- 1Department of Animal and Rangeland Sciences, Oregon State University, Corvallis, OR, United States
- 2Department of Biological and Ecological Engineering, Oregon State University, Corvallis, OR, United States
Agrivoltaic systems are designed to mutually benefit solar energy and agricultural production in the same location for dual-use of land. This study was conducted to compare lamb growth and pasture production from solar pastures in agrivoltaic systems and traditional open pastures over 2 years in Oregon. Weaned Polypay lambs grew at 120 and 119 g head−1 d−1 in solar and open pastures, respectively in spring 2019 (P = 0.90). The liveweight production between solar (1.5 kg ha−1 d−1) and open pastures (1.3 kg ha−1 d−1) were comparable (P = 0.67). Similarly, lamb liveweight gains and liveweight productions were comparable in both solar (89 g head−1 d−1; 4.6 kg ha−1 d−1) and open (92 g head−1 d−1; 5.0 kg ha−1 d−1) pastures (all P > 0.05) in 2020. The daily water consumption of the lambs in spring 2019 were similar during early spring, but lambs in open pastures consumed 0.72 L head−1 d−1 more water than those grazed under solar panels in the late spring period (P < 0.01). No difference was observed in water intake of the lambs in spring 2020 (P = 0.42). Over the entire period, solar pastures produced 38% lower herbage than open pastures due to low pasture density in fully shaded areas under solar panels. The results from our grazing study indicated that lower herbage mass available in solar pastures was offset by higher forage quality, resulting in similar spring lamb production to open pastures. Our findings also suggest that the land productivity could be greatly increased through combining sheep grazing and solar energy production on the same land in agrivoltaics systems.
Introduction
Global food and energy demands are continuously increasing due to growing populations and economic growth. Development of efficient and integrated production systems is crucial to meet these demands in a sustainable manner. Solar energy production using photovoltaic panels causes substantially less carbon emissions than natural gas (DeMartis, 2018). Grasslands and croplands located in temperate agro-ecologies are ranked to be the best places to install solar panels for maximum energy production (Adeh et al., 2019). However, energy production in photovoltaic systems requires large areas of land, potentially causing a competition between agricultural uses (Marrou et al., 2013). In an attempt to overcome this competition, energy and agricultural production could be combined through pairing of photovoltaics with open field crop production (Goetzberger and Zastrow, 1982; Amaducci et al., 2018).
Agrivoltaics not only decreases the conflict between agricultural and energy sectors, but it efficiently and effectively promotes sustainable land use. These systems have the potential to increase global land productivity by an impressive 35–73% (Dupraz et al., 2011). Furthermore, agrivoltaics help to improve the productivity of farms, as there is a 30% increase in economic value for those that combine shade-tolerant crop production and solar generated electricity as compared to more traditional practices (Dinesh and Pearce, 2016). This added income from the solar panels is especially beneficial, as the panels themselves can partially displace grain, vegetable, and fruit crops, which has the potential to decrease yields by 5–20% in locations such as Germany (Apostoleris and Chiesa, 2019). However, not all crops face a decreased yield with the introduction of the photovoltaics. For example, a study conducted in Massachusetts reported that crop growth and yield improved with the use of solar panels. Crops that tend to do well in these conditions are those that benefit from the added protection from sunlight or excessive evaporation (Apostoleris and Chiesa, 2019). In addition to improving some crop yields and increasing economic and land productivity, agrivoltaics may also benefit the solar energy industry further by supporting bringing more ground mounted photovoltaic systems back into the mainstream market, thereby increasing the economy further. Furthermore, agrivoltaics provide ecosystem service benefits such as providing nectar and pollen sources for bees and pollinators (Graham and Higgins, 2019; Hernandez et al., 2019).
While a growing body of information has been generated on the crop production in agrivoltaic systems, there is a paucity of information on the impact of solar panels on pasture and animal production. To the best of our knowledge, there is no study conducted to investigate the livestock production in agrivoltaics systems. The precursor for the livestock production in agrivoltaics is silvopastoral and agroforestry production systems, which involve planting trees in or around fields used for agricultural and animal production purposes (Dinesh and Pearce, 2016). Traditional silvopastoral studies report multiple benefits of livestock grazing under tree shade such as extended grazing period due to lower evapotranspiration, higher nutritive value of the forages and lower environmental pollution (Dixon, 1995; Lima et al., 2019). Although shade reduces the light interception potential of the crops, a higher soil moisture achieved by the installation of photovoltaics can be a more water efficient farming, leading to a significant increase in late season biomass of forages. Solar panels in agrivoltaic systems can provide cool microclimate for grazing livestock, promoting animal welfare by providing shelter from sun, wind, and predators. Livestock in particular sheep can also be used in targeted grazing systems to control the excess understory biomass production to reduce the cost of labor and herbicide applications (DeMartis, 2018). The objective of this study was to compare pasture production, lamb growth, grazing behavior and nutritive value of forages in traditional open and solar pastures in agrivoltaics.
Materials and Methods
Site
The grazing experiment was carried out at the Oregon State University in Corvallis, OR (44° 34′ N, 123° 18′ W 78 m a.s.l.) in spring 2019 and 2020. All procedures were approved by the Institutional Animal Care and Use Committee (ACUP# 5146) prior to the commencement of the experiment. Experimental plots were located within the Rabbit Hills Solar Farm, a 2.4 ha, 1.4 MW agrivoltaic farm which combines sheep grazing with solar energy production. The 1.65 m wide photovoltaic panels are oriented east-west, and tilted south at an 18° angle. The lowest edge of the panel is 1.1 m above the ground, and rows of panels are 6 m apart. The soil type is a combination of Woodburn silt loam, Amity Silt loam and Bashaw silty clay (USDA Soil Survey Staff, 2020). The soil test result is presented in Table 1.
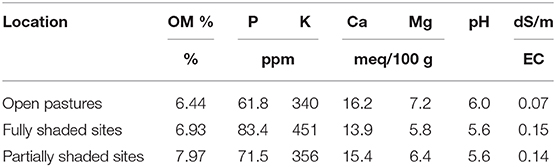
Table 1. Soil test results from open, fully and partially shaded pasture sites (75 mm depth) in winter 2019.
Experimental Design and Grazing Management
The experiment layout was a randomized complete block design with three replicates (blocks). A 0.6 ha pasture paddock under solar panels and adjacent open areas was fenced and divided into three, 0.2-ha blocks to serve as replicates. Each block was divided into two subplots (0.1 ha), which were randomly assigned to the grazing under solar panels and grazing in open pasture fields (control). In solar pastures, the distance between solar panels was 6 m giving a 3-m fully shaded and 3-m partially shaded areas (Adeh et al., 2019). Each solar pasture contained four solar arrays and four solar panels. Thus, these pastures had 50% open (partially shaded) and 50% fully shaded areas. In February 2019, a pasture seed mixture containing festulolium cv. Perun (X Festulolium braunii), white clover cv. Seminole (Trifolium repens L.), chicory cv. Antler (Cichorium intybus L.), plantain cv. Boston (Plantago lanceolata L.), was overdrilled into existing pastures using a lawn overseeder (The classen TS-20, Southampton, PA, USA) to improve pasture production and forage quality. The seed mixture was also broadcasted in the areas that were difficult to access with overseeder under solar panels (5–10% of the area under solar panels). All plots were fertilized with 50 kg N/ha as urea in February 2019 and 2020.
Weaned Polypay lambs (2.5 months old) were stratified by sex and liveweight (mean LW = 24.6 ± 4.1 kg in 2019 and 26.6 ± 3.0 kg in 2020) and allocated randomly to treatments in both years. Each treatment had a core group of 9 lambs (testers) with spare lambs (regulators) in spring. A put-and-take grazing system was used to match feed demand with changing supply (Bransby, 1989). The groups of lambs were randomly assigned to one of six, 0.1 ha pastures where they continuously grazed from 17 April to 12 June 2019 at the stocking rate of 60 weaned lambs ha−1 in the first period (17 April−15 May) and 30 and 36.6 weaned lambs ha−1 stocking rate in open and solar panel pastures, respectively in late spring period (15 May−12 June). In 2020, grazing commenced on 30 March and extended through 11 June. The average stocking rate was 50 and 30 weaned lambs ha−1 in the first (30 March−4 May) and second periods (4 May-11 June) in both solar and open pastures. Animals had free access to mineral supplement and fresh water in portable water troughs connected to a permanent water supply in open pastures and under solar fields.
Measurements
Herbage dry matter (DM) production (kg DM ha−1) was measured inside 1-m2 grazing exclosure cages (n = 4) during active growth in spring, summer, and autumn under fully shaded, partially shaded and open areas. Herbage growth was measured from a rectangular quadrat (0.25 m2), harvested using electric hand clippers to a stubble height of ~3 cm. After collecting the forage cuts, cages were placed over a new area where the pasture was pre-mown to 3 cm of stubble height at the start of each new growth period. Forage cuts were sub-sampled for sorting into botanical fractions (grass, forbs, weed, and dead material) before drying. All herbage from the quadrat cuts were oven dried at 65°C for 48 h. Herbage growth rates were calculated at each harvest by dividing total DM production by the number of elapsed days since the previous harvest.
Liveweight gain (g head−1 d−1) was determined by weighing individual tester animals prior to and following each grazing period. Lambs were held overnight without food and water and weighed “empty” the following morning. Liveweight gain per head of tester lambs was calculated from the change in weight between each liveweight measurement date. Liveweight gain (kg ha−1 d−1) was calculated by multiplying liveweight gain per head of tester lambs by the number of testers plus regulator lambs per hectare.
Lamb foraging behavior was scored by visual scanning of each lamb at 5-min intervals from 8 a.m. to 4 p.m. in early and late spring periods (Orr et al., 2005). Lambs' activity in open pastures and under solar panels was scored for grazing, ruminating and idling parameters by three observers. The location of lambs was recorded as fully shaded or in solar alleys. Grazing was defined as when a lamb is actively eating with their heads down. Lambs were recorded as idling if they had no specific jaw movements either standing or laying down. Grazing, ruminating and idling time were converted from the observation scores and multiplied by 5, assuming the same behavior over the previous 5 min. Group water intake of the lambs were also determined in early and late spring periods in both 2019 and 2020. Water troughs were filled with 60 L water on a daily basis and consumption was calculated based on the amount of water disappeared from the troughs within a 24 h period. A second trough in grazing excluded areas in both open and solar pastures were placed to take into account of evaporation and rainfall. The measurement was repeated three subsequent days during each period.
Herbage mass (kg DM ha−1) was measured in each plot using a rising plate meter (PM; Jenquip, Feilding, New Zealand) by collecting 50 measurements on a weekly basis. Rising plate meter measurements were calibrated by regression against the herbage masses that were obtained from 12, 0.25-m2 quadrats. Calibrations of herbage pasture masses were performed in April 2018 and March 2019. Calibration curves for each treatment were generated by fitting a single line through all the data. Random pluck samples were collected from pre-grazing allocations of each pasture to determine chemical and botanical compositions of forage on offer. A total of 50–75 pluck samples, representative of herbage offered to lambs, were collected by hand randomly across pasture in each plot at weekly intervals. Subsamples were sorted into botanical components then dried at 65°C for 48 h. Percentage botanical composition of samples on a dry weight basis was then calculated. A well-mixed bulk sample was ground in a Wiley mill with a 1 mm screen (Thomas/Wiley, Swedesboro, NJ) and shipped to a commercial laboratory for NIRS analyses of the samples (Dairy One Forage Laboratory, Ithaca, NY) (https://dairyone.com/download/forage-forage-lab-analyticalprocedures).
Land Equivalent Ratio (LER) and Net Return of Spring Grazing
Land use efficiency for total annual herbage DM yield (kg DM ha−1 y−1) and lamb liveweight production (kg ha−1 d−1) in agrivoltaics was assessed using land equivalent ration (LER) formula. LER is specified as the sum of the respective yield ratios of dual land use (agrivoltaics) to single land use (traditional) as described by Trommsdorff et al. (2021):
Where a and b are the biological yield (forage or animal) and electricity production, respectively. When LER > 1 the dual use (agrivoltaics) system is more efficient and advantageous than traditional single use power or pasture-based livestock production systems, whereas LER < 1 indicates a lower land use efficiency in dual use system as compared to single land use system. In our calculation, solar power production was assumed to be the same (yield ratio = 1) in both dual (agrivoltaics) and single use (photovoltaics) systems as the design and establishment of solar panels were arranged to maximize the energy production.
To calculate the net returns from the onsite grazing activity, we averaged the daily liveweight production (kg ha−1 d−1) in 2019 and 2020, multiplied by the average number of grazing days across both years, and assumed an average price of $316.19 per hundred kg weight based on the USDA average price of lambs in 2018 (USDA Agricultural Marketing Service, 2018).
Statistical Analysis
Liveweight gain per head (g head−1 d−1) and per ha (kg ha−1 d−1) were analyzed by one-way ANOVA with repeated measures for each liveweight gain measurement period. Pasture production and water consumption of lambs (L/d) was analyzed by one-way ANOVA with three replicates at each date. Separation of treatment means declared significant by ANOVA were compared by Fisher's method of protected least significant difference (LSD) at a P = 0.05. The computations were conducted using GENSTAT statistical software (Payne, 2009).
Results
Climatic Conditions
The site has a long-term mean (LTM) annual precipitation of 1086 mm. The annual precipitation was 704 mm in 2019 which was substantially lower than the LTM (Figure 1A). The precipitation in 2020 was greater in January, May and June than the LTM. Mean air (Figure 1B) and soil (Figure 1D) temperatures followed a similar trend in open and partially shaded areas throughout the experiment period. While mean air temperature were lower in fully shaded areas than open and partially shaded areas during spring and summer months, soil temperature appeared to be greater in spring but lower in summer in fully shaded areas than other areas. Overall, soil moisture (VWC) content was similar in both open and partially shaded areas but greater in spring and summer months in fully shaded areas (Figure 1C).
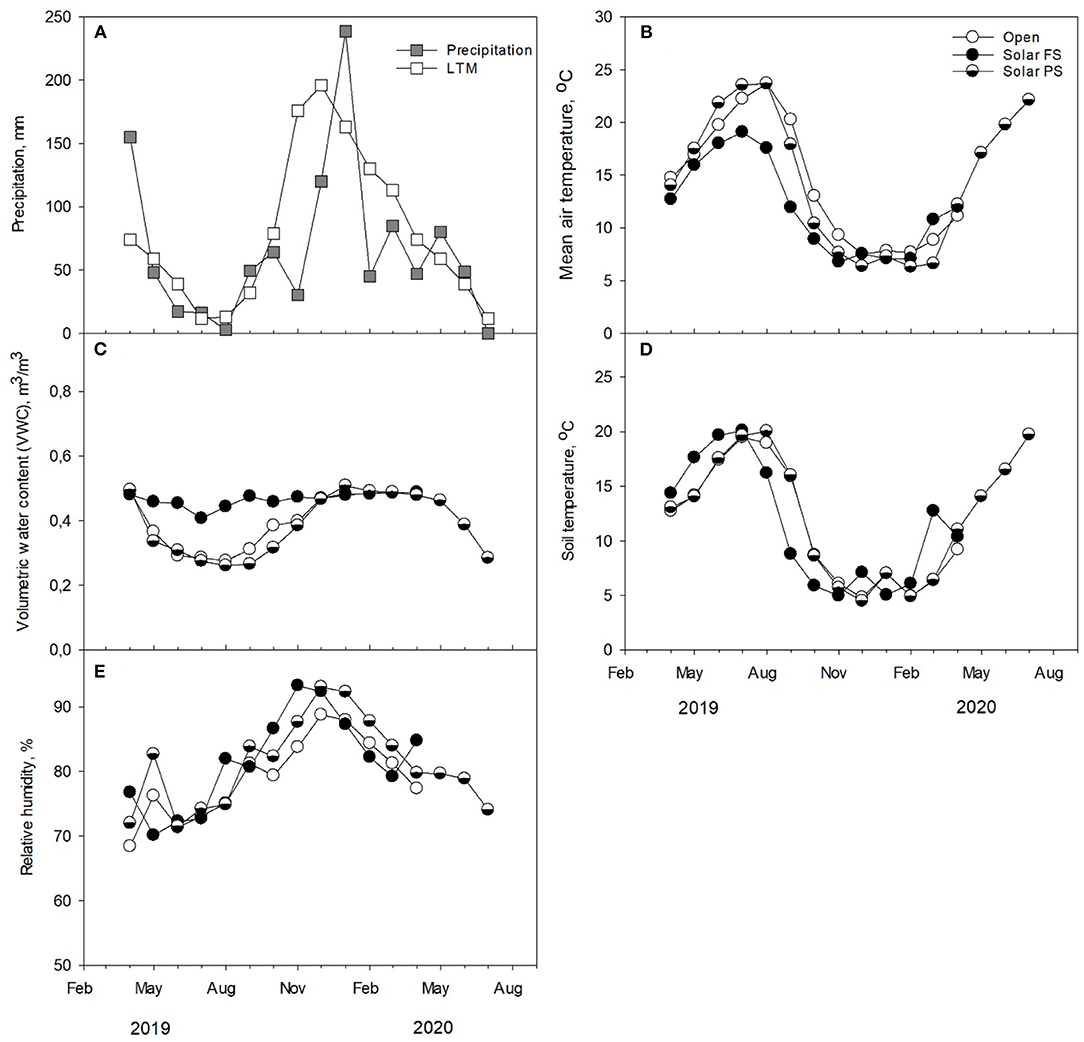
Figure 1. Mean monthly precipitation (A) and microclimatic conditions (B–E) in fully (FS) and partially shaded (PS) areas under solar panels and open pastures in 2019 and 2020.
Pasture Dry Matter (DM) Production
In 2019, total herbage yield in spring-summer period was 3,609, 2,893, and 3,700 kg DM ha−1 for open pastures, partially shaded, and fully shaded solar pastures, respectively (Figure 2). While the DM yield from open and partially shaded areas was similar, pastures under fully shaded sites were substantially lower (P < 0.01). Seasonal herbage DM production between open pastures and partially shaded areas did not differ in 2019 (P > 0.05). However, the forage production in the fully shaded areas under solar panels was lower (P < 0.05) than open pastures on 23 May and 23 October while it was greater (P < 0.05) than open pastures on 11 July.
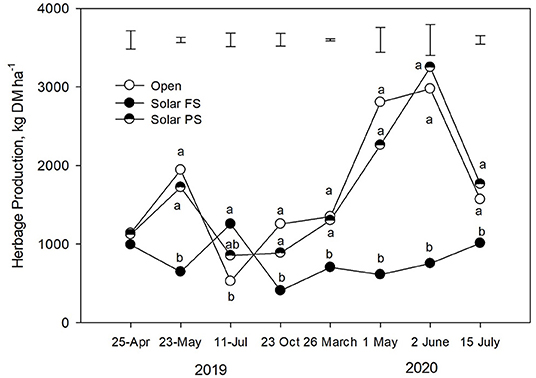
Figure 2. Seasonal herbage dry matter (DM) production (kg DM ha−1) in fully (FS) and partially shaded (PS) areas under solar panels and open pastures in 2019 and 2020. a−bLowercase letters indicate statistical differences for total herbage production according to Fisher's unprotected least significant difference (α = 0.05). Bars represent SEM.
Earlier closing of the pastures in fall 2019 resulted in greater spring-summer 2020 production for both open and partially shaded pastures, while pasture production in fully shaded areas remained similar. In spring-summer 2020 total herbage yield in spring-summer period was 8,700, 3,079, and 8,579 kg DM ha−1 for open pastures, partially shaded and fully shaded solar pastures, respectively (P < 0.01). On average, the pasture production was 9–33% less in agrivoltaics systems than open pastures (P < 0.01).
Herbage Mass on Offer
The open pastures had a higher mean herbage mass than solar pastures in both years (Figures 3A,B). In spring 2019, the herbage mass in open pastures remained relatively stable throughout the grazing period, ranging from 1,268 kg DM ha−1 to 1,487 kg DM ha−1. The herbage mass in solar pastures decreased from 1,268 kg DM ha in week 2 to 1,025 kg DM ha in week 3 and remained under 1,200 kg DM ha−1 until the end of the grazing. In spring 2020, herbage mass in open pastures fluctuated from 998 kg DM ha−1 to 1178 kg DM ha−1 until the end of April before it started to increase reaching to 1,510 kg DM ha in mid-May. The herbage mass in solar pastures were mostly stable fluctuating 999 kg DM ha−1 to 1,162 kg DM ha−1. However, the difference in weekly herbage mass was only significant (P < 0.05) on 4 June and 11 July in 2019 and 17 April and 21 May in 2020.
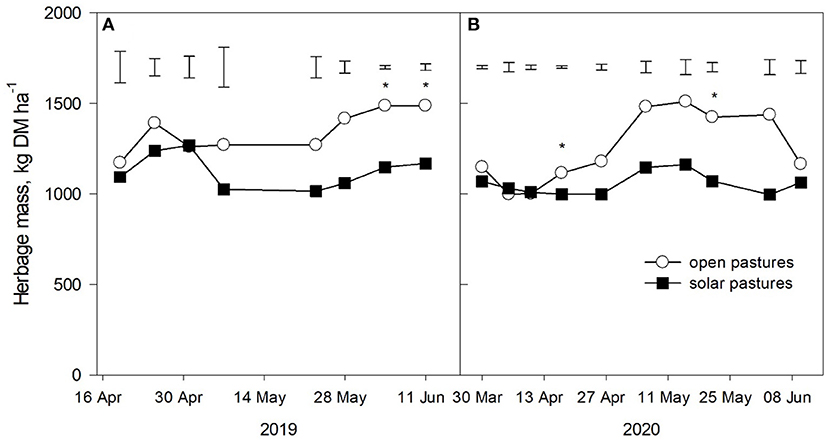
Figure 3. Weekly herbage mass (kg DM ha−1) in spring 2019 (A) and 2020 (B) in open and solar pastures. Bars represent SEM. *indicates above the period when the difference was significant according to Fisher's unprotected least significant difference (α = 0.05).
Botanical Composition and Nutritive Value of Herbage on Offer
In spring 2019, averaged across the grazing periods, the grass components of pastures were 83.3% and 82.8% in open and solar pastures, respectively (P = 0.83; Figure 4A). Forb components of open pastures (3.8%) were greater (P < 0.05) than solar pastures, which had only 1.7% legume content. A treatment × period interaction (P < 0.05) occurred for weed content. In period 1, both pastures had similar weed contents, while the solar pasture had greater broadleaved weed contents than open pasture by 6.2%. In spring 2020, grass content of pastures were similar (P = 0.06) in both open and solar pastures but the grass component increased from 74% in period 1 to 79.8% in period 2 (P < 0.05; Figure 4B). Averaged across the periods, the forb content of open pastures was 17.2% and this was greater (P < 0.01) than solar pastures that had only 10.2% forb content. Both pastures tended to have lower (P = 0.06) forb content in Period 2 than Period 1. Both pastures had comparable broadleaved weed (P = 0.26) and dead material contents (P = 0.67).
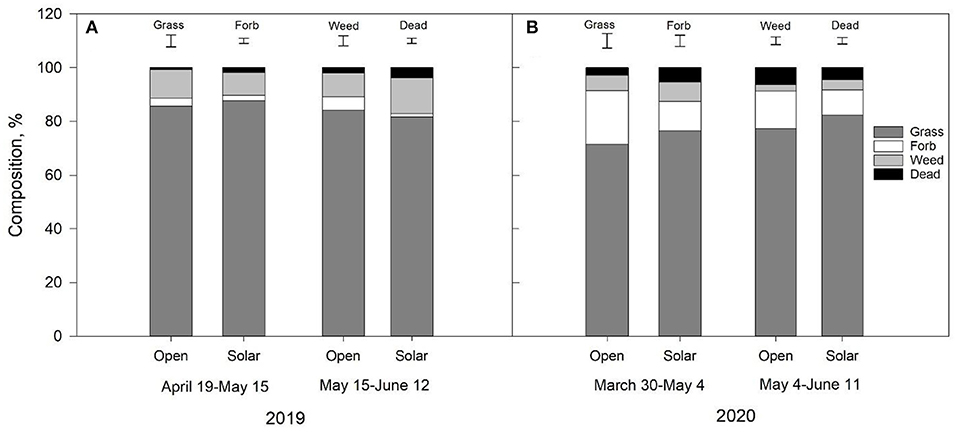
Figure 4. Botanical composition of pasture on offer (%) in open and solar pastures in 2019 (A) and 2020 (B). Bars represent SEM.
In spring 2019, average CP content of pastures reduced sharply (P < 0.01) from 21.6% on April 19 to 15.8% on May 1 and remained relatively stable until the end of grazing season (Figure 5A). Overall, solar pastures had greater (P < 0.05) CP content than open pastures. Similarly, ME and WSC contents of pastures declined (P < 0.01) as the season progressed (Figures 5C,D). While the difference between pastures for their NDF contents were not significant (P = 0.16), open pastures had consistently greater (P < 0.05) WSC except on June 11 (Figures 5B,D). The NDF content of pastures increased (P < 0.01) from 47.5% on April 19 to 57.1% on June 11 but both pastures had comparable (P = 0.11) NDF contents. Similar seasonal trends were observed in the change of chemical composition of pasture in spring 2020 (Figures 5E,H). However, for almost all chemical composition parameters, the nutritive value of pastures were greater in spring 2020 than spring 2019. Similar to spring 2019, solar pastures had greater CP (P < 0.01) and lower NDF and WSC contents (P < 0.05) than open pastures. In addition, in spring 2020, ME content of solar pastures were also greater (P < 0.01) than open pastures (Figure 5G).
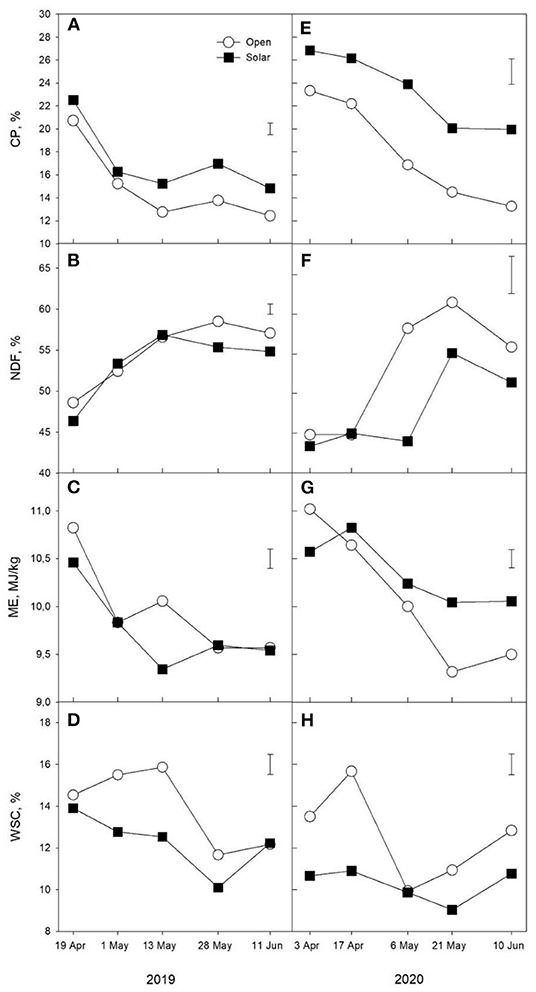
Figure 5. Nutritive value of pasture on offer (%) in open and solar pastures in 2019 (A–D) and 2020 (E–H). Bars represent SEM.
Lamb Production
Averaged across the grazing periods, weaned lambs grew at 120 and 119 g head−1 d−1 under solar panels and open pastures, respectively in spring 2019 (P = 0.90; Figure 6A). Although a higher stocking density (36.6 lambs/ha) at the pastures under solar panels was maintained than open pastures (30 lambs/ha) in the late spring period, the liveweight production between grazing under solar panels (1.5 kg ha−1 d−1) and open pastures (1.3 kg ha−1 d−1) were comparable (P = 0.67; Figure 6C). Similarly, in spring 2020, lambs in both solar and open pastures had similar liveweight gains (P = 0.64; Figure 6B). In period 1, lambs grew at 130 g head−1 d−1 as the season progressed the average daily liveweight gains of the lambs dropped to 51 g head−1 d−1 (P < 0.01). Liveweight production of the lambs were similar as both open and solar pastures were grazed at same stocking rates in both periods (P = 0.97; Figure 6D).
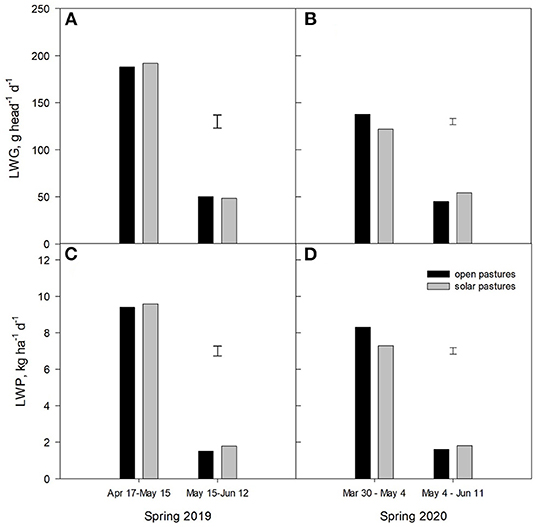
Figure 6. Liveweight gains [LWG, g head−1 d−1; (A,B)] and liveweight production [LWP, kg ha−1 d−1; (C,D)] of lambs grazing under solar panels and open pastures in spring 2019 (A,C) and 2020 (B,D). Bars represent SEM.
Foraging Behavior and Water Intake
Lambs grazing both pasture types had similar foraging behaviors in both April and May 2020 (Figures 7A,B). Overall, total grazing, ruminating, idling and drinking times did not differ depending on the pasture type (All P > 0.05). In April, lambs spent more time (P < 0.01) grazing pastures in the morning and afternoon than the noontime. However, the time that lambs spent ruminating did not change (P = 0.22) depending on the time of the day. Lambs expressed more idling behavior in the noontime (P < 0.05) followed by afternoon while they spent the least time idling in the morning. The time spent drinking did not differ depending on the pasture type (P = 0.52) and time of the day (P = 0.13). In May, there was a tendency for interaction (P = 0.054) for the pasture type and grazing time of the day (Figure 7B). While the grazing times in morning were similar in both pasture types, lambs in solar pastures appeared to graze more during noon but much less in afternoon compared to those grazing open pastures. No differences were observed in ruminating (All P > 0.05) and drinking time regarding the time of the day or pasture types but lambs spent more time (P < 0.05) idling during noon than morning and afternoon.
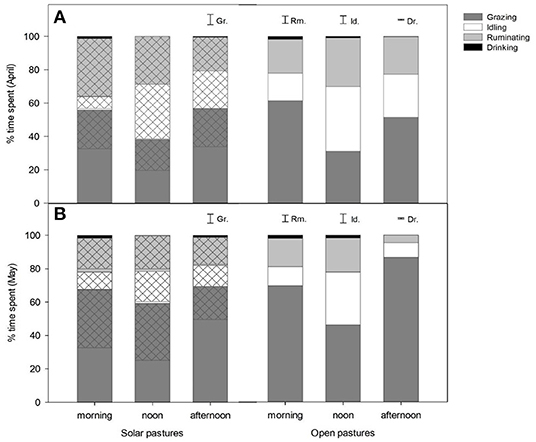
Figure 7. Lamb foraging behavior in open and solar pastures in April (A) and May 2020 (B). Crosshatch represents the time spent under full shade. Bars represent SEM. Gr = grazing, Rm = ruminating, Id = idling, Dr = drinking.
Lambs grazing solar pastures spent 96.1 and 96.5% of their idling time in shade directly under solar panels in April and May, respectively (P = 0.95) (Figures 7A,B). They also did their ruminating activities predominantly under the shade of the panels with an average 99.8% and 92.7% of their time in April and May, respectively. No period or time of the day effects were observed for the ruminating or idling time that was spent under shade (All P > 0.05). However, there was a tendency for period time of the day interaction for grazing time spent under shade (P = 0.09). On average, lambs only spent 43.5% of their grazing activities under solar panels, with no obvious time of the day difference in April. The grazing time that was spent under solar panels was similar in May (46.5%). However, there was a difference in time of the day that they spent grazing activities under solar panels. While, they also undertook over 50% of their grazing activity in shade as well in the morning and noon the lambs spent only 29% of their grazing time under solar panels in the afternoon.
In 2019, the daily water consumption of the lambs was similar during early spring, but lambs in open pastures consumed 0.72 L head−1 d−1 more water than those grazed under solar panels in the late spring period (P < 0.01; Figure 8A). In spring 2020, the daily water intake of the lambs was 1.48 and 1.32 L head−1 d−1 for the lambs in open and solar pastures, respectively but the difference was not significant at neither early nor late spring periods (P = 0.42; Figure 8B). The water intake of the lambs increased from 0.59 L head−1 d−1 in early spring to 2.21 L head−1 d−1 in the late spring period (P < 0.01).
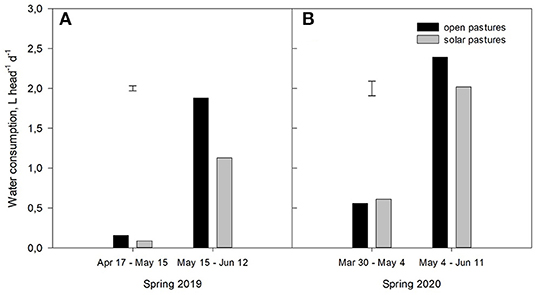
Figure 8. Daily water consumption (L head−1 d−1) of lambs grazing in solar and open pastures in spring 2019 (A) and 2020 (B). Bars represent SEM.
Land Equivalent Ratio and Net Economic Return of Solar Grazing
Land use efficiency of herbage DM yield (kg DM ha−1 y−1) and lamb liveweight production (kg ha−1 d−1) in agrivoltaics was 1.81 and 2.04, respectively in 2019. The LER of herbage DM yield was 1.68, while the LER of liveweight production was 1.97 in 2020 (Figure 9). Averaged across the years, our net return from grazing was $1,046 ha−1 year−1 in open pastures, and $1,029 ha−1 year−1 in solar areas.
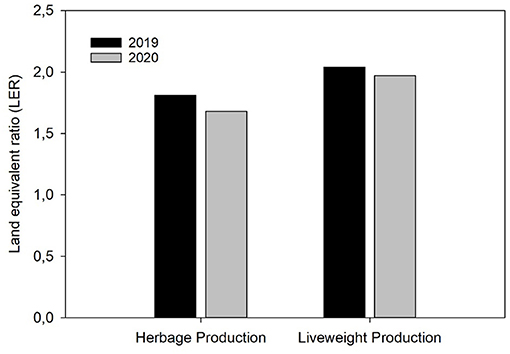
Figure 9. Land equivalent ratio of herbage (kg DM ha−1) and liveweight production (LWP, kg ha−1 d−1) in 2019 and 2020.
Discussion
Shade and Animal Trampling Reduced the Pasture Production in Fully Shaded Areas in Solar Pastures
Light interception is one of the primary drivers of plant growth together with nutrients, temperature and available soil moisture (Rayburn and Griggs, 2020). Previous studies that were conducted in artificial shade conditions (Varella et al., 2001; Dodd et al., 2005) or in silvopastoral systems (Devkota et al., 2009) reported that light was the main determining factor for the understory forage production. In the current study, the lower total annual forage yield in solar pastures compared to open pastures was a consequence of poor production in fully shaded areas. This is also in agreement with the conclusion of Hawke and Knowles (1997) who reported that of all the factors (e.g., nutrients, temperature) shade was the major limiting factor in understory forage production in temperate agroforestry systems.
It was reported that quality of light received by forages under a tree canopy does influence the annual growth cycle of understory forages (Krueger, 1981). A substantial variation in seasonal production patterns annual growth cycle in particular for the forages in fully shaded areas was also detected due to pertinent microclimatic conditions. The lower temperatures early in spring and higher availability of soil moisture later in spring coupled with overall low light intensity under the solar panels delayed the initial flush of spring growth of sparse pastures in these areas. In contrast, growth rates of pastures under the solar panels had an increasing trend toward summer, whereas pasture growth rates in open and partially shaded areas were slowing down during the same periods. Although the greater growth rates of pastures under solar panels was only evident in late spring-early summer in 2019 due to sparsity and lower density of these pastures, high summer production form the forages grown under solar panels could help reduce the summer feed gap when shade tolerant pasture mixtures are established and careful grazing management is applied.
While reduced light is the primary reason for the inferior production in solar pastures, trampling of the forages by livestock in fully shaded areas further penalized the biomass yield. A heavy traffic of livestock as evidenced by the foraging behavior observations resulted in decimation of forages under the solar panels, consequently leading to 9–33% less in solar pastures than open pastures. In traditional open pastures, Edmond (1964) reported a 4–39% reduction in annual biomass production of perennial ryegrass that was trodden nine times by sheep over a period of 11 months, compared with the non-pugged control treatment. Typically, soil consolidation due to pugging in saturated soils can cause severe deterioration to soil physical conditions, such as a reduction of volume of large pores resulting in poor hydraulic conductivity (Drewry, 2006). In contrast, from ungrazed pastures under the solar panels within the same site, Adeh et al. (2018) reported a 90% increase biomass per unit area, and 330% increase in water use efficiency under the solar panels during late spring-summer. This indicates the need for controlling grazing to avoid excess trampling and take advantage of high biomass production potential in solar pastures in late spring-summer period.
It is of note that the current study was conducted on an unimproved, grass-dominated pasture established over 20 years ago and managed with no (e.g., irrigation) or low inputs (e.g., fertilizer, lime). We expect that the pasture conditions are representative to most grazed agrivoltaic systems in Pacific Northwest. Overseeding to improve the forage diversity and quality yielded little benefit with forbs contributing only 3.8% to the botanical composition during the grazing season in spring 2019. The effect of forbs in pasture composition and quality was more pronounced in 2020 when both open (17.2%) and solar pastures (10.2%) had substantially greater forb contents. The lower forb content in solar pastures was mainly because of the poorer establishment under full shade areas. In agreement with our finding, Dodd et al. (2005) also noted a decline in legume content under artificial shade. Consequently, the overall pasture production from both open and solar pastures did not compare favorably to the biomass yield from young, unirrigated pastures in the same location where annual forage production was 13.4 t DM ha−1 y−1 (Wilson, 2020). It was of note that earlier closing of the pastures from grazing in fall 2019 resulted in greater spring-summer 2020 production and extended grazing period for both open and partially shaded pastures, while pasture production in fully shaded areas remained similar.
Lamb Production Did Not Differ Despite Lower Herbage on Offer in Solar Pastures
Feeding value, a function of nutritive value and dry matter intake of the forages is the primary determinant of the animal performance in pasture based-livestock systems (Waghorn and Clark, 2004). Thus, the production level of grazing livestock is highly dependent on pasture quality and daily forage allowance (Penning et al., 1986). In particular, the high forage quality in dryland systems is crucial to bring the lambs to the slaughter weight before the onset of summer drought. The lamb growth rates in the current study was similar to those reported by Warner and Sharrow (1984) for spring (73-165 g head−1 d−1) and early summer (−13–98 g head−1 d−1) periods in a 3 year-grazing study. In contrast, Gultekin et al. (2020) reported greater lamb liveweight gains in dryland hill pastures in Pacific Northwest where the lamb growth rates were maintained over 141 g head−1 d−1 in the late spring season (May-June). It is of note that the newly established pastures reported in Gultekin et al. (2020) study contained over 20% legume indicating the value of high legume content of pastures for improved forage quality and high lamb growth rates particularly in dryland pastures (Hyslop et al., 2000; Mills et al., 2015).
In the current study, the reduction in lamb liveweight gains was of note, despite the lower stocking rate in late, spring and pasture mass being maintained < 1,000 kg DM ha−1. Overall, lamb growth rates and spring lamb production in the current study were similar in both production systems, although herbage mass on offer remained greater in open compared to solar pastures in the late spring period. Jamieson and Hodgson (1979) noted a 39% decline in lamb herbage intake as the herbage mass was reduced from 3,000 to 1,000 kg OM ha−1. In contrast, the overall quality of solar pastures were greater as evidenced by the chemical composition of forage on offer. Although not quantified, we also observed grasses in open pastures contained more seed heads possibly because earlier phenological development as compared to the grasses in shaded areas (Krueger, 1981). It is also probable that at lower pasture masses in solar pastures, lambs may have done a closer grazing, suppressing the stem elongation and seedhead production (Garay et al., 1997). Consequently, it appears that higher forage nutritive value in solar pastures during the same period offset the lower herbage mass, leading to similar lamb liveweight gains in both systems. It is of note that our results on forage quality are also in line with the findings of several studies that reported improved forage nutritive values from both natural and artificial shade environments (Ciavarella et al., 2000; Kallenbach et al., 2006). For example, Dodd et al. (2005) reported a 0.2% increase in herbage N concentration while Ciavarella et al. (2000) noted a 0.6% increase in shaded pasture compared to unshaded pastures.
Lambs Spent Their Time Predominantly Under Shade and had Similar or Lower Water Intake Than Those Grazing Open Pastures
A further reason for comparable lamb growth in both systems might have been due to less heat stress that the lambs experienced under shade in solar pastures. Provision of shade as a mitigation strategy for the heat stress also leads to lower maintenance energy requirement and reduces production losses (Russel and Wright, 1983). It is likely that the lambs grazing under the solar panels might have required less maintenance energy to regulate their body temperatures. In the current study, the lambs in solar pastures spent their ruminating and idling activities predominantly under shade (<96%), while 45% of their grazing activity took place in shade directly under the solar panels. Similarly, in a recent silvopastoral study, Pent et al. (2020) noted that lambs spent over 90% of daylight hours within the boundaries of the shade. Cloete et al. (2000) recorded that lambs born in shaded paddocks were 3.8% heavier at weaning than those were born and raised in paddocks without shade. Similarly, Kendall et al. (2006) recorded higher milk yields from the grazing Holstein Friesian dairy cows that were provided shade in temperate pastures due to reduced level of moderate heat stress.
Foraging behavior observed in spring 2020 did not substantially differ among lambs grazing solar or open pastures, although the lambs grazing solar panels appeared to be more active during noontime. In line with our findings, Rovira and Velazco (2010) noted that provision of shade did not affect grazing behavior of cattle during daylight hours in Uruguay.
A feature of the results was that the lambs grazing solar pastures consumed less water in late spring period in 2019. However, the effect of shade on group apparent water consumption of lambs was not consistent and significant in spring grazing in 2020. Contrasting reports in literature on the response of livestock to shade have also been reported from different climatic zones and with various classes of animals. The studies conducted in the prevailing Mediterranean climatic regions of the world reported that sheep that were provided shade consumed less water than those did not have access to any shade (Olivares and Caro, 1998; Cloete et al., 2000). In contrast, Silanikove (1987) reported no difference between sheltered and unsheltered sheep for their water and feed intake in a hot Mediterranean climate. It is of note that grazing livestock can adapt to the environmental conditions such as heat stress through thermoregulatory responses. However, the benefit of shade provided by solar panels in agrivoltaics on animal welfare and water consumption can be more apparent in hot climatic regions of the world. Furthermore, agrivoltaics systems may alleviate the need of artificial shelter provision to livestock, also reducing the initial infrastructure cost in pasture-based livestock production.
Land Use Efficiency in Agrivoltaics Was Substantially Greater Compared to Single Use System
One of the features of our results was remarkably high land use efficiency in agrivoltaics system as indicated by LER of herbage and spring lamb production. The LER of herbage yield obtained in the current study is quite comparable to LER of grass-clover pasture (1.67–1.70) reported by Trommsdorff et al. (2021). It is of note that solar panels in the Trommsdorff et al. (2021) study was designed for an optimized energy and crop production with panels having a vertical clearance of 5 m and a width clearance of up to 19 m. Despite lower total annual herbage yield in agrivoltaics as compared to traditional open pastures in the current study, combining energy and pasture-based lamb production appears to be greatly advantageous. This finding is in line with Dupraz et al. (2011) who suggested that global land productivity in agrivoltaics systems could be increased by 35–73%. The land use efficiency in agrivoltaics in particular where energy and animal production was combined on the same land can possibly be increased further through more optimized design, choice of shade tolerant pasture species and sustainable livestock management practices.
Our net returns for grazing in solar pastures were 1.6% ($17 ha−1 year−1) less than in open pastures, which is a small percentage when considering the potential profits from photovoltaics energy production in agrivoltaics system. While we only attempted to calculate net profits form spring grazing in the current study, an economic or a life cycle analyses of agrivoltaics system taking into account of photovoltaics component (e.g., establishment, maintenance, energy production) would provide a more detailed analyses from a whole production system standpoint.
Conclusion
This study reveals that successful agrivoltaic systems are possible where lamb and energy production can be produced simultaneously from the same land. Comparable spring lamb growth and liveweight production per hectare from open and solar pastures demonstrate that agrivoltaic systems would not decrease the production value and potential of the land. In contrast, LER indicated that the dual-purpose management enables increasing the land productivity up to 1.81 for pasture production and 2.04 for spring lamb production through combining sheep grazing and solar energy production on the same land as compared to single use systems. In addition to the increased land productivity and improved animal welfare, the results from this study support the benefits of agrivoltaics as a sustainable agricultural system. Overall, lower pasture yields under in fully shaded areas under the solar panels were the main cause of inferior pasture production in agrivoltaic sites in the current study. When designing pasture mixtures for agrivoltaic systems, a selection of pasture species that are not only tolerant to shade but also persistent under heavy traffic should be considered. Limiting the daily grazing time (e.g., on-off grazing: 3 h-grazing/d only) or rotational grazing pastures at low grazing intensities may be viable options for sustainable grazing of seasonally wet soils under solar panels.
Data Availability Statement
The original contributions presented in the study are included in the article/supplementary material, further inquiries can be directed to the corresponding author/s.
Ethics Statement
The animal study was reviewed and approved by Oregon State University Animal Care and Use Committee.
Author Contributions
All authors listed have made a substantial, direct and intellectual contribution to the work, and approved it for publication.
Funding
This research was funded by the Agricultural Research Foundation (ARF) of Oregon State University.
Conflict of Interest
The authors declare that the research was conducted in the absence of any commercial or financial relationships that could be construed as a potential conflict of interest.
Acknowledgments
The authors express their appreciation to Dr. Massimo Bionaz, Yunus Gultekin, Azad Dazaea and Holly Broadbent for providing assistance in fieldwork and undergraduate students for their help with the lamb foraging behavior observations.
References
Adeh, E. H., Good, S. P., Calaf, M., and Higgins, C. W. (2019). Solar PV power potential is greatest over croplands. Sci. Rep. 9:11442. doi: 10.1038/s41598-019-47803-3
Adeh, E. H., Selker, J. S., and Higgins, C. W. (2018). Remarkable agrivoltaic influence on soil moisture, micrometeorology and water-use efficiency. PLoS ONE 13:e0203256. doi: 10.1371/journal.pone.0203256
Amaducci, S., Yin, X., and Colauzzi, M. (2018). Agrivoltaic systems to optimise land use for electric energy production. Appl. Energy 220, 545–561 doi: 10.1016/j.apenergy.2018.03.081
Apostoleris, H., and Chiesa, M. (2019). High-concentration photovoltaics for dual-use with agriculture. AIP Conf. Proc. 2149:050002. doi: 10.1063/1.5124187
Bransby, D. I. (1989). “Compromises in the design and conduct of grazing experiments,” in Grazing Research: Design, Methodology, and Analysis. CSSA Special Publication, ed G. C. Marten (Madison, WI: Crop Science Society of America and American Society of Agronomy), 53–67. doi: 10.2135/cssaspecpub16.c5
Ciavarella, T. A., Simpson, R. J., Dove, H., Leury, B. J., and Sims, I. M. (2000). Diurnal changes in the concentration of water-soluble carbohydrates in Phalaris aquatica L. pasture in spring, and the effect of short-term shading. Aust. J. Agric. Res. 51, 749–756. doi: 10.1071/AR99150
Cloete, S. W. P., Muller, C. J. C., and Durand, A. (2000). The effects of shade and shearing date on the production of Merino sheep in the Swartland region of South Africa. S. Afr. J. Anim. Sci. 30, 164–171. doi: 10.4314/sajas.v30i3.3848
DeMartis, C. (2018). Solar Farming in Maine: An Objective Overview. Student Policy Briefs. Available online at: https://digitalcommons.usm.maine.edu/fsp-policy-briefs/1 (accessed December 7, 2020).
Devkota, N. R., Kemp, P. D., Hodgson, J., Valentine, I., and Jaya, I. K. D. (2009). Relationship between tree canopy height and the production of pasture species in a silvopastoral system based on alder trees. Agroforestry Syst. 76, 363–374. doi: 10.1007/s10457-008-9192-8
Dinesh, H., and Pearce, J. M. (2016). The potential of agrivoltaic systems. Renewable Sustain. Energy Rev. 54, 299–308. doi: 10.1016/j.rser.2015.10.024
Dixon, R. K. (1995). Agroforestry systems: sources of sinks of greenhouse gases? Agroforestry Syst. 31, 99–116. doi: 10.1007/BF00711719
Dodd, M. B., McGowan, A. W., Power, I. L., and Thorrold, B. S. (2005). Effects of variation in shade level, shade duration and light quality on perennial pastures. NZ. J. Agric. Res. 48, 531–543. doi: 10.1080/00288233.2005.9513686
Drewry, J. J. (2006). Natural recovery of soil physical properties from treading damage of pastoral soils in New Zealand and Australia: a review. Agric. Ecosyst. Environ. 114, 159–169. doi: 10.1016/j.agee.2005.11.028
Dupraz, C., Marrou, H., Talbot, G., Dufour, L., Nogier, A., and Ferard, Y. (2011). Combining solar photovoltaic panels and food crops for optimising land use: towards new agrivoltaic schemes. Renewable Energy 36, 2725–2732. doi: 10.1016/j.renene.2011.03.005
Edmond, D. B. (1964). Some effects of sheep treading on the growth of 10 pasture species. NZ. J. Agric. Res. 7, 1–16. doi: 10.1080/00288233.1964.10419994
Garay, A. H., Matthew, C., and Hodgson, J. (1997). Effect of spring grazing management on perennial ryegrass and ryegrass-white clover pastures: 2. Tiller and growing point densities and population dynamics. NZ. J. Agric. Res. 40, 37–50. doi: 10.1080/00288233.1997.9513228
Goetzberger, A., and Zastrow, A. (1982). On the coexistence of solar-energy conversion and plant cultivation. Int. J. Solar Energy 1, 55–69. doi: 10.1080/01425918208909875
Graham, M., and Higgins, C. W. (2019). Pollinator-Focused Solar: Observations in a Dual-Use Agriculture System. AGUFM, 2019, GC31J-1339.
Gultekin, Y., Filley, S. J., Smallman, M. A., Hannaway, D. B., and Ates, S. (2020). Pasture production, persistence of legumes and lamb growth in summer-dry hill pastures. Grass Forage Sci. 76, 159–172. doi: 10.1111/gfs.12497
Hawke, M. F., and Knowles, R. L. (1997). Temperate agroforestry systems in New Zealand, in Temperate Agroforestry Systems, eds A. M. Gordon, and S. M. Newman (Wallingford: CAB International), 85–118.
Hernandez, R., Armstrong, A., Burney, J., Ryan, G., Moore-O'Leary, K., Diédhiou, I., et al. (2019). Techno–ecological synergies of solar energy for global sustainability. Nat. Sustain. 2, 560–568. doi: 10.1038/s41893-019-0309-z
Hyslop, M. G., Fraser, T. J., Smith, D. R., Knight, T. L., Slay, M. W. A., and Moffat, C. A. (2000). Liveweight gain of young sheep grazing tall fescue or perennial ryegrass swards of different white clover content. Proc. NZ. Soc. Anim. Prod. 60, 51–54.
Jamieson, W. S., and Hodgson, J. (1979). The effects of variation in sward characteristics upon the ingestive behaviour and herbage intake of calves and lambs under a continuous stocking management. Grass Forage Sci. 34, 273–282. doi: 10.1111/j.1365-2494.1979.tb01479.x
Kallenbach, R. L., Kerley, M. S., and Bishop-Hurley, G. J. (2006). Cumulative forage production, forage quality and livestock performance from an annual ryegrass and cereal rye mixture in a pine walnut silvopasture. Agrofor. Syst. 66, 43–53.
Kendall, P. E., Nielsen, P. P., Webster, J. R., Verkerk, G. A., Littlejohn, R. P., and Matthews, L. R. (2006). The effects of providing shade to lactating dairy cows in a temperate climate. Livest. Sci. 103, 148–157. doi: 10.1016/j.livsci.2006.02.004
Lima, M. A., Paciullo, D. S., Morenz, M. J., Gomide, C. A., Rodrigues, R. A., and Chizzotti, F. H. (2019). Productivity and nutritive value of Brachiaria decumbens and performance of dairy heifers in a long-term silvopastoral system. Grass Forage Sci. 74, 160–170. doi: 10.1111/gfs.12395
Marrou, H., Dufour, L., Guilioni, L., Salles, J. M., Loisel, P., Nogier, A., et al. (2013). Designing farming systems combining food and electricity production,” in 4th International Symposium for Farming Systems Design (Lanzhou), 109–110.
Mills, A., Lucas, R. J., and Moot, D. J. (2015). “MaxClover” grazing experiment II: sheep liveweight production from six grazed dryland pastures over 8 years. NZ. J. Agric. Res. 58, 57–77. doi: 10.1080/00288233.2014.978481
Olivares, A., and Caro, T. W. (1998). Tree shade effects on water intake and weight gains of grazing sheep. Agro Sur. 26, 77–80. doi: 10.4206/agrosur.1998.v26n1-09
Orr, R. J., Cook, J. E., Young, K. L., Champion, R. A., and Rutter, S. M. (2005). Intake characteristics of perennial ryegrass varieties when grazed by yearling beef cattle under rotational grazing management. Grass Forage Sci. 60, 157–167. doi: 10.1111/j.1365-2494.2005.00463.x
Payne, R. W. (2009). The Guide to GenStat Release 2 Part 2: Statistics. Harpenden: Lawes Agricultural Trust, Rothamsted Experimental Station. doi: 10.1002/wics.32
Penning, P. D., Hooper, G. E., and Treacher, T. T. (1986). The effect of herbage allowance on intake and performance of ewes suckling twin lambs. Grass Forage Sci. 41, 199–208. doi: 10.1111/j.1365-2494.1986.tb01805.x
Pent, G. J., Greiner, S. P., Munsell, J. F., Tracy, B. F., and Fike, J. H. (2020). Lamb performance in hardwood silvopastures, II: animal behavior in summer. Transl. Anim. Sci. 4, 363–375. doi: 10.1093/tas/txz177
Rayburn, E. B., and Griggs, T. C. (2020). Light interception and the growth of pastures under ideal and stressful growing conditions on the allegheny plateau. Plants 9:734. doi: 10.3390/plants9060734
Rovira, P., and Velazco, J. (2010). The effect of artificial or natural shade on respiration rate, behaviour and performance of grazing steers. NZ. J. Agric. Res. 53, 347–353. doi: 10.1080/00288233.2010.525785
Russel, A. J. F., and Wright, I. A. (1983). The use of blood metabolites in the determination of energy status in beef cows. Anim. Sci. 37, 335–343.
Silanikove, N. (1987). Impact of shelter in hot Mediterranean climate on feed intake, feed utilization and body fluid distribution in sheep. Appetite 9, 207–215. doi: 10.1016/S0195-6663(87)80014-4
Trommsdorff, M., Kang, J., Reise, C., Schindele, S., Bopp, G., Ehmann, A., et al. (2021). Combining food and energy production: design of an agrivoltaic system applied in arable and vegetable farming in Germany. Renewable Sustain. Energy Rev. 140:110694. doi: 10.1016/j.rser.2020.110694
USDA Agricultural Marketing Service (2018). Annual Statistics Summary: Livestock, Poultry, and Grain Market News (2018 Livestock, Meat, Poultry, Grain and Slaughter Data). USDA Agricultural Marketing Service.
USDA Soil Survey Staff (2020). Web Soil Survey. Washington, DC: USDA-NRCS. Available online at: https://websoilsurvey.sc.egov.usda.gov (accessed November 12, 2020).
Varella, A. C., Moot, D. J., Lucas, R. J., McNeil, D. L., Peri, P. L., and Pollock, K. M. (2001). “Different methods of artificial shade for agro-silvipastoral research,” in XIX International Grassland Congress (Sao Pedro, São Paulo).
Waghorn, G. C., and Clark, D. A. (2004). Feeding value of pastures for ruminants. NZ. Vet. J. 52, 320–331. doi: 10.1080/00480169.2004.36448
Warner, J. R., and Sharrow, S. H. (1984). Set stocking, rotational grazing and forward rotational grazing by sheep on western Oregon hill pastures. Grass Forage Sci. 39, 331–338. doi: 10.1111/j.1365-2494.1984.tb01704.x
Keywords: grazing, solar farming, pasture production, sustainability, agrivoltaics, solar grazing, dual-use agriculture, food energy water nexus
Citation: Andrew AC, Higgins CW, Smallman MA, Graham M and Ates S (2021) Herbage Yield, Lamb Growth and Foraging Behavior in Agrivoltaic Production System. Front. Sustain. Food Syst. 5:659175. doi: 10.3389/fsufs.2021.659175
Received: 27 January 2021; Accepted: 26 March 2021;
Published: 29 April 2021.
Edited by:
Bruno José Rodrigues Alves, Brazilian Agricultural Research Corporation (EMBRAPA), BrazilReviewed by:
Lydia Cranston, Massey University, New ZealandSusan Robertson, Charles Sturt University, Australia
Copyright © 2021 Andrew, Higgins, Smallman, Graham and Ates. This is an open-access article distributed under the terms of the Creative Commons Attribution License (CC BY). The use, distribution or reproduction in other forums is permitted, provided the original author(s) and the copyright owner(s) are credited and that the original publication in this journal is cited, in accordance with accepted academic practice. No use, distribution or reproduction is permitted which does not comply with these terms.
*Correspondence: Serkan Ates, c2Vya2FuLmF0ZXNAb3JlZ29uc3RhdGUuZWR1