- 1Bioenergy Lab, Biogas Development and Training Center, School of Biotechnology, Kalinga Institute of Industrial Technology, Bhubaneswar, India
- 2School of Biotechnology, Kalinga Institute of Industrial Technology, Bhubaneswar, India
A multitude of roles is played by microbes in food and agriculture that include nutrient cycling and management, organic matter decomposition and fermentation. Plant growth promoting rhizobacteria (PGPR), representing microbial groups and with ability of colonizing plant roots, influence plant growth through various indirect and direct modes in order to promote its growth and/or protect it from diseases or damage due to insect attack. Thus, PGPR research has received renewed interest worldwide. Increasing number of crop-specific PGPR are being commercialized these days. Approaches like seed-inoculation and soil application either alone or in combination with bacterial culture/product for increased nutrient availability through phosphate solubilisation, potassium solubilisation, sulfur oxidation, nitrogen fixation, iron, and copper chelation are gaining popularity. Arbuscular mycorrhizal fungi (AMF) are root fungal symbiont that improve management of abiotic stress such as phosphorus deficiency. PGPR involves roles like production of indole acetic acid (IAA), ammonia (NH3), hydrogen cyanide (HCN), catalase, etc. PGPR also improve nutrient uptake by altering the level of plant hormone that enhances root surface area by increasing its girth and shape, thereby helping in absorbing more nutrients. PGPR facilitate seed germination, seedling growth and crop yield. An array of microbes including Pseudomonas, Azospirillum, Azotobacter, Klebsiella, Enterobacter, Alcaligenes, Arthrobacter, Burkholderia, Bacillus, and Serratia enhance plant growth. Various Pseudomonas sp. have demonstrated significant increase in germination, seedling growth and yield in different agricultural crops, including wheat. Hence, developing a successful crop-specific PGPR formulation, the candidate should possess characteristics like high rhizosphere competence, extensive competitive saprophytic ability, growth enhancing ability, ease of mass production, broad-spectrum action, safety toward the environment and compatibility with other partnering organisms.
Introduction
The twenty-first century has been witnessing rapid rise in human population along with critical issues in global agroecosystems, leading to decreased productivity and degeneration of sustainable agroecosystem. Food is a fundamental need of people that assumes a significant function in human well-being and societal advancements. While feeding the geometrically progressing human population, required balanced value-added food to supplement a diet is significantly difficult. In contrast, there is a decrease in crop productivity, which may pose additional concern to address before long. The ever-increasing population exhibits pressure on arable land to increase crop yield, leading to indiscriminate use of chemical fertilizers, insecticides, pesticides, etc. by the farmers. Agrochemicals runoffs from such land adversely affect life on Earth through bioaccumulation and biomagnifications through the food chain. Also, the pesticides utilized to fight against plant illness affect the beneficial natural insect, soil fertility and soil microbiota adversely (Khatoon et al., 2020), and obviously additionally impact human well-being. Alongside, the soil acidity due to these strong chemicals is also altered (Slepetiene et al., 2020). Anthropogenic activities are liable to cause ecological damage and deter soil health, ultimately depleting the nonrenewable assets. It is therefore essential to adopt various environment-friendly ways. In the present circumstance, sustainable agriculture is essential as it offers the capacity to meet not only our present needs but also ensure a healthy future, something that can't be realized through the conventional harmful agrarian practices (Santoyo et al., 2017). Crop yields have been affected by a wide range of ecological destabilization emerging from complex issues (Shrivastava and Kumar, 2015). For example, salinity as a significant environmental abiotic stress is a serious global agricultural concern, turning the cultivated areas in to uncultivated region especially within the arid and semiarid zones at an estimated rate of around 1–2% annually.
Around 20% of the whole arable zone has become transformed to uncultivated region as a consequence of salinity (Rasool et al., 2013). Salinity brings about extreme change in the development and metabolism in plant (e.g., changes in plant physiology, morphology, and biochemistry) (Gupta and Huang, 2014). Drought is another abiotic stress in most aerable lands, particularly in the arid and semiarid regions affecting crop productivity (Bodner et al., 2015). Due to more serious and regular droughts owing to climate change, it is predicted that more than 50% of the arable land will have critical plant growth issues by 2050. Drought affects the crop adversely by influencing water association, photosynthetic assimilation, and supplement take-up (Osakabe et al., 2014). In present times, heavy metal contamination may pose a threat to ecology. Since these metals are hard to remove, environmentalists are worried about their harmfulness to the ecology and biological systems (Etesami, 2018). Some of these metals are needed at very low quantity for various plant metabolic processes, but at very high levels these metals affect the phytological and microbial network adversely (Ali et al., 2015). Heavy metals add to the inadequacy and irregularity of basic plant supplements (Etesami, 2018). Likewise, flood is another stress factor that affects plant productivity. All these non-biological stresses are increasing day by day due to global climatic change. Therefore, it is important to reduce environmental stress by employing ecofriendly strategies, including eco-accommodating and sustainable utilization of beneficial microbes.
Plant growth promoting rhizobacteria (PGPR) are critical players in agriculture (Etesami and Maheshwari, 2018). It helps in crop well-being by fixing nitrogen, solubilising phosphate, reducing heavy metals, producing phytohormones (like auxin, gibberellins, cytokinins etc.), mineralising soil organic matter, decomposing crop residue, suppressing phytopathogens, etc. (He et al., 2019). A detailed overview of the different direct and indirect mechanisms of action by plant growth promoting microbiomes is highlighted in Figure 1. PGPR offer critical knowledge to the agricultural and environmental biotechnology by providing several new genes and understanding of the biochemical pathways for enzymes, antibiotics, and numerous other value-based bioproducts (Backer et al., 2018). The efficacy of PGPR relies on its growth phase, the variability in soil ecology, and the species and age of plant/crop. PGPR adjust plant-soil chemistry thereby facilitating plant growth and well-being (Table 1). To utilize PGPR successfully, it is imperative to understand the mechanisms through which they influence and guarantee sustainable agriculture (Figure 2).
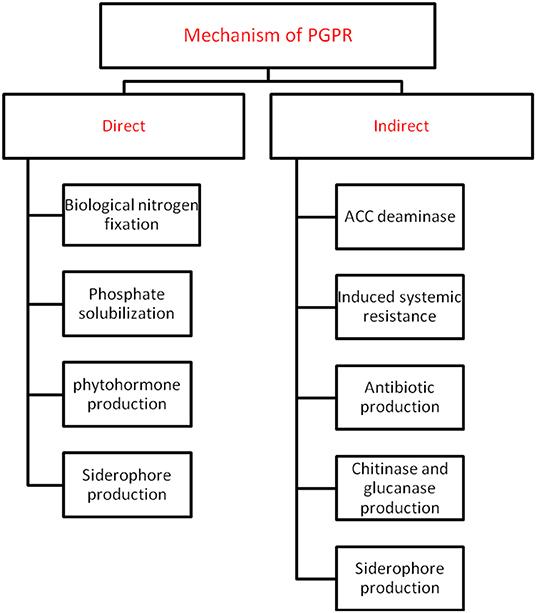
Figure 1. An overview of different types of direct and indirect mechanisms adopted by PGPR against abiotic stress.
Rhizosphere: the Hub for Microbial Habitat
Rhizosphere is characterized as the zone for various soil biological and chemical attributes and influence plant root secretions. It is the hotspot for intense interactions between the soil and the microflora (Kumar et al., 2015), impacting beneficial and or remaining negative or neutral. Their interactions affect plant growth and productivity heavily. Several microbial groups, like bacteria, fungi, protozoa, and algae coexist in the rhizosphere. Of all these, the bacteria that promote plant growth are the most abundant (Bahadur et al., 2017). Growth promoting bacteria in general and rhizobacteria in particular exhibit extensive interaction in rhizospheric zone. Giving an extraordinary environment inside the rhizosphere, plant releases many compounds as root exudates that is high in sugars, amino acids, organic acids, flavonoids, proteins, and fatty acids. Such root exudates are usually low molecular weight compounds, non-metabolically released. The exudates act as signals either to repel various microbial pathogens or to gather beneficial microbes owing to the physiological status, plant species, and the microflora (Ahmed et al., 2019). The exudates may also act as rhizospheric messenger molecules between plant root and rhizobacterial organisms (Lucini et al., 2019), thus are significant growth triggers for soil microbes that assume a crucial role in advancing plant development and in mobilizing protection against phytopathogens. Along with root exudates, plants also release additional components like metabolically released or secreted compounds, lysates released from moribund cells during autolysis, and mucilage (a plant polysaccharide) in the rhizospheric zone. All such secretions act as chemoattractants for rhizospheric microflora. Few PGPR can enter the root, setting up endophytic association (Wozniak et al., 2019 and Papik et al., 2020). Some of them overcome the endodermis barrier, rising above from root cortex to vascular systems, developing as endophytes (inside leaves, stem, and other organs). The degree of host plant endophytic relationship mirrors the capacity of these microbes to adapt to different explicit biological specialties. Such bacteria-plant relationships can be derived for crop well-being and productivity.
PGPR and Abiotic Stress
Any unfavorable environmental condition that may affect the functional diversity of microbes and also the physicochemical properties of soil can dictate abiotic stress. Numerous drastic conditions including heavy metal toxicity, salinity, drought, and flooding affecting the plant microbiome and the surrounding ecology are abiotic stress.
Heavy Metals
Hyper-aggregation of noxious metals like Hg, As, Cd, and Pb in soil result in plant stress, and extraordinarily diminish crop productivity. Such metal aggregations directly influence soil pH and its texture, thereby hampering crop growth by imparting negative consequences on a few biological processes (Hamid et al., 2021). Majority of the microbes, particularly the heterotrophs, receive food through carbonaceous forms from the host plant through the symbiotic association. Thus, there is a decrease in the effect of pollutants on plants added by the help of these (Zafar-ul-Hye et al., 2013). Though PGPR helps in plant growth and productivity, it also improves soil properties through various mechanisms to regulate soil metal contaminants (Table 2). Some peculiar binding metal peptides are associated with metal chelation or accumulation. Transformation mechanisms by PGPR, like metal bioaccumulation, biosorption, precipitation, oxidation, and reduction of metal in enzymatic pathway, decreases the toxic effect of heavy metal ions (Sharma and Archana, 2016).
Induced Systemic Tolerance (IST) in Metal Stress
Induced systemic resistance induces abiotic stress tolerance toward metals. The genetic basis of the process is well-known (Hobman and Crossman, 2015; Wheaton et al., 2015). Contaminating metals in the rhizosphere hinders plant nutrient uptake resulting in a delayed plant growth (Lal et al., 2018). This could be addressed by inoculating PGPR having metal resistance ability. PGPR can effectively stimulate IST against abiotic stress in plants. IAA delivering PGPR managed heavy metal stress while improving nitrogen fixation and other growth conditions (Guo et al., 2020). Cellulosimicrobium reportedly enhanced the flowering period under chromium (Cr6+) stress (Karthik and Arulselvi, 2017). The isolated and characterized Cr6+ resistant bacteria could also dissolve phosphate, produce ammonia, and secrete enzymes like lipase and amylase in the rhizosphere of Phaseolus vulgaris. Factors responsible to activate IST in host plants are recognized as similar to those of induced systemic resistance (ISR). ISR induction correlates with factors like bacterial external membrane lipopolysaccharides, biosurfactants, siderophores, volatile organic compounds, and other microbial metabolites. Studies show that gibberellic acid (GA) eased metal toxicity by reducing Cd2+ absorption (Zhu et al., 2012). Rhizobacteria also possess a metal rejection mechanism to protect the delicate cell parts from heavy metals, changing their cell wall and membrane.
Siderophores in Metal Sequestration
Siderophores are microbial metabolites that form trace metal complexes. These are organic compounds of lower molecular weight having a good iron affinity. Microbes produce these in iron-deficient condition. Microbially released siderophores perceivably resist metal stress efficiently. Microbial siderophores formed tie up with metal ions making the limited metal ion bioavailable. Streptomycetes siderophores assimilated iron and diminished Cd2+ retention (Dimkpa et al., 2009a). Bacterial siderophore reduced the oxidative stress minimizing the toxic impact of metals and accelerating plant biomass growth (Dimkpa et al., 2009b).
Biosurfactants in Metal Reduction
Amphiphilic composite biosurfactants found primarily on the microbial cell surface. They enhance trace metal tolerance and help in ousting soil metal. Because of the amphiphilic nature, these hold together more closely to noxious metals (Gupta and Kumar, 2017), by showing an undeviating connection between biosurfactants and trace metals. This binding nature of biosurfactants with heavy metal helps reduce its concentration in soil (Lal et al., 2018).
Impact of Organic and Inorganic Acids in Heavy Metal Reduction
PGPR produce many low molecular weights natural (organic) acids, like oxalic and citric acids (Archana et al., 2012), which demonstrably reduce metal stress in farming. These form less-noxious metal complexes and facilitate plant growth in metal-contaminated soils. These complexes (e.g., metallic oxalate crystal) enhance resistance in plants by reducing the adverse cytological effects of the native metal ions by inactivating them (Gao et al., 2010). Inorganic acids by PGPR could decrease metal stress through precipitation. Microbial organic acid is perceived to have a solids limit in chelating heavy metals (Gadd, 2010). Insolubilising and immobilizing heavy metals is either instantaneous via the enzymatic action (Pagnanelli et al., 2010), or through the circuit with the aid of bacterial oxidation of Fe, or through involvement of microbial inorganic acids like H2S, H2CO3 and H3PO4 (Zhou et al., 2013).
Bacterial EPS in Metal Reduction
Extracellular polymeric substances (EPS) are heavy molecular weight homo- or hetero-polysaccharides microbial polymers (Staudt et al., 2004). It binds to bacterial cell surface, present internally as a capsule or is secreted externally. Extracellular polysaccharides such as lipopolysaccharides, polysaccharides, dissolvable peptides, and glycoprotein released by rhizospheric bacteria (Hassan et al., 2017) have a significant number of anion-restricting locales that help in evacuation or recuperation of heavy metals from the rhizosphere through biosorption (Mishra et al., 2017). Due to the composition, it assumes a pivotal job in heavy metal decontamination by diminishing their availability in soil and plant systems (Rajkumar et al., 2012).
Salinity
Salinity conditions are detrimental for agro-economy. The primary reason for the salinity issue is attributed to the accumulating salts due the use of agrichemicals over long (Rengasamy, 2002). There is alternation in plant homeostasis in salt-stressed region in the soil, leading to nutritional imbalance. Being sessile, plants can't run and escape from the situation, but rather struggle and acclimatize to it. Studies identify the significance of PGPR to boost growth and productivity in salt-stressed plant for sustainable agriculture (Venkateswarlu et al., 2008).
Halotolerance by Scavenging (ROS)
The reactive oxygen species (ROS) like , O2, and H2O2 is formed under salinity stress significantly damaging the cell, known as oxidative stress. An enzymatic and nonenzymatic protective antioxidant system is activated to neutralize such toxicity by managing the H2O2 level (Bharti and Barnawal, 2019). Enzymes like catalase and ascorbate peroxidase and non-enzymatic components like ascorbate routinely regulate the ROS levels (Kapoor et al., 2015). Along with ROS production, the salt-stressed plant is hypohydrated. PGPR capable to produce the enzymatic and non-enzymatic components help the plant to survive under salt stress. ROS-responsive signaling and regulatory genes of PGPR are beneficial (Miller et al., 2010). By boosting the antioxidants and polyamines, some PGPR mitigate the increased soil salinity, elevating the photosynthetic performance (Radhakrishnan and Baek, 2017). PGPR produces antioxidants like catalase, and neutralizes ROS-mediated oxidative stress. Improved performance of SOD in salt-stressed plants inoculated with PGPR assumes a critical role in superoxide scavenging.
Halotolerance by Decreasing Ethylene Level
Ethylene, a gaseous hormone, helps in controlling and regulating plant growth (Fahad et al., 2015). Usually, two ethylene peaks measured in plant tissues, the first usually being smaller than the second. First peak is seen when the plant is subjected to stress, and the second peak shows up after a couple of hours. A quick ethylene antecedent is 1-aminocyclopropane-1-carboxylate (ACC). Some PGPR produce ACC deaminase that protects plants from ethylene stress. ACC deaminase in plants breaks apart ACC to form α-ketobutyrate and ammonia, consequently reducing the ethylene level. As a sink for ACC, PGPR formulation with active ACC deaminase decreases plant ethylene levels (Glick, 2014). In comparison to the control without microbial inoculations, seedlings treated with ACC deaminase-containing bacteria decreased ethylene in plants exposed to salt stress (Barnawal et al., 2017), thus an effective method to mitigate salt stress to an extent.
Drought
Drought is a leading factor that hinders worldwide agricultural productivity. It is believed to have played down the national cereal production by 9–10% (Lesk et al., 2016). The capacity of a plant to sustain and endure during drought situation is its drought resistance. Solutions to increase abiotic stress tolerance like drought in plants enabling its growth meeting food needs under restricted accessibility of water resources need to be established (Mancosu et al., 2015). Abscisic acid (ABA) improves drought tolerance owing to a few responsible active signaling genes like DSM2, Os-NAP, and OsNAC5. These genes facilitate an increase in the yield during drought (Goswami and Suresh, 2020).
Improving Root System for Water Uptake
An increased number of rootlets with lesser diameter with greater depth in the root system is a novel approach to sustain plant productivity in drought conditions (Ngumbi and Kloepper, 2016) as it allows draft-stressed plants to enhance the hydraulic conductance by raising the area of contact with the available water in soil with an increase in volume (Comas et al., 2013). It is suggested that modifications in the root architecture caused by bacteria maximizes the total area of the root resulting in improved nutrient and water absorption facilitating the overall growth (Timmusk et al., 2014). Comprehensive studies are needed to understand the underlying mechanism. Naik et al. (2020) has reported the root elongation property of a nano formulation (SomRE) to overcome abiotic stress without any adverse effect on the soil microbiota.
Facilitating Shoot Growth
Decreased accessible leaf surface to restrict evaporative loss is an adoptive mechanism in plants to address drought stress which may stunt shoot growth (Skirycz and Inzé, 2010). Treating such plants with PGPR improves shoot growth; plants inoculated with successful PGPR strains may retain close-to-average shoot growth in drought stress, resulting in enhanced crop productivity.
Relative Water Content
Perhaps a better approach to evaluate plant water status is measure its relative water content (RWC) in leaf as it is involved in tissue metabolic activity. A reduced RWC indicates turgor deficiency that restricts cellular development and diminished plant growth (Ngumbi and Kloepper, 2016). Maximizing RWC could significantly enhance drought tolerance. PGPR-treated plants reportedly managed RWC better compared to the non-treated plants. Dodd et al. (2010) found that better RWC could be a consequence of change in the physiological activity like stomatal closure. Grover et al. (2014) reported 24% enhancement RWC in drought-stressed sorghum plants inoculated with Bacillus sp. strain KB 129.
Osmotic Adjustment in Drought Tolerance
Osmotic modification is a major adaptation strategy in plants that allow them to tackle drought-stress (Farooq et al., 2009). Proteins, enzymes, cell organelles, and membrane are safe from oxidative destruction (Huang et al., 2014). Osmotic adaptation in drought stress is the active accumulation of inorganic and organic compatible solutes (Kiani et al., 2007). The solutes include ammonium compounds like sucrose, non-protein amino acids like proline, betaine, glycine, polyols like mannitol, inorganic ions like calcium, and organic acids like malate. They preserve cell turgor and reduce water demand while maintaining the water content.
Proline is a fundamental osmolyte in plants experiencing drought-stress (Huang et al., 2014). Proline also helps stabilize subcellular structures like proteins and membranes, scavenge free radicals and buffer the capacity for cellular redox (Hayat et al., 2012). Critical studies suggest that plants with higher proline levels can withstand dry season (Lum et al., 2014). PGPR inoculation helps increase proline levels in plants. A combined three PGPR strains (Bacillus cereus AR156, B. subtilis SM21, and Serratia sp. XY21) enhanced proline concentration 3–4-folds in cucumber leaves compared to the control (Wang et al., 2012). The high proline level protected cucumber plants from excess drying. PGPR application increased concentrations of dissolvable sugar and free amino acids in maize (Bano et al., 2013). Additional free amino acids and dissolvable sugars alongside proline are essential for withstanding conditions of extreme drought.
PGPR and Plant Growth Substances
Externally applied chemical growth regulators and various phytohormones like cytokinins, abscisic acid, gibberellins, auxins, and ethylene facilitate plant growth and development. Jasmonic acids (JAs) reportedly play an important role in drought tolerance in plants. Its exogenous utilization enhances the formation of various antioxidants to withstand drought. Antioxidants' influence has been highlighted in crops including in Desi chickpea cultivated under drought stress. The expression pattern of the jasmonate signaling pathway gene (MYC2) is significantly outlined (Domenico et al., 2012). PGPR encourage growth of drought-stressed plants (Bresson et al., 2014) by controlling and adjusting the phytohormones and growth regulators. Plant growth is encouraged by gibberellins and cytokinins and inhibited by ET and abscisic acid (Taiz and Zeiger, 2010). Drought stress increases the levels of growth inhibitors. Studies have highlighted that SomRE (an organic root growth promoter) help to transport nutrients to the upper portion of plant for their growth and development. SomRE contains vulcanine and borreline that help in root elongation (Naik et al., 2020).
Flood
Reports indicate the effect of rhizobacteria on plant physiology when exposed to flooding (Ravanbakhsh et al., 2017). Studies reveal that plant roots associated with bacterial population impact in regulating ethylene. Such exchange of gas reduces during flooding, resulting in rapid accumulation of inside plant. Accumulated ethylene regulates the traits related to flood adaptation (Sasidharan and Voesenek, 2015). Ravanbakhsh et al. (2017) highlighted the role of R. palustris in ACC deaminase production which led to a reduction in the ethylene levels. ACC level rises during flood (low oxygen condition) attributed to the action of both ACC synthase and ACC oxidase genes. High ACC concentration accumulated in the root is reduced by ACC deaminase which helps ACC to diffuse out of the roots. This mechanism helps reduce ethylene levels during and after flooding. Any disturbance in the ethylene signaling pathway leads to a drastic reduction in the responses to flood situations (Ravanbakhsh et al., 2017).
PGPR and Biotic Stress
Biotic stress in plants is brought about by living forms, particularly bacteria, viruses, fungi, insects, and nematodes. Such stress interferes directly with host nutrients causing plant death. Both pre- and post-harvest loss occurs due to biotic stress. Although few microbes participate in the biological control of pathogens, yet PGPR is known to create protection from many diseases following various mechanisms including bacteriocin, antibiosis, volatile organic compound (VOC) production, and lysis through the extracellular enzyme (Hamid et al., 2021). The microbial stimulants are seen to be effective in the suppression of a variety of plant-pathogen which ultimately leads to sound development in the harvest.
Bacteriocin
Bacteriocins (bacterial toxins against bacteria) are peptide secretions with narrow-spectrum antimicrobial activity. Bacteriocins are produced by Gram-negative (e.g., colicin) as well as Gram-positive (e.g., nisin) bacteria (Zimina et al., 2020). These toxins are very specific in their action and eliminate competitor bacterial species (Rooney et al., 2020). Bacteriocins have shown promising results under in vitro conditions against bacterial spot disease in tomatoes (Príncipe et al., 2018).
Antibiosis
Antibiotics produced by PGPR are more efficient than others due to their antimicrobial, insecticidal, antiviral, phytotoxic, cytotoxic, and anthelminthic properties (Fernando et al., 2018). Numerous species of Pseudomonas produce a wide scope of antifungal antibiotics, including 2,4-diacetylphloroglucinol (2,4-DAPG), butyrolactones, rhamnolipids, N-butylbenzene sulfonamide (Ramadan et al., 2016). Bacillus species also excrete a large variety of antibiotics, including bacilysin, bacillaene, mycobacillin, etc. Additionally, they produce various lipopeptide biosurfactants e.g., bacillomycin with antibiotic activity (Wang et al., 2015).
VOC Production
PGPR secretes numerous Volatile Organic Compound (VOC), which are biocontrol specialists for certain nematodes and microorganisms. Some illustrations of VOC are benzene, cyclohexane, tetradecane, 2-(benzyloxy)- 1-ethanamine. HCN is one of the VOC (delivered by rhizospheric microbes) having the capacity in the biocontrol of some phytopathogens (Kanchiswamy et al., 2015). HCN of Pseudomonas sp. can hinder some pathogenic growths (Hamid et al., 2021). Likewise, VOCs emitted by Bacillus spp. are successful inhibitors of fungus (Santoro et al., 2016). Along with the role of biological control, VOC plays role in pollinator attraction through communication signals (Liu and Brettell, 2019).
Lysis via Extracellular Enzyme
Lytic compounds produced by PGPR give another powerful system to infectious microbes. Extracellular enzyme of rhizobacteria (chitinase and β-1,3- glucanase) are associated with lysis of cell wall (Goswami et al., 2016). As the fungal cell wall is mostly made out of chitin and β−1,4-N-acetyl-glucosamine, Chitinase and β-1,3-glucanase of rhizobacteria can degrade them and act as are strong antifungals. For instance, P. fluorescens LPK2 and S. fredii KCC5 discharge β-glucanases and chitinases help in under-expression of the wilts which is brought about by Fusarium udum and F. oxysporum (Ramadan et al., 2016). Microbes show insecticidal action which has been accounted for protease, lipase, and chitinolytic activity (Rakshiya et al., 2016). PGPR with ACC deaminase action moreover assumes a vital part taking all things together sorts of stresses, including biocontrol.
CO-Metabolism of PGPR
As a part of the metabolic cooperation with plants, it is suggested that plants provide amino acids, sugars, organic acids, and other carbon sources to microbes dwelling in the rhizopsheric region (Jones et al., 2009). This niche is valuable to explore metabolic associations between plants and rhizomicrobes (Jacoby and Kopriva, 2019). Rhizospheric microbial metabolites are considered crucial in ecological success. Various rhizomicrobes that share this habitat have important ecological roles based on their substrate uptake patterns (Ghoul and Mitri, 2016). The idea of niche differentiation can be based on different rhizomicrobial strains coexisting in the same niche exhibiting partitioned metabolism. If two strains have similar substrate uptake pattern then the fittest survives, leading to competitive exclusion of the strain that is less fit (Jacoby and Kopriva, 2019). Often a rhizobacteria strain functions in a way that it excretes a novel metabolite that was not a part of the native root. This leads to the formation of a new niche that could be inhabited by cross-feeding strains (Ponomarova et al., 2017). When ample amount of sugar is added, soil microbes quickly proliferate giving a notion that there is carbon limitation in the growth of microbes (Blagodatskaya and Kuzyakov, 2013). Thus, it is attributed that plants contain ample amount of carbon that diffuse to outside through multiple metabolic pathways.
Even though carbon fixation occurs primarily through respiration, carbon is released by plants through rhizospheric deposition (Pausch and Kuzyakov, 2018). While rhizomicrobes provide metabolites to plants, the plant rhizodeposits provide a range of metabolites offering huge opportunities both to attract and inhibit specific microbial strains (Chagas et al., 2018). Rhizomicrobes provide nitrogen, phosphorus and iron to plants in utilizable forms that are crucial for growth. Rhizomicrobes provide plants with phytohormones like ACC deaminase, cytokinin, and indole-3-acetic acid which aid in its growth and development (Kumar et al., 2019). Thus, such cometabolism allows a healthy symbiotic relationship between the plants and rhizomicrobes.
Criteria to Select Suitable PGPR Candidate
For the development of a successful PGPR formulation, the rhizobacterial species should possess the following characteristics (Jeyarajan and Nakkeeran, 2000):
• Should be enhancing plant growth
• Should be amenable for mass multiplication
• Should possess high rhizospheric competence
• Should have high competitive saprophytic ability
• Should demonstrate broader activity spectrum
• Should be ecologically compatible to other inhabiting rhizobacteria
• Ability to tolerate abiotic (thermal, desiccation, radiations, and oxidizing agents) stress
• Should be environmentally safe.
PGPR as Biofertiliser
Biofertilisers are the live formulation of beneficial microbes which assists in the availability of nutrients by their biological activity and builds up soil health and thus soil microflora. So, the main component of this biofertiliser is plant growth promoting microbes (PGPM). This PGPM can be categorized into three major groups, i.e., Arbuscular mycorrhizal organisms (AMF), plant development advancing rhizobacteria (PGPR), and nitrogen-fixing rhizobia (Vejan et al., 2016), which are considered to be helpful for plant development and nourishment. Nonetheless, it has been accounted for that PGPR has been utilized worldwide as biofertilizers, adding to expanded yields and soil quality. Subsequently, with likely PGPR commitment, it could prompt sustainable agribusiness. Such biofertilizers are available in both solid and liquid forms, and liquid formulations are found to be more effective. Liquid formulations are primarily of three types, i.e., root inoculation, seed inoculation and soil inoculation (Lopes et al., 2021). Upon applying Burkholderia phytofirmans biofertiliser to Ryegrass root, seed, and soil, soil inoculation method was most efficient in improving the production of plant biomass, phytoremediation and hydrocarbon degradation (Afzal et al., 2013).
Challenges With PGPR
One constraint in using PGPR is their property of natural variation. It is difficult to predict the response of an organism in field conditions (unlike in controlled laboratory environment). Another challenge is that PGPR are living in nature and they must be able to propagate artificially and mass produced in an optimized manner with regard to their viability and biological activity until field application. Like Rhizobium, PGPR bacteria will not live in soil for long, and over time cultivators will need to reinoculate to restore their population.
Conclusion and Future Prospects
Crop yield is affected by a wide range of ecological concern emerging from complex natural conditions. It is important to address environmental (abiotic) stress through ecofriendly strategies. A way to accomplish eco-accommodative sustainable agriculture is utilizing the beneficial microbes. PGPR help soil and crop well-being through various ways. For microbes, rhizosphere, where soil and microfauna have extreme intense interactions, is a hotspot. Among all, plant growth promoting bacteria are most abundant in the rhizosphere. PGPR improve soil properties through various mechanisms regulating soil contaminations. PGPR help to adapt to abiotic stresses like salinity, drought, flood stress, and also to the biotic stresses. Additionally, PGPR also helps plants to adapt to flood stress. Rhizosphere and PGPR rhizomicrobiome cometabolise, the former as the source of nutrients for the rhizomicrobes and the rhizomicrobe biotransform nitrogen, phosphorus and iron converting them into more utilizable forms for the plants. Understanding the genetics in PGPR investigation and engineering it is a more promising futuristic approach, which shall provide overexpression of the desired traits in the participating strain. As microbes behave differently in the laboratory and field conditions, there is also a need to propagate PGPR in field conditions for them to regain their biological activity and viability.
Author Contributions
PM has contributed to the first drafting and formatting of the manuscript. PKS outlined the manuscript and has designed the figures. DC has helped in preparing table and figure for the manuscript. SM and RP have developed the skeleton for the manuscript along with editing and other valuable scientific inputs. All authors contributed to the article and approved the submitted version.
Conflict of Interest
The authors declare that the research was conducted in the absence of any commercial or financial relationships that could be construed as a potential conflict of interest.
Acknowledgments
Authors acknowledge their respective affiliations for infrastructural facilities.
References
Afzal, M., Khan, S., Iqbal, S., Sajjad, M., Qaiser, M., and Khan, M. (2013). Inoculation method affects colonization and activity of Burkholderia phytofirmans PsJN during phytoremediation of diesel-contaminated soil. Int. Biodeterior. Biodegrad. 85, 331–336. doi: 10.1016/j.ibiod.2013.08.022
Ahemad, M., and Khan, M. S. (2012). Alleviation of fungicide-induced phytotoxicity in greengram [Vigna radiata (L.) Wilczek] using fungicide-tolerant and plant growth promoting Pseudomonas strain. Saudi J. Biol. Sci. 19, 451–459. doi: 10.1016/j.sjbs.2012.06.003
Ahmed, T., Shahid, M., Noman, M., Hussain, S., Khan, M. A., Zubair, M., et al. (2019). “Plant growth-promoting rhizobacteria as biological tools for nutrient management and soil sustainability,” in Plant Growth Promoting Rhizobacteria for Agricultural Sustainability, eds A. Kumar and V. S. Meena (Singapore: Springer), 95–110. doi: 10.1007/978-981-13-7553-8_5
Ait-Kaki, A., Kacem-Chaouche, N., Ongena, M., Kara-Ali, M., Dehimat, L., Kahlat, K., et al. (2014). In vitro and in vivo characterization of plant growth promoting Bacillus strains isolated from extreme environments of eastern Algeria. Appl. Biochem. Biotechnol. 172, 1735–1746. doi: 10.1007/s12010-013-0617-0
Ali, S., Chaudhary, A., Rizwan, M., Anwar, H. T., Adrees, M., Farid, M., et al. (2015). Alleviation of chromium toxicity by glycinebetaine is related to elevated antioxidant enzymes and suppressed chromium uptake and oxidative stress in wheat (Triticum aestivum L.). Environ. Sci. Pollut. Res. 22, 10669–10678. doi: 10.1007/s11356-015-4193-4
Archana, G., Buch, A., and Kumar, G. N. (2012). “Pivotal role of organic acid secretion by rhizobacteria in plant growth promotion.” in Microorganisms in Sustainable Agriculture and Biotechnology. (Dordrecht: Springer), 35–53. doi: 10.1007/978-94-007-2214-9_3
Backer, R., Rokem, J. S., Ilangumaran, G., Lamont, J., Praslickova, D., Ricci, E., et al. (2018). Plant growth-promoting rhizobacteria: context, mechanisms of action, and roadmap to commercialization of biostimulants for sustainable agriculture. Front. Plant Sci. 9:1473. doi: 10.3389/fpls.2018.01473
Bahadur, I., Maurya, B. R., Meena, V. S., Saha, M., Kumar, A., and Aeron, A. (2017). Mineral release dynamics of tricalcium phosphate and waste muscovite by mineral-solubilizing rhizobacteria isolated from indo-gangetic plain of India. Geomicrobiol. J. 34, 454–466. doi: 10.1080/01490451.2016.1219431
Bano, Q., Ilyas, N., Bano, A., Zafar, N., Akram, A., and Hassan, F. (2013). Effect of Azospirillum inoculation on maize (Zea mays L.) under drought stress. Pak. J. Bot. 45, 13–20.
Barnawal, D., Bharti, N., Pandey, S. S., Pandey, A., Chanotiya, C. S., and Kalra, A. (2017). Plant growth-promoting rhizobacteria enhance wheat salt and drought stress tolerance by altering endogenous phytohormone levels and TaCTR1/TaDREB2 expression. Physiol. Plant. 161, 502–514. doi: 10.1111/ppl.12614
Bharti, N., and Barnawal, D. (2019). “Amelioration of salinity stress by PGPR: ACC deaminase and ROS scavenging enzymes activity,” in PGPR Amelioration in Sustainable Agriculture, eds A. K. Singh, A. Kumar, and P. K. Singh (Cambridge: Woodhead Publishing), 85–106. doi: 10.1016/B978-0-12-815879-1.00005-7
Blagodatskaya, E., and Kuzyakov, Y. (2013). Active microorganisms in soil: critical review of estimation criteria and approaches. Soil Biol. Biochem. 67, 192–211. doi: 10.1016/j.soilbio.2013.08.024
Bodner, G., Nakhforoosh, A., and Kaul, H. P. (2015). Management of crop water under drought: a review. Agron. Sustain. Dev. 35, 401–442. doi: 10.1007/s13593-015-0283-4
Bresson, J., Vasseur, F., Dauzat, M., Labadie, M., Varoquaux, F., Touraine, B., et al. (2014). Interact to survive: Phyllobacterium brassicacearum improves Arabidopsis tolerance to severe water deficit and growth recovery. PLoS ONE. 9:e107607. doi: 10.1371/journal.pone.0107607
Chagas, F. O., de Cassia Pessotti, R., Caraballo-Rodríguez, A. M., and Pupo, M. T. (2018). Chemical signaling involved in plant–microbe interactions. Chem. Soc. Rev. 47, 1652–1704. doi: 10.1039/C7CS00343A
Cheng, K. Y., Karthikeyan, R., and Wong, J. W. (2019). Microbial electrochemical remediation of organic contaminants: possibilities and perspective. Microb. Electrochem. Technol. 25, 613–640. doi: 10.1016/B978-0-444-64052-9.00025-X
Comas, L., Becker, S., Cruz, V. M. V., Byrne, P. F., and Dierig, D. A. (2013). Root traits contributing to plant productivity under drought. Front. Plant Sci. 4:442. doi: 10.3389/fpls.2013.00442
Dimkpa, C. O., Merten, D., Svatoš, A., Büchel, G., and Kothe, E. (2009a). Siderophores mediate reduced and increased uptake of cadmium by Streptomyces tendae F4 and sunflower (Helianthus annuus), respectively. J. Appl. Microbiol. 107, 1687–1696. doi: 10.1111/j.1365-2672.2009.04355.x
Dimkpa, C. O., Merten, D., Svatoš, A., Büchel, G., and Kothe, E. (2009b). Metal-induced oxidative stress impacting plant growth in contaminated soil is alleviated by microbial siderophores. Soil Biol. Biochem. 41, 154–162. doi: 10.1016/j.soilbio.2008.10.010
Dinnage, R., Simonsen, A. K., Barrett, L. G., Cardillo, M., Raisbeck-Brown, N., Thrall, P. H., et al. (2019). Larger plants promote a greater diversity of symbiotic nitrogen-fixing soil bacteria associated with an Australian endemic legume. J. Ecol. 107, 977–991. doi: 10.1111/1365-2745.13083
Dodd, I. C., Zinovkina, N. Y., Safronova, V. I., and Belimov, A. A. (2010). Rhizobacterial mediation of plant hormone status. Ann. Appl. Biol. 157, 361–379. doi: 10.1111/j.1744-7348.2010.00439.x
Domenico, S., Bonsegna, S., Horres, R., Pastor, V., Taurino, M., Poltronieri, P., et al. (2012). Transcriptomic analysis of oxylipin biosynthesis genes and chemical profiling reveal an early induction of jasmonates in chickpea roots under drought stress. Plant. Physiol. Biochem. 61, 115–122. doi: 10.1016/j.plaphy.2012.09.009
Etesami, H. (2018). Bacterial mediated alleviation of heavy metal stress and decreased accumulation of metals in plant tissues: mechanisms and future prospects. Ecotoxicol. Environ. Saf. 147, 175–191. doi: 10.1016/j.ecoenv.2017.08.032
Etesami, H., and Maheshwari, D. K. (2018). Use of plant growth promoting rhizobacteria (PGPRs) with multiple plant growth promoting traits in stress agriculture: action mechanisms and future prospects. Ecotoxicol. Environ. Saf . 156, 225–246. doi: 10.1016/j.ecoenv.2018.03.013
Fahad, S., Nie, L., Chen, Y., Wu, C., Xiong, D., Saud, S., et al. (2015). Crop plant hormones and environmental stress. Sust. Agric. Rev. 371–400. doi: 10.1007/978-3-319-09132-7_10
Farooq, M., Wahid, A., Kobayashi, N., Fujita, D. B. S. M. A., and Basra, S. M. A. (2009). Plant drought stress: effects, mechanisms and management. Sust. Agric. 153–188. doi: 10.1007/978-90-481-2666-8_12
Fernando, W., Nakkeeran, S., Zhang, Y., and Savchuk, S. (2018). Biological control of Sclerotinia Sclerotiorum (lib.) de Bary by Pseudomonas and Bacillus species on canola petals. Crop. Prot. 26, 100–107. doi: 10.1016/j.cropro.2006.04.007
Gadd, G. M. (2010). Metals, minerals and microbes: geomicrobiology and bioremediation. Microbiol. 156, 609–643. doi: 10.1099/mic.0.037143-0
Gao, Y., Miao, C., Mao, L., Zhou, P., Jin, Z., and Shi, W. (2010). Improvement of phytoextraction and antioxidative defense in Solanum nigrum L. under cadmium stress by application of cadmium-resistant strain and citric acid. J. Haz. Mat. 181, 771–777. doi: 10.1016/j.jhazmat.2010.05.080
Ghavami, N., Alikhani, H. A., Pourbabaei, A. A., and Besharati, H. (2017). Effects of two new siderophore-producing rhizobacteria on growth and iron content of maize and canola plants. J. Plant Nut. 40, 736–746. doi: 10.1080/01904167.2016.1262409
Ghoul, M., and Mitri, S. (2016). The ecology and evolution of microbial competition. Trends Microbiol. 24, 833–845. doi: 10.1016/j.tim.2016.06.011
Glick, B. R. (2014). Bacteria with ACC deaminase can promote plant growth and help to feed the world. Microbiol. Res. 169, 30–39. doi: 10.1016/j.micres.2013.09.009
Goswami, D., Thakker, J. N., and Dhandhukia, P. C. (2016). Portraying mechanics of plant growth promoting rhizobacteria (PGPR): a review. Cogent Food Agric. 2:1127500. doi: 10.1080/23311932.2015.1127500
Goswami, M., and Suresh, D. (2020). Plant growth-promoting rhizobacteria-alleviators of abiotic stresses in soil: a review. Pedosphere. 30, 40–61. doi: 10.1016/S1002-0160(19)60839-8
Grover, M., Madhubala, R., Ali, S. Z., Yadav, S. K., and Venkateswarlu, B. (2014). Influence of Bacillus spp. strains on seedling growth and physiological parameters of sorghum under moisture stress conditions. J. Basic Microbiol. 54, 951–961. doi: 10.1002/jobm.201300250
Guo, J., Muhammad, H., Lv, X., Wei, T., Ren, X., Jia, H., et al. (2020). Prospects and applications of plant growth promoting rhizobacteria to mitigate soil metal contamination: a review. Chemosphere. 246:125823. doi: 10.1016/j.chemosphere.2020.125823
Gupta, B., and Huang, B. (2014). Mechanism of salinity tolerance in plants: physiological, biochemical, and molecular characterization. Int. J. Genom. 2014:701596. doi: 10.1155/2014/701596
Gupta, P., and Kumar, V. (2017). Value added phytoremediation of metal stressed soils using phosphate solubilizing microbial consortium. World J. Microbiol. Biotechnol. 33, 1–15. doi: 10.1007/s11274-016-2176-3
Hameeda, B., Harini, G., Rupela, O. P., Wani, S. P., and Reddy, G. (2008). Growth promotion of maize by phosphate-solubilizing bacteria isolated from composts and macrofauna. Microbiol. Res. 163, 234–242. doi: 10.1016/j.micres.2006.05.009
Hamid, B., Zaman, M., Farooq, S., Fatima, S., Sayyed, R. Z., Baba, Z. A., et al. (2021). Bacterial plant biostimulants: a sustainable way towards improving growth, productivity, and health of crops. Sustainability. 13:2856. doi: 10.3390/su13052856
Hashami, S. Z., Nakamura, H., Ohkama-Ohtsu, N., Kojima, K., Djedidi, S., Fukuhara, I., et al. (2019). Evaluation of immune responses induced by simultaneous inoculations of soybean (Glycine max [L.] Merr.) with soil bacteria and rhizobia. Microbes Environ. 2019:ME18110. doi: 10.1264/jsme2.ME18110
Hassan, T. U., Bano, A., and Naz, I. (2017). Alleviation of heavy metals toxicity by the application of plant growth-promoting rhizobacteria and effects on wheat grown in saline-sodic field. Int. J. Phytoremed. 19, 522–529. doi: 10.1080/15226514.2016.1267696
Hayat, S., Hayat, Q., Alyemeni, M. N., Wani, A. S., Pichtel, J., and Ahmad, A. (2012). Role of proline under changing environments: a review. Plant Signal. Beh. 7, 1456–1466. doi: 10.4161/psb.21949
He, Y., Pantigoso, H. A., Wu, Z., and Vivanco, J. M. (2019). Co-inoculation of Bacillus sp. and Pseudomonas putida at different development stages acts as a biostimulant to promote growth, yield and nutrient uptake of tomato. J. Appl. Microbiol. 127, 196–207. doi: 10.1111/jam.14273
Hobman, J. L., and Crossman, L. C. (2015). Bacterial antimicrobial metal ion resistance. J. Med. Microbiol. 64, 471–497. doi: 10.1099/jmm.0.023036-0
Huang, B., DaCosta, M., and Jiang, Y. (2014). Research advances in mechanisms of turfgrass tolerance to abiotic stresses: from physiology to molecular biology. Crit. Rev. Plant Sci. 33, 141–189. doi: 10.1080/07352689.2014.870411
Jacoby, R. P., and Kopriva, S. (2019). Metabolic niches in the rhizosphere microbiome: new tools and approaches to analyse metabolic mechanisms of plant–microbe nutrient exchange. J. Exp. Bot. 70, 1087–1094. doi: 10.1093/jxb/ery438
Jeyarajan, R., and Nakkeeran, S. (2000). “Exploitation of microorganisms and viruses as biocontrol agents for crop disease management,” in Biocontrol Potential and its Exploitation in Sustainable Agriculture, eds R. K. Upadhyay, K. G. Mukerji, and B. P. Chamola (Boston, MA: Springer), 95–116. doi: 10.1007/978-1-4615-4209-4_8
Jones, D. L., Nguyen, C., and Finlay, R. D. (2009). Carbon flow in the rhizosphere: carbon trading at the soil–root interface. Plant Soil. 321, 5–33. doi: 10.1007/s11104-009-9925-0
Kanchiswamy, C. N., Malnoy, M., and Maffei, M. E. (2015). Chemical diversity of microbial volatiles and their potential for plant growth and productivity. Front. Plant Sci. 6:151. doi: 10.3389/fpls.2015.00151
Kaneko, T., Nakamura, Y., Sato, S., Asamizu, E., Kato, T., Sasamoto, S., et al. (2000). Complete genome structure of the nitrogen-fixing symbiotic bacterium Mesorhizobium loti. DNA Res. 7, 331–338. doi: 10.1093/dnares/7.6.331
Kapoor, D., Sharma, R., Handa, N., Kaur, H., Rattan, A., Yadav, P., et al. (2015). Redox homeostasis in plants under abiotic stress: role of electron carriers, energy metabolism mediators and proteinaceous thiols. Front. Environ. Sci. 3:13. doi: 10.3389/fenvs.2015.00013
Karthik, C., and Arulselvi, P. I. (2017). Biotoxic effect of chromium (VI) on plant growth-promoting traits of novel Cellulosimicrobium funkei strain AR8 isolated from Phaseolus vulgaris rhizosphere. Geomicrobiol. J. 34, 434–442. doi: 10.1080/01490451.2016.1219429
Khatoon, Z., Huang, S., Rafique, M., Fakhar, A., Kamran, M. A., and Santoyo, G. (2020). Unlocking the potential of plant growth-promoting rhizobacteria on soil health and the sustainability of agricultural systems. J. Environ. Manag. 273, 111–118. doi: 10.1016/j.jenvman.2020.111118
Kiani, S. P., Talia, P., Maury, P., Grieu, P., Heinz, R., Perrault, A., et al. (2007). Genetic analysis of plant water status and osmotic adjustment in recombinant inbred lines of sunflower under two water treatments. Plant Sci. 172, 773–787. doi: 10.1016/j.plantsci.2006.12.007
Koenig, R. L., Morris, R. O., and Polacco, J. C. (2002). tRNA is the source of low-level trans-zeatin production in Methylobacterium spp. J. Bacteriol. 184, 1832–1842. doi: 10.1128/JB.184.7.1832-1842.2002
Kuan, K. B., Othman, R., Abdul Rahim, K., and Shamsuddin, Z. H. (2016). Plant growth-promoting rhizobacteria inoculation to enhance vegetative growth, nitrogen fixation and nitrogen remobilisation of maize under greenhouse conditions. PLoS ONE 11:e0152478. doi: 10.1371/journal.pone.0152478
Kumar, A., Patel, J. S., Meena, V. S., and Ramteke, P. W. (2019). Plant growth-promoting rhizobacteria: strategies to improve abiotic stresses under sustainable agriculture. J. Plant Nut. 42, 1402–1415. doi: 10.1080/01904167.2019.1616757
Kumar, A., Vandana, R. S., Singh, M., and Pandey, K. D. (2015). Plant Growth Promoting Rhizobacteria (PGPR). A Promising Approach for Disease Management. Microbes and Environmental Management. New Delhi: Studium Press, 195–209.
Lal, S., Ratna, S., Said, O. B., and Kumar, R. (2018). Biosurfactant and exopolysaccharide-assisted rhizobacterial technique for the remediation of heavy metal contaminated soil: an advancement in metal phytoremediation technology. Environ. Tech. Innov. 10, 243–263. doi: 10.1016/j.eti.2018.02.011
Lawrance, S., Varghese, S., Varghese, E. M., and Asok, A. K. (2019). Quinoline derivatives producing Pseudomonas aeruginosa H6 as an efficient bioherbicide for weed management. Biocat. Agric. Biotechnol. 18:101096. doi: 10.1016/j.bcab.2019.101096
Lesk, C., Rowhani, P., and Ramankutty, N. (2016). Influence of extreme weather disasters on global crop production. Nature. 529, 84–87. doi: 10.1038/nature16467
Liu, H., and Brettell, L. E. (2019). Plant defense by VOC-induced microbial priming. Trends Plant Sci. 24, 187–189. doi: 10.1016/j.tplants.2019.01.008
Lopes, M. J. D. S., Dias-Filho, M. B., and Gurgel, E. S. C. (2021). Successful plant growth-promoting microbes: inoculation methods and abiotic factors. Front. Sust. Food Syst. 5:48. doi: 10.3389/fsufs.2021.606454
Lucini, L., Colla, G., Moreno, M. B. M., Bernardo, L., Cardarelli, M., Terzi, V., et al. (2019). Inoculation of Rhizoglomus irregulare or Trichoderma atroviride differentially modulates metabolite profiling of wheat root exudates. Phytochem. 157, 158–167. doi: 10.1016/j.phytochem.2018.10.033
Lucy, M., Reed, E., and Glick, B. R. (2004). Applications of free-living plant growth-promoting rhizobacteria. Antonie van Leeuwenhoek. 86, 1–25. doi: 10.1023/B:ANTO.0000024903.10757.6e
Lum, M. S., Hanafi, M. M., Rafii, Y. M., and Akmar, A. S. N. (2014). Effect of drought stress on growth, proline and antioxidant enzyme activities of upland rice. J. Anim. Plant Sci. 24, 1487–1493.
Mancosu, N., Snyder, R. L., Kyriakakis, G., and Spano, D. (2015). Water scarcity and future challenges for food production. Water. 7, 975–992. doi: 10.3390/w7030975
Manzoor, M., Gul, I., Ahmed, I., Zeeshan, M., Hashmi, I., Amin, B. A. Z., et al. (2019). Metal tolerant bacteria enhanced phytoextraction of lead by two accumulator ornamental species. Chemosphere. 227, 561–569. doi: 10.1016/j.chemosphere.2019.04.093
Miller, G., Suzuki, N., Ciftci-Yilmaz, S., and Mittler, R. (2010). Reactive oxygen species homeostasis and signalling during drought and salinity stresses. Plant Cell Environ. 33, 453–467. doi: 10.1111/j.1365-3040.2009.02041.x
Mishra, J., Prakash, J., and Arora, N. K. (2016). Role of beneficial soil microbes in sustainable agriculture and environmental management. Climate Change Environ. Sust. 4, 137–149. doi: 10.5958/2320-642X.2016.00015.6
Mishra, J., Singh, R., and Arora, N. K. (2017). Alleviation of heavy metal stress in plants and remediation of soil by rhizosphere microorganisms. Front. Microbiol. 8:1706. doi: 10.3389/fmicb.2017.01706
Mohanram, S., and Kumar, P. (2019). Rhizosphere microbiome: revisiting the synergy of plant-microbe interactions. Ann. Microbiol. 69, 307–320. doi: 10.1007/s13213-019-01448-9
Naik, K., Mishra, S., Somei, M., Awano, R., Srichandan, H., Singh, P. K., et al. (2020). Effect of a root growth promoter on selected crops grown in India. Plant Physiol. Rep. 25, 284–297. doi: 10.1007/s40502-020-00515-7
Ngumbi, E., and Kloepper, J. (2016). Bacterial-mediated drought tolerance: current and future prospects. Appl. Soil Ecol. 105, 109–125. doi: 10.1016/j.apsoil.2016.04.009
Nouioui, I., Cortés-Albayay, C., Carro, L., Castro, J. F., Gtari, M., Ghodhbane-Gtari, F., et al. (2019). Genomic insights into plant-growth-promoting potentialities of the genus Frankia. Front. Microbiol. 10:1457. doi: 10.3389/fmicb.2019.01457
Ongena, M., and Jacques, P. (2008). Bacillus lipopeptides: versatile weapons for plant disease biocontrol. Trends Microbiol. 16, 115–125. doi: 10.1016/j.tim.2007.12.009
Osakabe, Y., Osakabe, K., Shinozaki, K., and Tran, L. S. P. (2014). Response of plants to water stress. Front. Plant Sci. 5:86. doi: 10.3389/fpls.2014.00086
Ouhaibi-Ben Abdeljalil, N., Vallance, J., Gerbore, J., Rey, P., and Daami-Remadi, M. (2016). Bio-suppression of Sclerotinia stem rot of tomato and biostimulation of plant growth using tomato-associated rhizobacteria. J. Plant. Pathol. Microbiol. 7:2. doi: 10.4172/2157-7471.1000331
Pagnanelli, F., Viggi, C. C., and Toro, L. (2010). Development of new composite biosorbents from olive pomace wastes. Appl. Surf. Sci. 256, 5492–5497. doi: 10.1016/j.apsusc.2009.12.146
Papik, J., Folkmanova, M., Polivkova, M., Suman, J., and Uhlik, O. (2020). The invisible life inside plants: deciphering the riddles of endophytic bacterial diversity. Biotechnol. Adv. 44:107614. doi: 10.1016/j.biotechadv.2020.107614
Paramanandham, P., Rajkumari, J., Pattnaik, S., and Busi, S. (2017). Biocontrol potential against Fusarium oxysporum sp. lycopersici and Alternaria solani and tomato plant growth due to Plant Growth–Promoting Rhizobacteria. Int. J. Veg. Sci. 23, 294–303. doi: 10.1080/19315260.2016.1271850
Pausch, J., and Kuzyakov, Y. (2018). Carbon input by roots into the soil: quantification of rhizodeposition from root to ecosystem scale. Global Change Biol. 24, 1–12. doi: 10.1111/gcb.13850
Ponomarova, O., Gabrielli, N., Sévin, D. C., Mülleder, M., Zirngibl, K., Bulyha, K., et al. (2017). Yeast creates a niche for symbiotic lactic acid bacteria through nitrogen overflow. Cell Sys. 5, 345–357. doi: 10.1016/j.cels.2017.09.002
Príncipe, A., Fernandez, M., Torasso, M., Godino, A., and Fischer, S. (2018). Effectiveness of tailocins produced by Pseudomonas fluorescens SF4c in controlling the bacterial-spot disease in tomatoes caused by Xanthomonas vesicatoria. Microbiol. Res. 212, 94–102. doi: 10.1016/j.micres.2018.05.010
Radhakrishnan, R., and Baek, K. H. (2017). Physiological and biochemical perspectives of non-salt tolerant plants during bacterial interaction against soil salinity. Plant Physiol. Biochem. 116, 116–126. doi: 10.1016/j.plaphy.2017.05.009
Rajkumar, M., Sandhya, S., Prasad, M. N. V., and Freitas, H. (2012). Perspectives of plant-associated microbes in heavy metal phytoremediation. Biotechnol. Adv. 30, 1562–1574. doi: 10.1016/j.biotechadv.2012.04.011
Rakshiya, Y. S., Verma, M. K., and Sindhu, S. S. (2016). Efficacy of antagonistic soil bacteria in the management of subterranean termites (Isoptera). Res. Environ. Life Sci. 9, 949–955.
Ramadan, E. M., AbdelHafez, A. A., Hassan, E. A., and Saber, F. M. (2016). Plant growth-promoting rhizobacteria and their potential for biocontrol of phytopathogens. Afr. J. Microbiol. Res. 10, 486–504. doi: 10.5897/AJMR2015.7714
Rasool, S., Hameed, A., Azooz, M. M., Siddiqi, T. O., and Ahmad, P. (2013). “Salt stress: causes, types and responses of plants,” in Ecophysiology and Responses of Plants Under Salt Stress. (New York, NY: Springer), 1–24. doi: 10.1007/978-1-4614-4747-4_1
Rathore, A. S., and Gupta, R. D. (2015). Chitinases from bacteria to human: properties, applications, and future perspectives. Enzyme Res. 2015:791907. doi: 10.1155/2015/791907
Ravanbakhsh, M., Sasidharan, R., Voesenek, L. A., Kowalchuk, G. A., and Jousset, A. (2017). ACC deaminase-producing rhizosphere bacteria modulate plant responses to flooding. J. Ecol. 105, 979–986. doi: 10.1111/1365-2745.12721
Reichman, S. M. (2014). Probing the plant growth-promoting and heavy metal tolerance characteristics of Bradyrhizobium japonicum CB1809. Eur. J. Soil Biol. 63, 7–13. doi: 10.1016/j.ejsobi.2014.04.001
Rengasamy, P. (2002). Transient salinity and subsoil constraints to dryland farming in Australian sodic soils: an overview. Aus. J. Exp. Agricul. 42, 351–361. doi: 10.1071/EA01111
Rooney, W. M., Grinter, R. W., Correia, A., Parkhill, J., Walker, D. C., and Milner, J. J. (2020). Engineering bacteriocin-mediated resistance against the plant pathogen Pseudomonas syringae. Plant Biotechnol. J. 18, 1296–1306. doi: 10.1111/pbi.13294
Santoro, M. V., Bogino, P. C., Nocelli, N., Cappellari, L. D. R., Giordano, W. F., and Banchio, E. (2016). Analysis of plant growth-promoting effects of fluorescent Pseudomonas strains isolated from Mentha piperita rhizosphere and effects of their volatile organic compounds on essential oil composition. Front. Microbiol. 7:1085. doi: 10.3389/fmicb.2016.01085
Santoyo, G., Pacheco, C. H., Salmerón, J. H., and León, R. H. (2017). The role of abiotic factors modulating the plant-microbe-soil interactions: toward sustainable agriculture. A review. Spanish J. Agricul. Res. 15:13. doi: 10.5424/sjar/2017151-9990
Sasidharan, R., and Voesenek, L. A. (2015). Ethylene-mediated acclimations to flooding stress. Plant Physiol. 169, 3–12. doi: 10.1104/pp.15.00387
Selvakumar, G., Mohan, M., Kundu, S., Gupta, A. D., Joshi, P., Nazim, S., et al. (2008). Cold tolerance and plant growth promotion potential of Serratia marcescens strain SRM (MTCC 8708) isolated from flowers of summer squash (Cucurbita pepo). Let. Appl. Microbiol. 46, 171–175. doi: 10.1111/j.1472-765X.2007.02282.x
Sharma, R. K., and Archana, G. (2016). Cadmium minimization in food crops by cadmium resistant plant growth promoting rhizobacteria. Appl. Soil Ecol. 107, 66–78. doi: 10.1016/j.apsoil.2016.05.009
Sharma, S., Chen, C., Navathe, S., Chand, R., and Pandey, S. P. (2019). A halotolerant growth promoting rhizobacteria triggers induced systemic resistance in plants and defends against fungal infection. Sci. Rep. 9, 1–17. doi: 10.1038/s41598-019-40930-x
Shrivastava, P., and Kumar, R. (2015). Soil salinity: A serious environmental issue and plant growth promoting bacteria as one of the tools for its alleviation. Saudi J Biol. Sci. 22, 123–131. doi: 10.1016/j.sjbs.2014.12.001
Skirycz, A., and Inzé, D. (2010). More from less: plant growth under limited water. Cur. Opin. Biotechno. 21, 197–203. doi: 10.1016/j.copbio.2010.03.002
Slepetiene, A., Volungevicius, J., Jurgutis, L., Liaudanskiene, I., Amaleviciute-Volunge, K., Slepetys, J., et al. (2020). The potential of digestate as a biofertilizer in eroded soils of Lithuania. Waste Manag. 102, 441–451. doi: 10.1016/j.wasman.2019.11.008
Sriprang, R., Hayashi, M., Ono, H., Takagi, M., Hirata, K., and Murooka, Y. (2003). Enhanced accumulation of Cd2+ by a Mesorhizobium sp. transformed with a gene from Arabidopsis thaliana coding for phytochelatin synthase. Appl. Environ. Microbiol. 69, 1791–1796. doi: 10.1128/AEM.69.3.1791-1796.2003
Staudt, C., Horn, H., Hempel, D. C., and Neu, T. R. (2004). Volumetric measurements of bacterial cells and extracellular polymeric substance glycoconjugates in biofilms. Biotechnol. Bioeng. 88, 585–592. doi: 10.1002/bit.20241
Tahir, H. A., Gu, Q., Wu, H., Raza, W., Hanif, A., Wu, L., et al. (2017). Plant growth promotion by volatile organic compounds produced by Bacillus subtilis SYST2. Front. Microbiol. 8:171. doi: 10.3389/fmicb.2017.00171
Timmusk, S., Abd El-Daim, I. A., Copolovici, L., Tanilas, T., Kännaste, A., Behers, L., et al. (2014). Drought-tolerance of wheat improved by rhizosphere bacteria from harsh environments: enhanced biomass production and reduced emissions of stress volatiles. PLoS ONE 9:e96086. doi: 10.1371/journal.pone.0096086
Vaikundamoorthy, R., Rajendran, R., Selvaraju, A., Moorthy, K., and Perumal, S. (2018). Development of thermostable amylase enzyme from Bacillus cereus for potential antibiofilm activity. Bioorganic Chem. 77, 494–506. doi: 10.1016/j.bioorg.2018.02.014
Vejan, P., Abdullah, R., Khadiran, T., Ismail, S., and Nasrulhaq Boyce, A. (2016). Role of plant growth promoting rhizobacteria in agricultural sustainability-a review. Molecules. 21:573. doi: 10.3390/molecules21050573
Venkateswarlu, B., Desai, S., and Prasad, Y. G. (2008). Agriculturally important microorganisms for stressed ecosystems: challenges in technology development and application. Agricul. Imp. Micro.1, 225–246.
Verma, P., Yadav, A. N., Khannam, K. S., Kumar, S., Saxena, A. K., and Suman, A. (2016). Molecular diversity and multifarious plant growth promoting attributes of Bacilli associated with wheat (Triticum aestivum L.) rhizosphere from six diverse agro-ecological zones of India. J. Basic Microbiol. 56, 44–58. doi: 10.1002/jobm.201500459
Wang, C. J., Yang, W., Wang, C., Gu, C., Niu, D. D., Liu, H. X., et al. (2012). Induction of drought tolerance in cucumber plants by a consortium of three plant growth-promoting Rhizobacterium strains. PLoS ONE 7:e52565. doi: 10.1371/journal.pone.0052565
Wang, X., Mavrodi, D. V., Ke, L., Mavrodi, O. V., Yang, M., Thomashow, L. S., et al. (2015). Biocontrol and plant growth-promoting activity of rhizobacteria from Chinese fields with contaminated soils. Microb. Biotechnol. 8, 404–418. doi: 10.1111/1751-7915.12158
Wani, P. A., and Khan, M. S. (2012). Bioremediaiton of lead by a plant growth promoting Rhizobium species RL9. Bact. J. 2, 66–78. doi: 10.3923/bj.2012.66.78
Wheaton, G., Counts, J., Mukherjee, A., Kruh, J., and Kelly, R. (2015). The confluence of heavy metal biooxidation and heavy metal resistance: implications for bioleaching by extreme thermoacidophiles. Minerals. 5, 397–451. doi: 10.3390/min5030397
Wozniak, M., Gałazka, A., Tyśkiewicz, R., and Jaroszuk-Sciseł, J. (2019). Endophytic bacteria potentially promote plant growth by synthesizing different metabolites and their phenotypic/physiological profiles in the biolog GEN III MicroPlateTM Test. Int. J. Mol. Sci. 20, 5283. doi: 10.3390/ijms20215283
Wu, S. C., Cheung, K. C., Luo, Y. M., and Wong, M. H. (2006). Effects of inoculation of plant growth-promoting rhizobacteria on metal uptake by Brassica juncea. Environ. Pol. 140, 124–135. doi: 10.1016/j.envpol.2005.06.023
Yanni, Y. G., Rizk, R. Y., Abd El-Fattah, F. K., Squartini, A., Corich, V., Giacomini, A., et al. (2001). The beneficial plant growth-promoting association of Rhizobium leguminosarum bv. trifolii with rice roots. Funct. Plant Biol. 28, 845–870. doi: 10.1071/PP01069
Yavar, A., Sarmani, S., Hamzah, A., and Khoo, K. S. (2014). Phytoremediation of mercury contaminated soil using Scripus mucronatus exposed by bacterial. Int. Conf. Ag. Eco. Med. Sci. 7:6. doi: 10.15242/IICBE.C0214072
Zafar-ul-Hye, M., Ahmad, M., and Shahzad, S. M. (2013). Short Communication Synergistic effect of rhizobia and plant growth promoting rhizobacteria on the growth and nodulation of lentil seedlings under axenic conditions. Soil Environ. 32, 79–86.
Zaidi, S., Usmani, S., Singh, B. R., and Musarrat, J. (2006). Significance of Bacillus subtilis strain SJ-101 as a bioinoculant for concurrent plant growth promotion and nickel accumulation in Brassica juncea. Chemosphere. 64, 991–997. doi: 10.1016/j.chemosphere.2005.12.057
Zhang, X., Baars, O., and Morel, F. M. (2019). Genetic, structural, and functional diversity of low and high-affinity siderophores in strains of nitrogen fixing Azotobacter chroococcum. Metallomics. 11, 201–212. doi: 10.1039/C8MT00236C
Zhou, Q., Chen, Y., Yang, M., Li, W., and Deng, L. (2013). Enhanced bioremediation of heavy metal from effluent by sulfate-reducing bacteria with copper–iron bimetallic particles support. Bioresour. Technol. 136, 413–417. doi: 10.1016/j.biortech.2013.03.047
Zhu, X. F., Jiang, T., Wang, Z. W., Lei, G. J., Shi, Y. Z., Li, G. X., et al. (2012). Gibberellic acid alleviates cadmium toxicity by reducing nitric oxide accumulation and expression of IRT1 in Arabidopsis thaliana. J. Haz. Mat. 239, 302–307. doi: 10.1016/j.jhazmat.2012.08.077
Keywords: PGPR, rhizosphere, hydrogen cyanide, indole acetic acid, nitrobacter, sulfur solubilisation, root microbiome, sustainable agriculture
Citation: Mohanty P, Singh PK, Chakraborty D, Mishra S and Pattnaik R (2021) Insight Into the Role of PGPR in Sustainable Agriculture and Environment. Front. Sustain. Food Syst. 5:667150. doi: 10.3389/fsufs.2021.667150
Received: 12 February 2021; Accepted: 06 May 2021;
Published: 30 June 2021.
Edited by:
Somsubhra Chakraborty, Indian Institute of Technology Kharagpur, IndiaReviewed by:
Durgesh K. Jaiswal, Banaras Hindu University, IndiaSuprasanna Penna, Bhabha Atomic Research Centre (BARC), India
Copyright © 2021 Mohanty, Singh, Chakraborty, Mishra and Pattnaik. This is an open-access article distributed under the terms of the Creative Commons Attribution License (CC BY). The use, distribution or reproduction in other forums is permitted, provided the original author(s) and the copyright owner(s) are credited and that the original publication in this journal is cited, in accordance with accepted academic practice. No use, distribution or reproduction is permitted which does not comply with these terms.
*Correspondence: Snehasish Mishra, c25laGFzaXNoLm1pc2hyYUBnbWFpbC5jb20=; Ritesh Pattnaik, cml0ZXNocGF0dG5haWtAZ21haWwuY29t
†These authors have contributed equally to this work and share first authorship