A Signature of Photosynthesis. ADVANCES in Photosynthesis and Respiration, eds G. C. Papageorgiou and Govindjee (Berlin: Springer), 279–319.
Seleiman, M. F., Al-Suhaibani, N., Ali, N., Akmal, M., Alotaibi, M., Refay, Y., et al. (2021). Drought stress impacts on plants and different approaches to alleviate its adverse effects. Plants 10, 1–25. doi: 10.3390/plants10020259
Semedo, J. N., Rodrigues, A. P., Lidon, F. C., Pais, I. P., Marques, I., Gouveia, D., et al. (2021). Intrinsic non-stomatal resilience to drought of the photosynthetic apparatus in Coffea spp. is strengthened by elevated air [CO2]. Tree Physiol. 41, 708–727. doi: 10.1093/treephys/tpaa158
Singleton, V., and Rossi, J. (1965). Colorimetry of total phenolics with phosphomolybdic-phosphotungstic acid reagents. Am. J. Enol. Vitic. 16, 144–158.
Sobuj, N., Virjamo, V., Zhang, Y., Nybakken, L., and Julkunen-Tiitto, R. (2018). Impacts of elevated temperature and CO2 concentration on growth and phenolics in the sexually dimorphic Populus tremula (L.). Environ. Exp. Bot. 146, 34–44. doi: 10.1016/j.envexpbot.2017.08.003
Sunarharum, W. B., Williams, D. J., and Smyth, H. E. (2014). Complexity of coffee flavor: a compositional and sensory perspective. Food Res. Int. 62, 315–325. doi: 10.1016/j.foodres.2014.02.030
Tamayose, C. I., Santos, E. A., Roque, N., Costa-Lotufo, L. V., and Ferreira, M. J. P. (2019). Caffeoylquinic acids: separation method, antiradical properties and cytotoxicity. Chem. Biodivers. 16:e1900093. doi: 10.1002/cbdv.201900093
Vega, F. E., Ziska, L. H., Simpkins, A., Infante, F., Davis, A. P., Rivera, J. A., et al. (2020). Early growth phase and caffeine content response to recent and projected increases in atmospheric carbon dioxide in coffee (Coffea arabica and C. canephora). Sci. Rep. 10, 1–11. doi: 10.1038/s41598-020-62818-x
Xu, Z., Jiang, Y., and Zhou, G. (2015). Response and adaptation of photosynthesis, respiration, and antioxidant systems to elevated CO2 with environmental stress in plants. Front. Plant Sci. 6:701. doi: 10.3389/fpls.2015.00701
Keywords: climate change, water deficit, photosynthesis, specialized metabolites, coffee
Citation: Catarino ICA, Monteiro GB, Ferreira MJP, Torres LMB, Domingues DS, Centeno DC, Lobo AKM and Silva EA (2021) Elevated [CO2] Mitigates Drought Effects and Increases Leaf 5-O-Caffeoylquinic Acid and Caffeine Concentrations During the Early Growth of Coffea Arabica Plants. Front. Sustain. Food Syst. 5:676207. doi: 10.3389/fsufs.2021.676207
Received: 04 March 2021; Accepted: 30 June 2021;
Published: 28 July 2021.
Edited by:
Herminia Prieto Martinez, Universidade Federal de Viçosa, BrazilReviewed by:
Vuk M. Maksimović, University of Belgrade, SerbiaCopyright © 2021 Catarino, Monteiro, Ferreira, Torres, Domingues, Centeno, Lobo and Silva. This is an open-access article distributed under the terms of the Creative Commons Attribution License (CC BY). The use, distribution or reproduction in other forums is permitted, provided the original author(s) and the copyright owner(s) are credited and that the original publication in this journal is cited, in accordance with accepted academic practice. No use, distribution or reproduction is permitted which does not comply with these terms.
*Correspondence: Emerson A. Silva, ZWFzaWx2YUBpYm90LnNwLmdvdi5icg==
Disclaimer: All claims expressed in this article are solely those of the authors and do not necessarily represent those of their affiliated organizations, or those of the publisher, the editors and the reviewers. Any product that may be evaluated in this article or claim that may be made by its manufacturer is not guaranteed or endorsed by the publisher.
Research integrity at Frontiers
Learn more about the work of our research integrity team to safeguard the quality of each article we publish.