- 1Department of Plant Production and Protection, NEIKER-Basque Institute for Agricultural Research and Development, Derio, Spain
- 2Department of Conservation of Natural Resources, NEIKER-Basque Institute for Agricultural Research and Development, Derio, Spain
- 3Department of Biotechnology and Crop Protection, IMIDA-Murcian Institute for Agrarian and Food Research and Development, La Alberca, Spain
Phytophthora capsici is one of the oomycetes that affects protected pepper crops in different agroclimatic areas of Spain. Currently, environmentally friendly strategies such as biodisinfestation for plant disease control have become increasingly popular. In this study, the effect of released gases during biodisinfestation with a fresh manures mixture amendment on P. capsici oospore viability was determined. A biodisinfestation trial was performed in a greenhouse located in northern Spain (Biscay), with a mixture of fresh sheep (2 kg m−2) and dry poultry manures (0.5 kg m−2) followed by soil sealing with a transparent polyethylene plastic film for 21 days (onset June 15th). Gases were sampled from the aerial cavity of biodisinfested plots at different days after soil sealing (0–1–2–3–4–7–9–11, and 14 days). Vacutainer tubes were incubated at 20°C with oospores of P. capsici that were previously placed under vacuum and refilled with extracted gases. Treatments assayed were gases from different sampling times (0–1–2–3–4–7–9–11–14 days, and succession of days 1–2–3–4–7–9–11–14) combined with different exposure times (7–14–21 days) at 20°C in the laboratory. Control treatments were included: air-tubes and vacuum-tubes. An additional reference treatment under real field conditions was also considered: buried oospores at 15 cm depth in the biodisinfested plots. Oospore viability was determined with the plasmolysis method. The most effective treatment was the succession of gases collected during all sampling days. The significant but slight reduction in oospore viability by exposure to the different gas treatments was consistent with the low dose of applied amendment and the low soil temperature registered at 15 cm depth during soil biodisinfestation (>25°C−100% time, >35°C−23%, >40°C−3%). The above circumstances might have generated a small quantity of gases with low impact on oospore viability. The biodisinfested soil at 15 cm depth reference treatment showed the lowest oospore viability in all the exposure times assayed. The overlap of thermal and higher biofumigation effects in this treatment could likely be responsible for its greater efficacy. A disinfectant effect purely attributable to released gases throughout biodisinfestation has been demonstrated. We believe that our research will serve as a base for future application in agro-environments with reduced thermal inactivation effects.
Introduction
Phytophthora root rot is a soilborne plant disease of pepper plants (Capsicum annuum L.) that imposes important economic losses for pepper crops worldwide, including the Mediterranean area (De-Cara-García et al., 2018), and areas with a humid temperate climate, such as the Basque Country (Larregla et al., 2015) in Spain. In the Basque Country (northern Spain), the main causal agents of this disease are the oomycetes Phytophthora capsici Leonian and P. cryptogea Pethybridge & Lafferty. The existence of the two complementary mating types of P. capsici in the area allows sexual reproduction, formation of survival spores (oospores) and its adaptability to the environment due to potential genetic variation resulting from meiosis (Etxeberria et al., 2011a). P. capsici is able to survive in soil for extended periods of time due to its oospores that act as the main source of initial inoculum that causes primary infection in the next crop cycle (Erwin and Ribeiro, 1996).
Strategies recommended for management of Phytophthora root rot involved integrated approaches that focus on cultural practices. For many years, plant disease control was broadly based in pre-plant chemical fumigation. However, the use of chemical fumigants involves human health risks, environmental regulations, and economic restraints (Rosskopf et al., 2020). Thus, at the present time, there is a growing pursuit for the use of secure, healthy, and environmentally friendly strategies.
Several strategies based on the use of organic amendments for soilborne disease management have been studied to improve its efficacy and to elucidate the soil disease suppression action mechanisms that are directly or indirectly involved in their application (Bonanomi et al., 2010, 2018; Gamliel and Stapleton, 2017; Rosskopf et al., 2020). One of these approaches combines soil solarization with organic amendments for an improved control of soilborne pests, named “biosolarization” (Katan, 2005; Ros et al., 2008) or “biodisinfestation” (de la Fuente et al., 2009). The term soil solarization refers to a soil disinfestation method which uses passive solar heating of moist soil mulched with transparent plastic sheeting for the control of pathogens, mainly through a direct thermal inactivation mechanism (Katan et al., 1976; Stapleton, 2000). Furthermore, the term biofumigation was first coined to define the application of brassicaceous plant material in soil with the aim of controlling soilborne pathogens (Kirkegaard et al., 1993). Since then, it has adopted a broader meaning that includes the use of other organic materials and reflects the mode of action of active volatile compounds that are generated in the soil after incorporation and decomposition of organic amendments (Stapleton, 2000; Stapleton and Bañuelos, 2009). In this study, the term biodisinfestation will be applied for the combined use of an organic amendment and soil plastic tarping without implying that soil heating is the most important mechanism.
Numerous studies have demonstrated the benefits of combining organic amendments with solarization (Gamliel and Stapleton, 1993a,b; Gamliel et al., 2000; Stapleton, 2000). Early on, it was observed that a series of chemical and microbial processes in the soil is activated when the soil is heated by covering it with a transparent plastic film and proper organic material is added as amendments. This includes its vapor and liquid phases as well as processes such as the degradation of organic matter, the release of various volatile and soluble compounds, and changes in the microbial balance of the soil. Most notably, studies suggested these chain reactions produce better control of soilborne pests, including some types that were not well-controlled by either method separately (Gamliel and Stapleton, 2017).
Previous reports have related the release of nitrogenous compounds from animal manures combined with soil solarization to the lethal effect on certain soil microbiota (Oka, 2010; Arriaga et al., 2011; Núñez-Zofío et al., 2011, 2012) and also to the increased crop growth response after treatment (Gamliel and Stapleton, 1993a; Stapleton, 2000). Ammonia (NH3) has been widely reported to negatively affect the survival or germination of certain soilborne oomycetes (Tsao and Oster, 1981; Riga et al., 2000; Arriaga et al., 2011; Núñez-Zofío et al., 2011, 2012), and soilborne fungi and nematodes (Tenuta and Lazarovits, 2002; Oka, 2010; Gandariasbeitia et al., 2021).
In southeastern Spain, biosolarization with fresh sheep manure showed its efficacy in the reduction of Phytophthora spp. (P. capsici and P. nicotianae) soil inoculum and disease control when it was carried out in August or at the beginning of September (Núñez-Zofío et al., 2013; Lacasa et al., 2015) as well as if it was repeated for two or three consecutive years (Guerrero et al., 2005, 2006) under greenhouse conditions. In the humid subtropical climate of south-eastern United States, increased efficacy of soil solarization by cabbage amendment on the reduction of populations of P. capsici and P. nicotianae was observed, but these reductions were not as effective as the chemical fumigant methyl bromide in eliminating the pathogens at a depth of 25 cm under open field conditions. Soil temperature under solarization treatments reached a maximum of 47°C at a 10 cm depth, but only 41°C at 25 cm (Chellemi et al., 1997; Coelho et al., 1999). In the temperate humid climate of northern Spain, biodisinfestation under greenhouse conditions with fresh animal manure in spring (March-April) significantly reduced the P. capsici inoculum survival rate (30.6%) when compared with the non-treated control (61.1%), and the solarization treatment (94.4%) (Núñez-Zofío et al., 2010; Arriaga et al., 2011). Similarly, biodisinfestation in autumn (September-October) with fresh and semicomposted animal manures reduced initial oospore viability by 40%. However, oospores continued to be infective when they were tested in a bioassay with pepper plants (Núñez-Zofío et al., 2011). Greenhouse soil temperature at 15 cm depth did not exceed 33°C in the biodisinfestation treatment with fresh manure in spring or autumn. Repeated biodisinfestation with fresh and semicomposted animal manures during three consecutive crop seasons improved soil quality and provided effective control of pepper Phytophthora root rot in protected crops located in the temperate humid climate of northern Spain, a region where solarization has been proven non-effective. The repeated manure applications allowed for a reduction in the dose of organic amendment needed for an effective disease control (Núñez-Zofío et al., 2010, 2011, 2012; Arriaga et al., 2011).
One advantage of biodisinfestation is that it can be applied in organic production as well as conventional farming (Guerrero et al., 2013; Gamliel and Stapleton, 2017). Furthermore, the use of these strategies can not only be observed from a crop and soil health point of view, but also from a circular bioeconomy or a life cycle assessment point of view (Oldfield et al., 2017). This is due to the upcycling of the extremely heterogeneous organic materials, such as green and animal manures along with agro-industrial by-products that are used as amendments for soil biodisinfestation.
In situations where there is not solar radiation enough, biosolarization effectiveness is reduced and the biofumigation effect acquires more importance. This is the case of the Basque Country (northern Spain), where the greenhouse pepper crop season (March-September) prevents the practice of biodisinfestation in the months of maximum solar radiation (June–August) (Gandariasbeitia et al., 2019; Ojinaga et al., 2020). To better understand the mechanisms involved in the reduction of P. capsici soil inoculum survival previously observed after biodisinfestation with fresh animal manure in spring (Arriaga et al., 2011) or autumn (Núñez-Zofío et al., 2011) in northern Spain, the main objective in the present study was to assess the effect only attributable to gases sampled at different time intervals throughout biodisinfestation on P. capsici oospore viability. This study was carried out in early summer (June) with the aim of increasing the effectiveness of biofumigation component in biodisinfestation. The experiment was carried out at an innocuous controlled temperature for the pathogen (20°C) in order to ensure the absence of oospores inactivation by thermal effects. Oospore viability change rates over exposure time to the different gases sampled throughout the biodisinfestation treatment were also integrated in the study.
Materials and Methods
Field Trial Characteristics
A field trial was established in an experimental greenhouse located at NEIKER Research Station (Derio, 43°18'20” N-−3°53'0” W; Biscay, northern Spain) in spring-summer 2009. The greenhouse had 1,200 m2. Soil type texture was clay loam (46.3% clay, 40.0% silt, and 13.7% sand), with a pH of 6.8, an organic matter content of 55 g kg−1, a total nitrogen content of 2.2 g kg−1, a C/N ratio of 14.2, a phosphorous content of 99.0 mg kg−1 and an electrical conductivity of 1.42 dS m−1. The region has a temperate humid climate with an annual mean temperature of 12°C (maximum mean temperature in summer 25°C) and rainfall of 1,200 mm year−1.
A mixture of fresh sheep manure and dry poultry manure (70%: 30% on a dry weight basis) was added at a dose (fresh weight) of 25,630 kg ha−1 (equivalent to 9,920 kg ha−1 dry weight) and incorporated in the soil until a depth of 20 cm using a rototiller. The applied amendment mixture had the following characteristics: pH 8.3 and EC 10.1 dS m−1, organic matter: 587 g kg−1, total N: 29.4 g kg−1, total P: 16.1 g kg−1, total K: 30.9 g kg−1 and a relation C/N = 11.6. The applied amendment represented a total nitrogen fertilization dose of 292 kg N ha−1 which was consistent with the advised rates for green pepper greenhouse fertilization in the region (CBPA, 2011) for an expected pepper fruit yield of 57,600 kg ha−1. The organic nitrogen mineralization rates for the sheep and poultry manures in the first year after the amendment mixture application were estimated at 45 and 75% respectively, which provided a mineral nitrogen dose of 170 kg ha−1. This amount was equivalent to the advised mineral nitrogen fertilization dose based on the green pepper crop yield in the region (3 kg N t−1 yield x yield 57.6 t ha−1 = 173 kg N ha−1). A drip irrigation system (comprised of 2 L h−1 emitters spaced 0.40 m apart in the same row, with 0.50 m between drip rows) was placed over the amended soil. Amended soil was then sealed with a transparent polyethylene plastic film 0.05 mm thick and was moistened the first day for 4.5 h, and for another 4.5 h on the second day, equivalent to a total volume of water of 90 L m−2. Amended and moistened soil remained sealed for approximately 3 weeks (from June 15th to July 6th).
Throughout the experiment, ambient and soil temperature (15 cm depth) were registered using sensors connected to a data-logger (HOBO H21-002 Micro Station, Onset Computer Corporation, USA).
Production of Oospores
Oospores of P. capsici were produced in vitro by pairing isolates of different mating types on soft pea agar (filtered cooking broth of 200 g L−1 of peas in distilled water for 30 min was supplemented with 7.5 g of agar and autoclaved at 121°C for 20 min) in order to assist oospore extraction according to the method proposed by Pittis and Shattock (1994). The agar was supplemented with 0.1 g L−1 β-sitosterol to increase oospore formation. Spanish isolates from pepper (00/004, 02/206 and 06-13-03) of the A1 genetic compatibility type were mated with A2 isolates (CBS 554.88 and CBS 370.72) from the Dutch Type Culture Collection (Centraalbureau voor Schimmelcultures, CBS). After 4 weeks of incubation in the dark at 20°C, oospores were extracted from the agar by blending in sterile distilled water (10 mL plate−1). The oospore suspension was filtered through a 100 μm nylon mesh and oospores were then placed in 1 x 1 cm2 25 μm nylon meshes (Sefar Nitex 03 25/19, SEFAR, Switzerland) by vacuum filtration according to the method proposed by Lumsden (1980). At least 500 oospores were placed in each mesh piece, to ensure that a minimum number of 100 oospores were available for counting and observation. The oospores initial viability was determined before treatments and was equal to 41.2 ± 1.5% (mean ± standard error). Oospores viability percentage values were relativized to the initial viability value which was equal to 100 ± 3.6%.
Sampling of Buried Oospores From the Greenhouse Biodisinfestation Trial
In the case of the biodisinfested soil control treatment at 15 cm depth (SOIL; see treatment abbreviations in epigraph 2.6), embedded oospores in 25 μm nylon meshes were placed within 5 × 5 cm bags made from permeable 1,550 μm nylon mesh (Sefar Nitex 06-1550/60). A total of three bags were buried at 15 cm soil depth in each greenhouse experimental plot in one location (1 location × 1 depth × 3 exposure times) before the biodisinfestation treatment onset. One bag from each greenhouse biodisinfested plot and location at 15 cm depth was removed after 7, 14, and 21 days from the treatment onset.
Sampling of Gases From the Greenhouse Biodisinfestation Trial
Several gas-tight silicone plugs were inserted in the sealing plastic film of each greenhouse biodisinfested plot and were placed two meter away from the edge of the plastic film in each plot. Gases produced during soil biodisinfestation were sampled at different time intervals in the aerial cavity between the amended soil surface and the plastic film. The samples were taken with a plastic syringe (50 mL volume) that was punctured into the silicone plugs of the plastic film.
Oospores Incubation in Laboratory Controlled Conditions With Gases From the Greenhouse Biodisinfestation Trial
Embedded oospores in 25 μm nylon meshes were placed in 10 mL volume Vacutainer® (BD, Plymouth, England) glass tubes, with each tube containing one nylon mesh. The vacutainer tubes containing oospores were closed with a hermetic silicone septum and maintained at 20°C in a precision stove in the laboratory until they were used for collecting gas samples in the greenhouse biodisinfestation trial. A vacuum was created in each vacutainer tube containing oospores by means of a syringe. Next, gases sampled from the aerial cavity of the greenhouse biodisinfested plots were injected into the tubes. Once the tubes were filled with the gases, they were transported back to the laboratory and incubated in the stove at 20°C. The duration of the journey was <2 h and the tubes with oospores were transported in a portable fridge to maintain temperatures of 20–25°C, a range considered to be innocuous for P. capsici oospores viability, as reported by Etxeberria et al. (2011b). Vacutainer tubes containing oospores and gases samples collected in the greenhouse were incubated at 20°C in a precision stove in the dark with an exposure time of 7, 14, and 21 days for each Biodisinfestation gas, as well as the two control treatments C_va and C_ai (see treatment abbreviations in epigraph 2.6).
Treatments and Experimental Design
The samples of biodisinfestation gases were obtained from three points located in the center (two meters from the end of the plastic) of each of three biodisinfested plots located in the central zone of the greenhouse. The plot size was 24 m x 4 m. The samples of gases released in each biodisinfested replicate plot were taken at different time intervals from the onset of biodisinfestation (days after setting sealing plastic film): 0, 1, 2, 3, 4, 7, 9, 11, 14, and succession of sampling days 1–2–3–4–7–9–11–14.
The experiment was factorial with a treatment structure of two crossed factors: (i) Biodisinfestation gas (gases sampled at different time intervals from biodisinfestation onset) with thirteen levels that included three control treatments: day 0 (G_00), day 1 (G_01), day 2 (G_02), day 3 (G_03), day 4 (G_04), day 7 (G_07), day 9 (G_09), day 11 (G_11), day 14 (G_14), every sampling day (1-2-3-4-7-9-11-14) (G_ev), Control 1 of vacuum. (C_va), Control 2 of air (C_ai), and Control 3 of biodisinfested soil at 15 cm depth (SOIL); and (ii) Exposure time with three levels: 7, 14, and 21 days. These exposure times were selected for incubation of oospores and gases because it has been shown that the formation and release of biotoxic volatile compounds are predominantly found during the first 3 weeks from the biodisinfestation onset (Gamliel and Stapleton, 2017). Thus, the total number of combined treatments for the two crossed factors was 39. The vacuum-tube control treatment (C_va) consisted of vacuum-tubes. The air-tube control treatment (C_ai) consisted of an air sample that was taken outside the greenhouse experiment at the onset of biodisinfestatión. The biodisinfested soil control treatment at 15 cm depth (SOIL) consisted of embedded oospores in 25 μm nylon meshes which were buried at 15 cm soil depth in each of the three replicate plots (see epigraph 2.3).
The experimental unit was the nylon mesh inside each vacutainer tube, where at least 100 oospores were counted and observed for each combined factorial treatment (Biodisinfestation gas x Exposure time) and replicate plot. The factorial treatments were arranged in a complete randomized design of three replicate plots.
Determination of Oospores Survival
At the end of incubation period, oospore survival was determined using the plasmolysis method (Jiang and Erwin, 1990), effective on P. capsici oospores according to Etxeberria et al. (2011a). For each mesh, 100 oospores were counted and observed microscopically and the number of oospores that plasmolyzed was considered viable.
Statistical Analysis
Discrete percent data of oospore viability (number of oospores that plasmolyzed out of the number observed) in each experimental unit (mesh of oospores) was the response variable, for which a binomial distribution was used. The response variable was analyzed using the generalized linear mixed models (GLMM) procedure (proc GLIMMIX) of SAS 9.4 software with a repeated measures mixed model ANOVA of one factor (Biodisinfestation gas) over time (Exposure time). Biodisinfestation gas and Exposure time were considered fixed factors and each replicate plot was a random factor. Each factorial treatment was considered to be nested in each replicate plot. The Laplace direct likelihood approximation method was used to model goodness of fit. A first order autoregressive covariance structure provided the best relation of data over time (lower AICC statistics). The default residual pseudo-likelihood estimation method was used to continue the analysis. All pairwise differences among least squares means within the interaction of Biodisinfestation gas × Exposure time were adjusted for P-values with the Tukey-Kramer test to maintain an overall experiment-wise type I error rate of α = 0.05. For significant interactions, tests of simple effects (Schabenberger and Pierce, 2002) were performed to detect differences. A linear regression model was adjusted for the proportion of viable oospores over exposure time with separate slopes and intercepts for each level of the Biodisinfestation gas factor treatment and to test differences among them according to Stroup (2018). Linear contrasts of different combinations of the Biodisinfestation gas factor levels were tested for significant differences among slopes and intercepts.
Results
Daily Air and Soil Temperatures in the Greenhouse Biodisinfestation Trial
In the greenhouse biodisinfestation trial, the average daily temperatures for ambient air, biodisinfested soil at 15 cm, and 30 cm depth were 25.3, 32.5, and 29.8°C respectively. Maximum daily air temperatures varied from 29.8 to 54.0°C with an average value of 42.1°C and minimum daily air temperatures varied from 11.5 to 19.2°C with an average value of 15.6°C. In the biodisinfested soil at 15 cm depth, maximum daily soil temperatures ranged from 32.9 to 41.4°C with an average value of 36.8°C and minimum daily soil temperatures ranged from 26.4 to 32.2°C with an average value of 29.2°C. In the biodisinfested soil at 30 cm soil depth, maximum daily soil temperatures ranged from 28.8 to 32.6°C with an average value of 30.6°C and minimum daily soil temperatures ranged from 27.6 to 31.4°C with an average value of 29.1°C (Figure 1). In the biodisinfested soil at 15 cm depth, the number of cumulative hours above 30, 35, 37.5, and 40°C were 367, that represented percentages of the biodisinfestation treatment duration of 73, 23, 12, and 3% respectively. In the biodisinfested soil at 30 cm depth, the number of cumulative hours above 30°C was 204 h (40% treatment duration) and no temperature exceeded 32.5°C. The averaged hourly soil temperatures calculated for the total duration of the biodisinfestation treatment showed a temperature variation in each 24-h cycle that ranged from 7°C (36.1–29.1) to 1.2°C (30.4–29.2) at 15 and 30 cm depth respectively.
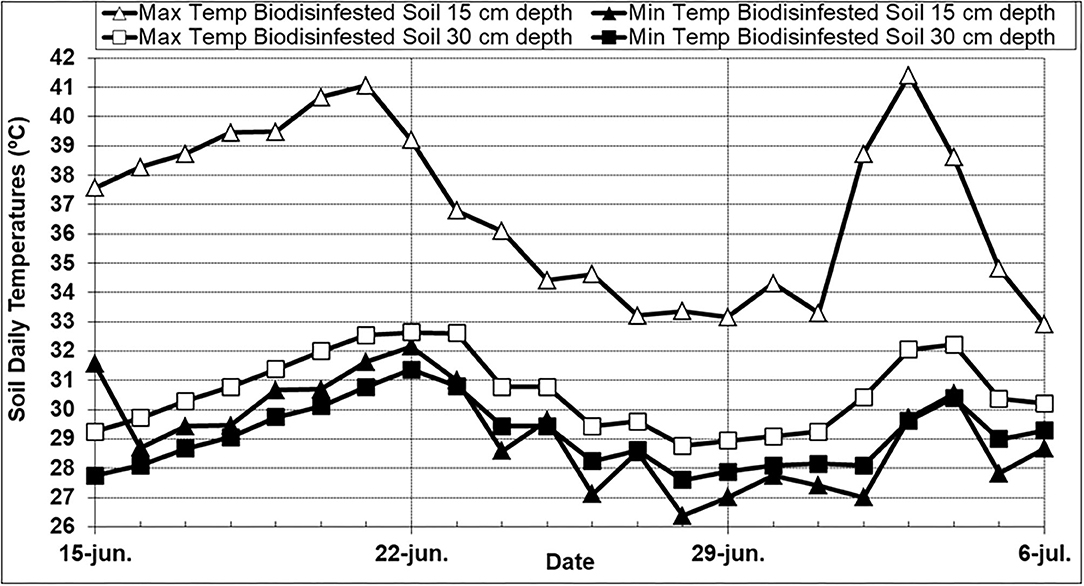
Figure 1. Maximum and minimum daily soil temperatures (15 and 30 cm depth) registered for the 21-day duration (June 15–July 6th) of the biodisinfestation treatment in the greenhouse field trial located in northern Spain (Derio; Biscay). Biodesinfestation amendment was a mixture of fresh sheep and poultry manures (2 + 0.5 kg.m−2). Soil was tarped with a 0.05 mm-thick transparent low density polyethylene plastic film.
Effects of Treatments on Oospore Viability
Gases produced during biodisinfestation and exposure time both affected pathogen survival. Viability of P. capsici oospores was significantly affected by the factors Biodisinfestation gas (P < 0.0001), Exposure time (P < 0.0001) and the interaction Biodisinfestation gas x Exposure time (P < 0.0001) (Table 1). The statistical generalized linear mixed model selected for the binomial data was valid as indicated by the “Generalized Chi-Square/DF” fit statistics which was close to 1 (Generalized Chi-Square/DF = 1.03) (Table 1). Viable oospores were detected under all treatments combinations of gas sampling times and exposure times (Figure 2).
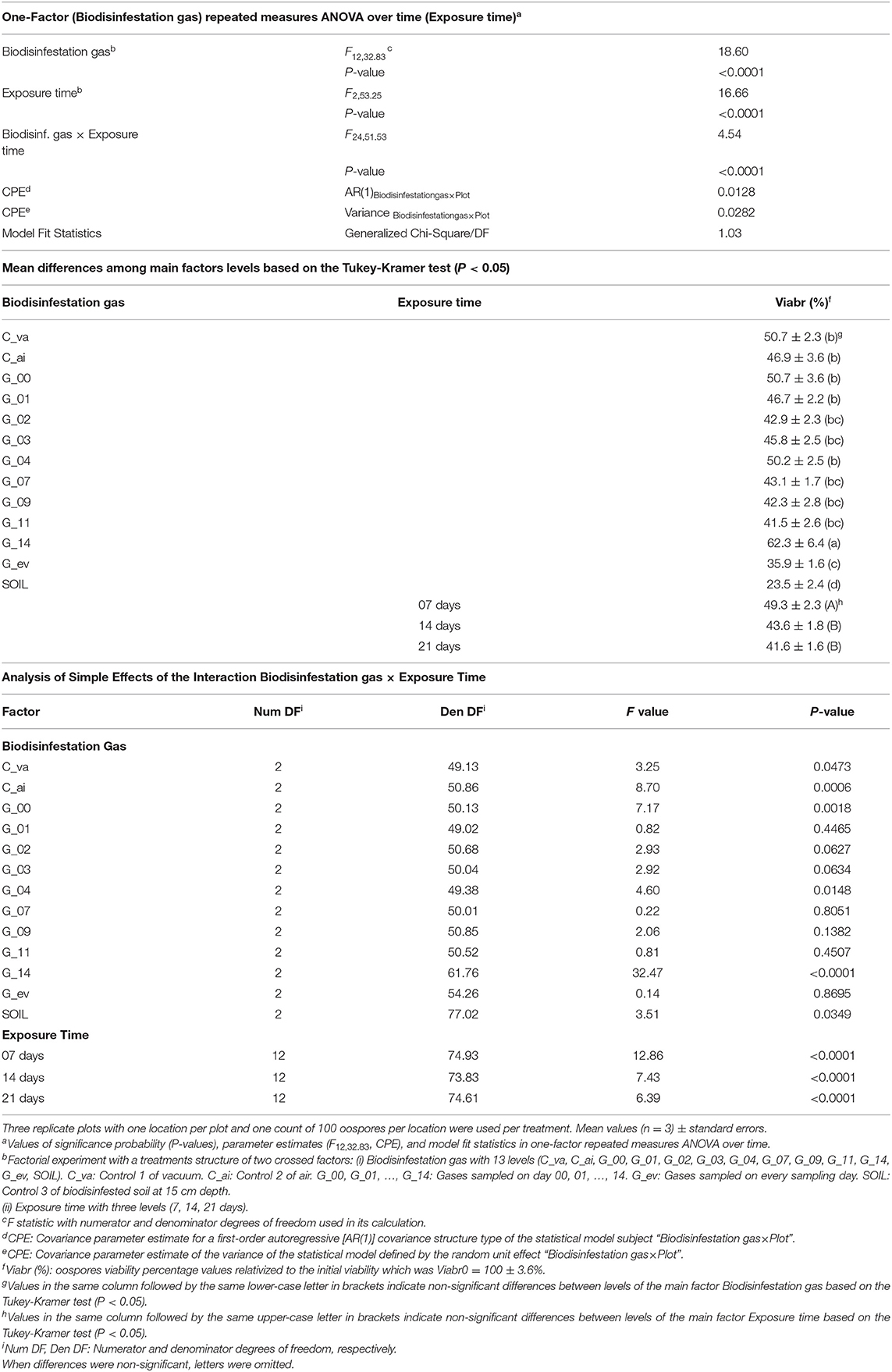
Table 1. Effect of Biodisinfestation gas and Exposure time on Phytophthora capsici oospores viability. Results of analysis of variance, mean differences among main factors levels, and analysis of simple effects of the significant interaction.
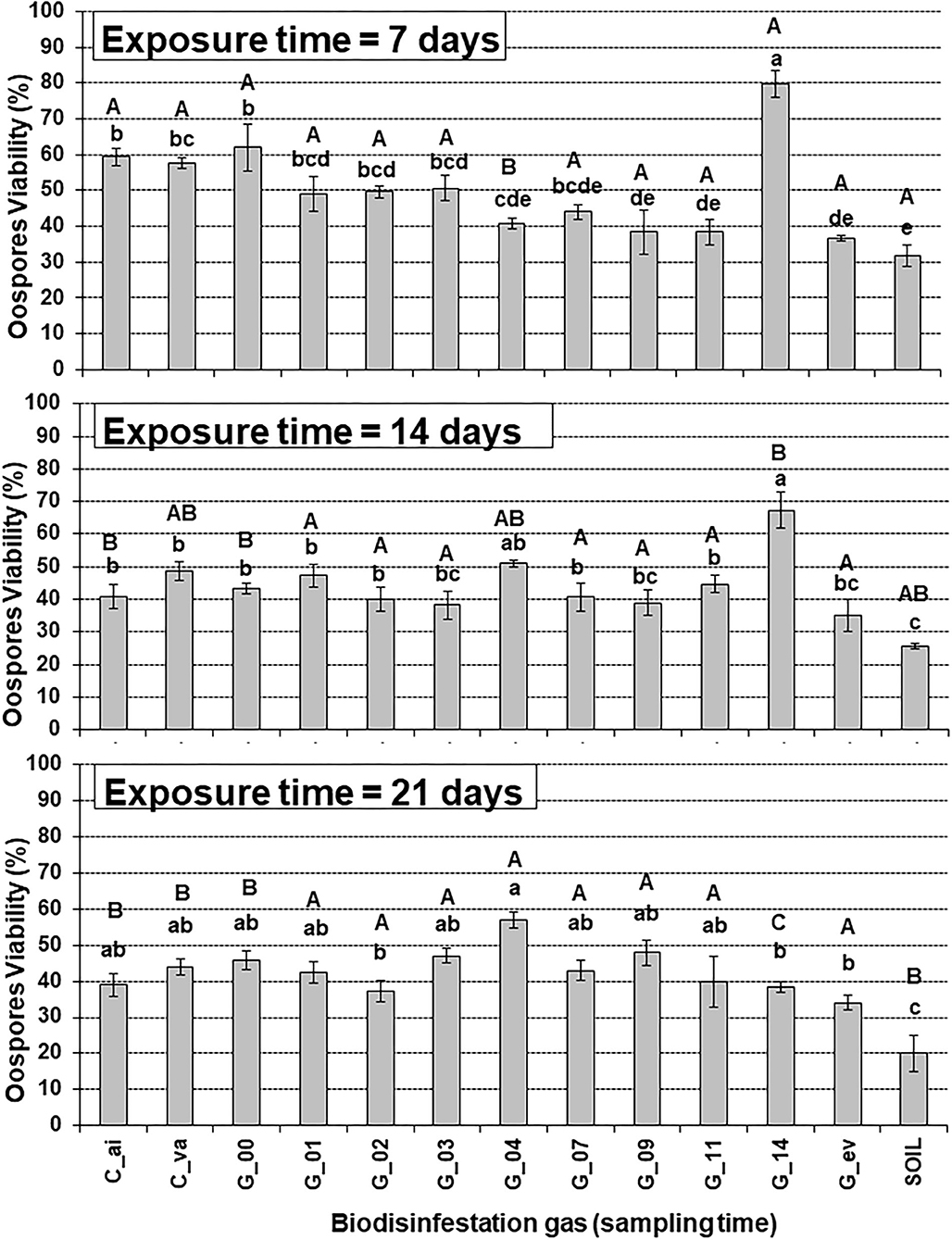
Figure 2. Analysis of the interaction of Biodisinfestation gas and Exposure time on Phytophthora capsici oospores viability. Error bars represent standard error of the mean (n = 3) from three replicate counts of 100 oospores each. Values with different lower-case letter indicate significant differences between Biodisinfestation gas within a given exposure time and upper-case letters compare exposure times for a given Biodisinfestation gas based on the Tukey-Kramer test (P < 0.05). C_va: Control 1 of vacuum. C_ai: Control 2 of air. G_00, G_01, …, G_14: Gases sampled on day 00, 01, …, 14. G_ev: Gases sampled on every sampling day. SOIL: Control 3 of biodisinfested soil at 15 cm depth.
On average, the most effective treatments ordered from higher to lower efficacy, for the main factor Biodisinfestation gas, were: SOIL, G_ev, G_11, G_09, G_07, G_03, and G_02, and for the main factor Exposure time, were: 21 and 14 days (Table 1).
The analysis of simple effects of the interaction Biodisinfestation gas x Exposure time was significant in six of the thirteen levels of the first factor and in all the levels of the second factor respectively, indicating that oospore viability was differently affected by Exposure time within six levels (C_va, C_ai, G_00, G_04, G_14, SOIL) of the Biodisinfestation gas and was also differently affected by Biodisinfestation gas within the three levels of Exposure time (7, 14, and 21 days) (Table 1).
For the shortest exposure time (7 days), the gases sampled at day 9, day 11, and every day (G_09, G_11, G_ev) showed a significant lower viability than the air and vacuum untreated controls (C_ai, C_va) with reduction rate percentages of 34, 34, and 36% when compared with the vacuum control (C_va), respectively (Figure 2). Conversely, for exposure time of 14 days, none of the biodisinfestation gases differ significantly from the untreated controls (Figure 3), with reduction rates comprised between 16 and 21% for gases sampled in days 2, 3, 7, and 9 (G_02, G_03, G_07, G_09), and 28% for every day (G_ev), when compared with the vacuum control (C_va), respectively. For the longer exposure time of 21 days, differences among biodisinfestation gases were reduced and none of them differ significantly from the untreated controls, with highest reduction rates of 15, 9, 13, and 23% for gases sampled in days 2, 11, 14, and every day (G_02, G_11, G_14, G_ev), when compared with the vacuum control (C_va), respectively (Figure 2).
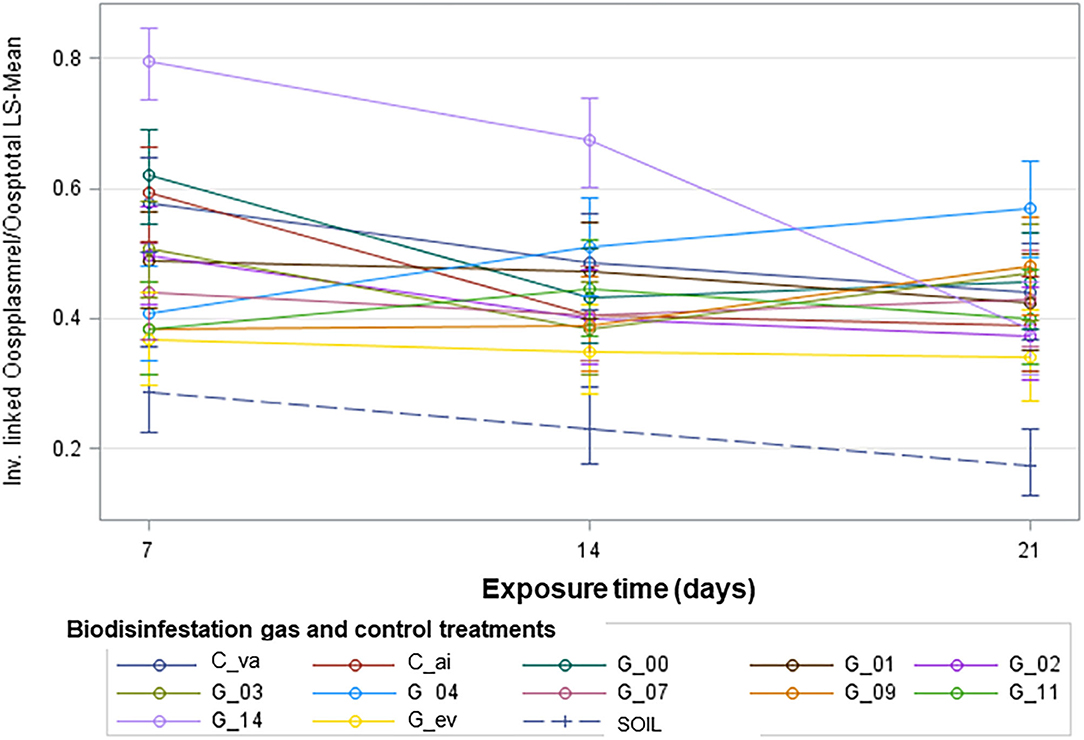
Figure 3. Graphical representation is shown for the pattern of change of the proportion of viable oospores over exposure time for each level of the Biodisinfestation gas factor treatment. Inverse linked of relativized Plasmolyzed oospores/Total oospores Least Square-Means with 95% confidence limits for the interaction Biodisinfestation gas x Exposure time. C_va: Control 1 of vacuum. C_ai: Control 2 of air. G_00, G_01, …, G_14: Gases sampled on day 00, 01, …, 14. G_ev: Gases sampled on every sampling day. SOIL: Control 3 of biodisinfested soil at 15 cm depth.
Gases sampled at day 14 (G_14) produced a significant higher viability than the untreated controls (C_ai, C_va) for exposure times of 7 and 14 days and showed increase rates of 38% and 38% when compared with the vacuum control (C_va), respectively. On the contrary, for the longer exposure time of 21 days, non-significant differences were shown and a decrease rate of 13% was observed when compared with the vacuum control (Figure 2).
The gases sampled every day (G_ev) was the second most effective biodisinfestation gas treatment and was significantly different from untreated controls (C_va, C_ai) for the shorter exposure time (7 days) but was not different for the longer exposure times (14 and 21 days) with oospore viability reduction percentages of 36, 28, and 23% when compared with the vacuum control (C_va) for exposure times of 7, 14, and 21 days, respectively (Figure 2).
In contrast, the biodisinfested soil control treatment at 15 cm depth (SOIL) was always the most effective treatment, significantly different from the untreated controls (C_va, C_ai), and with reduction percentage rates of 45, 47, and 55% when compared with the vacuum control (C_va) for exposure times of 7, 14, and 21 days, respectively (Figure 2).
The graphical analysis of the proportion of viable oospores over exposure time for each biodisinfestation gas treatment appeared to have a different pattern of change (Figure 3). With this in mind, a linear regression model was fitted for the proportion of viable oospores over exposure time with separate slopes for each Biodisinfestation gas type. A first model included a term for lack of fit, in order to test for non-linear trends (Table 2A). The result for the term Biodisinfestation gas * Exposure time (F13, 35.35 = 1.57; P = 0.1425) indicated that there was no evidence of lack of fit from linear regression over Exposure time (Table 2A). This allowed us to move to the next step: drop the lack-of-fit term (Biodisinfestation gas * Exposure time) from the model and focus on the estimated slopes for linear regression of the proportion of viable oospores over Exposure time and whether there was statistical evidence that they differ by Biodisinfestation gas.
A second model of linear regression was fitted and statistically significant evidence was obtained for the model terms (Biodisinfestation Gas (intercept), F13, 71.83 = 10.28, P < 0.0001; E * Biodisinfestation Gas (slope), F13, 77.57 = 8.73, P < 0.0001) (Table 2B). Separate slopes and intercepts for each level of the Biodisinfestation gas factor treatment (Supplementary Table 1) were estimated for the linear regression of the proportion of viable oospores over exposure time. The linear regression model equation was:
where E = Exposure time (days).
Significant differences among intercepts and slopes of the linear regression equations of the proportion of viable oospores over exposure time were tested with linear contrast of different combinations of the Biodisinfestation gas factor levels (Supplementary Table 2). Linear contrast showed that there was evidence of statistically significant difference for slopes and intercepts between the average of vacuum and air untreated controls (C_va, C_ai) vs. the average of biodisinfestation gases (Pintercept = 0.0054; Pslope = 0.0139), the average of untreated controls vs. gases sampled every day (Pintercept = 0.0004; Pslope = 0.0439), the average of untreated controls vs. gases sampled in day 14 (Pintercept <0.0001; Pslope = 0.0001), the average of untreated controls vs. the average of gases sampled in days 1–2–3–4 (Pintercept = 0.0010; Pslope = 0.0021), the average of untreated controls vs. the average of gases sampled in days 7–9–11 (Pintercept <0.0001; Pslope = 0.0001), the average of gases sampled in days 7–9–11 vs. the gases sampled in day 14 (Pintercept <0.0001; Pslope <0.0001), and the average of gases sampled in days 1–2–3–4 vs. the gases sampled in day 14 (Pintercept <0.0001; Pslope <0.0001). Significant differences were also found for slopes of the biodisinfested soil vs. the average of gases sampled in days 1–2–3–4–7–9–11 (Pslope = 0.0124), the biodisinfested soil vs. the average of gases sampled in days 1–2–3–4 (Pslope = 0.0357), and the biodisinfested soil vs. the average of gases sampled in days 7–9–11 (Pslope = 0.0061). Lastly, significant differences were found for intercepts of the gases sampled every day vs. the average of gases sampled in days 1–2–3–4–7–9–11–14 (Pintercept = 0.0328), and the biodisinfested soil vs. the average of gases sampled in days 1–2–3–4–7–9–11–14-every day (Pintercept = 0.0336).
Discussion
Although none of the biodisinfestation gases completely eliminated the P. capsici ooospore viability, a significant effect purely attributable to the gases released at different time intervals from the onset of biodisinfestation was observed in the reduction of inoculum, in agreement with previous studies with other soilborne pathogens and biotoxic volatile compounds generated in solarized organic-amended soil (Gamliel and Stapleton, 1993a,b; Stapleton, 2000; Zhang et al., 2021).
The small effect on oospore viability of sampled biodisinfestation gases in our experiment was consistent with the low-medium dose of organic amendment applied (25,630 kg ha−1) for an intensive greenhouse crop, and with the low-medium temperature registered at 15 cm depth in the biodisinfested soil (only 115, 61, and 13 cumulative hours above 35, 37.5, and 40°C respectively). Both factors could have ultimately resulted in the generation of a small quantity of biotoxic volatile compounds and therefore, in a low impact on the survival of P. capsici oospores, as previously reported with various Phytophthora spp. survival spores (Guerrero et al., 2010; Larregla et al., 2014; Lacasa et al., 2015; Gandariasbeitia et al., 2019) or with other soilborne fungal pathogens survival structures (Gamliel et al., 2000; Stapleton, 2000, Gamliel and Stapleton, 2017).
The low disinfectant efficacy of biodisinfestation gases on oospore viability observed in this study in northern Spain (Biscay) contrasted with the higher efficacy of a previous experiment carried out with the same methodology in southeastern Spain (Murcia) (Larregla et al., 2014). The differences in efficacy between both studies seemed to be closely related to the different applied doses of organic amendment (70,000 kg ha−1 of fresh sheep manure in southeastern Spain vs. 25,630 kg ha−1 in northern Spain) and the 15 cm depth biodisinfested soil temperatures (average daily minimum-maximum values of 37.7–42.1°C in southeastern Spain vs. 29.2–36.8°C in northern Spain). In the trial of this study in northern Spain, the assayed conditions might have been insufficient to achieve the critical threshold (Gamliel and Stapleton, 1993a) which is required for the generation of a sufficient amount of biotoxic gases in the soil atmosphere to affect the oospore survival.
Although no analyses were made for identification of the released gases sampled in the field biodisinfestation trial of this study, ammonia volatilisation, among other volatile compounds, might have contributed to the reduction of oospore survival during biodisinfestation. In a previous experiment carried out in early spring (17th March to 21st April) in the same soil and with the same amendment mixture type applied at a quadruple dose (fresh weight) of 10,000 kg ha−1 (equivalent to 1,360 kg N ha−1), mean ammonia concentration measured in the manure amended soil atmosphere under plastic sheets was 14.8 mg NH3 m−3 and it decreased 45% after 35 days of biodisinfestation (Arriaga et al., 2011). Ammonia volatilisation is regulated by NH4+-N concentration in the soil solution and is modeled by factors such as pH and temperature (Beutier and Renon, 1978). A low soil organic carbon content is also another critical factor in the accumulation of ammonia, while a high level prevents its generation (Tenuta and Lazarovits, 2002). The differences in NH3 concentration between both experiments could be attributed to the different NH4+-N content, which would be determined by the rate of N organic content (Norg) mineralisation. We could hypothesize similar or even slightly higher NH3 concentration in our study when compared with the previous experiment of Arriaga et al. (2011). Indeed, higher NH4+-N availability would be expected by higher rates of Norg mineralisation which are favored by temperature differences during soil biodisinfestation. Average daily temperatures of air and 15 cm depth biodisinfested soil were 25.3 and 32.5°C in the present experiment versus 18.3 and 21.2°C in the previous experiment of Arriaga et al. (2011).
Our results with oomycetes share similarities with the findings obtained in previous studies with other soilborne pathogens such as fungi and nematodes. Laboratory and field tests showed that fresh chicken manure was an effective non-chemical soil fumigant that effectively prevented soil-borne pathogens, reduced Fusarium oxysporum and Phytophthora spp. soil inoculum, improved soil condition, and increased strawberry yield (Zhang et al., 2021). Stapleton et al. (1991) observed that composted chicken manure alone at 5,381 kg ha−1 (equivalent to 304 kg ha−1 of total N and 17.6 kg ha−1 of NH4+-N) significantly reduced Pythium ultimum, and when combined with a diurnal heating regime (42°C high 8 h; 18°C low) and an incubation time of 4 weeks, the Pythium population was eradicated. Although increasing NH4+-N concentrations gave better control of P. ultimum and Meloidogyne incognita in incubator fertilizer/solarization simulation laboratory experiments, field results showed that the addition of nitrogen sources to the solarization process did not increased the control efficacy against P. ultimum and V. dahliae (Stapleton et al., 1991). In contrast, the high levels of ammonia detected in soil atmospheres 3 and 7 days after the incorporation of Vicia villosa as green manure were related to the reduction of chlamydospores viability of Thielaviopsis basicola and ammonia was responsible for the observed suppressiveness in field (Candole and Rothrock, 1997).
In our study, the most effective treatment for all the gases sampled at different times was the succession of gases sampled every day (G_ev). This treatment showed a viability reduction rate of 41% compared with the untreated control for an exposure time of 21 days. Similarly, the higher reduction (72% for an exposure time of 34 days) was also obtained for the same treatment in the previous study in southeastern Spain (Larregla et al., 2014). The following more effective biodisinfestation gas treatments were similar in both studies, since they were detected between days 4 and 11 in the present study in northern Spain (reduction of 24–34%) and between days 3 and 9 (reduction of 17-28%) in the previous study in southeastern Spain. This behavior would be in line with studies which have shown that the formation and release of biotoxic volatile compounds are predominantly found during the first 3 weeks of solarization of the soil amended with organic materials. The concentrations of volatile compounds drop to low levels after this time (Gamliel and Stapleton, 2017).
In our experiment, longer exposure times (14 and 21 days) were not more effective than the shorter time (7 days) for all the gases sampled at different times and even the differences among them were no longer observed when exposure time increased. These results were not expected and differ considerably from earlier studies (Ebben et al., 1983; Katan and Gamliel, 2010), which showed that in vitro toxicity of a chemical fumigant to soilborne phytopathogenic fungi was higher when the fumigant concentration x time product was increased. However, it is likely that the explanation for this is that the sampled gas volume in the vacutainer tubes was small (10 mL) and with the increase of exposure time, the escape of gases could have caused a decrease in their concentration and the absence of toxic effect on the pathogen spores. This explanation would be consistent with the result that the biodisinfested soil control (SOIL) was the only treatment that improved disinfectant efficacy when exposure time increased. It also confirms our previous findings where the succession of gases sampled every day (G_ev) was the most effective treatment (Larregla et al., 2014). In order to avoid gas exhaustion and negligible effects on survival by low gas concentrations, the use of methodological devices (Klein et al., 2007) which allow the exposition of fungal spores to larger volumes of gas samples are recommended in future experiments. In that way, the methodological underestimation of the biofumigant effect of gases on actual field conditions could be minimized.
The lowest oospore viability was shown in the biodisinfested soil control treatment (SOIL) for all the assayed exposure times. The overlap of thermal, higher biofumigation effects and microbial activity effects in a synergistic mode of action could well be responsible for these results (Stapleton et al., 1991; Hoitink and Boehm, 1999; Gamliel et al., 2000). Therefore, this could explain that oospore survival in the field biodisinfested soil control treatment (SOIL) would be lower than in the remaining laboratory treatments with only biofumigation effects caused by gases.
Oospores in the moist biodisinfested soil control treatment (SOIL) received a “heat dosage” (13 cumulative hours above 40°C) that was below the threshold (112 cumulative hours at 40°C) required for direct thermal inactivation (Etxeberria et al., 2011b). However, oospores in the biodisinfested soil received a considerable amount of “sub-lethal” heat (115 and 61 cumulative hours above 35 and 37.5°C respectively), that could make them less pathogenic and more susceptible to stress factors such as chemical toxicants or microbial antagonists (Chellemi et al., 1994; Stapleton and DeVay, 1995; Tjamos and Fravel, 1995; Oka, 2010). Although thermal sensitivity of the target pathogen(s) varies widely among species, in general terms, sublethal heat can be defined as a temperature range to weaken target pathogen(s) and, in general, is established for soil temperatures below 38-40°C (Stapleton, 2000; Oka, 2010).
The linear regression of the proportion of viable oospores over exposure time for each level of the biodisinfestation gas factor treatment evidenced significant differences in quantity (intercept) and change rate (slope). Significant differences were found between the succession of biodisinfestation gases sampled every day (G_ev) and the untreated controls (C_va, C_ai). This is in agreement with in vitro biofumigation experiments using different volatility isothiocyanates and the growth-rate response of soilborne fungal pathogens (Sarwar et al., 1998; Kirkegaard, 2009). In our experiment, a significant higher change rate (slope) on survival of oospores was detected in the biodisinfested soil control treatment (SOIL) with regard to the average of gases sampled in days 1–2–3–4–7–9–11 (G_11). This is consistent with Gamliel and Stapleton (1993b), where they obtained an interactive effect of heating soil amended at 38°C with composted chicken manure on the reduction of inoculum densities of fungal pathogens.
A methodology to evaluate a high number of organic amendments for their greater biofumigant efficacy has been obtained in this work. This methodology could be applied for an initial screening of a high number of organic amendments with the final aim of choosing the most suitable amendments for further evaluation with the use of more expensive, time-consuming, and long-lasting field tests that would include the pathosystem to be controlled.
In our study, an effect purely attributable to the released gases through biodisinfestation has been observed in the reduction of long term survival propagules (oospores) of the oomycete plant pathogen P. capsici. Thus, our results suggest the significant biofumigant effect of fresh animal manures in the reduction of the inoculum of a soilborne pathogen in suboptimal conditions for solarization.
In conclusion, the biodisinfestation practice could also be effective in agro-environments with reduced thermal inactivation effects. In a circular bioeconomy context, it appears necessary to continue further the assessment of varied proximity byproducts for their use as organic amendments including a good biofumigant effect and also adapted to the different crops requirements.
Data Availability Statement
The raw data supporting the conclusions of this article will be made available by the authors, without undue reservation.
Author Contributions
SL carried out the conception and design of the research. MG, MO, SM, MMG, AO-B, and SL participated in the other stages of the work, including the performing of experiments, data analyzing, revision of the intellectual content and the drafting of the paper. All authors contributed to the article and approved the submitted version.
Funding
This research was funded by FEDER (project INIA RTA 2015-00060-C04-04 Comprehensive revaluation of by-products based on their potential uses: obtainment of biofumigants and fertilizers and project MICINN RTA PID2019-106148RR-C44 Integration of revalorized agro-food by-products into an enhanced circular economy model: new applications for horticultural and extensive crops in commercial facilities) and by the Deparment of Environment, Territorial Planning, Agriculture and Fisheries of the Basque Government (projects REVABIO and REVAL2). MG was the recipient of a predoctoral contract (INIA-2017-0043) of the State Training Subprogram of the Spanish Ministry of Economy, Industry and Competitiveness (MICINN); MO was the recipient of a PhD contract Introduction of resistance to Tobamovirus and other viruses in landraces of Gernika pepper and Ibarra chili pepper (Order of 24 of October, 2018 of the Minister of Economic Development and Competitiveness of the Basque Government).
Conflict of Interest
The authors declare that the research was conducted in the absence of any commercial or financial relationships that could be construed as a potential conflict of interest.
Acknowledgments
The authors would like to thank Mireia Núñez-Zofío for her helpful support in laboratory tasks execution, Leire Abaunza, Javier Elorrieta, and Berdaitz Juaristi for their help to carry out the greenhouse field trial in NEIKER, and Ashley Dresser for her revision of the English text.
Supplementary Material
The Supplementary Material for this article can be found online at: https://www.frontiersin.org/articles/10.3389/fsufs.2021.663915/full#supplementary-material
References
Arriaga, H., Núñez-Zofío, M., Larregla, S., and Merino, P. (2011). Gaseous emissions from soil biodisinfestation by animal manure on a greenhouse pepper crop. Crop Prot. 30, 412–419. doi: 10.1016/j.cropro.2010.12.012
Beutier, D., and Renon, H. (1978). Representation of NH3-H2S-H2O, NH3-CO2-H2O, and NH3-SO2-H2O vapor-liquid equilibria. Ind. Eng. Chem. Process Des. Dev. 17, 220–230. doi: 10.1021/i260067a002
Bonanomi, G., Antignani, V., Capodilupo, M., and Scala, F. (2010). Identifying the characteristics of organic soil amendments that suppress soil-borne plant diseases. Soil Biol. Biochem. 42, 136–144. doi: 10.1016/j.soilbio.2009.10.012
Bonanomi, G., Lorito, M., Vinale, F, and Woo, S. L. (2018). Organic amendments, beneficial microbes, and soil microbiota: toward a unified framework for disease suppression. Annu. Rev. Phytopathol. 56, 1–20. doi: 10.1146/annurev-phyto-080615-100046
Candole, B. L., and Rothrock, C. S. (1997). Characterization of the suppressiveness of hairy vetch-amended soils to Thielaviopsis basicola. Phytopathology 87, 197–202. doi: 10.1094/PHYTO.1997.87.2.197
CBPA (2011). Código de Buenas Prácticas Agrarias de la Comunidad Autónoma del País Vasco. Decreto 112/2011 de 7 de junio. BOPV (Boletín Oficial del País Vasco) 116, 1-9. Departamento de Medio Ambiente, Planificación Territorial, Agricultura y Pesca. Avaiable online at: https://www.euskadi.eus/y22-bopv/es/bopv2/datos/2011/06/1103188a.pdf (accessed January 24, 2021).
Chellemi, D. O., Olsen, S. M., and Mitchell, D. J. (1994). Effects of soil solarization and fumigation on survival of soilborne pathogens of tomato in northern Florida. Plant Dis. 78, 1167–1172. doi: 10.1094/PD-78-1167
Chellemi, D. O., Olson, S. M., Mitchell, D. J., Secker, I., and McSorley, R. (1997). Adaptation of soil solarization to the integrated management of soilborne pests of tomato under humid conditions. Phytopathology 87, 250–258. doi: 10.1094/PHYTO.1997.87.3.250
Coelho, L., Chellemi, D. O., and Mitchell, D. J. (1999). Efficacy of solarization and cabbage amendment for the control of Phytophthora spp. in North Florida. Plant Dis. 83, 293–299. doi: 10.1094/PDIS.1999.83.3.293
de la Fuente, E., Soria, A. C., Díez-Rojo, M. A., Piedra-Buena, A., García-Álvarez, A., Almendros, G., et al. (2009). Solid-phase micro-extraction (SPME) in the early detection of potentially active volatile compounds from organic wastes used for the management of soilborne pathogens. J. Environ. Sci. Heal. A 44, 1004–1010. doi: 10.1080/10934520902996930
De-Cara-García, M., Fernández-Plaza, M., and Gómez-Vázquez, J. (2018). Pathogenic and biological characterization of Phytophthora capsici isolates from zucchini and pepper in Southeast Spain. Span. J. Agric. Res. 16:e1005. doi: 10.5424/sjar/2018162-13129
Ebben, M. N., Gandy, D. G., and Spencer, D. M. (1983). Toxicity of methyl bromide in soilborne fungi. Plant Pathol. 32, 429–433. doi: 10.1111/j.1365-3059.1983.tb02857.x
Erwin, D. C., and Ribeiro, O. K. (1996). “Morphology and identification of Phytophthora species,” in Phytophthora Diseases Worldwide, eds D.C. Erwin, and O.K. Ribeiro (Saint Paul, MN: American Phytopathological Society Press), 96–144.
Etxeberria, A., Mendarte, S., and Larregla, S. (2011a). Determination of viability of Phytophthora capsici oospores with the tetrazolium bromide staining test versus a plasmolysis method. Rev. Iberoam. Micol. 1, 43–49. doi: 10.1016/j.riam.2010.11.005
Etxeberria, A., Mendarte, S., and Larregla, S. (2011b). Thermal inactivation of Phytophthora capsici oospores. Rev. Iberoam. Micol. 28, 83–90. doi: 10.1016/j.riam.2011.01.004
Gamliel, A., Austerweil, M., and Kritzman, G. (2000). Non-chemical approach to soilborne pest management—organic amendments. Crop Prot. 19, 847–853. doi: 10.1016/S0261-2194(00)00112-5
Gamliel, A., and Stapleton, J. J. (1993a). Effect of soil amendment with chicken compost or ammonium phosphate and solarization on pathogen control, rhizosphere microorganisms, and lettuce growth. Plant Dis. 77, 886–891. doi: 10.1094/PD-77-0886
Gamliel, A., and Stapleton, J. J. (1993b). Characterization of antifungal volatile compounds evolved from solarized soil amended with cabbage residues. Phytopathology 83, 899–905. doi: 10.1094/Phyto-83-899
Gamliel, A., and Stapleton, J. J. (2017). “Combining soil solarization with organic amendments for the control of soilborne pests,” in Soil Solarization: Theory and Practice, eds A. Gamliel, and J. Katan (St. Paul, MN: APS Press), 109–120. doi: 10.1094/9780890544198.015
Gandariasbeitia, M., López-Pérez, J. A., Juaristi, B., Abaunza, L., and Larregla, S. (2021). Biodisinfestation with agricultural by-products developed long-term suppressive soils against Meloidogyne incognita in lettuce crop. Front. Sustain. Food Syst. 5:663248. doi: 10.3389/fsufs.2021.663248
Gandariasbeitia, M., Ojinaga, M., Orbegozo, E., Ortiz-Barredo, A., Núñez-Zofío, M., Mendarte, S., et al. (2019). Phytophthora capsici soil inoculum control through winter biodisinfestation with Brassica green manures application in protected pepper crops of Northern Spain. Span. J. Agric. Res. 17:e1005. doi: 10.5424/sjar/2019171-13808
Guerrero, M. M., Lacasa-Martínez, C. M., Hernández-Piñera, A., Martínez-Alarcón, V., and Lacasa-Plasencia, A. (2013). Evaluation of repeated biodisinfestation using Brassica carinata pellets to control Meloidogyne incognita in protected pepper crops. Span. J. Agric. Res. 11, 485–493. doi: 10.5424/sjar/2013112-3275
Guerrero, M. M., Ros, C., Guirao, P., Martínez, M. A., Martínez, M. C., Barceló, N., et al. (2005). Biofumigation plus solarisation efficacy for soil disinfestation in sweet pepper greenhouses in the Southeast of Spain. Acta Hort. 698, 293–297. doi: 10.17660/ActaHortic.2005.698.39
Guerrero, M. M., Ros, C., Lacasa, C., Martínez, V., Lacasa, A., Fernández, P., et al. (2010). Effect of biosolarization using pellets of Brassica carinata on soilborne pathogens in protected pepper crops. Acta Hort. 883, 337–344. doi: 10.17660/ActaHortic.2010.883.42
Guerrero, M. M., Ros, C., Martínez, M. A., Martínez, M. C., Bello, A., and Lacasa, A. (2006). Biofumigation vs biofumigation plus solarization to control Meloidogyne incognita in sweet pepper. Bulletin OILB/srop 29, 313–318.
Hoitink, H. A. J., and Boehm, M. J. (1999). Biocontrol within the context of soil microbial communities: a substrate-dependent phenomenon. Annu. Rev. Phytopathol. 37, 427–446. doi: 10.1146/annurev.phyto.37.1.427
Jiang, J., and Erwin, D. C. (1990). Morphology, plasmolysis, and tetrazolium bromide stain as criteria for determining viability of Phytophthora oospores. Mycologia 82, 107–113. doi: 10.1080/00275514.1990.12025847
Katan, J. (2005). Soil disinfestation: one minute before methyl bromide phase out. Acta Hortic. 698, 19–26. doi: 10.17660/ActaHortic.2005.698.1
Katan, J., and Gamliel, A. (2010). “Soil solarization-−30 years on: what lessons have been learned?,” in Recent Developments in Management of Plant Diseases, eds U. Gisi, I. Chet, and M. L. Gullino (Dordrecht: Springer), 265–284. doi: 10.1007/978-1-4020-8804-9_19
Katan, J., Greenberger, A., Alon, H., and Grinstein, A. (1976). Solar heating by polyethylene mulching for the control of diseases caused by soilborne pathogens. Phytopathology 66, 683–689. doi: 10.1094/Phyto-66-683
Kirkegaard, J. A. (2009). “Biofumigation for plant disease control – from the fundamentals to the farming system,” in Disease Control in Crops: Biological and Environmentally Friendly Approaches, eds D. Walters (Hoboken, NJ: Wiley-Blackwell), 172–195. doi: 10.1002/9781444312157.ch9
Kirkegaard, J. A., Gardner, P. A., Desmarchelier, J. M., and Angus, J. F. (1993). “Biofumigation: using Brassica species to control pests and diseases in horticulture and agriculture,” in Proc. 9th Aust. Res. Assem. Brassicas, eds N. Wratten, and R. Mailer (Wagga Wagga, NSW: NSW Agric), 77–82.
Klein, E., Katan, J., Austerweil, M., and Gamliel, A. (2007). Controlled laboratory system to study soil solarization and organic amendment effects on plant pathogens. Phytopathology 97, 1476–1483. doi: 10.1094/PHYTO-97-11-1476
Lacasa, C. M., Martínez, V., Hernández, A., Ros, C., Lacasa, A., Guerrero, M. M., et al. (2015). Survival reduction of Phytophthora capsici oospores and P. nicotianae chlamydospores with Brassica green manures combined with solarization. Sci. Hort. 197, 607–618. doi: 10.1016/j.scienta.2015.10.024
Larregla, S., Guerrero, M. M., Mendarte, S., and Lacasa, A. (2015). “Biodisinfestation with oorganic amendments for soil fatigue and soil-borne pathogens control in protected pepper crops,” in Organic Amendments and Soil Suppressiveness in Plant Disease Management, eds M. K. Meghvansi, and A. Varma (Switzerland: Springer), 437–456. doi: 10.1007/978-3-319-23075-7_21
Larregla, S., Núñez-Zofío, M., Fernández-Molina, P., Martínez-Alarcón, V., Lacasa-Martinez, C. M., and Guerrero-Díaz, M. M. (2014). Reduction of Phytophthora capsici oospores viability by gases released during soil biosolarization of protected pepper crops in southeastern Spain. Acta Hort. 1044, 113–118. doi: 10.17660/ActaHortic.2014.1044.12
Lumsden, R. D. (1980). A nylon fabric technique for studying the ecology of Phytium aphanidermatum and other fungi in soil. Phytopathology 71, 282–285. doi: 10.1094/Phyto-71-282
Núñez-Zofío, M., Garbisu, C., and Larregla, S. (2010). Application of organic amendments followed by plastic mulching for the control of Phytophthora root rot of pepper in northern Spain. Acta Hort. 883, 353–360. doi: 10.17660/ActaHortic.2010.883.44
Núñez-Zofío, M., Larregla, S., and Garbisu, C. (2011). Application of organic amendments followed by soil plastic mulching reduces the incidence of Phytophthora capsici in pepper crops under temperate climate. Crop Prot. 30, 1563–1572. doi: 10.1016/j.cropro.2011.08.020
Núñez-Zofío, M., Larregla, S., and Garbisu, C. (2012). Repeated biodisinfection controls the incidence of Phytophthora root and crown rot of pepper while improving soil quality. Span J. Agric. Res. 3, 794–805. doi: 10.5424/sjar/2012103-571-11
Núñez-Zofío, M., Larregla, S., Garbisu, C., Guerrero, M. M., Lacasa, C. M., and Lacasa, A. (2013). Application of sugar beet vinasse followed by solarization reduces the incidence of Meloidogyne incognita in pepper crops while improving soil quality. Phytoparasitica 41, 181–191. doi: 10.1007/s12600-012-0277-6
Ojinaga, M., Gandariasbeitia, M., Orbegozo, E., Ortíz, A., Guerrero, M. M., Lacasa, C. M., et al. (2020). Biodisinfestation for Meloidogyne and Verticillium control in commercial protected crops in the Basque Country Atlantic area (northern Spain). Acta Hort. 1270, 327–336. doi: 10.17660/ActaHortic.2020.1270.40
Oka, Y. (2010). Mechanisms of nematode suppression by organic soil amendments—a review. Appl. Soil Ecol. 44, 101–115. doi: 10.1016/j.apsoil.2009.11.003
Oldfield, T. L., Achmon, Y., Perano, K. M., Dahlquist-Willard, R. M., VanderGheynst, J. S., et al. (2017). A life cycle assessment of biosolarization as a valorization pathway for tomato pomace utilization in California. J. Clean. Prod. 141, 146–156. doi: 10.1016/j.jclepro.2016.09.051
Pittis, J. E., and Shattock, R. C. (1994). Viability, germination and infection potential of oospores of Phytophthora infestans. Plant Pathol. 43, 237–426. doi: 10.1111/j.1365-3059.1994.tb02700.x
Riga, P., Goikoetxea, X., and Larregla, S. (2000). In vitro toxicity of HNO2 and NH3 in Phytophthora capsici mycelium growth. Acta Hort. 532, 225–228. doi: 10.17660/ActaHortic.2000.532.30
Ros, M., García, C., Hernández, M. T., Lacasa, A., Fernández, P., and Pascual, J. A. (2008). Effects of biosolarization as methyl bromide alternative for Meloidogyne incognita control on quality of soil under pepper. Biol. Fertil. Soils 45, 37–44. doi: 10.1007/s00374-008-0307-1
Rosskopf, E., Di Gioia, F., Hong, J. C., Pisani, C., and Kokalis-Burelle, N. (2020). Organic amendments for pathogen and nematode control. Annu. Rev. Phytopathol. 58, 277–311. doi: 10.1146/annurev-phyto-080516-035608
Sarwar, M., Kirkegaard, J. A., Wong, P. T. W., and Desmarchelier, J. M. (1998). Biofumigation potential of Brassicas. III. In vitro toxicity of isothiocyantes to soil-borne fungal pathogens. Plant. Soil 201, 103–112. doi: 10.1023/A:1004381129991
Schabenberger, O., and Pierce, F. J. (2002). “Linear mixed models for clustered data,” in Contemporary Statistical Models for the Plant and Soil Sciences, eds O. Schabenberger, and F. J. Pierce (Boca Raton, FL: CRC Press LLC), 403–524. doi: 10.1201/9781420040197
Stapleton, J. J. (2000). Soil solarization in various agricultural production systems. Crop Prot. 19, 837–841. doi: 10.1016/S0261-2194(00)00111-3
Stapleton, J. J., and Bañuelos, G. S. (2009). Biomass crops can be used for biological disinfestation and remediation of soils and water. Calif. Agric. 63, 41–46. doi: 10.3733/ca.v063n01p41
Stapleton, J. J., and DeVay, J. E. (1995). “Soil solarization: a natural mechanism of integrated pest management,” in Novel Approaches to Integrated Pest Management, ed R. Reuveni (Boca Raton, FL: Lewis Publishers), 309–322.
Stapleton, J. J., DeVay, J. E., and Lear, B. (1991). “Simulated and field effects of ammonia-based fertilizers and soil solarization on pathogens survival, soil fertility, and crop growth,” in Proc. of the First Int. Conference on Soil Solarization - 19-25 February 1990 - Amman (Jordan), Plant Production and Protection Paper 109, eds J. E. DeVay, J. J. Stapleton, and C. L. Elmore (Rome: FAO), 331–342.
Stroup, W. W. (2018). “Analysis of non-Gaussian data,” in Applied Statistics in Agricultural, Biological, and Environmental Sciences, ed B. Glaz, and K. M. Yeater (Madison, WI: ASA, CSSA, and SSSA Books), 449–509. doi: 10.2134/appliedstatistics.2015.0081.c16
Tenuta, M., and Lazarovits, G. (2002). Ammonia and nitrous acid from nitrogenous amendments kill the microsclerotia of Verticillium dahliae. Phytopathology 92, 255–264. doi: 10.1094/PHYTO.2002.92.3.255
Tjamos, E. C., and Fravel, D. R. (1995). Detrimental effects of sublethal heating and Talaromyces flavus on microsclerotia of Verticillium dahliae. Phytopathology 85, 388–392. doi: 10.1094/Phyto-85-388
Tsao, P. H., and Oster, J. J. (1981). Relation of ammonia and nitrous acid to suppression of Phytophthora in soils amended with nitrogenous organic substances. Phytopathology 71, 53–59. doi: 10.1094/Phyto-71-53
Keywords: capsicum annuum, pepper, Phytophthora capsici, oospores, biodisinfestation, biosolarization, biofumigation, animal manure
Citation: Larregla S, Gandariasbeitia M, Ojinaga M, Mendarte S, Guerrero MdM and Ortiz-Barredo A (2021) Gases Released During Soil Biodisinfestation of Pepper Greenhouses Reduce Survival of Phytophthora capsici Oospores in Northern Spain. Front. Sustain. Food Syst. 5:663915. doi: 10.3389/fsufs.2021.663915
Received: 04 February 2021; Accepted: 22 June 2021;
Published: 19 July 2021.
Edited by:
Engracia Madejon, Institute of Natural Resources and Agrobiology of Seville (CSIC), SpainReviewed by:
Margarita Ros, Spanish National Research Council, SpainSylvia Patricia Fernández-Pavía, Michoacana University of San Nicolás de Hidalgo, Mexico
Copyright © 2021 Larregla, Gandariasbeitia, Ojinaga, Mendarte, Guerrero and Ortiz-Barredo. This is an open-access article distributed under the terms of the Creative Commons Attribution License (CC BY). The use, distribution or reproduction in other forums is permitted, provided the original author(s) and the copyright owner(s) are credited and that the original publication in this journal is cited, in accordance with accepted academic practice. No use, distribution or reproduction is permitted which does not comply with these terms.
*Correspondence: Santiago Larregla, c2xhcnJlZ2xhQG5laWtlci5ldXM=