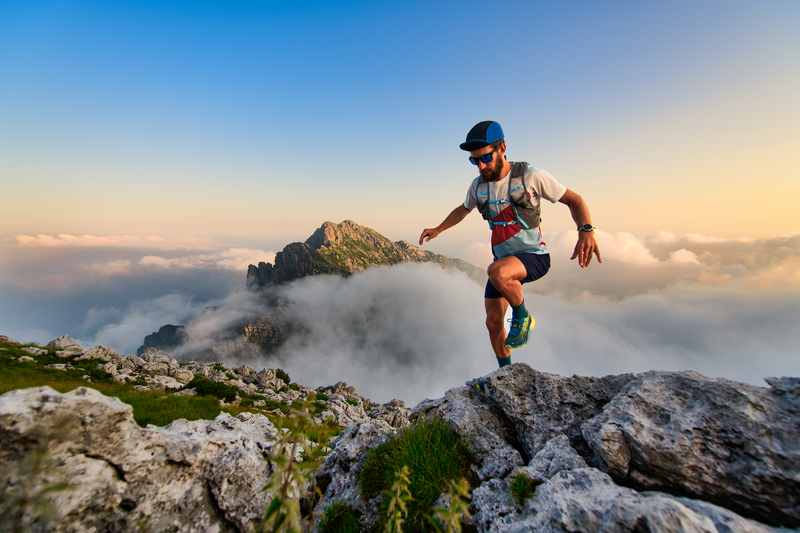
94% of researchers rate our articles as excellent or good
Learn more about the work of our research integrity team to safeguard the quality of each article we publish.
Find out more
REVIEW article
Front. Sustain. Food Syst. , 06 July 2021
Sec. Crop Biology and Sustainability
Volume 5 - 2021 | https://doi.org/10.3389/fsufs.2021.667546
This article is part of the Research Topic Increasing Resilience and Adaptability to Climate Change of Vulnerable Groups in Agriculture View all 12 articles
A correction has been applied to this article in:
Corrigendum: PGPR in agriculture: a sustainable approach to increasing climate change resilience
Growing environmental concerns are potentially narrowing global yield capacity of agricultural systems. Climate change is the most significant problem the world is currently facing. To meet global food demand, food production must be doubled by 2050; over exploitation of arable lands using unsustainable techniques might resolve food demand issues, but they have negative environmental effects. Current crop production systems are a major reason for changing global climate through diminishing biodiversity, physical and chemical soil degradation, and water pollution. The over application of fertilizers and pesticides contribute to climate change through greenhouse gas emissions (GHG) and toxic soil depositions. At this crucial time, there is a pressing need to transition to more sustainable crop production practices, ones that concentrate more on promoting sustainable mechanisms, which enable crops to grow well in resource limited and environmentally challenging environments, and also develop crops with greater resource use efficiency that have optimum sustainable yields across a wider array of environmental conditions. The phytomicrobiome is considered as one of the best strategies; a better alternative for sustainable agriculture, and a viable solution to meet the twin challenges of global food security and environmental stability. Use of the phytomicrobiome, due to its sustainable and environmentally friendly mechanisms of plant growth promotion, is becoming more widespread in the agricultural industry. Therefore, in this review, we emphasize the contribution of beneficial phytomicrobiome members, particularly plant growth promoting rhizobacteria (PGPR), as a strategy to sustainable improvement of plant growth and production in the face of climate change. Also, the roles of soil dwelling microbes in stress amelioration, nutrient supply (nitrogen fixation, phosphorus solubilization), and phytohormone production along with the factors that could potentially affect their efficiency have been discussed extensively. Lastly, limitations to expansion and use of biobased techniques, for instance, the perspective of crop producers, indigenous microbial competition and regulatory approval are discussed. This review largely focusses on the importance and need of sustainable and environmentally friendly approaches such as biobased/PGPR-based techniques in our agricultural systems, especially in the context of current climate change conditions, which are almost certain to worsen in near future.
To feed a dramatically growing world population, agricultural output must increase by 50% to sustain ~9 billion people by 2050 (Alexandratos and Bruinsma, 2012). However, as the intensification of food production increases, the over-application of chemical fertilizers (Canfield et al., 2010) and the exploitation of arable land (Pastor et al., 2019) contribute further to greenhouse gas (GHG) emissions (Smith et al., 2013) and climate change (Richardson et al., 2012). The agricultural output response to climate change, setting aside any possible compensation due to increasing CO2 levels, is 17% (Nelson et al., 2014). In addition to reducing crop productivity, climate change is also resulting in higher prices of agricultural products, increasing the risk of food insecurity for 77 million people by 2050 (Janssens et al., 2020). Climate change has caused significant yield losses to major cereal crops with about 3.8 and 5.5% yield reductions for maize and wheat, respectively (Lobell et al., 2011; Lipper et al., 2014). Climate change is rearing up, and with it, significant increases in global temperature, and occurrence of other abiotic stresses that are adversely affecting crop productivity. In such a situation, sustainable practices and the application of environmentally friendly technologies can help break this feedforward loop by improving resource use efficiency and increasing yield under a range of more extreme environmental conditions (Pareek et al., 2020), with the aim to improve healthy food production while reducing unsustainable inputs, thereby controlling extreme climatic conditions, and to improve soil health by sequestrating soil carbon, maintaining soil organic matter and inorganic nutrients (Drost et al., 2020). Some plants may grow reasonably well under more extreme growth conditions as they have evolved the plasticity to manage these variations. However, the productivity of most agricultural plants will decline, as more extreme environmental pressures will exceed their capacity to respond to stress. The rhizosphere, rhizoplane, and endosphere, the soil near the roots, the root surfaces, and the spaces between plant cells, respectively, are the plant-influenced areas with the greatest microbial diversity (Reinhold-Hurek et al., 2015); it affects plant growth and crop productivity, and has vital effects on carbon sequestration and the capacity for phytoremediation (Ojuederie and Babalola, 2017; Berlanas et al., 2019). Importantly, in the entire ecosystem, bacterial community composition is significantly co-related to soil properties; this is important as microbial abundance can mediate GHG emission (Ho et al., 2017). Moreover, the microbes living in rhizosphere contribute to efficient carbon cycling between the soil and the atmosphere and can reduce the loss of soil carbon through their metabolic activity (Bardgett et al., 2008). Host plants and soil properties, among other environmental conditions, have substantial influences on rhizosphere microbial diversity and abundance (Qiao et al., 2017). Importantly, it has been reported that a group of beneficial microbes, part of the phytomicrobiome, not only contribute to crop yield improvement but also enhance plant ability to resist biotic/abiotic stresses (Backer et al., 2018; Lyu et al., 2020). Beneficial phytomicrobiome members, including plant growth promotion rhizobacteria (PGPR), enhance plant growth by improving nutrient absorption, producing phytohormones, and releasing antibiotics to manage biotic stress (Lyu et al., 2020; Sindhu et al., 2020). The plant host and its associated phytomicrobiome are defined as the holobiont (Simon et al., 2019; Lyu et al., 2021); the unique opportunities that reside with plant-associated microbes have been recognized for several decades. More recently, the focus of this synergistic relationship has been shifting to the signal exchange aspect. Root exudates, including organic acids, sugar, vitamins, and other molecules, affect the abundance and behavior of plant-associated microbes (Huang et al., 2019). Reciprocally, the growth of host plants depend on microbe-to-plant signals (Ortíz-Castro et al., 2009). In this way, utilization of phytomicrobiomes (microbial inoculation, signal exogenous application) can be deployed to achieve the goal of establishing a more sustainable and resilient agricultural production system without additional chemical fertilization application.
This work aims to understand the microbe-microbe and plant-microbe interactions, including those mediated by signal exchange, and estimate the role of beneficial microbes, especially PGPR, as a sustainable approach to improving crop production. The need for development of a general formulation of this technology for global application against the challenges associated with climate change is also addressed.
The global food production gains in the 20th century after the green revolution was primarily based on two general areas of advance: chemical inputs (commercial fertilizers and pesticides) and genetic modifications through targeted breeding and gene manipulation. However, the continuous use of chemical fertilizers and pesticides, and their subsequent adverse effects on the environment have changed thinking around this. Scientists are approaching different techniques that could sustainably increase crop production including the utilization of phytomicrobiome members, which is now being recognized as a “fresh” green revolution (Lyu et al., 2020). The application of beneficial microbes on food crops has been studied extensively, however, their implementation in the field is very limited. The incorporation of phytomicrobiome members in agricultural systems as a sustainable approach for disease management and nutrient supplements could reduce the negative effects associated with the excess application of chemical inputs (fertilizers and pesticides) (Antar et al., 2021b). In addition, phytmicrobiome members have been employed as an effective strategy to mitigate certain biotic and abiotic stresses that could affect crop growth and production (Khan et al., 2020) (Figure 1).
Nitrogen (N) is one of the most important mineral nutrients for plants as it is an integral part of most of the plant physiological processes including photosynthesis and protein synthesis (Alori et al., 2017). Nitrogen, in the form of dinitrogen, makes up 79% of the earth's atmosphere, however, due to its triplet covalent bond, it has a very low level of reactivity, and can't be used directly by the plants. Nitrogen fertilizers, as the most efficient way of nitrogen supplement, have become a fundamental part of our crop production and agricultural systems, however, their continuous and ineffective excessive use is directly or indirectly contributing to the climate change by contaminating the environment through eutrophication, lethal emissions into the atmosphere or toxic deposition in ground water and other water bodies. It is estimated that only around 50% of added nitrogen is recovered by cropping systems (Bouchet et al., 2016), however, the remaining unavailable 50% stays in the soil as organic complexes (~98% of the total soil nitrogen) or escapes through volatilization, leaching and runoff. Although, CO2 is considered as the main culprit in climate change, nitrous oxide (N2O); being 265 times more effective at heat trapping than CO2 (Pep, 2019), is also a very important contributor. Therefore, alternatives should be considered that could sustainably increase nitrogen use efficiency (NUE) or at least reduce the fertilizer inputs for some extent. The members of phytomicrobiome, not all perhaps, have the capability to substantially reduce the need for soil nitrogen supplements either by fixing atmospheric nitrogen directly through legume-rhizobium interaction or indirectly, by assisting the nitrogen fixers through their secretions (Naamala and Smith, 2020). Nitrogen fixers are basically categorized into two major groups; the symbionts and the free-living nitrogen fixers, solely based on their type of association developed with plants. The symbiotic nitrogen fixers which include genera such as: Rhizobium, Sinorhizobium, Azoarcus, Mesorhizobium, Frankia, Allorhizobium, Bradyrhizobium, Burkholderia, Azorhizobium, and some Achromobacter strains (Babalola, 2010; Pérez-Montaño et al., 2014; Turan et al., 2016). The more notable bacterial genera of free living nitrogen fixers are: Azoarcus, Herbaspirillum., Gluconacetobacter, Azospirillum, and Azotobacter (Vessey, 2003).
These microorganisms utilize a substantial amount of energy to reduce the atmospheric nitrogen into available forms. For each mole of nitrogen fixed, 16 moles of ATP are required, and this energy primarily comes from oxidizing the organic molecules. To obtain these energy rich molecules, the non-photosynthetic nitrogen fixers completely rely on other organisms, while, photoautotrophic microorganism use sugars, produced by photosynthesis. In addition to the associated and symbiotic nitrogen fixing microorganisms; the most dominant and extensively studied group of nitrogen-fixing PGPMs, obtain these compounds from their host in exchange for the nitrogen fixed (Wagner, 2011). Total N2 fixation in the world is estimated to be ~175 Tg, of which symbiotic nitrogen fixation in legumes counts for ~80 Tg by fixing 20–200 kg N fixed ha−1 yr−1, and the other near half is industrially fixed while producing N fertilizers (~88 Tg) (Hillel, 2008). Symbiotic nitrogen fixation begins with the crosstalk between nitrogen-fixing bacteria (e.g., rhizobia) and the host plant (legume) in the form of signal compounds, which will eventually lead to the formation of specialized structures (root nodules) where atmospheric nitrogen is reduced into available forms (primarily NH3) (Naamala et al., 2016). More than 70 % of legumes develop symbiotic relationships with rhizobia, and fix up to 200 Kg N ha−1. Legumes usually don't require nitrogen supplements, as they don't respond to fertilizers as long as they are capable of fixing atmospheric nitrogen, through symbiotic relationships with microbes, except for extensive application of N-fertilizers, which cause them reduce or completely shut down their nitrogen fixation because exploitation of the supplied fertilizer requires less energy than fixation of N2 from the atmosphere. In addition to legumes, several studies of PGPRs have demonstrated that their application can reduce required application rates of chemical fertilizers to non-legumes. For example, PGPR inoculants on tomato coupled with application of 75% of the recommended N fertilizer rate, resulted in similar plant growth, yield and nutrient uptake, as compared to recommended fertilizer rate without PGPR inoculation, allowing a 25% reduction in chemical fertilizer supplementation. In addition, the co-inoculation of PGPR with AMF reduced the fertilizer input by 30% without any reduction in plant growth or yield (Adesemoye et al., 2009). Similarly, PGPR application with 80% of the recommended rates of nitrogen and phosphorus increased maize yield and biomass production by 11.7 and 17.9%, respectively, indicating a 20% reduction in fertilizer nitrogen and phosphorus input without hampering the growth and production of maize (Sood et al., 2018).
Phosphorus (P) is the second most highly required macronutrient required by plants, after nitrogen (Azziz et al., 2012; Tak et al., 2012). The total phosphorus content in soil has been reported to be in between 0.05 and 0.06%, but only 0.1% of that is available to plants because of its poor solubility, and its affinity with the soil matrix and organic complexes. Traditionally, to address phosphorus deficiencies, phosphorus-based fertilizers have been effectively adopted to recharge soil phosphorus, which is immediately available to plants. However, phosphorus supplementation through commercial fertilizers is an expensive approach, and the phosphorus often becomes unavailable to plants as it can readily be lost from the soil, and then can mix into local waterways and contaminate terrestrial and aquatic environments (Adesemoye and Kloepper, 2009).
Many beneficial microorganisms, including bacteria and fungi living in the soil, and those associated with plant roots are capable of solubilizing otherwise insoluble soil phosphorus (Bechtaoui et al., 2020). Phosphorus solubilizing bacteria (PSB) has been reported to reduce required P dosage by 25% (Sundara et al., 2002), and its influence increases when co-inoculated with other PGPR or AMF, as suggested by a 50% reduction in P supplementation (Khan et al., 2009). The principal mechanism followed by almost all phosphorus solubilizing microbes is to produce metabolites, mostly organic acids, in the form of gluconic and keto gluconic acids, which through their hydroxyl and carboxyl groups chelate the cations bound to phosphate (Bates and Lynch, 2001; Vassilev et al., 2006; Heydari et al., 2007), thereby solubilize the insoluble phosphorus into the soil solution, and make it accessible for plant uptake (Riaz et al., 2021). There is an array of phosphorus solubilizing bacteria that are capable of mobilizing forms of phosphorus, which are poorly accessible. These taxa include Bacillus circulans, Agrobacterium spp, Pseudomonas spp (Babalola and Glick, 2012), Bacillus (Raj et al., 2014), Rhizobium (Tajini et al., 2012), Paenibacillus (Bidondo et al., 2011), Burkholderia (Istina et al., 2015), Azotobacter (Kumar et al., 2014), Enterobacter, and Erwinia (Chakraborty et al., 2009). Similarly, the most efficient phosphate solubilizing fungi (PSF) are generally strains of Alternaria, Achrothcium, Aspergillus, Cephalosporium, Arthrobotrys, Curvularia, Cladosporium, Rhizopus, Chaetomium, Cunninghamella, Glomus, Helminthosporium, Fusarium, Micromonospora, Mortierella, Myrothecium, Penicillium, Phoma, Pythium, Pichia fermentans, Populospora, Rhizoctonia, Trichoderma, and many others (Srinivasan et al., 2012; Sharma et al., 2013).
Many microorganisms, especially fungal and bacterial species, are involved in mutual intimate relationships with plants and are able to solubilize potassium (K) in the soil (Gundala et al., 2013; Setiawati and Mutmainnah, 2016). The first study on potassium solubilization (Muentz, 1890) demonstrated the role of microorganisms in solubilizing potassium bearing rocks. A broad range of K-solubilizing microbes including, Bacillus edaphicus (Sheng and He, 2006), Bacillus megaterium, Arthrobacter sp. (Keshavarz Zarjani et al., 2013), and Paenibacillus glucanolyticus (Sangeeth et al., 2012) have been shown to release potassium from insoluble and fixed forms of K minerals by degrading silicate minerals. Studies carried out on the effects of plant growth promoting microbes (PGPM) on plant growth promotion revealed that the growth promotion was linked to increased potassium availability, related to secretion of organic acids by the K-solubilizing microorganisms (Badr et al., 2006; Sheng and He, 2006). Organic acids such as oxalate, citrate, acetate, ferulic acid and coumaric acid produced by microorganisms present in the soil increases the mineral dissolution rate and production of protons through acidification of the soil rhizosphere leading to the solubilization of mineral K (Prajapati and Modi, 2012; Setiawati and Mutmainnah, 2016). Thus, in order to achieve biological development for sustainable agriculture, researchers concluded that, the use of PGPM such as potassium solubilizing bacteria (PSB) can be a reliable biofertilizer, enhancing plant nutrient availability and so allowing reduced use of chemical fertilizers (Vessey, 2003; Archana et al., 2012; Prajapati et al., 2013).
Iron is a vital element for plants and other photosynthetic organisms since it plays a pivotal role as an enzymatic cofactor for various metabolic processes such as photosynthesis, amino acid synthesis, respiration, nitrogen fixation, and oxygen transport. Iron is one of the most abundant elements in the earth's crust; commonly exists in two oxidation states: Fe2+ and Fe3+; the later of which is much less accessible to the plants due to its formation of insoluble iron oxides/hydroxides (Zuo and Zhang, 2011). Studies have revealed that some plant growth promoting bacteria (PGPB) sequester iron from the soil by releasing low molecular weight compounds (400–1,500 Da). Such iron-chelating compounds, siderophores, are able to bind ferric ions and ultimately make iron readily available for uptake by plant cells (Dalcorso et al., 2013; Saha et al., 2013; Goswami et al., 2016). In addition, siderophores secreted by PGPB have a much higher affinity to sequester iron than those produced by fungi or the plant itself (Saha et al., 2016).
Many microorganisms have been isolated and screened to evaluate their ability to produce siderophores, from both marine and terrestrial ecosystems (Sandy and Butler, 2009; Rezanka et al., 2018). Over 500 terrestrial and marine siderophores, with different chemical structures, have been identified so far (Chu et al., 2010; Hider and Kong, 2010). These are classified into four main groups: phenolates, hydroxamates, pyoverdines and carboxylates (Daly et al., 2017). More than 90% of siderophore-producing bacterial isolates belong to the gram-negative bacteria; Enterobacter and Pseudomonas dominate; few gram-positive genera such as Bacillus and Rhodococcus are able to produce siderophores - <2% of the total (Tian et al., 2009). From a bioprospecting perspective, studies suggest that most rhizobacteria, screened either from soil or plant root tissues, have the capability to enhance plant growth through siderophore production if inoculated into iron deficient soils (Tian et al., 2009). Production of siderophores by beneficial soil/plant associated microbes is also an important mechanism in terms of biological control, by outcompeting plant pathogens for iron sources, resulting in restriction of iron availability to these deleterious plant pathogens (Shanmugaiah et al., 2015).
Zinc (Zn) is an essential plant micronutrient, crucial for plant growth and development, required in very minute concentrations ranging from 5 to 100 mg kg−1 (Goteti et al., 2013). Zn plays a pivotal role in plant growth as it is an essential component of key plant physiological processes including chlorophyl formation, and activation enzymes involved in auxin and carbohydrate metabolism, synthesis of proteins, lipids and nucleic acids (Krämer and Clemens, 2005), and in the context of developing climate scenarios, it helps the plants withstand more extreme environmental conditions, including drought and extremes of temperature (Umair Hassan et al., 2020). However, zinc in most of agricultural soils is either deficient or exists in fixed forms in the soil, making it unavailable to plants (Sadeghzadeh, 2013). As reported by the Food and Agricultural Organization (FAO), more than 50% of the soils around the world are zinc deficient (FAO, 2002), mainly due to Zn association with naturally occurring mineral forms such as zincite (ZnO), sphalerite (ZnFe), smithsonite (ZnCO3), zinc silicates (ZnSiO3), willemite (ZnSiO4), and zinc sulfide (ZnS) (Saravanan et al., 2011) which are generally unavailable for plant uptake.
One way to alleviate Zn deficiency is the application of inorganic fertilizers, although this comes with a degree of environmental damage and, as indicated, much of it becomes unavailable to plants. Perhaps a better strategy for overcoming this problem is using plant growth promoting rhizobacteria (PGPR), which are known for their role in solubilizing naturally occurring cation bearing minerals. PGPR, have been shown to solubilize unavailable forms of zinc through chelation, exchange reaction mechanisms, acidification and dissolution processes by secreting organic acids into the soil (Hussain et al., 2015). Subramanian et al. (2009) reported both bacteria and fungi to increase Zn nutrient availability in the rhizosphere by solubilizing the unavailable forms of zinc. A number of studies clearly demonstrate that inoculation of Zn mobilizing PGPR significantly increase yield of cereal crops including, but not limited to, maize (Goteti et al., 2013), wheat (Kutman et al., 2010; Ullah et al., 2015; Kamran et al., 2017), and rice (Tariq et al., 2007; Vaid et al., 2014).
As global food demand rises, due to increasing requirements for staple crops to feed the dramatically growing population, an increasing demand for pesticides and synthetic fertilizers is required to increase crop productivity, but this has the potential to lead to serious environmental problems. Although, we may not be able to substitute mineral fertilizers with biofertilizers at this time, we at least assure a significant reduction in chemical and unsustainable inputs by incorporating beneficial microbes into agricultural production, thereby contributing to climate change mitigation.
Root-associated microbes including symbiotic or endophytic bacteria play a huge role in the production of plant growth hormones (phytohormones) which influence seed germination, development of root systems for better nutrient uptake, development/elaboration of vascular tissue, shoot elongation, flowering and overall plant growth (Sgroy et al., 2009; Antar et al., 2021a). Several studies indicate the potential of enhanced plant stress tolerance and growth promotion through hormones. These include abscisic acid in corn (Sgroy et al., 2009), cytokinins in wheat (Kudoyarova et al., 2014), auxin in rice and Lavandula dentate (Pereira et al., 2016; Etesami and Beattie, 2017) and gibberellins in cucumber, tomato, young radish and rice (Kang et al., 2014). In plants, hormone levels can be modulated through microbe-produced plant growth regulators, which exert effects close to those of exogenous plant phytohormonal applications (Egamberdieva, 2009; Turan et al., 2014). Microbe-produced phytohormones such as auxins and cytokinins resemble plant-synthesized phytohormones and regulate plant hormone levels influencing photosynthetic processes to promote plant growth and development, and activates defense responses to pathogens (Backer et al., 2018).
Auxins are an important group of hormones for plant growth and development. Indole Acetic Acid (IAA) is the most commonly found and physiologically active phytohormone in plants, active in upregulating and downregulating gene expression. Shoot apical meristems of plants produce IAA in the form of free/diffusible auxins and can be found in almost all plant tissues (Maheshwari et al., 2015). It has been reported that more than 80% of rhizospheric bacteria are able to synthesize and release auxins. IAA production, which is common among rhizospheric bacteria, involves several biosynthesis pathways, and is carried out by a range of bacterial genera including Aeromonas, Azotbacter, Bacillus, Bradyrhizobium, Burkholderia, Enterobacter, Mesorhizobium, Pseudomonas, Rhizobium, and Sinorhizobium (Ahmad et al., 2008; Celloto et al., 2012; Sharma et al., 2016; Cakmakci et al., 2020). In some cases, a single bacterial strain produces IAA using more than one pathway. These biosynthesis pathways can be independent of, or dependent on, tryptophan, an important precursor molecule for IAA (Kashyap et al., 2019), with pathways sourcing from decomposed roots or exudates from bacterial cells (Spaepen et al., 2007; Egamberdieva et al., 2017).
The ability of rhizospheric beneficial bacteria to synthesize IAA under salinity stress conditions could play a crucial role in balancing and regulating IAA levels in the roots, leading to improved plant responses to salinity stress (Egamberdieva et al., 2015). It has recently been reported that microbe-produced IAA can enhance root and shoot biomass production under water deficit conditions (Kumar et al., 2019). In addition to IAA, various physiological processes regulating plant growth and development can be controlled through many PGPR-synthesized phytohormones such as indole lactic acid (ILA), indole-3-butyric acid (IBA), indole-3-propionic acid (IPA), indole-3-pyruvic acid (IPA), 2,4-dichlorophenoxy acetic acid (2,4-D) and 2-methyl-4-chlorophenoxy acetic acid (MCPA) and tryptophol (TOL) (Ijaz et al., 2019; Swarnalakshmi et al., 2020).
Cytokinins are another group of hormones influencing plant growth and development by regulating physiological processes involved in seed germination, cell division, apical dominance, root and shoot growth, flower and fruit production, leaf senescence, interactions of plants with pathogens, nutrient mobilization and assimilation (Egamberdieva et al., 2015; Akhtar et al., 2020). It has been reported that cytokinin alone or through its interactions other phytohormones, such as auxin and abscisic acid, could promote the growth of salt stressed plants, enhancing tolerance by altering gene expression (Kang et al., 2012; Kunikowska et al., 2013). Like auxins, PGPR such as Arthrobacter, Bacillus, Azospirillum and Pseudomonas have been reported to synthesize cytokinins, causing their positive impacts on root system. Cytokinin producing PGPR are not only important for promoting plant growth and development, but are effective biocontrol agents against various pathogens as well (Naz et al., 2009; Maheshwari et al., 2015). It is well-documented that plants and plant-associated microorganisms contain more than 30 growth-promoting cytokinin compounds released at various concentrations (Hayat et al., 2012; Amara et al., 2015).
In the past two decades, several studies have reported the effects of cytokinin producing PGPR on root system architecture, plant growth and tolerance to biotic and abiotic stresses including drought (Arkhipova et al., 2007; Dodd et al., 2010; Egamberdieva et al., 2015), salinity (Naz et al., 2009; Zhou et al., 2017; Cordero et al., 2018), bacterial pathogens (Naseem et al., 2014; Grosskinsky et al., 2016; Spallek et al., 2018; Dermastia, 2019), fungal pathogens (Mishra et al., 2018; Spallek et al., 2018; Vrabka et al., 2019) and insect pests (Giron and Glevarec, 2014; Brutting et al., 2018; Zhang et al., 2018).
As the climate change conditions continue to develop, more extreme environmental conditions are becoming more frequent, for example, drought, salinity, high and low temperatures, heavy metal toxicity, and nutrient deficiency, which can cause extensive annually reductions in overall crop production, yield and quality worldwide (Acquaah, 2009; Awasthi et al., 2014; Shrivastava and Kumar, 2015; Mishra et al., 2017). Climate change has aggravated the frequency and intensity of abiotic stresses, specifically drought and high temperature, causing remarkable losses in principal cereal species such as wheat, maize, and barely (Lobell and Field, 2007; Vogel et al., 2019). A recent heat wave and drought resulted in a reduced crop yield which caused a lack of fodder across the European countries (Mazumdaru, 2018). A co-occurrence of different abiotic stresses affecting crops in field environments is unfavorable for plant growth, development, and production (Mittler, 2006). High levels of soil salinity and drought, and their subsequent secondary effects including osmotic, oxidative and ionic stress, are considered to be major hindrances of agriculture output (Kaushal and Wani, 2016). When plants encounter stressful conditions, internal metabolism is disrupted by metabolic enzyme inhibition, substrate scarcity, excess demand for various compounds, or a combination of these factors. Hence, metabolic reconfiguration is obligatory to meet the requirements for anti-stress agents including compatible solutes, antioxidants, and proteins to resist unfavorable conditions (Obata and Fernie, 2012). Advances in molecular studies have identified signal transduction pathways and characteristics of underlying plant stress responses mechanisms, highlighting several physical, biochemical, and physiological changes by each stress elicitors. Implementing a sustainable strategy to improve plant resistance against such environmental limitations is of great importance to secure and optimize global food production. One of the eco-friendly approaches is the application of PGPR and/or their byproducts (Mayak et al., 2004; Bano and Fatima, 2009; Piccoli and Bottini, 2013; Zafar-Ul-Hye et al., 2014; Qin et al., 2016; Abd El-Daim et al., 2019; Ipek et al., 2019), which can sustainably assist the plants to withstand the extreme environmental conditions (Table 1).
PGPR mediated plant osmolytes homeostasis results from accumulation of specific solutes, including proline, sugars, polyamines, betaines, polyhydric alcohols, and other amino acids, and plays a major role in retaining turgor-driven cellular swelling to withstand osmotic stress resulting from drought and high levels of soil salinity (Vurukonda et al., 2016). PGPR discharge osmolytes, which work in combination with those produced by plants, to synergistically maintain plant health by improving plant growth and development (Sandhya et al., 2010; Vardharajula et al., 2011). Inoculation of maize with three PGPR strains caused increased choline and glycine betaine accumulation and leaf relative water content, resulting in plant resistance and growth under drought conditions (Gou et al., 2015). In another study, application of a combination of PGPR, compost, and mineral fertilizer caused higher levels of soluble sugar and proline content, which enhanced the ability to maintain membrane stability, chlorophyll content, and water potential in wheat during stressful conditions (Kanwal et al., 2017). Likewise, higher plant tolerance to water scarcity reported in cultivars of rice inoculated with PGPR consortia was associated with the accumulation of proline (Gusain et al., 2015).
Biotic stresses, such as pests and diseases, are a common problem in agricultural production and results in significant crop loss. Increases in global temperature and changes in precipitation, in some parts of the world, due to climate change, has led to new crop pests and diseases (Naamala and Smith, 2020). Tools such as biotechnology and plant breeding have been used to address these pressures. Although successful outcomes have been observed, plant breeding is a long process and developed cultivars may succumb to new pests and diseases. PGPM can act as biocontrol agents for plant protection against various pathogens including fungi, bacteria, viruses and insects (Mishra et al., 2015; Myresiotis et al., 2015; Ali et al., 2020). They have several advantages compared to chemical pesticides, including being safe for humans and the environment, degrading more easily in soil and having lower potential to result in the development of resistance in the pathogens (Berg and Smalla, 2009). Previous literature has indicated the potential for disease reduction in major crops, such as rice, wheat, and corn by using seeds treated with microbial biocontrol agents (Heydari et al., 2007; Karthiba et al., 2010; Senthilraja et al., 2013). PGPM are a hopeful approach that can complement or supplement existing integrated biotic stress management practices, such as crop rotation, cautious and limited use of chemicals, as well as plant breeding and biotechnology. Research has shown a number of promising PGPM strains for use in pathogen biocontrol (Table 2), some of which have already been commercialized (Alizadeh et al., 2013; Moussa et al., 2013; De Vrieze et al., 2018; Zhao et al., 2018), as single strains or as a consortium. For instance, Trichoderma harzianum Tr6, and Pseudomonas sp. Ps14, enhanced cucumber's resistance to Fusarium oxysporum f. sp. radicis cucumerinum through induced systemic resistance (ISR) (Alizadeh et al., 2013). Pseudomonas fluorescens and Bacillus subtilis were reported to reduce the negative effects of Fusarium graminearum on wheat (Moussa et al., 2013). Bacillus cepacia mitigated the effect of Fusarium oxysporum and Fusarium culmorum in potato under storage conditions (Recep et al., 2009). Pseudomonas migulae Pf014 and Bacillus amyloliquefaciens Bs006 were reported to mitigate the effects of Fusarium oxysporum in cape gooseberry (Díaz et al., 2013). Bacillus and species, such as Bacillus subtilis (Sneb 815), Pseudomonas putida (Sneb 821), and Pseudomonas fluorescens have been reported to affect the growth cycle of Meloidogyne incognita (Zhao et al., 2018; Viljoen et al., 2019). A consortium of Fusarium oxysporum Fo162 and Rhizobium etli induced systemic resistance to Aphis gossypii (Martinuz et al., 2012). Bacillus subtilis slowed the growth of Bemisia tabaci in tomato plants.
Consortia are believed to be more effective at controlling biotic stress (Alizadeh et al., 2013; Zhao et al., 2018) than single inoculants. Although this has been shown in some research, others have shown the contrary. Therefore, more research is needed to come to a sound conclusion. However, consortia have some advantages over single strains which may lead a better efficiency. For instance, microbial species may synergistically interact and confer benefits to each other (De Vrieze et al., 2018; Zhao et al., 2018). Such benefits may include production of secondary metabolites such as exopolysaccharides that might render the non-producing strain resistant to stress (Mehnaz, 2016), or the breakdown of substrates to forms that other members of the consortium can use (Bender et al., 2016). Perhaps this may explain why ineffective strains sometimes become effective in a consortium. For instance, in an experiment conducted by Santhanam et al. (2015), two bacterial strains with insignificant effects on reducing mortality in tobacco due to sudden wilt pathogens, became effective upon inclusion in a consortium with three other bacteria (Santhanam et al., 2015). However, some PGPM may be inefficient in a consortium but efficient as single strains (Zhao et al., 2018). In conclusion, it is not always true that PGPM in a consortium will perform better than single strain.
PGPM employ a number of mechanisms to mitigate biotic stress. They include direct mechanisms such as hyper parasitism and the production of substances such as antibiotics, which antagonize the pathogen (De Vrieze et al., 2018), as well as indirect mechanisms such as ISR (Alizadeh et al., 2013; Martínez-Medina et al., 2017; Romera et al., 2019) and competition for nutrients and niche space (Recep et al., 2009; Vanitha and Ramjegathesh, 2014; Tripathi et al., 2018). ISR enables the whole plant to develop more resistance to pathogens. ISR is largely a jasmonic acid and ethylene dependent pathway, that can function without the pathogenesis-related (PR) gene (Romera et al., 2019) although the signaling pathway may be PGPM and host plant species dependent (Alizadeh et al., 2013). It can be induced by transcription factor MYB72, hormones, and signal molecules such as auxins and nitric oxide (Zamioudis et al., 2015; Martínez-Medina et al., 2017; Romera et al., 2019). The process through which PGPM elicit ISR is not yet fully understood, although it is suggested that volatile organic compounds and microbe associated molecular patterns (MAMPS) are some of the major elicitors (Martínez-Medina et al., 2017; Tyagi et al., 2018).
Production of defense enzymes such as 1-aminocyclopropane1-carboxylate (ACC) deaminase has also been reported as a mechanism through which PGPM mitigate biotic stress (Toklikishvili et al., 2010; Dixit et al., 2016). PGPM may also attract natural enemies of the pathogen, thereby indirectly controlling the biotic stress (Schausberger et al., 2012; Alizadeh et al., 2013; Pangesti et al., 2015). There are also incidences where a biocontrol agent did not have a significant effect on the biotic stress response but increased crop yield in their presence. For instance, increased yield, in the presence of aphids was observed in bell pepper plants treated with Bacillus subtilis and Bacillus amyloliquefaciens (Herman et al., 2008). This may particularly be of interest in areas where the biotic stress has become unresponsive to other control and management options. A PGPM may possess one or more mechanisms of biocontrol. For instance, species from genres Bacillus, Pseudomonas, Alcaligenes, Rhizobium, Aeromonas, and Streptomyces have been identified as biocontrol agents, and their ability to control plant pathogens have been well-documented (Ahmad et al., 2008; Alemu, 2016; Das et al., 2017; Zachow et al., 2017; Abdelmoteleb and González-Mendoza, 2020). These biocontrol agents produce biological compounds (secondary metabolites) that have broad spectrum effects, which may cause beneficial activity against plant pathogens. Among these, hydrogen cyanide (HCN) produced by many biocontrol agents has activity against a wide range of plant pathogens. HCN, sometimes referred to as prussic acid, is a volatile broad-spectrum secondary metabolite produced by many rhizobacteria; it plays a crucial role in biological control of many pathogenic bacteria in the soil. For example, the suppression of sunflower charcoal rot and tomato root knot diseases caused by Macrophomina phaseolina and Meloidogyne javanica, respectively, was attributed to the production of HCN secreted by bacterial strains (Siddiqui et al., 2006; Reetha et al., 2014). In addition, Vanitha and Ramjegathesh (2014) observed siderophore, antibiotic, and HCN production in Pseudomonas fluorescens species which affected proliferation of Macrophomina phaseolina (Tassi) Goid. Production of ACC- deaminase is believed to be the mechanism by which Paenibacillus lentimorbus B-30488 (B-30488) mitigates Scelerotium rolfsii in tomato (Dixit et al., 2016). Recent studies show that HCN may promote plant growth by hindering plant pathogens. The inhibition process starts in mitochondria where HCN disrupts electron transport to reduce energy supply to the cell, eventually leading to the death of pathogenic organisms.
Research in biocontrol is still on going and better strains will be discovered while existing ones may be improved. Use of microbial compounds either together with microbial cells or independently, is already a research area of interest. Such research may be able to solve some of the shortcomings associated with use of biocontrol technology, such as inconsistencies observed under field conditions. With some shortcomings of biocontrol addressed, the phytomicrobiome can be a good resource for mitigating biotic stress, particularly amidst the threat of climate change.
As climate change conditions continue to develop, we anticipate more frequent occurrences of extreme environmental limitation, making life stressful for living beings including microbes. The role of the phytomicrobiome in promoting plant growth under optimal and/or challenging environmental conditions is considerable. The incorporation of phytomicrobiome members into agricultural systems is an important and sustainable climate change mitigation strategy. However, there are certain factors that could substantially limit microbial efficiency, particularly under field conditions. Existing studies on plants and their associations with phytomicrobiome members, have demonstrated one perspective regarding these relationships, for example, the plant (host) and a specific associated microbe have generally been the sole focus. We have rarely considered how this association is affected by other members of the phytomicrobiome community, and what other factors need to be considered before incorporating biological techniques into natural growth conditions. In this section, we discuss some of the major factors that could adversely affect PGPR efficiency predominantly when exposed to natural soil conditions (Figure 2).
Soil temperatures, as a consequence of developing climate change conditions, have gradually continued to rise (Zhang et al., 2019) making it increasingly difficult for living beings to survive, including those living in close association with the plants. Microbes, like all other forms of life, depend on optimum temperature for optimal proliferation, community diversity and physiological activities (Wu et al., 2010). Under extreme temperatures both plants and their associated microbial community suffer from extreme heat and cold stress (Khare and Arora, 2015; Zhang et al., 2020), which in turn, trigger physiological response mechanisms (Ma et al., 2018) in order to survive non-optimal temperatures. Perhaps, the variations in the temperature and its effects on PGPR-plant interactions may result in positive or negative outcomes. Soil warming significantly increases microbial respiration as well as mortality rate (Wu et al., 2010; Schindlbacher et al., 2011) resulting in potential ineffectiveness of PGPR. For instance, some rhizobia are able to produce nodules while tolerating heat stress (Gray and Smith, 2005), however, their efficiency might still be affected by high temperatures. High temperatures are also associated with reductions in plant root hairs. As a result, there is reduced surface area for plant microbe interactions in the soil.
Some PGPR thrive under low temperatures and their ability to enhance plant performance under cold temperatures is being more widely exploited (Pedranzani et al., 2016; Liu et al., 2017; Ghorbanpour et al., 2018) but the overall understanding of their potential and mechanisms is still incomplete, due to variations related to the plant species the PGPR were isolated from. Studies have shown that PGPR isolated from areas of high temperatures are best adapted to high temperatures (Gray and Smith, 2005) and vice versa. Sometimes microbes can persist at temperatures as high as 45°C (Ali et al., 2011) but functionality as PGPR may be lost as it may be expending much of its energy responding to the very challenging external environment. Similarly, at low temperatures metabolic activities of cells are reduced, leading to inhibition of normal activities as reported for Bradyrhizobium japonicum at 15°C (Antoun and Prévost, 2005). Furthermore, low rhizosphere temperatures have been reported to inhibit synthesis and release of plant-to-PGPR signaling compounds (Pan and Smith, 1998) which hinders effective engagement in the early stages of symbiotic associations. The fact that higher and lower temperatures have shown effects on the efficacy of PGPR (Dutta and Podile, 2010; Wu et al., 2010) suggests that temperature has substantial impact on gene expression by these microorganisms. This further cautions that PGPR should be evaluated for their suitability at specific soil temperatures, and probably other soil attributes, to achieve the intended results after application as climate change continues to increase global temperature fluctuation.
In soil, the nutrient variability and availability to both plants and microbes is strongly affected by soil pH. pH is the measure of hydrogen cation concentration in the soil colloidal solution (Neina, 2019), which affects most chemical reactions in the soil. Microbial community and population dynamics have been shaped by pH variation in the soil. As a result, acidic soils are dominated by Acidobacteria (Shen et al., 2013), while Actinobacteria increasingly dominate in more alkaline soils (Jeanbille et al., 2016). The major effect of pH on the cell is the disruption of protein functioning (Hyyryläinen et al., 2001; Puissant et al., 2019). A very slight change in pH interferes with amino acid functional group ionization and impairs hydrogen bonding. This results in a change in protein folding leading to denaturation and cessation of enzymatic activities (Booth et al., 2002; Puissant et al., 2019).
Most rhizospheric microbes and plants share similar optimum pHs (near 6.0) for growth and survival. Environments with lower and higher pHs require microbes to adjust their biochemical properties; activities allowing adaptation to more extreme pH conditions may lead to altered microbial community structure (Roe et al., 1998). However, the relationship between these microbial survival mechanisms and plant growth promotion is not well-understood, and may result in positive or negative effects. Major functions of cells such as nutrient acquisition, cytoplasmic pH homeostasis, and protection of DNA and proteins are largely affected by low pH (Booth et al., 2002). Microorganisms can produce a thin biofilm composed of polysaccharides and proteins, which buffers the cell from changes in the pH (Wang et al., 2018), which may lead to reduced efficacy for PGPR.
Nutrient availability in the soil plays a major role in the maintenance of soil health and its productivity. Inherently less fertile soils tend to have smaller PGPR populations (Bhattarai et al., 2015) and the introduction of new microbes through soil inoculation results in poor microbial colonization of the area due to a lack of nutrients. Therefore, the efficacy of PGPR depends not only on less competition for resources (Ashman and Puri, 2013) and lower levels of antagonistic effects from other microbes, but also on the availability of nutrients in the soil; hence rapid rhizosphere colonization ultimately benefits the host plant. However, a higher diversity of microbial taxa in fertile soils results in a more complex inter- and intraspecies interactions which permit suppression antagonistic microbes. Furthermore, many studies of the legume-rhizobia symbiosis indicate that BNF efficiency tends to decrease in soils with high levels of soil N (Guinet et al., 2018; Romanyà and Casals, 2019). This emphasizes that sometimes scarcity of resources/stress creates more demand for the PGPR, to increase efficiency in assisting plants.
Plant-microbe interaction is, in many cases, dictated by host specificity, which limits broad spectrum application of PGPR across many plant species. In the soil, where diverse groups of microbes exist, a lack of specificity would result in higher competition among microbial taxa. Host plants—PGPR interactions, in many cases, are specific (Figueiredo et al., 2010), in part because their initial process of association involves signal cross talk between partners (Chagas et al., 2018). Signaling impacts PGPR efficacy in the soil in two main ways. Signaling between plants and microbes (Kan et al., 2017) is essential for the overall efficacy of many PGPR. Any alteration of the exudates produced by plant roots and signal molecules produced by microbes reduces the recognition of potential symbiotic partners. Factors such as rhizosphere temperature, soil pH, and fertility are all essential in plant and microbe growth and a deviation from optimal conditions may result in the production of altered root exudates and signaling compounds. Population related signal exchange within bacterial taxa is termed quorum sensing (QS) (Ryan et al., 2015; Smith et al., 2015; Kan et al., 2017; Chagas et al., 2018). QS shapes the behavior of the microbial populations by allowing or restricting interactions. N-acyl homoserine lactone (AHL) was the first QS identified from gram-negative bacteria (Eberhard et al., 1981). QS has a wide range of influences on microbe-to-microbe interactions such as initiating virulence and the production of antimicrobial compounds (Clinton and Rumbaugh, 2016; Chagas et al., 2018). Furthermore, QS is involved in plant-microbe interactions as well as microbe inter- and intraspecies communications, which all have the potential to affect plant growth and development (Clinton and Rumbaugh, 2016).
Most of this limited mutualistic association between plants and their PGPR reduces the possibility of plants benefiting from a wide range of microbes, which are either native, or new to a specific environment (Figueiredo et al., 2010; Wandrag et al., 2013). There is limited knowledge regarding promiscuity (ability to associate with a wide range of plant species) of PGPR, except for legume-rhizobia symbiosis (Keet et al., 2017). Promiscuous PGPR, such as some rhizobia, in most cases have shown inconsistent results in terms of efficacy (Labuschagne et al., 2010). Due to the specificity of interactions, it is not surprising to find that strains within the same species of PGPR can show differences in their effectiveness when interacting with the same host plant species (Dwivedi et al., 2015; Keet et al., 2017).
There are many challenges associated with the introduction of specific PGPR into a new environment, such as competition and antagonistic effects of indigenous microbes (Clinton and Rumbaugh, 2016). In the soil, competition is high as the result of low nutrients and energy sources providing only about 5% of required nutrients when compared to simulated laboratory conditions (Ashman and Puri, 2013). Only meaningfully competitive microbes are able to survive during constant vying for nutrients, including carbon and nitrogen (Stengel and Gelin, 1998). Most studies hold the view that PGPR, to be ecologically competent, must be able to colonize the plant environment, while interacting harmoniously with indigenous microbes, to improve plant growth (Trivedi et al., 2012). Therefore, the efficiency of introduced PGPR meaningfully depends on the diversity of the indigenous population. The introduced PGPR may face antagonistic effects from the native soil microbes. This is a common defensive mechanism for most of microbes, against invasive microbes; some reports indicate that as much as 90% of Actinomycete sp. isolated from the rhizosphere show this behavior against Bradyrhizobium japonicum (Pugashetti et al., 1982). Moreover, soil microbes could alter root exudate characteristics leading to poor or no attraction of the inoculated PGPR, reducing rhizoshpere colonization (Gupta Sood, 2003).
The effectiveness of beneficial microbes; successful association with the host and stability in the rhizosphere, is directly associated with varying environmental conditions and host related factors that could drastically affect microbial growth, stability and efficiency with regard to interactions with plants and other phytomicrobiome members. Considering the limitations and uncertainties associated with the use of microbial strains, alternate techniques that could bypass such limitations have been introduced which could improve plant health directly by stimulating plant growth or indirectly by helping other beneficial microbes. For instance, the use of microbial compounds and/or signals isolated from microbial cultures has shown promising effects on crop production with or without microbial strains under both stressed (drought and salinity) and unstressed environmental conditions (Nazari and Smith, 2020). These microbe-based compounds could be of different types with specific chemical distinctions. For instance, many of the known secreted microbial compounds are broad-spectrum non-ribosomal antibiotics, metabolic by-products, organic acids, lytic agents, and bacteriocins.
Growth stimulating compounds are usually excreted in response to externally generated stimuli, for instance, a signal received from the host plant, indicating nutrient deficiency or other suboptimal environmental conditions, and affecting microbial species in the rhizosphere (Nazari and Smith, 2020). However, the secretion of certain compounds does not necessarily require a specific environmental condition or an external stimulus. For instance, certain bacterial strains, when cultured in artificial media, produced compounds and/or signals that successfully promote plant growth, even in extreme environmental growth conditions. Perhaps such microbe-based compounds are able to provide benefit to a wider range of crop species as compared to microbial strains themselves, with the benefit of being unaffected by crop environmental conditions and without the specificities associated with microbes.
However, the isolation, identification and eventual commercialization of such compounds follow a very technical and complex procedure, which primarily begins by the isolation of plant associated “ecto” or endophytic microbes, respectively, from the rhizosphere and plant tissues, particularly from roots. This could be achieved through bioprospecting, the sampling of plants or soil from a range of habitats, followed by immediate microbial isolation in the laboratory. Once rhizobacteria are isolated from the plant or soil, they can then be subjected to in vitro screening assays to assess their ability to enhance plant growth and their possible role as biocontrol agents against phytopathogens. Promising strains that show plant growth stimulation or antagonistic activities can then be screened under controlled environmental conditions during early plant growth, where eventually the effective strains are chosen and validated for further analysis under field conditions for their ability to enhance crop growth. After successful repetitive trials, most of the firms, after formulating the product, tend to commercialize biological strains as biofertilizers and/or biocontrol products, and so far, in North America more than 33 PGPR-based products have been registered for commercial use (Nakkeeran et al., 2005). The number of industries involved in commercial biological-based products has increased drastically in the 21st century, due to high demand for their application as a method of biocontrol and crop protection. However, considering the shelf life and unpredictable behavior of the desired biological strain, and most importantly the limiting factors discussed above, producers or distributors go a step ahead by isolating and identifying the growth stimulating compounds produced by growth promoting microbes. Thus, after successful field trials, the microbial strains are further cultured and screened for the growth stimulating compounds which includes isolation, identification and further experimentation on crops under various growth conditions. In order to commercialize these products or make it available for local users, these products have to go through an intensive vetting and registration processes established by the health or food security departments of concerned regions, following much the same pattern described above for microbial strains. After government approval the products can be commercialized and mad available to crop producers (Figure 3).
The bacteriocin thuricin 17, a subclass IId bacteriocin with a molecular weight of 3.162 kDa, is a single, small peptide isolated from a bacterium found in soybean root tissue which possesses inhibitory properties to related microbial strains. The bacterium was identified as Bacillus thuringiensis NEB17, an endophytic bacterium (Gray et al., 2006a,b). This kind of bacteriocin acts either as a microbe-microbe or a microbe-plant signaling molecule in the rhizosphere; it not only hampers competing microorganisms sharing its niche but also physically extends the niche by triggering plant growth, particularly when exposed to abiotic stress. These bacteriocins benefit the growth and development of important crops such as soybean, canola and corn (Lee et al., 2009; Schwinghamer et al., 2016a,b; Subramanian et al., 2016). Interestingly, thuricin 17 has no inhibitory effect against symbiotic nitrogen fixing rhizobia or other PGPR members (Gray and Smith, 2005). This signal molecule enhances plant growth through direct and indirect mechanisms (Nazari and Smith, 2020). Induction of resistance to disease (Mabood et al., 2014) and inhibition of pathogens are indirect mechanisms for plant growth stimulation. In direct stimulation, thuricin 17 binds to receptors in leaves or roots, acting as a stress signal, causing the enhancement of metabolic pathways such as increases in photosynthetic rates. It is well-established that plants raise photosynthetic rates under biotic stress to compensate for damaged tissues (Nowak and Caldwell, 1984). In this manner, thuricin 17 has activated stress responses without a real stress necessarily being present, resulting in an increase in net photosynthesis (Gray and Smith, 2005).
Lipochitoolicosaccharides are host specific signal compounds that are essential for establishing legume-rhizobia symbiotic associations. The signal exchange is crucial in facilitating the plant endophytic association with phytomicrobiome members. Before any physical contact, both partners begin this mutualistic interaction by exchanging signals. In legume-rhizobia association, isoflavonoids exuded from legume roots are perceived by rhizobia through a NodD (LysM-RLK) receptor, activating nod genes. However, distinct chemical signals are secreted in legume species, and only the correct rhizobia respond to that specific signal. In response to plant signals, rhizobia secret a combination of Nod factors (LCOs) and effector proteins, which are perceived by Nod factor-specific (LysM-RLK) receptors in plants (Shah and Smith, 2020). The receptor for the lipo-chitooligosaccharides is a LysM kinase for the legume-rhizobia symbioses; this receptor system seems to have initially evolved for pathogen detection almost 2 billion years ago (Gust et al., 2012; Carotenuto et al., 2017).
Lipo-chitooligosaccharides have been shown to increase plant growth for a wide range of plant species, including Zea mays, Oryza sativa (Poaceae), Beta vulgaris (Chenopodaceae), Glycine max, Phaseolus vulgaris (Fabaceae), and Gossypium hirsutum (Malvaceae) (Prithiviraj et al., 2003), particularly when plants are growing under stressful conditions (Subramanian and Smith, 2015; Zipfel and Oldroyd, 2017) including drought (Hu et al., 2013) and salinity. For instance, a proteomic study on salt stressed plants revealed that LCOs have a big impact on proteins involved in carbon and energy metabolic pathways indicating a promising and improved growth effect under salt stress (Subramanian et al., 2016). Signaling plays a crucial role in establishing successful associations but can be interrupted by specific environmental conditions, thus utilization of inoculants with LCO already present may compensate for the limiting effects of stressful conditions (e.g., drought), as determined by Cerezini et al. (2016).
With a growing need to sustainably increase crop productivity and counter the effects of climate change, the phytomicrobiome is a promising area of research (Kashyap et al., 2018). However, to be environmentally effective, the use of microbes and microbe-based compounds should be practiced on a larger scale. Despite conducting successful trials in laboratories and/or in controlled plant growth conditions, and having reasonable knowledge regarding microbial efficiency, we have not been overly successful in transferring these techniques to the field. In order to bridge this substantial gap, there is a need to understand the inconsistencies, uncertainties, issues and challenges following the use of microbes or microbe-based compounds in the field.
Since 1960, the agrochemicals (fertilizers and pesticides) and other farm technologies, which are the main underpinnings of the green revolution, have been the mainstay of agricultural production. The efficiency and immediate effect of chemical fertilizers and pesticides have developed a deep-rooted confidence among farmers and growers, making it difficult for novel techniques to be implemented and/or substituted (Moser et al., 2008). Undoubtedly, the contribution of these agro-inputs in increasing crop productivity is indispensable, however, the increasing GHG emissions from unsustainable agricultural practices has changed some of the thinking around this. So far, we haven't introduced any technologies that could totally substitute for fertilizers and other chemical inputs, however, sustainable techniques, for instance, organic fertilizes, humic substances, or bio-based products (microbes and microbe-based compounds) have been developed that could, to at least some extent, assist in combatting climate change by reducing the use of chemical inputs (fertilizers and pesticides). It may be challenging to transfer these techniques; particularly microbe-based products, to the field as it depends on farmers' mindsets and whether or not they are willing to take risks by implementing new strategies to their fields, especially those that may come with uncertainties.
The first thing that prevents farmers from adopting microbe-based techniques is the lack of practical evidence of their effectiveness in the field (Moser et al., 2008). It is certainly difficult for farmers to adopt technologies that lack the surety of being effective, or to give up more efficient techniques (pesticides and fertilizers) that have demonstrated efficacy for over 50 years. Secondly, in comparison to PGPR strains or PGPR related products, agro-chemicals in small doses have been found to be more effective and immediate in terms of nutrient availability and pathogen control, hence further limiting the likelihood of adopting PGPR and biobased products. Thirdly, high fixed costs of biofertilizers, biopesticides, and bio-compounds can pose a large disadvantage, driving potential adopters to rely on agro-chemicals. Since these products are new to the market, their prices will go down only when they are distributed and/or produced on a large scale.
In addition, considering the “cost to efficiency” ratios of bioproducts, chemical additives provide better efficiency at much lower costs. To tackle these issues and inconsistencies, more research should be done to raise awareness among growers and address their unique needs. We must develop economically viable products and provide practical evidence of the product's efficacy. In addition, there is an ultimate need to educate farmers regarding climate change, its consequences, and the role PGPM based products could play in mitigating climate change.
One of the major challenges of using PGPMs is their unpredictable behavior in the field compared to under controlled environmental conditions. It is very difficult to predict an organism's reaction in natural environments even after conducting successful and effective trials in laboratories. One of the major reasons for this is plant-microbe specificity (Mushtaq, 2020). However, another challenge usually faced by PGPR developers is root colonization and viability of the desired inoculant. In order to populate an inoculant and to attain an effective response, the inoculant should colonize the host at a certain population density (Mcnear, 2013). However, the crop specificity of PGPR can be independent of root colonization. Beside crop specificity, environmental factors can strongly influence the viability and colonization of a PGPM strain, for instance soil type, temperature, moisture, and the presence of other competitive microbial entities in the rhizosphere. These vary from crop to crop and field to field (Saharan and Nehra, 2011), making it difficult and far more complex for the PGPR developers and commercial vendors to provide separate PGPR inoculants for different crops grown under different environmental conditions.
In order to address the inconsistencies associated with specificity, adaptability and colonization of single microbial inoculants, inoculants with two or more microbial species, or microbial consortia, constitute a potential approach (Naamala and Smith, 2020). While, microbial consortia have their own limitations, if well-formulated, they may be more promising than single strains by synergistically interacting with each other and/or helping other beneficial phytomicrobiome members in the rhizosphere. This may indirectly benefit host plants through the production of specific compounds that facilitate the colonization by other microbes, or might act as a signaling compound, further facilitating plant-microbe association (Bender et al., 2016). In addition, as the viability of PGPR strains varies among crop species and environmental conditions, microbial consortia consisting of two or more members from different genera or phyla with varying tolerance to environmental conditions have a better chance of survival and adaptability. However, it is very challenging to have compatible members in a consortium. It is reasonably probable that some of the members will produce compounds that are lethal to other members or may hinder their growth promoting capabilities (Jha and Saraf, 2015).
Considering the limitations and uncertainties associated with the use of microbial strains, microbial compounds and/or products are suggested to be suitable alternatives that could sustainably promote crop growth by successfully circumventing some of the critical environmental limitations, hindering microbial efficiency. For instance, the use of microbial compounds (e.g., thuricin 17 and lipo-chitooligosaccharides) has shown promising effects on crop production even under extreme environmental growth conditions (drought and salinity) (Nazari and Smith, 2020). Perhaps such microbe-based compounds will be able to provide benefit to a wider range of crop species as compared to microbial strains, with the benefit of being unaffected by crop environmental conditions and without the specificities associated with microbes. However, further molecular experiments are still required to better understand the metabolite-plant species-microbe combinations, and the time-course effect of host rhizosphere chemistry following the use of selective inoculants.
One of the obstacles to the expansion and production of microbe-based products are risk assessment and testing policies set by every country, and sometimes subnational jurisdictions, to avoid the production and distribution of lethal/damaging organisms (Tabassum et al., 2017). Regulatory processes for biological or biobased products follow a very complex and extensive protocol set by the regulatory and health authorities of each country. The most intensive constraints for registering a biological or biobased product are the extensive time period, complex documentation and high fees associated with the whole process of product registration. Since, the registration and regulatory policies vary with country; as each country, or in some cases, each sub-jurisdiction has their own rules and norms to be addressed in order to register biobased products, and it can be very difficult for firms to meet the regulatory requirements, should they want to introduce their product in multiple regions or countries. Usually, a products registration requires a national approval with a certification provided by the Directorate General of Health or any other concerned regulatory authority. The product will then undergoes extensive and critical inspections and/or reviews by experts, supervised by the food safety authorities and national commissions of that particular country or region (Basu et al., 2021). Eventually, the firm will be notified with approvals and certification, which will allow the producers to commercialize their product under the strict policies and instructions of the certification authority. Climate change is a global issue, and sustainable techniques that could contribute to mitigating climate change should have simpler regulatory and registration policies. In addition, countries should be flexible or at least develop less complex policies for registering and transporting native and imported microbial technologies. Doing so might help firms or PGPR developers to feasibly expand and distribute PGPR-based techniques within and outside the country of origin.
The growing human population is causing demand of increased food production, but under conditions of intensifying climate change and a finite base of farmland. So far, chemical application (e.g., fertilization and pesticides) and molecular techniques (e.g., gene modification) have been used to address this challenge. As a more sustainable agricultural approach, PGPR that regularly establish mutualistic interactions with host plants related to nutrient absorption (N fixation, P and K solubilization and siderophore production), enhanced stress resistance (abiotic and biotic), regulation of plant development and physiology through signal compound production, including phytohormones and specific inter-organismal signal compounds. In a similar way, root exudates, secreted by host plants into the rhizosphere as a reduced carbon resource for phytomicrobiome members, help provide a stable habitat for microbe growth. Thus, the application of PGPR is an available and under-exploited mechanism to enhance yield and improve resilience of crop plants to the various conditions challenging crop growth, development and yield. However, environmental conditions, such as the soil temperature, pH and soil fertility do not just affect plant development, they also have influence on the efficiency of PGPR, which in turn alters the ability of cultivated plants to produce biomass and food materials under climate change related environmental extremes.
Because of the beneficial effects provided by beneficial phytomicrobiome members, their utilization and commercialization are now being much more widely considered. Potential products, such as the exogenous application of microbe-to-plant signal compounds (e.g., thuricin 17 and LCOs) already show positive effects on crop growth and development, and biomass and food production by key crops under specific abiotic stress conditions; often those associated with climate change. However, work still needs to be done to expand utilization of these products and potential products on a wider range crops, instead specific crop species. In addition, it is still unclear if the biodiversity of the plant-associated microbial community will be affected by the use of these products; at the same time the abundant community of soil microbes extant at the time PGPR technologies are applied can affect the growth of these added microbes and their ability to provide vital effects to the plant-microbe-soil ecosystem. Furthermore, <1% of soil and plant-associated microbes can currently be cultured in vitro (Pham and Kim, 2012). Mutualistic and parasitic relationships between microbes and plants already exist, and have done so for several billion years; there is a considerable amount still to be learned about and exploited regarding these relationships.
All authors listed have made a substantial, direct and intellectual contribution to the work, and approved it for publication.
This research was funded by the Natural Science and Engineering Research Council of Canada Grant No. RGPIN 2020-07047.
The authors declare that the research was conducted in the absence of any commercial or financial relationships that could be construed as a potential conflict of interest.
Abd El-Daim, I. A., Bejai, S., and Meijer, J. (2019). Bacillus velezensis 5113 induced metabolic and molecular reprogramming during abiotic stress tolerance in wheat. Sci. Rep. 9, 1–18. doi: 10.1038/s41598-019-52567-x
Abdelmoteleb, A., and González-Mendoza, D. (2020). A novel streptomyces rhizobacteria from desert soil with diverse anti-fungal properties. Rhizosphere 16:100243. doi: 10.1016/j.rhisph.2020.100243
Adesemoye, A. O., and Kloepper, J. W. (2009). Plant–microbes interactions in enhanced fertilizer- use efficiency. Appl. Microbiol. Biotechnol. 85, 1–12. doi: 10.1007/s00253-009-2196-0
Adesemoye, A. O., Torbert, H. A., and Kloepper, J. W. (2009). Plant growth-promoting rhizobacteria allow reduced application rates of chemical fertilizers. Microb. Ecol. 58, 921–929. doi: 10.1007/s00248-009-9531-y
Ahmad, F., Ahmad, I., and Khan, M. (2008). Screening of free-living rhizospheric bacteria for their multiple plant growth promoting activities. Microbiol. Res. 163, 173–181. doi: 10.1016/j.micres.2006.04.001
Akhtar, S. S., Mekureyaw, M. F., Pandey, C., and Roitsch, T. (2020). Role of cytokinins for interactions of plants with microbial pathogens and pest insects. Front. Plant Sci. 10:1777. doi: 10.3389/fpls.2019.01777
Al-Ani, R. A., and Adhab, M. A. (2013). Bean yellow mosaic virus (BYMV) on broadbean: characterization and resistance induced by Rhizobium leguminosarum. J. Pure Appl. Microbiol. 7, 135–142.
Alemu, F. (2016). Isolation of Pseudomonas flurescens from rhizosphere of faba bean and screen their hydrogen cyanide production under in vitro stduy, Ethiopia. Am. J. Life Sci. 4:13.
Alexandratos, N., and Bruinsma, J. (2012). World Agriculture Towards 2030/2050: the 2012 Revision. ESA Working Paper No. 12-03, FAO, Rome.
Ali, S., Hameed, S., Shahid, M., Iqbal, M., Lazarovits, G., and Imran, A. (2020). Functional characterization of potential PGPR exhibiting broad-spectrum antifungal activity. Microbiol. Res. 232:126389. doi: 10.1016/j.micres.2019.126389
Ali, S. Z., Sandhya, V., Grover, M., Linga, V. R., and Bandi, V. (2011). Effect of inoculation with a thermotolerant plant growth promoting Pseudomonas putida strain AKMP7 on growth of wheat (Triticum spp.) under heat stress. J. Plant Interactions 6, 239–246. doi: 10.1080/17429145.2010.545147
Alizadeh, H., Behboudi, K., Ahmadzadeh, M., Javan-Nikkhah, M., Zamioudis, C., Pieterse, C. M., et al. (2013). Induced systemic resistance in cucumber and Arabidopsis thaliana by the combination of Trichoderma harzianum Tr6 and Pseudomonas sp. Ps14. Biol. Control 65, 14–23. doi: 10.1016/j.biocontrol.2013.01.009
Alori, E. T., Dare, M. O., and Babalola, O. O. (2017). Microbial inoculants for soil quality and plant health, in Sustainable Agriculture Reviews, eds. E. Lichtfouse (Cham: Springer), 281–307. doi: 10.1007/978-3-319-48006-0_9
Amara, U., Khalid, R., and Hayat, R. (2015). Soil bacteria and phytohormones for sustainable crop production, in Bacterial Metabolites in Sustainable Agroecosystem, ed D. K. Maheshwari (Cham: Springer International Publishing), 87–103. doi: 10.1007/978-3-319-24654-3_5
Ansari, F., Jabeen, M., and Ahmad, I. (2021). Pseudomonas azotoformans FAP5, a novel biofilm-forming PGPR strain, alleviates drought stress in wheat plant. Int. J. Environ. Sci. Technol. 18, 1–16. doi: 10.1007/s13762-020-03045-9
Antar, M., Gopal, P., Msimbira, L. A., Naamala, J., Nazari, M., Overbeek, W., et al. (2021a). Inter-organismal signaling in the rhizosphere, in Rhizosphere Biology: Interactions Between Microbes and Plants (Singapore: Springer), 255–293. doi: 10.1007/978-981-15-6125-2_13
Antar, M., Lyu, D., Nazari, M., Shah, A., Zhou, X., and Smith, D. L. (2021b). Biomass for a sustainable bioeconomy: an overview of world biomass production and utilization. Renew. Sustain. Energy Rev. 139:110691. doi: 10.1016/j.rser.2020.110691
Antoun, H., and Prévost, D. (2005). Ecology of plant growth promoting rhizobacteria, in PGPR: Biocontrol and Biofertilization, eds. Z. A. Siddiqui (Dordrecht: Springer), 1–38. doi: 10.1007/1-4020-4152-7_1
Archana, D., Nandish, M., Savalagi, V., and Alagawadi, A. (2012). Screening of potassium solubilizing bacteria (KSB) for plant growth promotionalactivity. Bioinfolet-A Q. J. Life Sci. 9, 627–630.
Arkhipova, T. N., Prinsen, E., Veselov, S. U., Martinenko, E. V., Melentiev, A. I., and Kudoyarova, G. R. (2007). Cytokinin producing bacteria enhance plant growth in drying soil. Plant Soil 292, 305–315. doi: 10.1007/s11104-007-9233-5
Ashman, M., and Puri, G. (2013). Essential soil Science: A Clear and Concise Introduction to Soil Science. Malden, MA: John Wiley & Sons.
Awasthi, R., Kaushal, N., Vadez, V., Turner, N. C., Berger, J., Siddique, K. H., et al. (2014). Individual and combined effects of transient drought and heat stress on carbon assimilation and seed filling in chickpea. Functional Plant Biology 41, 1148–1167. doi: 10.1071/FP13340
Azziz, G., Bajsa, N., Haghjou, T., Taul,é, C., Valverde, Á., Igual, J. M., et al. (2012). Abundance, diversity and prospecting of culturable phosphate solubilizing bacteria on soils under crop–pasture rotations in a no-tillage regime in Uruguay. Appl. Soil Ecol. 61, 320–326. doi: 10.1016/j.apsoil.2011.10.004
Babalola, O. O. (2010). Beneficial bacteria of agricultural importance. Biotechnol. Lett. 32, 1559–1570. doi: 10.1007/s10529-010-0347-0
Babalola, O. O., and Glick, B. R. (2012). The use of microbial inoculants in African agriculture: current practice and future prospects. J. Food Agric. Environ. 10, 540–549.
Backer, R., Rokem, J. S., Ilangumaran, G., Lamont, J., Praslickova, D., Ricci, E., et al. (2018). Plant growth-promoting rhizobacteria: context, mechanisms of action, and roadmap to commercialization of biostimulants for sustainable agriculture. Front. Plant Sci. 9:1473. doi: 10.3389/fpls.2018.01473
Badr, M., Shafei, A., and Sharaf El-Deen, S. (2006). The dissolution of K and P-bearing minerals by silicate dissolving bacteria and their effect on sorghum growth. Res. J. Agricult. Biol. Sci. 2, 5–11.
Bano, A., and Fatima, M. (2009). Salt tolerance in Zea mays (L). following inoculation with Rhizobium and Pseudomonas. Biol. Fertility Soils 45, 405–413. doi: 10.1007/s00374-008-0344-9
Bano, A., and Muqarab, R. (2017). Plant defence induced by PGPR against Spodoptera litura in tomato (Solanum lycopersicum L.). Plant Biol. 19, 406–412. doi: 10.1111/plb.12535
Bardgett, R. D., Freeman, C., and Ostle, N. J. (2008). Microbial contributions to climate change through carbon cycle feedbacks. ISME J. 2, 805–814. doi: 10.1038/ismej.2008.58
Barnawal, D., Bharti, N., Pandey, S. S., Pandey, A., Chanotiya, C. S., and Kalra, A. (2017). Plant growth-promoting rhizobacteria enhance wheat salt and drought stress tolerance by altering endogenous phytohormone levels and TaCTR1/TaDREB2 expression. Physiol. Plantarum 161, 502–514. doi: 10.1111/ppl.12614
Basu, A., Prasad, P., Das, S. N., Kalam, S., Sayyed, R. Z., Reddy, M. S., et al. (2021). Plant Growth Promoting Rhizobacteria (PGPR) as green bioinoculants: recent developments, constraints, and prospects. Sustainability 13:140. doi: 10.3390/su13031140
Bates, T. R., and Lynch, J. P. (2001). Root hairs confer a competitive advantage under low phosphorus availability. Plant Soil 236, 243–250. doi: 10.1023/A:1012791706800
Bechtaoui, N., Raklami, A., Benidire, L., Tahiri, A.-I., Göttfert, M., and Oufdou, K. (2020). Effects of PGPR Co-inoculation on growth, phosphorus nutrition and phosphatase/phytase activities of faba bean under different phosphorus availability conditions. Polish J. Environ. Stud. 29, 1557–1565. doi: 10.15244/pjoes/110345
Belimov, A. A., Zinovkina, N. Y., Safronova, V. I., Litvinsky, V. A., Nosikov, V. V., Zavalin, A. A., et al. (2019). Rhizobial ACC deaminase contributes to efficient symbiosis with pea (Pisum sativum L.) under single and combined cadmium and water deficit stress. Environ. Experi. Botany 167:103859. doi: 10.1016/j.envexpbot.2019.103859
Bender, S. F., Wagg, C., and Van Der Heijden, M. G. (2016). An underground revolution: biodiversity and soil ecological engineering for agricultural sustainability. Trends Ecol Evolut. 31, 440–452. doi: 10.1016/j.tree.2016.02.016
Berg, G., and Smalla, K. (2009). Plant species and soil type cooperatively shape the structure and function of microbial communities in the rhizosphere. FEMS Microbiol. Ecol. 68, 1–13. doi: 10.1111/j.1574-6941.2009.00654.x
Berlanas, C., Berbegal, M., Elena, G., Laidani, M., Cibriain, J. F., Sagües, A., et al. (2019). The fungal and bacterial rhizosphere microbiome associated with grapevine rootstock genotypes in mature and young vineyards. Front. Microbiol. 10:1142. doi: 10.3389/fmicb.2019.01142
Bhattarai, A., Bhattarai, B., and Pandey, S. (2015). Variation of soil microbial population in different soil horizons. J. Microbiol. Exp. 2:00044. doi: 10.15406/jmen.2015.02.00044
Bidondo, L. F., Silvani, V., Colombo, R., Pérgola, M., Bompadre, J., and Godeas, A. (2011). Pre-symbiotic and symbiotic interactions between Glomus intraradices and two Paenibacillus species isolated from AM propagules. In vitro and in vivo assays with soybean (AG043RG) as plant host. Soil Biol. Biochem. 43, 1866–1872. doi: 10.1016/j.soilbio.2011.05.004
Booth, I. R., Cash, P., and O'byrne, C. (2002). Sensing and adapting to acid stress. Antonie Van Leeuwenhoek 81, 33–42. doi: 10.1023/A:1020565206835
Bouchet, A.-S., Laperche, A., Bissuel-Belaygue, C., Snowdon, R., Nesi, N., and Stahl, A. (2016). Nitrogen use efficiency in rapeseed. A review. Agronomy for Sustain. Dev. 36:38. doi: 10.1007/s13593-016-0371-0
Brutting, C., Crava, C. M., Schafer, M., Schuman, M. C., Meldau, S., Adam, N., et al. (2018). Cytokinin transfer by a free-living mirid to Nicotiana attenuata recapitulates a strategy of endophytic insects. Elife 7:e36268. doi: 10.7554/eLife.36268
Buysens, S., Heungens, K., Poppe, J., and Hofte, M. (1996). Involvement of pyochelin and pyoverdin in suppression of Pythium-induced damping-off of tomato by Pseudomonas aeruginosa 7NSK2. Appl. Environ. Microbiol. 62, 865–871. doi: 10.1128/aem.62.3.865-871.1996
Cakmakci, R., Mosber, G., Milton, A. H., Alaturk, F., and Ali, B. (2020). The effect of auxin and auxin-producing bacteria on the growth, essential oil yield, and composition in medicinal and aromatic plants. Curr. Microbiol. 77, 564–577. doi: 10.1007/s00284-020-01917-4
Canfield, D. E., Glazer, A. N., and Falkowski, P. G. (2010). The evolution and future of Earth's nitrogen cycle. Science 330, 192–196. doi: 10.1126/science.1186120
Carotenuto, G., Chabaud, M., Miyata, K., Capozzi, M., Takeda, N., Kaku, H., et al. (2017). The rice LysM receptor-like kinase OsCERK1 is required for the perception of short-chain chitin oligomers in arbuscular mycorrhizal signaling. New Phytol. 214, 1440–1446. doi: 10.1111/nph.14539
Celloto, V. R., Oliveira, A. J., Goncalves, J. E., Watanabe, C. S., Matioli, G., and Goncalves, R. A. (2012). Biosynthesis of indole-3-acetic acid by new Klebsiella oxytoca free and immobilized cells on inorganic matrices. ScientificWorldJournal 2012:495970. doi: 10.1100/2012/495970
Cerezini, P., Kuwano, B. H., Dos Santos, M. B., Terassi, F., Hungria, M., and Nogueira, M. A. (2016). Strategies to promote early nodulation in soybean under drought. Field Crops Res. 196, 160–167. doi: 10.1016/j.fcr.2016.06.017
Chagas, F. O., De Cassia Pessotti, R., Caraballo-Rodriguez, A. M., and Pupo, M. T. (2018). Chemical signaling involved in plant–microbe interactions. Chem. Soc. Rev. 47, 1652–1704. doi: 10.1039/C7CS00343A
Chakraborty, U., Chakraborty, B., Basnet, M., and Chakraborty, A. (2009). Evaluation of Ochrobactrum anthropi TRS-2 and its talc based formulation for enhancement of growth of tea plants and management of brown root rot disease. J. Appl. Microbiol. 107, 625–634. doi: 10.1111/j.1365-2672.2009.04242.x
Chandra, D., Srivastava, R., Glick, B., and Sharma, A. (2020). Rhizobacteria producing ACC deaminase mitigate water-stress response in finger millet (Eleusine coracana (L.) Gaertn.). 3. Biotech. 10:65. doi: 10.1007/s13205-019-2046-4
Chu, B. C., Garcia-Herrero, A., Johanson, T. H., Krewulak, K. D., Lau, C. K., Peacock, R. S., et al. (2010). Siderophore uptake in bacteria and the battle for iron with the host; a bird's eye view. Biometals 23, 601–611. doi: 10.1007/s10534-010-9361-x
Clinton, A., and Rumbaugh, K. P. (2016). Interspecies and interkingdom signaling via quorum signals. Isr. J. Chem. 56, 265–272. doi: 10.1002/ijch.201400132
Cordero, I., Balaguer, L., Rincón, A., and Pueyo, J. J. (2018). Inoculation of tomato plants with selected PGPR represents a feasible alternative to chemical fertilization under salt stress. J. Plant Nutr. Soil Sci. 181, 694–703. doi: 10.1002/jpln.201700480
Dalcorso, G., Manara, A., and Furini, A. (2013). An overview of heavy metal challenge in plants: from roots to shoots. Metallomics 5, 1117–1132. doi: 10.1039/c3mt00038a
Daly, D. H., Velivelli, S. L., and Prestwich, B. D. (2017). The role of soil microbes in crop biofortification, in Agriculturally Important Microbes for Sustainable Agriculture, eds. V. Meena, P. Mishra, J. Bisht, and A. Pattanayak (Singapore: Springer), 333–356. doi: 10.1007/978-981-10-5589-8_16
Das, K., Prasanna, R., and Saxena, A. K. (2017). Rhizobia: a potential biocontrol agent for soilborne fungal pathogens. Folia Microbiol. 62, 425–435. doi: 10.1007/s12223-017-0513-z
De Vrieze, M., Germanier, F., Vuille, N., and Weisskopf, L. (2018). Combining different potato-associated Pseudomonas strains for improved biocontrol of Phytophthora infestans. Front. Microbiol. 9:2573. doi: 10.3389/fmicb.2018.02573
Dermastia, M. (2019). Plant hormones in phytoplasma infected plants. Front. Plant Sci. 10:477. doi: 10.3389/fpls.2019.00477
Díaz, A., Smith, A., Mesa, P., Zapata, J., Caviedes, D., and Cotes, A. M. (2013). Control of fusarium wilt in cape gooseberry by trichoderma koningiopsis and PGPR. IOBC-WPRS Bull 86, 89−94.
Dixit, R., Agrawal, L., Gupta, S., Kumar, M., Yadav, S., Chauhan, P. S., et al. (2016). Southern blight disease of tomato control by 1-aminocyclopropane-1-carboxylate (ACC) deaminase producing Paenibacillus lentimorbus B-30488. Plant Signal. Behav. 11:e1113363. doi: 10.1080/15592324.2015.1113363
Dodd, I. C., Zinovkina, N. Y., Safronova, V. I., and Belimov, A. A. (2010). Rhizobacterial mediation of plant hormone status. Ann. Appl. Biol. 157, 361–379. doi: 10.1111/j.1744-7348.2010.00439.x
Drost, S. M., Rutgers, M., Wouterse, M., De Boer, W., and Bodelier, P. L. (2020). Decomposition of mixtures of cover crop residues increases microbial functional diversity. Geoderma 361:114060. doi: 10.1016/j.geoderma.2019.114060
Dutta, S., and Podile, A. R. (2010). Plant growth promoting rhizobacteria (PGPR): the bugs to debug the root zone. Crit. Rev. Microbiol. 36, 232–244. doi: 10.3109/10408411003766806
Dwivedi, S. L., Sahrawat, K. L., Upadhyaya, H. D., Mengoni, A., Galardini, M., Bazzicalupo, M., et al. (2015). Advances in host plant and rhizobium genomics to enhance symbiotic nitrogen fixation in grain legumes, in Advances in Agronomy, eds. Donald L. sparks (Elsevier), 1–116. doi: 10.1016/bs.agron.2014.09.001
Eberhard, A., Burlingame, A. L., Eberhard, C., Kenyon, G., Nealson, K. H., and Oppenheimer, N. (1981). Structural identification of autoinducer of Photobacterium fischeri luciferase. Biochemistry 20, 2444–2449.
Egamberdieva, D. (2009). Alleviation of salt stress by plant growth regulators and IAA producing bacteria in wheat. Acta Physiol. Plantarum 31, 861–864. doi: 10.1007/s11738-009-0297-0
Egamberdieva, D., Hashem, A., and Alqarawi, A. A. (2015). Microbial phytohormones have a key role in mitigating the salt-induced damages in plants, in Bacterial Metabolites in Sustainable Agroecosystem, ed. D. K. Maheshwari (Cham: Springer International Publishing), 283–296. doi: 10.1007/978-3-319-24654-3_10
Egamberdieva, D., Wirth, S. J., Alqarawi, A. A., Abd_Allah, E. F., and Hashem, A. (2017). Phytohormones and beneficial microbes: essential components for plants to balance stress and fitness. Front. Microbiol. 8:2104. doi: 10.3389/fmicb.2017.02104
Etesami, H., and Beattie, G. A. (2017). Plant-microbe interactions in adaptation of agricultural crops to abiotic stress conditions, in Probiotics and Plant Health, eds. V. Kumar, M. Kumar, S. Sharma, and R. Prasad (Singapore: Springer), 163–200. doi: 10.1007/978-981-10-3473-2_7
FAO (2002). Human Vitamin and Mineral Requirements. Bangkok: Food and Agriculture Organization of the United Nations.
Figueiredo, M. D. V. B., Seldin, L., De Araujo, F. F., and Mariano, R. D. L. R. (2010). Plant growth promoting rhizobacteria: fundamentals and applications, in Plant Growth and Health Promoting Bacteria, eds. D. Maheshwari (Berlin; Heidelberg: Springer), 21–43. doi: 10.1007/978-3-642-13612-2_2
Ghorbanpour, A., Salimi, A., Ghanbary, M. T., Pirdashti, H., and Dehestani, A. (2018). The effect of Trichoderma harzianum in mitigating low temperature stress in tomato (Solanum lycopersicum L.) plants. Sci. Hortic. 230, 134–141. doi: 10.1016/j.scienta.2017.11.028
Giron, D., and Glevarec, G. (2014). Cytokinin-induced phenotypes in plant-insect interactions: learning from the bacterial world. J. Chem. Ecol. 40, 826–835. doi: 10.1007/s10886-014-0466-5
Goswami, D., Thakker, J. N., and Dhandhukia, P. C. (2016). Portraying mechanics of plant growth promoting rhizobacteria (PGPR): A review. Cogent Food Agri. 2:1127500. doi: 10.1080/23311932.2015.1127500
Goteti, P. K., Emmanuel, L. D. A., Desai, S., and Shaik, M. H. A. (2013). Prospective zinc solubilising bacteria for enhanced nutrient uptake and growth promotion in maize (Zea mays L.). Int. J. Microbiol. 2013:869697. doi: 10.1155/2013/869697
Gou, W., Tian, L., Ruan, Z., Zheng, P., Chen, F., Zhang, L., et al. (2015). Accumulation of choline and glycinebetaine and drought stress tolerance induced in maize (Zea mays) by three plant growth promoting rhizobacteria (PGPR) strains. Pak. J. Bot. 47, 581–586.
Gray, E., Lee, K., Souleimanov, A., Di Falco, M., Zhou, X., Ly, A., et al. (2006a). A novel bacteriocin, thuricin 17, produced by plant growth promoting rhizobacteria strain Bacillus thuringiensis NEB17: isolation and classification. J. Appl. Microbiol. 100, 545–554. doi: 10.1111/j.1365-2672.2006.02822.x
Gray, E., and Smith, D. (2005). Intracellular and extracellular PGPR: commonalities and distinctions in the plant–bacterium signaling processes. Soil Biol. Biochem. 37, 395–412. doi: 10.1016/j.soilbio.2004.08.030
Gray, E. J., Di Falco, M., Souleimanov, A., and Smith, D. L. (2006b). Proteomic analysis of the bacteriocin thuricin 17 produced by Bacillus thuringiensis NEB17. FEMS Microbiol. Lett. 255, 27–32. doi: 10.1111/j.1574-6968.2005.00054.x
Grosskinsky, D. K., Tafner, R., Moreno, M. V., Stenglein, S. A., Garcia De Salamone, I. E., Nelson, L. M., et al. (2016). Cytokinin production by Pseudomonas fluorescens G20-18 determines biocontrol activity against Pseudomonas syringae in Arabidopsis. Sci. Rep. 6:23310. doi: 10.1038/srep23310
Guinet, M., Nicolardot, B., Revellin, C., Durey, V., Carlsson, G., and Voisin, A.-S. (2018). Comparative effect of inorganic N on plant growth and N 2 fixation of ten legume crops: towards a better understanding of the differential response among species. Plant Soil 432, 207–227. doi: 10.1007/s11104-018-3788-1
Gundala, P., Chinthala, P., and Sreenivasulu, B. (2013). A new facultative alkaliphilic, potassium solubilizing, Bacillus Sp. SVUNM9 isolated from mica cores of Nellore District, Andhra Pradesh, India. Research and Reviews. J. Microbiol. Biotechnol. 2, 1–7.
Gupta Sood, S. (2003). Chemotactic response of plant-growth-promoting bacteria towards roots of vesicular-arbuscular mycorrhizal tomato plants. FEMS Microbiol. Ecol. 45, 219–227. doi: 10.1016/S0168-6496(03)00155-7
Gusain, Y. S., Singh, U., and Sharma, A. (2015). Bacterial mediated amelioration of drought stress in drought tolerant and susceptible cultivars of rice (Oryza sativa L.). Afr. J. Biotechnol. 14, 764–773. doi: 10.5897/AJB2015.14405
Gust, A. A., Willmann, R., Desaki, Y., Grabherr, H. M., and Nürnberger, T. (2012). Plant LysM proteins: modules mediating symbiosis and immunity. Trends Plant Sci. 17, 495–502. doi: 10.1016/j.tplants.2012.04.003
Hassen, A. I., Bopape, F., and Sanger, L. (2016). Microbial inoculants as agents of growth promotion and abiotic stress tolerance in plants, in Microbial Inoculants in Sustainable Agricultural Productivity, eds. D. Singh, H. Singh, R. Prabha (New Delhi: Springer), 23–36. doi: 10.1007/978-81-322-2647-5_2
Hayat, R., Ahmed, I., and Sheirdil, R. A. (2012). An overview of plant growth promoting rhizobacteria (PGPR) for sustainable agriculture, in Crop Production for Agricultural Improvement, eds. M. Ashraf, M. Öztürk, M. S. A. Ahmad, and A. Aksoy. (Dordrecht: Springer), 557–579. doi: 10.1007/978-94-007-4116-4_22
Herman, M., Nault, B., and Smart, C. (2008). Effects of plant growth-promoting rhizobacteria on bell pepper production and green peach aphid infestations in New York. Crop Protection 27, 996–1002. doi: 10.1016/j.cropro.2007.12.004
Heydari, A., Misaghi, I., and Balestra, G. (2007). Pre-emergence herbicides influence the efficacy of fungicides in controlling cotton seedling damping-off in the field. Int. J. Agri. Res. 2, 1049–1053. doi: 10.3923/ijar.2007.1049.1053
Hider, R. C., and Kong, X. (2010). Chemistry and biology of siderophores. Nat. Prod. Rep. 27, 637–657. doi: 10.1039/B906679A
Hillel, D. (2008). Soil biodiversity, in Soil in the Environment, ed D. Hillel. (San Diego, CA: Academic Press), 163–174. doi: 10.1016/B978-0-12-348536-6.50017-4
Ho, A., Ijaz, U. Z., Janssens, T. K., Ruijs, R., Kim, S. Y., De Boer, W., et al. (2017). Effects of bio-based residue amendments on greenhouse gas emission from agricultural soil are stronger than effects of soil type with different microbial community composition. GCB Bioenergy 9, 1707–1720. doi: 10.1111/gcbb.12457
Hu, T., Li, L., Li, Y., Liu, X., Zhang, T., Liu, J., et al. (2013). Effects of LCO and TH17 on the yield and protective enzyme activities of oat under drought stress. Acta Botanica Boreali-Occidentalia Sinica 33, 2451–2458.
Huang, A. C., Jiang, T., Liu, Y.-X., Bai, Y.-C., Reed, J., Qu, B., et al. (2019). A specialized metabolic network selectively modulates Arabidopsis root microbiota. Science 364:6389. doi: 10.1126/science.aau6389
Hussain, A., Arshad, M., Zahir, Z. A., and Asghar, M. (2015). Prospects of zinc solubilizing bacteria for enhancing growth of maize. Pak. J. Agri. Sci. 52:4. Available online at: https://pakjas.com.pk/papers/2499.pdf.
Hyyryläinen, H. L., Bolhuis, A., Darmon, E., Muukkonen, L., Koski, P., Vitikainen, M., et al. (2001). A novel two-component regulatory system in Bacillus subtilis for the survival of severe secretion stress. Mol. Microbiol. 41, 1159–1172. doi: 10.1046/j.1365-2958.2001.02576.x
Ijaz, F., Riaz, U., Iqbal, S., Zaman, Q. U., Ijaz, M. F., Javed, H., et al. (2019). Potential of rhizobium and PGPR to enhance growth and fodder yield of berseem (Trifolium alexandrinum L.) in the presence and absence of tryptamine. Pak. J. Agri. Res. 32:406. doi: 10.17582/journal.pjar/2019/32.2.398.406
Ipek, M., Arikan, S., Pirlak, L., and Eşitken, A. (2019). Sustainability of crop production by PGPR under abiotic stress conditions, in Plant Growth Promoting Rhizobacteria for Agricultural Sustainability, eds. A. Kumar, and V. Meena (Singapore: Springer), 293–314. doi: 10.1007/978-981-13-7553-8_15
Istina, I. N., Widiastuti, H., Joy, B., and Antralina, M. (2015). Phosphate-solubilizing microbe from Saprists peat soil and their potency to enhance oil palm growth and P uptake. Proc. Food Sci 3, 426–435. doi: 10.1016/j.profoo.2015.01.047
Janssens, C., Havlík, P., Krisztin, T., Baker, J., Frank, S., Hasegawa, T., et al. (2020). Global hunger and climate change adaptation through international trade. Nat. Clim. Change 10, 829–835. doi: 10.1038/s41558-020-0847-4
Jeanbille, M., Buée, M., Bach, C., Cébron, A., Frey-Klett, P., Turpault, M. P., et al. (2016). Soil parameters drive the structure, diversity and metabolic potentials of the bacterial communities across temperate beech forest soil sequences. Microb. Ecol. 71, 482–493. doi: 10.1007/s00248-015-0669-5
Jha, C. K., and Saraf, M. (2015). Plant growth promoting rhizobacteria (PGPR): a review. J. Agri. Res. Dev. 5, 108–119.
Kamran, S., Shahid, I., Baig, D. N., Rizwan, M., Malik, K. A., and Mehnaz, S. (2017). Contribution of zinc solubilizing bacteria in growth promotion and zinc content of wheat. Front. Microbiol. 8:2593. doi: 10.3389/fmicb.2017.02593
Kan, J., Fang, R., and Jia, Y. (2017). Interkingdom signaling in plant-microbe interactions. Sci. China Life Sci. 60, 785–796. doi: 10.1007/s11427-017-9092-3
Kang, N. Y., Cho, C., Kim, N. Y., and Kim, J. (2012). Cytokinin receptor-dependent and receptor-independent pathways in the dehydration response of Arabidopsis thaliana. J. Plant Physiol. 169, 1382–1391. doi: 10.1016/j.jplph.2012.05.007
Kang, S.-M., Khan, A. L., You, Y.-H., Kim, J.-G., Kamran, M., and Lee, I.-J. (2014). Gibberellin production by newly isolated strain Leifsonia soli SE134 and its potential to promote plant growth. J. Microbiol. Biotechnol. 24, 106–112. doi: 10.4014/jmb.1304.04015
Kanwal, S., Ilyas, N., Batool, N., and Arshad, M. (2017). Amelioration of drought stress in wheat by combined application of PGPR, compost, and mineral fertilizer. J. Plant Nutr. 40, 1250–1260. doi: 10.1080/01904167.2016.1263322
Karthiba, L., Saveetha, K., Suresh, S., Raguchander, T., Saravanakumar, D., and Samiyappan, R. (2010). PGPR and entomopathogenic fungus bioformulation for the synchronous management of leaffolder pest and sheath blight disease of rice. Pest Manage. Sci. 66, 555–564. doi: 10.1002/ps.1907
Kashyap, B. K., Solanki, M. K., Pandey, A. K., Prabha, S., Kumar, P., and Kumari, B. (2019). Bacillus as plant growth promoting rhizobacteria (PGPR): a promising green agriculture technology, in Plant Health Under Biotic Stress (Springer), 219–236. doi: 10.1007/978-981-13-6040-4_11
Kashyap, P. L., Srivastava, A. K., Tiwari, S. P., and Kumar, S. (2018). Microbes for Climate Resilient Agriculture. Hoboken,NJ: John Wiley & Sons.
Kaushal, M., and Wani, S. P. (2016). Plant-growth-promoting rhizobacteria: drought stress alleviators to ameliorate crop production in drylands. Ann. Microbiol. 66, 35–42. doi: 10.1007/s13213-015-1112-3
Keet, J.-H., Ellis, A. G., Hui, C., and Le Roux, J. J. (2017). Legume–rhizobium symbiotic promiscuity and effectiveness do not affect plant invasiveness. Ann. Bot. 119, 1319–1331. doi: 10.1093/aob/mcx028
Keshavarz Zarjani, J., Aliasgharzad, N., Oustan, S., Emadi, M., and Ahmadi, A. (2013). Isolation and characterization of potassium solubilizing bacteria in some Iranian soils. Arch. Agronomy Soil Sci. 59, 1713–1723. doi: 10.1080/03650340.2012.756977
Khan, A. A., Jilani, G., Akhtar, M. S., Naqvi, S. M. S., and Rasheed, M. (2009). Phosphorus solubilizing bacteria: occurrence, mechanisms and their role in crop production. J. Agric. Biol. Sci 1, 48–58.
Khan, N., Bano, A., Ali, S., and Babar, M. A. (2020). Crosstalk amongst phytohormones from planta and PGPR under biotic and abiotic stresses. Plant Growth Regul. 90, 189–203. doi: 10.1007/s10725-020-00571-x
Khandelwal, S., Manwar, A., Chaudhari, B., and Chincholkar, S. (2002). Siderophoregenic bradyrhizobia boost yield of soybean. Appl. Biochem. Biotechnol. 102, 155–168. doi: 10.1385/ABAB:102-103:1-6:155
Khare, E., and Arora, N. K. (2015). Effects of soil environment on field efficacy of microbial inoculants, in Plant Microbes Symbiosis: Applied Facets, eds. N. Arora (New Delhi: Springer), 353–381. doi: 10.1007/978-81-322-2068-8_19
Kim, Y. C., Jung, H., Kim, K. Y., and Park, S. K. (2008). An effective biocontrol bioformulation against Phytophthora blight of pepper using growth mixtures of combined chitinolytic bacteria under different field conditions. Eur. J. Plant Pathol. 120, 373–382. doi: 10.1007/s10658-007-9227-4
Krämer, U., and Clemens, S. (2005). Functions and homeostasis of zinc, copper, and nickel in plants, in Molecular Biology of Metal Homeostasis and Detoxification, eds. M. J. Tamas, and E. Martinoia (Berlin; Heidelberg: Springer), 14, 215–271. doi: 10.1007/4735_96
Kudoyarova, G. R., Melentiev, A. I., Martynenko, E. V., Timergalina, L. N., Arkhipova, T. N., Shendel, G. V., et al. (2014). Cytokinin producing bacteria stimulate amino acid deposition by wheat roots. Plant Physiol. Biochem. 83, 285–291. doi: 10.1016/j.plaphy.2014.08.015
Kumar, A., Patel, J. S., Meena, V. S., and Srivastava, R. (2019). Recent advances of PGPR based approaches for stress tolerance in plants for sustainable agriculture. Biocatal. Agri. Biotechnol. 20:101271. doi: 10.1016/j.bcab.2019.101271
Kumar, S., Bauddh, K., Barman, S., and Singh, R. P. (2014). Amendments of microbial biofertilizers and organic substances reduces requirement of urea and DAP with enhanced nutrient availability and productivity of wheat (Triticum aestivum L.). Ecol. Eng. 71, 432–437. doi: 10.1016/j.ecoleng.2014.07.007
Kumar, S., Chauhan, P. S., Agrawal, L., Raj, R., Srivastava, A., Gupta, S., et al. (2016). Paenibacillus lentimorbus inoculation enhances tobacco growth and extenuates the virulence of Cucumber mosaic virus. PLoS ONE 11:e0149980. doi: 10.1371/journal.pone.0149980
Kunikowska, A., Byczkowska, A., Doniak, M., and Kazmierczak, A. (2013). Cytokinins resume: their signaling and role in programmed cell death in plants. Plant Cell Rep. 32, 771–780. doi: 10.1007/s00299-013-1436-z
Kutman, U. B., Yildiz, B., Ozturk, L., and Cakmak, I. (2010). Biofortification of durum wheat with zinc through soil and foliar applications of nitrogen. Cereal. Chem. 87, 1–9. doi: 10.1094/CCHEM-87-1-0001
Labuschagne, N., Pretorius, T., and Idris, A. (2010). Plant growth promoting rhizobacteria as biocontrol agents against soil-borne plant diseases, in Plant Growth and Health Promoting Bacteria, eds. D. Maheshwari (Berlin; Heidelberg: Springer), 211–230. doi: 10.1007/978-3-642-13612-2_9
Lee, K. D., Gray, E. J., Mabood, F., Jung, W.-J., Charles, T., Clark, S. R., et al. (2009). The class IId bacteriocin thuricin-17 increases plant growth. Planta 229, 747–755. doi: 10.1007/s00425-008-0870-6
Lipper, L., Thornton, P., Campbell, B. M., Baedeker, T., Braimoh, A., Bwalya, M., et al. (2014). Climate-smart agriculture for food security. Nat. Clim. Change 4, 1068–1072. doi: 10.1038/nclimate2437
Liu, X. M., Xu, Q. L., Li, Q. Q., Zhang, H., and Xiao, J. X. (2017). Physiological responses of the two blueberry cultivars to inoculation with an arbuscular mycorrhizal fungus under low-temperature stress. J. Plant Nutr. 40, 2562–2570. doi: 10.1080/01904167.2017.1380823
Lobell, D. B., and Field, C. B. (2007). Global scale climate–crop yield relationships and the impacts of recent warming. Environ. Res. Lett. 2:014002.
Lobell, D. B., Schlenker, W., and Costa-Roberts, J. (2011). Climate trends and global crop production since 1980. Science 333, 616–620. doi: 10.1126/science.1204531
Lyu, D., Backer, R., Subramanian, S., and Smith, D. L. (2020). Phytomicrobiome coordination signals hold potential for climate change-resilient agriculture. Front. Plant Sci. 11:634. doi: 10.3389/fpls.2020.00634
Lyu, D., Zajonc, J., Pagé, A., Tanney, C. A., Shah, A., Monjezi, N., et al. (2021). Plant Holobiont Theory: The Phytomicrobiome Plays a Central Role in Evolution and Success. Microorganisms 9, 675. doi: 10.3390/microorganisms9040675
Ma, J., Sun, C., Bai, L., Dong, R., Yan, Y., Yu, X., et al. (2018). Transcriptome analysis of cucumber roots reveals key cold-resistance genes induced by AM fungi. Plant Mol. Biol. Rep. 36, 135–148. doi: 10.1007/s11105-018-1066-2
Mabood, F., Zhou, X., and Smith, D. L. (2014). Microbial signaling and plant growth promotion. Canad. J. Plant Sci. 94, 1051–1063. doi: 10.4141/cjps2013-148
Maheshwari, D. K., Dheeman, S., and Agarwal, M. (2015). Phytohormone-Producing PGPR for sustainable agriculture, in Bacterial Metabolites in Sustainable Agroecosystem, ed. D. K. Maheshwari (Cham: Springer International Publishing), 159–182. doi: 10.1007/978-3-319-24654-3_7
Martínez-Medina, A., Van Wees, S. C., and Pieterse, C. M. (2017). Airborne signals from Trichoderma fungi stimulate iron uptake responses in roots resulting in priming of jasmonic acid-dependent defences in shoots of Arabidopsis thaliana and Solanum lycopersicum. Plant Cell Environ. 40, 2691–2705. doi: 10.1111/pce.13016
Martinuz, A., Schouten, A., Menjivar, R., and Sikora, R. (2012). Effectiveness of systemic resistance toward Aphis gossypii (Hom., Aphididae) as induced by combined applications of the endophytes Fusarium oxysporum Fo162 and Rhizobium etli G12. Biol. Cont. 62, 206–212. doi: 10.1016/j.biocontrol.2012.05.006
Mayak, S., Tirosh, T., and Glick, B. R. (2004). Plant growth-promoting bacteria confer resistance in tomato plants to salt stress. Plant Physiol. Biochem. 42, 565–572. doi: 10.1016/j.plaphy.2004.05.009
Mazumdaru, S. (2018). Calls for Farm Support Intensify as Europe Struggles With Heat wave, Drought. Available online at: https://www.dw.com/en/calls-for-farm-support-intensify-as-europe-struggles-with-heat-wave-drought/a-44902321 (accessed May 16, 2021).
Mcnear, D. H. (2013). The rhizosphere-roots, soil and everything in between. Nat. Educ. Knowl. 4:1. Available online at: http://www.nature.com/scitable/knowledge/library/the-rhizosphere-roots-soil-and-67500617
Mehnaz, S. (2016). An overview of globally available bioformulations. Bioformulat. Sustain. Agri. 267–281. doi: 10.1007/978-81-322-2779-3_15
Mishra, J., Singh, R., and Arora, N. K. (2017). Alleviation of heavy metal stress in plants and remediation of soil by rhizosphere microorganisms. Front. Microbiol. 8:1706. doi: 10.3389/fmicb.2017.01706
Mishra, S., Singh, A., Keswani, C., Saxena, A., Sarma, B., and Singh, H. (2015). Harnessing plant-microbe interactions for enhanced protection against phytopathogens, in Plant Microbes Symbiosis: Applied Facets, eds. N. Arora (New Delhi: Springer), 111–125. doi: 10.1007/978-81-322-2068-8_5
Mishra, V., Ellouze, W., and Howard, R. J. (2018). Utility of arbuscular mycorrhizal fungi for improved production and disease mitigation in organic and hydroponic greenhouse crops. J. Horticult. 5:3. doi: 10.4172/2376-0354.1000237
Mittler, R. (2006). Abiotic stress, the field environment and stress combination. Trends Plant Sci. 11, 15–19. doi: 10.1016/j.tplants.2005.11.002
Moser, R., Pertot, I., Elad, Y., and Raffaelli, R. (2008). Farmers' attitudes toward the use of biocontrol agents in IPM strawberry production in three countries. Biol. Contr. 47, 125–132. doi: 10.1016/j.biocontrol.2008.07.012
Moussa, T., Almaghrabi, O., and Abdel-Moneim, T. (2013). Biological control of the wheat root rot caused by Fusarium graminearum using some PGPR strains in Saudi Arabia. Ann. Appl. Biol. 163, 72–81. doi: 10.1111/aab.12034
Muentz, A. (1890). Sur la decomposition des roches et la formation de la terre arable. CR Acad Sci 110, 1370–1372.
Mushtaq, Z. (2020). PGPR: present role, mechanism of action and future prospects along bottlenecks in commercialization. EQA-Int. J. Environ. Q. 41, 9–15. doi: 10.6092/issn.2281-4485/11103
Myresiotis, C. K., Vryzas, Z., and Papadopoulou-Mourkidou, E. (2015). Effect of specific plant-growth-promoting rhizobacteria (PGPR) on growth and uptake of neonicotinoid insecticide thiamethoxam in corn (Zea mays L.) seedlings. Pest Manag. Sci. 71, 1258–1266. doi: 10.1002/ps.3919
Naamala, J., Jaiswal, S. K., and Dakora, F. D. (2016). Microsymbiont diversity and phylogeny of native bradyrhizobia associated with soybean (Glycine max L. Merr.) nodulation in South African soils. Syst. Appl. Microbiol. 39, 336–344. doi: 10.1016/j.syapm.2016.05.009
Naamala, J., and Smith, D. L. (2020). Relevance of plant growth promoting microorganisms and their derived compounds, in the face of climate change. Agronomy 10:1179. doi: 10.3390/agronomy10081179
Nakkeeran, S., Fernando, W. D., and Siddiqui, Z. A. (2005). Plant growth promoting rhizobacteria formulations and its scope in commercialization for the management of pests and diseases, in PGPR: Biocontrol and biofertilization (Springer), 257–296. doi: 10.1007/1-4020-4152-7_10
Naseem, M., Wolfling, M., and Dandekar, T. (2014). Cytokinins for immunity beyond growth, galls and green islands. Trends Plant Sci. 19, 481–484. doi: 10.1016/j.tplants.2014.04.001
Naz, I., Asghari, B., and Tamoor-Ul-Hassan. (2009). Isolation of phytohormones producing plant growth promoting rhizobacteria from weeds growing in Khewra salt range, Pakistan and their implication in providing salt tolerance to Glycine max L. Afr. J. Biotechnol. 8:1176. doi: 10.5897/AJB09.1176
Nazari, M., and Smith, D. L. (2020). A PGPR-produced bacteriocin for sustainable agriculture: a review of thuricin 17 characteristics and applications. Front. Plant Sci. 11:916. doi: 10.3389/fpls.2020.00916
Neina, D. (2019). The role of soil pH in plant nutrition and soil remediation. Appl. Environ. Soil Sci. 2019:5794869. doi: 10.1155/2019/5794869
Nelson, G. C., Valin, H., Sands, R. D., Havlík, P., Ahammad, H., Deryng, D., et al. (2014). Climate change effects on agriculture: economic responses to biophysical shocks. Proc. Natl. Acad. Sci. U.S.A. 111, 3274–3279. doi: 10.1073/pnas.1222465110
Ng, L., Sariah, M., Sariam, O., Radziah, O., and Abidin, M. Z. (2016). PGPM-induced defense-related enzymes in aerobic rice against rice leaf blast caused by Pyricularia oryzae. Eur. J. Plant Pathol. 145, 167–175. doi: 10.1007/s10658-015-0826-1
Nowak, R., and Caldwell, M. (1984). A test of compensatory photosynthesis in the field: implications for herbivory tolerance. Oecologia 61, 311–318. doi: 10.1007/BF00379627
Obata, T., and Fernie, A. R. (2012). The use of metabolomics to dissect plant responses to abiotic stresses. Cell. Mol. Life Sci. 69, 3225–3243. doi: 10.1007/s00018-012-1091-5
Ojuederie, O. B., and Babalola, O. O. (2017). Microbial and plant-assisted bioremediation of heavy metal polluted environments: a review. Int. J. Environ. Res. Public Health 14:1504. doi: 10.3390/ijerph14121504
Ortíz-Castro, R., Contreras-Cornejo, H. A., Macías-Rodríguez, L., and López-Bucio, J. (2009). The role of microbial signals in plant growth and development. Plant signaling & behavior 4, 701–712. doi: 10.4161/psb.4.8.9047
Pan, B., and Smith, D. (1998). Genistein and daidzein concentrations and contents in seedling roots of three soybean cultivars grown under three root zone temperatures. J. Agronomy Crop Sci. 180, 77–82. doi: 10.1111/j.1439-037X.1998.tb00374.x
Pangesti, N., Weldegergis, B. T., Langendorf, B., Van Loon, J. J., Dicke, M., and Pineda, A. (2015). Rhizobacterial colonization of roots modulates plant volatile emission and enhances the attraction of a parasitoid wasp to host-infested plants. Oecologia 178, 1169–1180. doi: 10.1007/s00442-015-3277-7
Pareek, A., Dhankher, O. P., and Foyer, C. H. (2020). Mitigating the Impact of Climate Change on Plant Productivity and Ecosystem Sustainability. Oxford: Oxford University Press UK. doi: 10.1093/jxb/erz518
Passari, A. K., Upadhyaya, K., Singh, G., Abdel-Azeem, A. M., Thankappan, S., Uthandi, S., et al. (2019). Enhancement of disease resistance, growth potential, and photosynthesis in tomato (Solanum lycopersicum) by inoculation with an endophytic actinobacterium, Streptomyces thermocarboxydus strain BPSAC147. PLoS ONE 14:e0219014. doi: 10.1371/journal.pone.0219014
Pastor, A., Palazzo, A., Havlik, P., Biemans, H., Wada, Y., Obersteiner, M., et al. (2019). The global nexus of food–trade–water sustaining environmental flows by 2050. Nat. Sustainab. 2, 499–507. doi: 10.1038/s41893-019-0287-1
Pedranzani, H., Rodríguez-Rivera, M., Gutiérrez, M., Porcel, R., Hause, B., and Ruiz-Lozano, J. M. (2016). Arbuscular mycorrhizal symbiosis regulates physiology and performance of Digitaria eriantha plants subjected to abiotic stresses by modulating antioxidant and jasmonate levels. Mycorrhiza 26, 141–152. doi: 10.1007/s00572-015-0653-4
Pep, C. (2019). Nitrogen Fertilisers Are Incredibly Efficient, But They Make Climate Change a Lot Worse. Available online at: https://theconversation.com/nitrogen-fertilisers-are-incredibly-efficient-but-they-make-climate-change-a-lot-worse-127103 (accessed January 1, 2021).
Pereira, S. I. A., Monteiro, C., Vega, A. L., and Castro, P. M. L. (2016). Endophytic culturable bacteria colonizing Lavandula dentata L. plants: Isolation, characterization and evaluation of their plant growth-promoting activities. Ecol. Eng. 87, 91–97. doi: 10.1016/j.ecoleng.2015.11.033
Pérez-Montaño, F., Alías-Villegas, C., Bellogín, R., Del Cerro, P., Espuny, M., Jiménez-Guerrero, I., et al. (2014). Plant growth promotion in cereal and leguminous agricultural important plants: from microorganism capacities to crop production. Microbiol. Res. 169, 325–336. doi: 10.1016/j.micres.2013.09.011
Pham, V. H., and Kim, J. (2012). Cultivation of unculturable soil bacteria. Trends Biotechnol. 30, 475–484. doi: 10.1016/j.tibtech.2012.05.007
Piccoli, P., and Bottini, R. (2013). Abiotic stress tolerance induced by endophytic PGPR, in Symbiotic Endophytes, eds. R. Aroca (Berlin; Heidelberg: Springer), 151–163. doi: 10.1007/978-3-642-39317-4_8
Prajapati, K., and Modi, H. (2012). Isolation and characterization of potassium solubilizing bacteria from ceramic industry soil. CIBTech J. Microbiol. 1, 8–14.
Prajapati, K., Sharma, M., and Modi, H. (2013). Growth promoting effect of potassium solubilizing microorganisms on Abelmoscus esculantus. Int. J. Agric. Sci. 3, 181–188.
Prithiviraj, B., Zhou, X., Souleimanov, A., Khan, W. M., and Smith, D. L. (2003). A host-specific bacteria-to-plant signal molecule (Nod factor) enhances germination and early growth of diverse crop plants. Planta 216, 437–445. doi: 10.1007/s00425-002-0928-9
Pugashetti, B., Angle, J., and Wagner, G. (1982). Soil microorganisms antagonistic towards Rhizobium japonicum. Soil Biol. Biochem. 14, 45–49. doi: 10.1016/0038-0717(82)90075-X
Puissant, J., Jones, B., Goodall, T., Mang, D., Blaud, A., Gweon, H. S., et al. (2019). The pH optimum of soil exoenzymes adapt to long term changes in soil pH. Soil Biol. Biochem. 138:107601. doi: 10.1016/j.soilbio.2019.107601
Qiao, Q., Wang, F., Zhang, J., Chen, Y., Zhang, C., Liu, G., et al. (2017). The variation in the rhizosphere microbiome of cotton with soil type, genotype and developmental stage. Sci. Rep. 7, 1–10. doi: 10.1038/s41598-017-04213-7
Qin, Y., Druzhinina, I. S., Pan, X., and Yuan, Z. (2016). Microbially mediated plant salt tolerance and microbiome-based solutions for saline agriculture. Biotechnol. Adv. 34, 1245–1259. doi: 10.1016/j.biotechadv.2016.08.005
Raj, D., Linda, R., and Babyson, R. S. (2014). Molecular characterization of phosphate solubilizing bacteria (PSB) and plant growth promoting rhizobacteria (PGPR) from pristine soil. Int. J. Innov. Sci. Eng. Technol. 1, 317–324.
Recep, K., Fikrettin, S., Erkol, D., and Cafer, E. (2009). Biological control of the potato dry rot caused by Fusarium species using PGPR strains. Biol. Cont. 50, 194–198. doi: 10.1016/j.biocontrol.2009.04.004
Reetha, A. K., Pavani, S. L., and Mohan, S. (2014). Hydrogen cyanide production ability by bacterial antagonist and their antibiotics inhibition potential on Macrophomina phaseolina (Tassi.) Goid. Int. J. Curr. Microbiol. Appl. Sci. 3, 172–178. Available online at: http://www.ijcmas.com/vol-3-5/A.Karmel%20Reetha,%20et%20al.pdf
Reinhold-Hurek, B., Bünger, W., Burbano, C. S., Sabale, M., and Hurek, T. (2015). Roots shaping their microbiome: global hotspots for microbial activity. Annu. Rev. Phytopathol. 53, 403–424. doi: 10.1146/annurev-phyto-082712-102342
Rezanka, T., Palyzová, A., and Sigler, K. (2018). Isolation and identification of siderophores produced by cyanobacteria. Folia Microbiol. 63, 569–579. doi: 10.1007/s12223-018-0626-z
Riaz, U., Murtaza, G., Anum, W., Samreen, T., Sarfraz, M., and Nazir, M. Z. (2021). Plant Growth-Promoting Rhizobacteria (PGPR) as biofertilizers and biopesticides,: in Microbiota and Biofertilizers: A Sustainable Continuum for Plant and Soil Health, eds K. R. Hakeem, G. H. Dar, M. A. Mehmood, and R. A. Bhat (Cham: Springer International Publishing), 181–196. doi: 10.1007/978-3-030-48771-3_11
Richardson, Y., Blin, J., and Julbe, A. (2012). A short overview on purification and conditioning of syngas produced by biomass gasification: catalytic strategies, process intensification and new concepts. Prog. Energy Combustion Sci. 38, 765–781. doi: 10.1016/j.pecs.2011.12.001
Roe, A. J., Mclaggan, D., Davidson, I., O'byrne, C., and Booth, I. R. (1998). Perturbation of anion balance during inhibition of growth of Escherichia coli by weak acids. J. Bacteriol. 180, 767–772. doi: 10.1128/JB.180.4.767-772.1998
Romanyà, J., and Casals, P. (2019). Biological nitrogen fixation response to soil fertility is species-dependent in annual legumes. J. Soil Sci. Plant Nutr. 20, 546–556. doi: 10.1007/s42729-019-00144-6
Romera, F. J., García, M. J., Lucena, C., Martínez-Medina, A., Aparicio, M. A., Ramos, J., et al. (2019). Induced systemic resistance (ISR) and Fe deficiency responses in dicot plants. Front. Plant Sci. 10:287. doi: 10.3389/fpls.2019.00287
Ryan, R. P., An, S.-Q., Allan, J. H., Mccarthy, Y., and Dow, J. M. (2015). The DSF family of cell–cell signals: an expanding class of bacterial virulence regulators. PLoS Pathog. 11:e1004986. doi: 10.1371/journal.ppat.1004986
Sadeghzadeh, B. (2013). A review of zinc nutrition and plant breeding. J. Soil Sci. Plant Nutr. 13, 905–927. doi: 10.4067/S0718-95162013005000072
Saha, M., Sarkar, S., Sarkar, B., Sharma, B. K., Bhattacharjee, S., and Tribedi, P. (2016). Microbial siderophores and their potential applications: a review. Environ. Sci. Pollut. Res. 23, 3984–3999. doi: 10.1007/s11356-015-4294-0
Saha, R., Saha, N., Donofrio, R. S., and Bestervelt, L. L. (2013). Microbial siderophores: a mini review. J. Basic Microbiol. 53, 303–317. doi: 10.1002/jobm.201100552
Saharan, B., and Nehra, V. (2011). Plant growth promoting rhizobacteria: a critical review. Life Sci. Med. Res. 21:30.
Sandhya, V., Ali, S. Z., Grover, M., Reddy, G., and Venkateswarlu, B. (2010). Effect of plant growth promoting Pseudomonas spp. on compatible solutes, antioxidant status and plant growth of maize under drought stress. Plant Growth Regul. 62, 21–30. doi: 10.1007/s10725-010-9479-4
Sandy, M., and Butler, A. (2009). Microbial iron acquisition: marine and terrestrial siderophores. Chem. Rev. 109, 4580–4595. doi: 10.1021/cr9002787
Sangeeth, K., Bhai, R. S., and Srinivasan, V. (2012). Paenibacillus glucanolyticus, a promising potassium solubilizing bacterium isolated from black pepper (Piper nigrum L.) rhizosphere. J. Spices Aromatic Crops 21, 118–124.
Santhanam, R., Weinhold, A., Goldberg, J., Oh, Y., and Baldwin, I. T. (2015). Native root-associated bacteria rescue a plant from a sudden-wilt disease that emerged during continuous cropping. Proc. Natl. Acad. Sci. U.S.A. 112, E5013–E5020. doi: 10.1073/pnas.1505765112
Saravanan, V., Kumar, M. R., and Sa, T. (2011). Microbial zinc solubilization and their role on plants, in Bacteria in Agrobiology: Plant Nutrient Management, eds. D. Maheshwari (Berlin; Heidelberg: Springer), 47–63. doi: 10.1007/978-3-642-21061-7_3
Sarkar, J., Chakraborty, B., and Chakraborty, U. (2018). Plant growth promoting rhizobacteria protect wheat plants against temperature stress through antioxidant signalling and reducing chloroplast and membrane injury. J. Plant Growth Regul. 37, 1396–1412. doi: 10.1007/s00344-018-9789-8
Schausberger, P., Peneder, S., Jürschik, S., and Hoffmann, D. (2012). Mycorrhiza changes plant volatiles to attract spider mite enemies. Funct. Ecol. 26, 441–449. doi: 10.1111/j.1365-2435.2011.01947.x
Schindlbacher, A., Rodler, A., Kuffner, M., Kitzler, B., Sessitsch, A., and Zechmeister-Boltenstern, S. (2011). Experimental warming effects on the microbial community of a temperate mountain forest soil. Soil Biol. Biochem. 43, 1417–1425. doi: 10.1016/j.soilbio.2011.03.005
Schwinghamer, T., Souleimanov, A., Dutilleul, P., and Smith, D. (2016a). Supplementation with solutions of lipo-chitooligosacharide Nod Bj V (C18: 1, MeFuc) and thuricin 17 regulates leaf arrangement, biomass, and root development of canola (Brassica napus [L.]). Plant Growth Regul. 78, 31–41. doi: 10.1007/s10725-015-0072-8
Schwinghamer, T., Souleimanov, A., Dutilleul, P., and Smith, D. L. (2016b). The response of canola cultivars to lipo-chitooligosaccharide (Nod Bj V [C18: 1, MeFuc]) and thuricin 17. Plant Growth Regul. 78, 421–434. doi: 10.1007/s10725-015-0104-4
Senthilraja, G., Anand, T., Kennedy, J., Raguchander, T., and Samiyappan, R. (2013). Plant growth promoting rhizobacteria (PGPR) and entomopathogenic fungus bioformulation enhance the expression of defense enzymes and pathogenesis-related proteins in groundnut plants against leafminer insect and collar rot pathogen. Physiol. Mol. Plant Pathol. 82, 10–19. doi: 10.1016/j.pmpp.2012.12.002
Setiawati, T. C., and Mutmainnah, L. (2016). Solubilization of potassium containing mineral by microorganisms from sugarcane rhizosphere. Agri. Agri. Sci. Proc. 9, 108–117. doi: 10.1016/j.aaspro.2016.02.134
Sgroy, V., Cassan, F., Masciarelli, O., Del Papa, M. F., Lagares, A., and Luna, V. (2009). Isolation and characterization of endophytic plant growth-promoting (PGPB) or stress homeostasis-regulating (PSHB) bacteria associated to the halophyte Prosopis strombulifera. Appl. Microbiol. Biotechnol. 85, 371–381. doi: 10.1007/s00253-009-2116-3
Shah, A., and Smith, D. L. (2020). Flavonoids in agriculture: chemistry and roles in, biotic and abiotic stress responses, and microbial associations. Agronomy 10:1209. doi: 10.3390/agronomy10081209
Shanmugaiah, V., Nithya, K., Harikrishnan, H., Jayaprakashvel, M., and Balasubramanian, N. (2015). Biocontrol mechanisms of siderophores against bacterial plant pathogens. Sustain. Approach. Control. Plant Pathog. Bacteria 24, 167–190.
Sharma, P., Kumawat, K., and Kaur, S. (2016). Plant growth promoting rhizobacteria in nutrient enrichment: current perspectives, in Biofortification of Food Crops, eds. U. Singh, C. Praharaj, S. Singh, and N. Singh (New Delhi: Springer), 263–289. doi: 10.1007/978-81-322-2716-8_20
Sharma, S. B., Sayyed, R. Z., Trivedi, M. H., and Gobi, T. A. (2013). Phosphate solubilizing microbes: sustainable approach for managing phosphorus deficiency in agricultural soils. SpringerPlus 2:587. doi: 10.1186/2193-1801-2-587
Shen, C., Xiong, J., Zhang, H., Feng, Y., Lin, X., Li, X., et al. (2013). Soil pH drives the spatial distribution of bacterial communities along elevation on Changbai Mountain. Soil Biol. Biochem. 57, 204–211. doi: 10.1016/j.soilbio.2012.07.013
Sheng, X. F., and He, L. Y. (2006). Solubilization of potassium-bearing minerals by a wild-type strain of Bacillus edaphicus and its mutants and increased potassium uptake by wheat. Can. J. Microbiol. 52, 66–72. doi: 10.1139/w05-117
Shrivastava, P., and Kumar, R. (2015). Soil salinity: a serious environmental issue and plant growth promoting bacteria as one of the tools for its alleviation. Saudi J. Biol. Sci. 22, 123–131. doi: 10.1016/j.sjbs.2014.12.001
Siddiqui, I. A., Shaukat, S. S., Sheikh, I. H., and Khan, A. (2006). Role of cyanide production by Pseudomonas fluorescens CHA0 in the suppression of root-knot nematode, Meloidogyne javanica in tomato. World J. Microbiol. Biotechnol. 22, 641–650. doi: 10.1007/s11274-005-9084-2
Simon, J.-C., Marchesi, J. R., Mougel, C., and Selosse, M.-A. (2019). Host-microbiota interactions: from holobiont theory to analysis. Microbiome 7, 1–5. doi: 10.1186/s40168-019-0619-4
Sindhu, S., Dahiya, A., Gera, R., and Sindhu, S. S. (2020). Mitigation of abiotic stress in legume-nodulating rhizobia for sustainable crop production. Agri. Res. 9:444. doi: 10.1007/s40003-020-00474-3
Smith, D. L., Praslickova, D., and Ilangumaran, G. (2015). Inter-organismal signaling and management of the phytomicrobiome. Front. Plant Sci. 6:722. doi: 10.3389/fpls.2015.00722
Smith, P., Haberl, H., Popp, A., Erb, K. H., Lauk, C., Harper, R., et al. (2013). How much land-based greenhouse gas mitigation can be achieved without compromising food security and environmental goals? Global Change Biol. 19, 2285–2302. doi: 10.1111/gcb.12160
Sood, G., Kaushal, R., Chauhan, A., and Gupta, S. (2018). Effect of conjoint application of indigenous PGPR and chemical fertilizers on productivity of maize (Zea mays L.) under mid hills of Himachal Pradesh. J. Plant Nutr. 41, 297–303. doi: 10.1080/01904167.2017.1381116
Spaepen, S., Vanderleyden, J., and Remans, R. (2007). Indole-3-acetic acid in microbial and microorganism-plant signaling. FEMS Microbiol. Rev. 31, 425–448. doi: 10.1111/j.1574-6976.2007.00072.x
Spallek, T., Gan, P., Kadota, Y., and Shirasu, K. (2018). Same tune, different song-cytokinins as virulence factors in plant-pathogen interactions? Curr. Opin. Plant Biol. 44, 82–87. doi: 10.1016/j.pbi.2018.03.002
Srinivasan, K., and Mathivanan, N. (2011). Plant growth promoting microbial consortia mediated classical biocontrol of sunflower necrosis virus disease. J. Biopestic. 4:65.
Srinivasan, R., Yandigeri, M. S., Kashyap, S., and Alagawadi, A. R. (2012). Effect of salt on survival and P-solubilization potential of phosphate solubilizing microorganisms from salt affected soils. Saudi J. Biol. Sci. 19, 427–434. doi: 10.1016/j.sjbs.2012.05.004
Srivastava, S., Bist, V., Srivastava, S., Singh, P. C., Trivedi, P. K., Asif, M. H., et al. (2016). Unraveling aspects of Bacillus amyloliquefaciens mediated enhanced production of rice under biotic stress of Rhizoctonia solani. Front. Plant Sci. 7:587. doi: 10.3389/fpls.2016.00587
Subramanian, K., Tenshia, V., Jayalakshmi, K., and Ramachandran, V. (2009). Role of arbuscular mycorrhizal fungus (Glomus intraradices)–(fungus aided) in zinc nutrition of maize. J. Agri. Biotechnol. Sustain. Dev. 1, 29–38. doi: 10.5897/JABSD.9000037
Subramanian, S., and Smith, D. L. (2015). Bacteriocins from the rhizosphere microbiome – from an agriculture perspective. Front. Plant Sci. 6:909. doi: 10.3389/fpls.2015.00909
Subramanian, S., Souleimanov, A., and Smith, D. L. (2016). Proteomic studies on the effects of lipo-chitooligosaccharide and thuricin 17 under unstressed and salt stressed conditions in Arabidopsis thaliana. Front. Plant Sci. 7:1314. doi: 10.3389/fpls.2016.01314
Sundara, B., Natarajan, V., and Hari, K. (2002). Influence of phosphorus solubilizing bacteria on the changes in soil available phosphorus and sugarcane and sugar yields. Field Crops Res. 77, 43–49. doi: 10.1016/S0378-4290(02)00048-5
Swarnalakshmi, K., Yadav, V., Tyagi, D., Dhar, D. W., Kannepalli, A., and Kumar, S. (2020). Significance of plant growth promoting rhizobacteria in grain legumes: growth promotion and crop production. Plants (Basel) 9:596. doi: 10.3390/plants9111596
Tabassum, B., Khan, A., Tariq, M., Ramzan, M., Khan, M. S. I., Shahid, N., et al. (2017). Bottlenecks in commercialisation and future prospects of PGPR. Appl. Soil Ecol. 121, 102–117. doi: 10.1016/j.apsoil.2017.09.030
Tajini, F., Trabelsi, M., and Drevon, J.-J. (2012). Combined inoculation with Glomus intraradices and Rhizobium tropici CIAT899 increases phosphorus use efficiency for symbiotic nitrogen fixation in common bean (Phaseolus vulgaris L.). Saudi J. Biol. Sci. 19, 157–163. doi: 10.1016/j.sjbs.2011.11.003
Tak, H. I., Ahmad, F., Babalola, O., and Inam, A. (2012). Growth, photosynthesis and yield of chickpea as influenced by urban wastewater and different levels of phosphorus. Int. J. Plant Res. 2, 6–13. doi: 10.5923/j. plant.20120202.02
Takishita, Y., Charron, J.-B., and Smith, D. L. (2018). Biocontrol rhizobacterium Pseudomonas sp. 23S induces systemic resistance in tomato (Solanum lycopersicum L.) against bacterial canker Clavibacter michiganensis subsp. michiganensis. Front. Microbiol. 9:2119. doi: 10.3389/fmicb.2018.02119
Tariq, M., Hameed, S., Malik, K. A., and Hafeez, F. Y. (2007). Plant root associated bacteria for zinc mobilization in rice. Pak. J. Botany 39:245.
Tian, F., Ding, Y., Zhu, H., Yao, L., and Du, B. (2009). Genetic diversity of siderophore-producing bacteria of tobacco rhizosphere. Brazil. J. Microbiol. 40, 276–284. doi: 10.1590/S1517-83822009000200013
Toklikishvili, N., Dandurishvili, N., Vainstein, A., Tediashvili, M., Giorgobiani, N., Lurie, S., et al. (2010). Inhibitory effect of ACC deaminase-producing bacteria on crown gall formation in tomato plants infected by Agrobacterium tumefaciens or A. vitis. Plant Pathol. 59, 1023–1030. doi: 10.1111/j.1365-3059.2010.02326.x
Tripathi, D. K., Singh, S., Gaur, S., Singh, S., Yadav, V., Liu, S., et al. (2018). Acquisition and homeostasis of iron in higher plants and their probable role in abiotic stress tolerance. Front. Environ. Sci. 5:86. doi: 10.3389/fenvs.2017.00086
Trivedi, P., Pandey, A., and Palni, L. M. S. (2012). Bacterial inoculants for field applications under mountain ecosystem: present initiatives and future prospects, in Bacteria in Agrobiology: Plant Probiotics, eds. D. Maheshwari (Berlin; Heidelberg: Springer), 15–44. doi: 10.1007/978-3-642-27515-9_2
Turan, M., Ekinci, M., Yildirim, E., Güne,ş, A., Karagöz, K., Kotan, R., et al. (2014). Plant growth-promoting rhizobacteria improved growth, nutrient, and hormone content of cabbage (Brassica oleracea) seedlings. Turkish J. Agri. Forestry 38, 327–333. doi: 10.3906/sag-1304-107
Turan, M., Kitir, N., Alkaya, Ü., Günes, A., Tüfenkçi, S., Yildirim, E., et al. (2016). Making soil more accessible to plants: the case of plant growth promoting rhizobacteria. IntechOpen 5, 61–69. doi: 10.5772/64826
Tyagi, S., Mulla, S. I., Lee, K.-J., Chae, J.-C., and Shukla, P. (2018). VOCs-mediated hormonal signaling and crosstalk with plant growth promoting microbes. Crit. Rev. Biotechnol. 38, 1277–1296. doi: 10.1080/07388551.2018.1472551
Ullah, A., Heng, S., Munis, M. F. H., Fahad, S., and Yang, X. (2015). Phytoremediation of heavy metals assisted by plant growth promoting (PGP) bacteria: a review. Environ. Exp. Bot. 117, 28–40. doi: 10.1016/j.envexpbot.2015.05.001
Umair Hassan, M., Aamer, M., Umer Chattha, M., Haiying, T., Shahzad, B., Barbanti, L., et al. (2020). The critical role of zinc in plants facing the drought stress. Agriculture 10:396. doi: 10.3390/agriculture10090396
Vaid, S. K., Kumar, B., Sharma, A., Shukla, A., and Srivastava, P. (2014). Effect of Zn solubilizing bacteria on growth promotion and Zn nutrition of rice. J. Soil Sci. Plant Nutr. 14, 889–910. doi: 10.4067/S0718-95162014005000071
Vanitha, S., and Ramjegathesh, R. (2014). Bio control potential of Pseudomonas fluorescens against coleus root rot disease. J. Plant Pathol. Microb. 5:216. doi: 10.4172/2157-7471.1000216
Vardharajula, S., Zulfikar Ali, S., Grover, M., Reddy, G., and Bandi, V. (2011). Drought-tolerant plant growth promoting Bacillus spp.: effect on growth, osmolytes, and antioxidant status of maize under drought stress. J. Plant Interact. 6, 1–14. doi: 10.1080/17429145.2010.535178
Vassilev, N., Vassileva, M., and Nikolaeva, I. (2006). Simultaneous P-solubilizing and biocontrol activity of microorganisms: potentials and future trends. Appl. Microbiol. Biotechnol. 71, 137–144. doi: 10.1007/s00253-006-0380-z
Vessey, J. K. (2003). Plant growth promoting rhizobacteria as biofertilizers. Plant Soil 255, 571–586. doi: 10.1023/A:1026037216893
Viljoen, J. J., Labuschagne, N., Fourie, H., and Sikora, R. A. (2019). Biological control of the root-knot nematode Meloidogyne incognita on tomatoes and carrots by plant growth-promoting rhizobacteria. Trop. Plant Pathol. 44, 284–291. doi: 10.1007/s40858-019-00283-2
Vogel, E., Donat, M. G., Alexander, L. V., Meinshausen, M., Ray, D. K., Karoly, D., et al. (2019). The effects of climate extremes on global agricultural yields. Environ. Res. Lett. 14:054010. Available online at: https://iopscience.iop.org/article/10.1088/1748-9326/ab154b/meta
Vrabka, J., Niehaus, E.-M., Münsterkötter, M., Proctor, R. H., Brown, D. W., Novák, O., et al. (2019). Production and Role of Hormones During Interaction of Fusarium Species With Maize (Zea mays L.) Seedlings. Front. Plant Sci. 9:1936. doi: 10.3389/fpls.2018.01936
Vurukonda, S. S. K. P., Vardharajula, S., Shrivastava, M., and Skz, A. (2016). Enhancement of drought stress tolerance in crops by plant growth promoting rhizobacteria. Microbiol. Res. 184, 13–24. doi: 10.1016/j.micres.2015.12.003
Wandrag, E. M., Sheppard, A., Duncan, R. P., and Hulme, P. E. (2013). Reduced availability of rhizobia limits the performance but not invasiveness of introduced A cacia. J. Ecol. 101, 1103–1113. doi: 10.1111/1365-2745.12126
Wang, C., Cui, Y., and Qu, X. (2018). Mechanisms and improvement of acid resistance in lactic acid bacteria. Arch. Microbiol. 200, 195–201. doi: 10.1007/s00203-017-1446-2
Wu, Y., Lin, H., Lin, Y., Shi, J., Xue, S., Hung, Y.-C., et al. (2017). Effects of biocontrol bacteria Bacillus amyloliquefaciens LY-1 culture broth on quality attributes and storability of harvested litchi fruit. Postharvest Biol. Technol. 132, 81–87. doi: 10.1016/j.postharvbio.2017.05.021
Wu, Y., Yu, X., Wang, H., Ding, N., and Xu, J. (2010). Does history matter? Temperature effects on soil microbial biomass and community structure based on the phospholipid fatty acid (PLFA) analysis. J. Soils Sediments 10, 223–230. doi: 10.1007/s11368-009-0118-5
Zachow, C., Müller, H., Monk, J., and Berg, G. (2017). Complete genome sequence of Pseudomonas brassicacearum strain L13-6-12, a biological control agent from the rhizosphere of potato. Stand. Genom. Sci. 12:6. doi: 10.1186/s40793-016-0215-1
Zafar-Ul-Hye, M., Muhammad, H., Zahir, F., Ahmad, Z., Hussain, M., and Hussain, A. (2014). Application of ACC-deaminase containing rhizobacteria with fertilizer improves maize production under drought and salinity stress. Int. J. Agric. Biol. 16, 591–596. Available online at: http://www.fspublishers.org/published_papers/37071_.pdf
Zamioudis, C., Korteland, J., Van Pelt, J. A., Van Hamersveld, M., Dombrowski, N., Bai, Y., et al. (2015). Rhizobacterial volatiles and photosynthesis-related signals coordinate MYB 72 expression in Arabidopsis roots during onset of induced systemic resistance and iron-deficiency responses. Plant J. 84, 309–322. doi: 10.1111/tpj.12995
Zhang, H., Dubreuil, G., Faivre, N., Dobrev, P., Kaiser, W., Huguet, E., et al. (2018). Modulation of plant cytokinin levels in theWolbachia-free leaf-mining speciesPhyllonorycter mespilella. Entomol. Exp. Appl. 166, 428–438. doi: 10.1111/eea.12681
Zhang, H., Liu, B., Zhou, D., Wu, Z., and Wang, T. (2019). Asymmetric soil warming under global climate change. Int. J. Environ. Res. Public Health 16:1504. doi: 10.3390/ijerph16091504
Zhang, H., Qian, Z., and Zhuang, S. (2020). Effects of soil temperature, water content, species, and fertilization on soil respiration in bamboo forest in subtropical China. Forests 11:99. doi: 10.3390/f11010099
Zhao, D., Zhao, H., Zhao, D., Zhu, X., Wang, Y., Duan, Y., et al. (2018). Isolation and identification of bacteria from rhizosphere soil and their effect on plant growth promotion and root-knot nematode disease. Biol. Control 119, 12–19. doi: 10.1016/j.biocontrol.2018.01.004
Zhou, C., Zhu, L., Xie, Y., Li, F., Xiao, X., Ma, Z., et al. (2017). Bacillus licheniformis SA03 Confers increased saline–alkaline tolerance in chrysanthemum plants by induction of abscisic acid accumulation. Front. Plant Sci. 8:1143. doi: 10.3389/fpls.2017.01143
Zipfel, C., and Oldroyd, G. E. (2017). Plant signalling in symbiosis and immunity. Nature 543, 328–336. doi: 10.1038/nature22009
Keywords: phytomicrobiome, PGPR, climate change, sustainability, abiotic stresses, phytohormones, biotic and abiotic stresses
Citation: Shah A, Nazari M, Antar M, Msimbira LA, Naamala J, Lyu D, Rabileh M, Zajonc J and Smith DL (2021) PGPR in Agriculture: A Sustainable Approach to Increasing Climate Change Resilience. Front. Sustain. Food Syst. 5:667546. doi: 10.3389/fsufs.2021.667546
Received: 19 February 2021; Accepted: 26 May 2021;
Published: 06 July 2021.
Edited by:
Giuseppina Rea, National Research Council (CNR), ItalyReviewed by:
Jay Prakash Verma, Banaras Hindu University, IndiaCopyright © 2021 Shah, Nazari, Antar, Msimbira, Naamala, Lyu, Rabileh, Zajonc and Smith. This is an open-access article distributed under the terms of the Creative Commons Attribution License (CC BY). The use, distribution or reproduction in other forums is permitted, provided the original author(s) and the copyright owner(s) are credited and that the original publication in this journal is cited, in accordance with accepted academic practice. No use, distribution or reproduction is permitted which does not comply with these terms.
*Correspondence: Donald L. Smith, ZG9uYWxkLnNtaXRoQG1jZ2lsbC5jYQ==
Disclaimer: All claims expressed in this article are solely those of the authors and do not necessarily represent those of their affiliated organizations, or those of the publisher, the editors and the reviewers. Any product that may be evaluated in this article or claim that may be made by its manufacturer is not guaranteed or endorsed by the publisher.
Research integrity at Frontiers
Learn more about the work of our research integrity team to safeguard the quality of each article we publish.