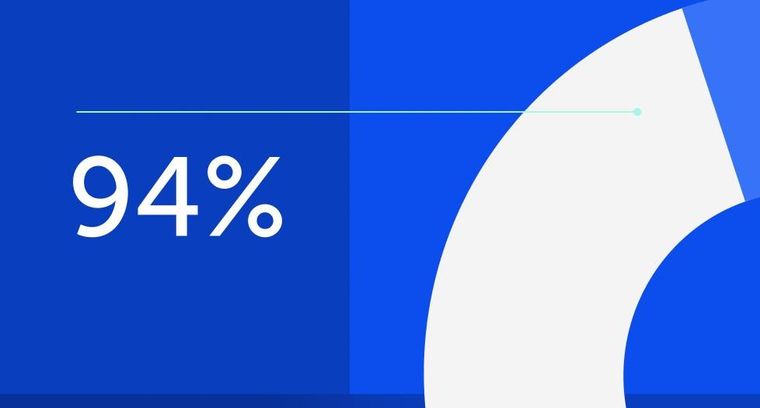
94% of researchers rate our articles as excellent or good
Learn more about the work of our research integrity team to safeguard the quality of each article we publish.
Find out more
REVIEW article
Front. Sustain. Food Syst., 25 February 2021
Sec. Crop Biology and Sustainability
Volume 5 - 2021 | https://doi.org/10.3389/fsufs.2021.606454
This article is part of the Research TopicPlant Growth-Promoting Microorganisms for Sustainable Agricultural ProductionView all 50 articles
Plant-microbe interactions have been the subject of several biotechnological studies, seeking sustainable development and environmental conservation. The inoculation of plant growth-promoting microbes (PGPM) in agricultural crops is considered an environmental-friendly alternative to chemical fertilization. Microbial inoculants are mainly inoculated onto seeds, roots and soil. PGPM improve plant growth by enhancing the availability of nutrients, the regulation of phytohormones, and by increasing plant tolerance against biotic and abiotic stresses. One of the main obstacles with PGPM research are the inconsistent results, which may be the result of inoculation methods and abiotic factors, such as soil (nutrient or heavy metal contents and pH), water availability, light intensity and temperature. This review addresses how the PGPM inoculants act on plant growth, what mechanisms they use to survive under stressful environmental conditions, and how inoculation methods and abiotic factors can interfere on the success of microbial inoculation in plants, serving as a basis for research on plants-microorganisms interaction.
The growth rate of global population demands for increasing food production. However, in many situations, boosting agricultural productivity relies heavily on the use of chemical fertilizers, which are economically unavailable to many farmers throughout the world and can cause negative environmental impacts. In addition, environmental stresses may also be major constraints to plant growth and yield, causing low crop productivity, affecting global food security (Souza et al., 2015; Mimmo et al., 2018; Khan et al., 2019; Asghari et al., 2020). Therefore, to increase global agricultural production in a more economically and environmentally sustainable way, there is the need to use less chemical fertilizers and increase plant tolerance to abiotic stresses. The use of plant growth-promoting microbes (PGPM) is a potentially advantageous technique for improving crop productivity, food quality and security in more sustainable and eco-friendly agricultural systems (Souza et al., 2015; Abhilash et al., 2016; Mimmo et al., 2018; Asghari et al., 2020; Etesami, 2020).
Rhizosphere fungal and bacterial community can harbor beneficial organisms known as PGPM. These organisms have the ability to colonize plant roots providing benefits to their hosts, by modulating the production of phytohormones, increasing the availability of soil nutrients, and the resistance against pathogens. Besides, minimizing the use of chemical fertilizers, mitigating biotic and abiotic stresses, and increasing plant production (Abhilash et al., 2016; Asghari et al., 2020; Etesami, 2020). The microorganisms used to increase agriculture productivity are Azospirillum, Bacillus, Burkholderia, Enterobacter, Flavobacterium, Pseudomonas, Rhizobium, Frankia, Klebsiella, Clostridium, Trichoderma, Beauveria, Serratia and Streptomyces (Abhilash et al., 2016; Oosten et al., 2017; Gouda et al., 2018).
PGPM act as biofertilizer, increasing the availability of nutrients, through bio-fixation of atmospheric nitrogen and solubilization of soil minerals, such as phosphorus and potassium. There are rhizobacteria that can facilitate the production of siderophores enhancing iron update (Bhat et al., 2019). They also directly promote plant growth as phytostimulator, influencing the phytohormones metabolism by enhancing auxin, cytokinins, abscisic acid, gibberellins production, and reduction of ethylene (Martínez-Viveros et al., 2010; Bhat et al., 2019; Khan et al., 2020). PGPM also act indirectly, as biopesticide or biocontrol agents increasing resistance against phytopathogens, through competition for nutrients, antagonism and induces systemic resistance (Abhilash et al., 2016; Bhat et al., 2019; Khan et al., 2020).
Considering all known factors that are involved in the PGPM activity, it is common to find numerous responses to inoculation with promising strains, which may be the result of the inoculation method and abiotic factors (Egamberdiyeva, 2007; Hernández-Montiel et al., 2017; Dutta and Bora, 2019; Fleming et al., 2019; Salwan et al., 2019; Etesami, 2020). To increase the success in the use of microbial biotechnology it is necessary to know how to improve plant-PGPM interaction, and how PGPM respond to changing environmental conditions, since terrestrial ecosystems are increasingly under anthropogenic influence. Therefore, this review addresses how the PGPM inoculants act on plant growth, what mechanisms they use to survive under stressful environmental conditions, and how inoculation methods and abiotic factors can interfere on the success of microbial inoculation on plant development.
Microbial inoculants combined or separate, can be inoculated into seed, leave, seedling roots, or soil. They colonize the rhizosphere or the interior of the plant, stimulating growth and plant tolerance against abiotic stresses. PGPM directly promote plant growth by enhancing the availability of nutrients, phytohormones regulation, and indirectly inducing systemic resistance (Abhilash et al., 2016; Bhat et al., 2019; Khan et al., 2020; Khoshru et al., 2020).
Under stress conditions plant growth is inhibited, mainly due to the increase in the production of reactive oxygen species, lipid peroxidation, accumulation of free radicals and high ethylene production, causing cell death. Hence, result in chlorosis, necrosis, leaf senescence, damage in photosynthesis apparatus, reduction in photosynthetic rates and chlorophyll content, and change in concentrations of metabolites. It also affects seed germination, seedling vigor, plant height, root development, reduce the biomass and productivity of crop plants (Sharma et al., 2012; Khoshru et al., 2020).
On the other hand, beneficial microbes improve plant growth by enhancing the availability of nutrients, the regulation of phytohormones, and by increasing plant tolerance against biotic and abiotic stresses. Based on its effects mentioned above, PGPM increase the nutritional, auxin, gibberellin, cytokinin and ACC-deaminase concentrations. Beneficial microbes can also secrete volatile metabolites (VOC), which can induce disease resistance and abiotic stress tolerance. In addition, PGPM can also mitigate stress by increasing exopolysaccharides, osmoregulants and antioxidants, and reducing the oxidative stress (Varma et al., 2019; Khan et al., 2020; Khoshru et al., 2020). Thus, PGPM promote the increase foliage and leaf area, chlorophyll content, photosynthetic rates, seed germination, seedling vigor, plant height, root development, and biomass production (Figure 1).
Figure 1. Beneficial microbes improve plant growth by enhancing the availability of nutrients, the regulation of phytohormones, and increasing plant tolerance against stresses. PGPM act as biofertilizer, increasing macro and micronutrient availability. They increase the concentrations of auxin, gibberellin, cytokinin, ACC-deaminase, and reduce ethylene levels. Beneficial microbes also produce volatile metabolites (VOC), that may induce disease resistance and abiotic stress tolerance. In addition, PGPM mitigate stress by increasing exopolysaccharides, osmoregulants (such as glycinebetaine—GB), and antioxidants (including catalase—CAT, superoxide dismutase—SOD, ascorbate—AsA and glutathione—GSH), reducing the reactive oxygen species—ROS and oxidative stress. Thus, PGPM promote the increase of leaf area, chlorophyll content, photosynthetic rates, seed germination, seedling vigor, plant height, root development and biomass production of plants.
PGPM has been studied as biofertilizer which could enhance the supply of macro and micronutrients, promote plant growth and reduce the need of chemical fertilization. Nitrogen, phosphorus and iron are essential nutrients for plants. Hence, in PGPM selection test, the nitrogen fixation, phosphate solubilization and siderophore production capacity are usually investigated.
Nitrogen is an essential macronutrient for synthesize of proteins and nucleic acids. The microbes viz., Azospirillum, Azotobacter, Achromobacter, Bradyrhizobium, Beijerinckia, Rhizobium, Clostridium, Klebsiella., Anabaena., Nostoc, Frankia are biological nitrogen fixers through reduction of nitrogen gas (N2) to ammonia (NH3) (Souza et al., 2015; Bhat et al., 2019).
Phosphorus is an essential macronutrient for production of phospholipids, adenosine triphosphate (ATP), and increase the photosynthesis. Nonetheless, a large proportion of P in the soil is in insoluble forms, making it unavailable for the plants. PGPM changes the pH of the soil to solubilize inorganic phosphates. In alkaline soils, PGPM reduces pH by excretion of organic acids, such as gluconate, citrate, lactate and succinate, solubilizing Ca3(PO4)2. In acid soils, PGPM increases the pH by production of protons, during the assimilation of ammonium (), solubilizing AlPO4 and FePO4 (Martínez-Viveros et al., 2010). Bacillus, Pseudomonas, Rhizobium, Achromobacter, Burkholderia, Microccocus, Agrobacterium, Erwinia sp., Penicillium sp. and Aspergillus sp. are capable of solubilizing inorganic phosphorus, transforming into forms capable of being absorbed by plants, such as monobasic () or dibasic phosphate (HPO4−2).
Iron is a micronutrient required to chlorophyll biosynthesis, photosynthesis and respiration. Burkholderia, Enterobacter, Grimontella and Pseudomonas are siderophore producers. Siderophores are chelator agents, with high specificity for binding iron, followed by the transportation and deposition of Fe3+ within bacterial cells. In this way, the excretion of siderophores improve plant nutrition and inhibit phytopathogens through iron sequestration from the environment (Souza et al., 2015; Varma et al., 2019).
Sulfur is an essential macronutrient found in cysteine and methionine. These amino acids are important in maintaining of enzymes and protein synthesis. Cysteine is important in cell division, and methionine is a precursor of ethylene, responsible for fruit ripening (Taiz and Zeiger, 2017). Bacillus are producers of volatile compounds, such as dimethyl disulfide that provides sulfur for plants. In addition, Bacillus and Aspergillus produces organic and inorganic acids, acidolysis, chelation and exchange reactions which are capable of solubilize potassium (Varma et al., 2019).
Phytohormones are organic compounds responsible for the development of plants. There are PGPM capable of modulating phytohormones. The effects of stress on plants are mitigated by microbial inoculants, through the production of auxin, cytokinin, gibberellin, ACC deaminase, abscisic acid, jasmonates, brassinosteroids, and strigolactones (Saravanakumar, 2012; Oosten et al., 2017; Arora et al., 2020; Khan et al., 2020).
Auxin and ACC-deaminase are usually investigated in PGPM selection tests. This is because, auxin produced by microorganisms will increase auxin in the plant, and promote plant growth by enhancing nutrient and water uptake. Microbial auxins also beneficial in the regulation of cell division, shoot growth, differentiation of vascular tissue, adventitious and lateral root, elongation and surface area of root. ACC-deaminase produced by microorganisms is a beneficial enzyme for reducing ethylene levels, mitigating stress in plants. High ethylene levels cause leaf chlorosis, necrosis, senescence, reduction in fruit yield, root development, leaf expansion, and photosynthesis (Souza et al., 2015). Pseudomonas sp. and Bacillus promote plants growth by increase auxin and ACC-deaminase (Samaddar et al., 2019; Danish et al., 2020; Khoshru et al., 2020).
PGPM can also promote plant growth by increasing gibberellin, improving seed germination, and the development of stem, leaves, flower and fruit. In addition, PGPM-induced cytokinin result in increased roots development, activity of vascular cambium, cell differentiation, and apical dominance (Gouda et al., 2018; Khan et al., 2020). Under stress conditions, PGPM inoculants are able to increase abscisic acid, jasmonates and brassinosteroids concentrations in plant. Under drought, cytokinin increase the abscisic acid, causing stomatal closure to reduce foliar water loss (Arora et al., 2020). Under drought or low temperature, jasmonates and brassinosteroids increase Ca2+ concentration in plant, acting intracellularly as secondary messenger under stress conditions (Oosten et al., 2017; Gouda et al., 2018; Khan et al., 2020).
Microbes are known to produce exopolysaccharides, forming a protective biofilm on root surface. This mechanism enhances water retention in soil particles and maintaining soil moisture in the root zone. In this way, it protects root cell against osmotic and ionic stress, regulating osmotic balance, under changing pH, saline stress, drought and temperature extremes.
To mitigate stress PGPM produce exopolysaccharides. This mechanism acts to stabilize the soil ionic balance, immobilizing Na+ under salinity stress. Exopolysaccharides are produced by Bacillus to increase its antimicrobial activity in the soil (Hashem et al., 2019).
PGPM inoculants also promote plant growth and tolerance to abiotic stresses by increasing antioxidants levels, reducing reactive oxygen species (ROS) and oxidative stress. Temperature, pH, heavy metal, water availability and UV-B radiation cause disruption of cellular homeostasis, increasing of reactive oxygen species, such as superoxide anion, hydroxyl radical, hydrogen peroxide and singlet oxygen. High concentrations of ROS is a primary result of abiotic stress, and are extremely harmful, causing oxidative stress in cell. Moreover, in chloroplasts, mitochondria, and peroxisomes, ROS induce oxidative damage to lipids, proteins, nucleic acid, enzyme inhibition and activation of programmed cell death (Sharma et al., 2012; Khoshru et al., 2020).
Microbial inoculants reduce the damaging effects of ROS, thus securing the cell, membranes, and biomolecules by increasing the production of antioxidants such as catalase (CAT), superoxide dismutase (SOD), ascorbate peroxidase (AsA), glutathione (GSH), carotenoids, tocopherols and phenolics (Gouda et al., 2018; Arora et al., 2020). Environmental stresses cause either reduction of CAT activity and increase the hydrogen peroxide (H2O2).
Catalase is also produced by microbes. Although it is relatively simple to examine whether microbes are catalase producers, this investigation is usually not performed on screening tests (Romeiro, 2007; Varma et al., 2019). The production of catalase by microorganisms must be carried out in screening tests, mainly with the objective of increasing the plant tolerance against abiotic stresses. This enzyme is efficient in H2O2 degradation, reducing ROS and oxidative stress, consequently increase the plant tolerance to abiotic stress. This is the simple analyses in PGPM, which should be routine in the selection tests.
Under stress conditions, microbial inoculants induce production of osmoregulants, such as carbohydrates, proteins, amino acids, lipids, proline, glycinebetaine, and trehalose (Oosten et al., 2017; Gouda et al., 2018; Khoshru et al., 2020). Thus, osmoregulation maintains the homeostasis, preventing membrane plasmolysis, increasing synthesis of heat shock proteins (HSPs) and regulating biological enzymatic mechanisms (Oosten et al., 2017; Khoshru et al., 2020). Under salinity, osmoregulators stabilize the osmotic balance across the membrane, maintain the turgor pressure, and ensure the correct folding of proteins (Sharma et al., 2012; Khoshru et al., 2020). Burkholderia sp. increases plant tolerance against low temperature by modifying carbohydrate metabolism (Fernandez et al., 2012). Under water stress, Pseudomonas fluorescens promote plant tolerance by increasing the activity of catalase and peroxidase, and the accumulation of proline (Saravanakumar et al., 2011). Beneficial microbes improve tolerance in plants, increasing the accumulation of osmolytes in the plant cell cytoplasm. This maintains the cell turgor and contributes to improved stress tolerance in plants (Khoshru et al., 2020). The osmoregulation mechanism is important for plants to survive and improve tolerance under extreme conditions by reducing cellular damage caused by abiotic stress (Fernandez et al., 2012; Khoshru et al., 2020).
Soil represents a heterogeneous environment that allows the development of many microorganisms, which are in continuous interaction with other species, under conditions of symbiosis, antagonism, mutualism, parasitism and as saprophytes (Gouda et al., 2018; Bhat et al., 2019). In the soil, PGPM inhabits the rhizosphere zone, playing a symbiotic mutualistic relationship with plants (Gouda et al., 2018).
The knowledge on physiological characteristics of the microbial inoculant and the host plant is essential to decide the best inoculation method (Strigul and Kravchenko, 2006; Souza et al., 2015; Etesami, 2020). Successful microbial inoculation depends on inoculation method, inoculum density, root colonization, which varies with multiplication and distribution microbes through the rhizosphere, microbial antagonism, plant physiological state, soil humidity, pH, temperature, host, and root exudates (Venturi and Keel, 2016; Hernández-Montiel et al., 2017; Msimbira and Smith, 2020). Microbial inoculants are placed mainly into seeds (Romeiro, 2007; Souza et al., 2015, Arora et al., 2020). However, exudate compounds vary with plant genotype and age, and are determinants for microorganism colonization. Therefore, the effects of different inoculation methods should be evaluated, as it influences the PGPM colonization and the success in promoting plant growth (Romeiro, 2007; Souza et al., 2015; Hernández-Montiel et al., 2017; Arora et al., 2020).
Abiotic conditions, such as soil nutrients, pH, heavy metals, drought, flood, light intensity, and temperature can change the rhizosphere, affecting the survival, diversity of microbes, and PGPM potential for improving plant growth and health (Souza et al., 2015; Venturi and Keel, 2016; Hernández-Montiel et al., 2017; Mahmood et al., 2019; Etesami, 2020).
Temperature, water availability and light intensity can modify the soil compositions, structure and moisture, C and N transformation, metabolic processes and microbial survival. The soil fertilizer, composition, moisture and organic matter modify pH, thus influence the nutrients availability and mineral toxicity, including iron and aluminum. Soil surface exposure to sunlight, more specifically gamma rays and ultraviolet light can cause mutations in the DNA of microorganisms or kill them (Mahmood et al., 2019; Varma et al., 2019; Etesami, 2020).
Soil conditions modify the microbiome diversity. In acidic soils, fungal activities are favored, while in alkaline soils are dominated by bacteria. Fungi disperse more intensively in the soil, while bacteria have access to smaller pore spaces only. Low soil moisture is detrimental mainly to bacteria, because they are single-celled organisms. On the other hand, fungal spores are more resistant, and survive in inactive states. High soil moisture, as in flooding environments, reduces oxygen, consequently reduces the redox potential of the soil and the mineralization of organic matter, resulting in lower biodiversity of microorganisms (Gouda et al., 2018; Etesami, 2020). Therefore, the changes in plant metabolism, composition of root exudates, and rhizosphere environment caused by abiotic factors, can negatively affect inoculation and necessities the reinoculation of microbes through soil drench.
The beneficial PGPM-plant interaction requires that the microorganisms are able to use root exudates for colonizing roots, quickly proliferate, compete with the native microbiota and adapt to environmental changes to mitigate abiotic stresses in plants (Souza et al., 2015; Hernández-Montiel et al., 2017; Mimmo et al., 2018; Msimbira and Smith, 2020).
To survive under stressful environments and promoting plant growth, the microbes developed several mechanisms. Some survive in low and high temperatures (psychrophiles and thermophiles), saline conditions (halophiles), and in acid and alkaline conditions (acidophiles and alkaliphiles) (Romeiro, 2007; Souza et al., 2015; Khoshru et al., 2020). Cell wall modification, alteration in metabolic responses and gene expression are also mechanisms against environmental stress (Sharma et al., 2012; Khoshru et al., 2020).
Quorum sensing is a communication system that enable the host colonization and the survival of microbe under stress conditions. It involves intercellular signaling and the regulating of microbe population. Some microorganisms, as Bacillus, secrete volatile metabolites (VOC), such as alkyl sulfides, indole, and terpenes. VOC can facilitate signaling across, microbial interactions by distributing easily through pores in the soil (Hashem et al., 2019; Varma et al., 2019).
Under low soil moisture, microbes accumulate amino acids, reducing their water potential, avoiding dehydration and death. Arbuscular mycorrhizal fungi modify the rhizosphere by amassing glomalin protein, increase the absorptive surface area and water holding capacity (Varma et al., 2019).
Under high light intensity, some microorganisms, such as Bacillus and Serratia, have pigmented that filter radiation and prevent DNA damage (Moeller et al., 2005; Zion et al., 2006; Varma et al., 2019). In pH extremes, microbes use proton transfer systems in their cytoplasm to maintain osmotic balance, control metabolic activities, and their cellular vitality. Some microbes, such as Azospirillum, Pseudomonas, and Bacillus can influence soil micronutrient availability by solubilization, chelation, oxidation reduction reactions, and alter soil pH acidifying their surroundings, and inhibiting other microbes (Souza et al., 2015; Abhilash et al., 2016; Oosten et al., 2017).
Inoculation methods introduce PGPM to host plant and influence the establishment and persistence of microorganism populations in the rhizosphere and their growth promoting effects (Strigul and Kravchenko, 2006; Hernández-Montiel et al., 2017). Inoculation should be carried out as close as possible to the rhizosphere, as there is a certain inability of microorganisms to move from the inoculation site to the rhizosphere. This is because microorganisms are not usually very mobile in the soil and, therefore, it has been suggested that nematodes are vectors for spreading microorganisms around the rhizosphere (Strigul and Kravchenko, 2006; Hernández-Montiel et al., 2017).
Besides inoculum density and inoculation method, plant response to PGPM inoculation depends on root colonization, which varies with microorganism's multiplication and distribution through the rhizosphere, microbial antagonism, soil humidity, pH, temperature, host, root exudates, as well as the plant physiological state (Venturi and Keel, 2016; Hernández-Montiel et al., 2017; Msimbira and Smith, 2020). After inoculation, the decrease in microorganism population, may be related to difficulties in adapting to their new environment. However, root exudates play a significant role in microorganisms' growth. Several biotic and abiotic factors influence the structural and functional diversity of microorganism communities. In this way, it is necessary to evaluate and select microorganisms from site-specific plant associations. The objective is to optimize the inoculant for applications in plant production. The physiological characteristics of the inoculant organism determine to a great extent its fate and activity in the soil (Strigul and Kravchenko, 2006; Souza et al., 2015; Etesami, 2020).
Inoculation can be performed with a single isolate or with more than one, called co-inoculation. In co-inoculation, microorganisms interact synergistically, increasing the efficiency of inoculation, resulting in improved plant development. Lopes et al. (2018a) in an assay comparing individual inoculation and co-inoculation of Pseudomona fluorences and Burkholderia pyrrocinea, found that these rhizobacteria when co-inoculated, stimulated root development, and increased growth and productivity of the tropical forage grass Brachiaria brizantha. Co-inoculation of PGPM also increased growth and quality in Triticum spp. (Upadhyay et al., 2011), Glycine max L. (Bakhshandeh et al., 2020), Capsicum annuum L. (Samaddar et al., 2019) and Mentha pulegium L. (Asghari et al., 2020).
Positive results obtained with co-inoculations, reinforce the importance of additional research to elucidate the interactions between microorganisms, envisioning the production of mixed inoculants, as an alternative of greater success of microbial biotechnology.
For the inoculation of plants with beneficial microorganisms, different techniques are being employed including seed, root, soil, and foliar inoculation (Figure 2; Table 1). The foliar inoculation is the least used, while seed inoculation are the most used methods (Romeiro, 2007; Souza et al., 2015; Arora et al., 2020). Nevertheless, variation in the composition and quantity of root exudates during plant growth and also environmental stresses, can influence the success of the microbe inoculants. It is therefore necessary to test different inoculation methods in the screening tests.
Seed inoculation method with PGPM is an alternative to chemical seed treatments. It consists in immersing the seed in a microorganism solution of known concentration (Romeiro, 2007; Lopes et al., 2018a). The seed germination process releases abundant carbohydrates and amino acids in the form of seed exudates (Ahemad and Kibret, 2014). In this way, these organisms introduced together with the inoculated seeds in the soil use the exudates as a nutritional source and colonize roots, as soon as they emerge (Ammor et al., 2008).
Interaction of PGPM with the plant roots modulate the level of phytohormones that are produced by plants. Phytohormones are organic compounds that modulate plant growth and are also capable of inducing tolerance in plants against various biotic and abiotic stresses (Khan et al., 2020). Microorganisms colonize plant tissues and synthesize phytohormones, such as gibberellin that improve germination (Bhat et al., 2019). PGPM is also capable of producing antimicrobial compounds that protect seeds against phytopathogens that cause seed rotting (Souza et al., 2015).
Seeds inoculation with Rhizobial and Bacillus sp. increased biomass production of Oryza sativa (Ullah et al., 2017) and Cicer arietinum L. (Khan et al., 2019), respectively. Seed inoculation with mycorrhizal fungi and plant growth-promoting rhizobacteria was more effective in promoting growth and wood production in Schizolobium parahyba, as compared to the seedling inoculation (Cely et al., 2016). In seed inoculation method, the inoculum remains dormant in the soil, until activated by the growing root tips. Under field conditions, it is often necessary to reinoculated to maintain effective cell densities (Martínez-Viveros et al., 2010). Seed inoculation method with Burkholderia phytofirmans has also been successfully used in phytoremediation of organic pollutants such as hydrocarbons (Afzal et al., 2013). Pseudomonas fluorescens was also beneficial when inoculated in the seed for increasing the vigor, biomass and resistance to water stress of Vigna radiata (Saravanakumar et al., 2011).
Root inoculation method consists of immersing roots in a microorganism solution (Romeiro, 2007). After inoculation, the seedling is planted on a proper substrate for its development. This method allows plant size standardization, as inoculation can be carried out on seedling of similar sizes. Another advantage of this inoculation method is that the inoculum is placed directly in contact with the host roots, improving root colonization (Ahemad and Kibret, 2014). This method can be preferentially used in plant species with asexual propagation, as PGPM have the ability to synthesize growth phytohormones such as auxin, that besides promoting plant growth, can also counteract phytopathogens that compromise plant survival after planting (Ahemad and Kibret, 2014; Gouda et al., 2018).
Root inoculation with Burkholderia sp. increased Vitis vinifera tolerance to low temperature, modified carbohydrate metabolism and increased plant growth and yield (Fernandez et al., 2012). In Oryza sativa, root inoculation with Rhizobial was more effective in increasing plant height and panicle length, as compared to the seed inoculation method (Ullah et al., 2017). These results also prove that inoculation method can influence the beneficial effect of microorganism in promoting plant growth.
Soil inoculation method consists of introducing PGPM directly into the soil, by drenching, soil incorporation (mixed in the substrate) or microcapsules (Romeiro, 2007; Hernández-Montiel et al., 2017; Prisa, 2020). In soil drenching, a microorganism solution is added as close as possible to the host roots (Romeiro, 2007; Lopes et al., 2018a). This is necessary because it is in the rhizosphere that the PGPM will be able to perform several critical functions for promoting plant development, such as phosphate solubilization, synthesis of siderophores and phytohormones (Gouda et al., 2018).
Inoculation of the forage grass (Brachiaria brizantha) with Burkholderia pyrrocinia and Pseudomonas fluorescens was not successful when carried out on seeds, but promote growth when inoculated by soil drenching 14 days after seedling emergence. This is because allelochemicals with negative allelopathic effects in these PGPM have been reduced over the growth stages of B. brizantha (Lopes et al., 2018a). In Cicer arietinum L., the soil inoculation of Bacillus resulted in better nodulation and growth than when inoculated on seeds (Bhattacharjya and Chandra, 2013).
Plant growth promoting rhizobacteria inoculated by soil incorporation, improved Ranunculus asiaticus growth, increasing the efficiency of nutrient and water absorption by roots (Prisa, 2020). Burkholderia phytofirmans was more efficient in improving Lolium multiflorum biomass production, when inoculated in soil. When inoculated in seeds, root or leaves, the indigenous microbiota made it difficult for inoculated bacteria to colonize successfully and promote plant growth (Afzal et al., 2013).
In Lycopersicon esculentum, growth and productivity were increased by soil inoculation with Pseudomonas putida delivered in microcapsules. According to Hernández-Montiel et al. (2017), soil inoculation with microcapsules offered greater protection and viability, since the release was gradual, improving adhesion, stability, and colonization of roots by PGPM.
Microbial activity in the soil is influenced by plant roots, soil structure and particle size, mineral composition and agricultural practices (Doornbos et al., 2012; Hartman and Tringe, 2019). Due to the production of root exudates, most microorganisms are accumulated in the rhizosphere. The rhizosphere is the region of the soil connected to plant roots, where compounds are exuded by plant roots to attract organisms. These compounds, can be beneficial, neutral, or harmful to plants (Doornbos et al., 2012; Souza et al., 2015; Bhat et al., 2019; Mahmood et al., 2019). Host plant uses root exudation compounds to select specific microbes in its rhizosphere microflora, establishing plant species-specific rhizosphere communities (Doornbos et al., 2012; Hartman and Tringe, 2019). Root exudation also determines which organisms will produce mucilage in the root system, reducing the roots peeling and improving the contact between the roots and the soil solution (Doornbos et al., 2012; Venturi and Keel, 2016; Gouda et al., 2018).
Abiotic factors can cause stress that interfere with plant metabolism, modifying root exudates compositions. This can affect the rhizosphere microbiome and plant-microbe interactions and, consequently, impair the potential benefit of PGPM to host plant (Figure 3). Abiotic stresses are responsible for most of the major losses in crop productivity. This is a serious problem to global food security. Utilization of PGPM may be an eco-friendly, sustainable, and cost-effective approach to overcome abiotic stresses in plants (Table 2).
Figure 3. Abiotic factors can cause stresses that impacts the plant metabolism, modifying root exudates compositions. This can affect the rhizosphere microbiome and plant-microbe interactions and, consequently, impair the potential benefit of plant growth-promoting microbes (PGPM) to host plant.
However, if there is a change in the exudative pattern of the plant, the same isolate and the same plant genotype may interact differently. Such changes can lead to genetic changes in microorganisms causing then to lose the ability to colonize the rhizosphere and, consequently, their PGPM potential (Oosten et al., 2017; Enebe and Babalola, 2018; Hartman and Tringe, 2019).
Soil pH is key for the solubility of different metal ions, nutrient availability and soil physical properties (Dutta and Bora, 2019; Msimbira and Smith, 2020). High or low soil pH is a worldwide problem to agricultural productivity (Dutta and Bora, 2019; Salwan et al., 2019; Zerrouk et al., 2019). Salinity of agriculture soils is also a serious constraint to plant growth. This stress condition causes nutrient deficiency, ion toxicity, osmotic and oxidative stress, reducing yield of agricultural crops (Dutta and Bora, 2019; Salwan et al., 2019).
Under salinity conditions or in alkaline soils, the high pH, affects the bioavailability of nutrients, causing osmotic stress, nutrient deficiency and increase in the reactive oxygen species production (Dutta and Bora, 2019; Salwan et al., 2019). In acidic soils, the low pH and high aluminum ions concentration cause toxicity and formation of phosphoric acid complexes, which makes phosphorus unavailable to plants (Dutta and Bora, 2019; Zerrouk et al., 2019).
The pH range 5.5–6.5 is optimal for plant growth and increasing production of root exudates to microbes. Bacteria are favored by neutral pH, but the fungi are favored by acidic pH conditions (Msimbira and Smith, 2020). Therefore, pH interferes in plant metabolism, which can also disrupt biological activities, inhibiting the microorganisms that inhabit the rhizosphere (Salwan et al., 2019). Saline stress inhibits seed germination, causes stomata closure affects seedling growth, the onset of flowering and fruiting set (Enebe and Babalola, 2018; Salwan et al., 2019). Aluminum toxicity reduces cell division, root growth, nutrient absorption, and phenolic metabolite production and increases reactive oxygen species production (Zerrouk et al., 2019; Msimbira and Smith, 2020).
Plant beneficial microorganism inoculation is a suitable strategy to improve plant tolerance to pH extremes, as reported to Bacillus and Trichoderma in Glycine max (Bakhshandeh et al., 2020), and Rhizobium and Paenibacillus increase pH tolerance in Triticum (El-Sayed and Hagab, 2020). Pseudomonas promote growth and increases tolerance to high salinity conditions in Capsicum annuum (Samaddar et al., 2019) and in Zea mays under salt and aluminum toxicity (Zerrouk et al., 2019) (Table 2).
Soil nutritional condition can also affect the PGPM efficiency. In soils with high nutrient profile there can be absence of root colonization; this is due to microorganism displacement to regions more abundant in nutrients (Egamberdiyeva, 2007; Bhat et al., 2019). Mathematical modeling indicates that PGPM inoculation is more efficient in nutrient poor soils, or stressed soils, because the development of the resident microflora is inhibited (Strigul and Kravchenko, 2006). Inoculation with Pseudomonas, Bacillus, and Mycobacterium are often more effective in promoting plant growth in nutrient-deficient soils (Egamberdiyeva, 2007; Mathimaran et al., 2020).
Heavy metal contamination in soils is toxic to most organisms and can also inhibit the effectiveness of inoculants. Heavy metals reduce soil fertility, affect the rhizosphere microbial community, plant photosynthetic efficiency, causes nutrient imbalance, and reduce yields (Mimmo et al., 2018). Beneficial microorganisms, such as Pseudomonas aeruginosa, Alcaligenes feacalis, and Bacillus subtilis are an effective remediation strategy in contaminated soils, increasing plant tolerance to heavy metals, as reported in Brassica juncea (Ndeddy Aka and Babalola, 2016).
Soil affects inoculation efficiency and influences the rhizosphere microflora (Egamberdiyeva, 2007; Souza et al., 2015). After inoculation, cell numbers will undergo a rapid decline, especially on unsterilized soils. In autoclaved soils, because there is no competition with other microorganisms, inoculants remain in high cell densities for many weeks. In nonsterile soils, because there is great competition with the resident soil microbiome and predation by protozoa and nematodes, inoculants populations will decline rapidly, until the population reach an equilibrium (Martínez-Viveros et al., 2010; Varma et al., 2019). For this reason, research work screening PGPM must be carried out in nonsterile soils, since the possibility of testing the competition efficiency of the inoculated microorganisms against those already native to the soil will be greater.
Rainfall is the major water source for growing crops in many parts of the world (Enebe and Babalola, 2018; Danish et al., 2020). Water availability, lack (drought) or excess (flood), can result in abiotic stress, limiting crop production (Enebe and Babalola, 2018; Ipek et al., 2019; Danish et al., 2020). PGPM can improve plant tolerance to drought stress (Fleming et al., 2019; Asghari et al., 2020), as Azotobacter chroococcum and Azospirillum brasilense in Mentha pulegium L. (Asghari et al., 2020), and Pseudomonas sp. and Azotobacter sp. in Cymbopogon citratus (Mirzaei et al., 2020) and Zea mays (Danish et al., 2020). Klebsiella variicola and Azospirillum sp. can improve flooding stress tolerance by adaptations, such as the formation of adventitious roots resulting from endogenous hormonal regulation, as reported in Glycine max (Kim et al., 2017) and Zea mays (Czarnes et al., 2020).
However, water stress can influence the plant-microbe interactions. Drought increases soil temperature, which can inhibit multiplication of beneficial microorganisms. Flooding reduces O2 availability in soil, restricting microorganisms that are not capable of anerobic respiration (Enebe and Babalola, 2018; Hartman and Tringe, 2019; Ipek et al., 2019). In addition, drought and flooding are stress that affect plant metabolism and photoassimilates production, interfering with production and composition of root exudates (Enebe and Babalola, 2018; Fleming et al., 2019; Hartman and Tringe, 2019; Danish et al., 2020). This will affect plant-microorganism interaction, which may inhibit the microorganism potential to promote plant growth. For this reason, it is important to know where the target plant species is usually adapted. Considering climate change, it is important to study how the excess and the lack of water would affect the interaction between plants and PGPM.
Light intensity influences plant metabolism, growth and production (Venturi and Keel, 2016). Light can interfere in plant-microorganisms interaction by modifying the amount and the chemical composition of root exudates (Venturi and Keel, 2016; Lopes et al., 2018b). Microbial inoculation demands carbohydrate allocation in exchange of nutrients delivered to plants. Beneficial microorganism inoculation can increase plant growth under limited light conditions, by increasing shade tolerance, as Kaistobacter sp. and Pseudomonas sp. in Ophiopogon japonicus and Lolium perenne (Fu et al., 2020).
However, under light-limited conditions (i.e., shade) the microbial root symbionts can create additive costs, inhibiting plant growth, as Glomus sp. Paraglomus sp. Rhizophagus sp. and Rhizobium in Phaseolus lunatus (Ballhorn et al., 2016). Aguilar-Chama and Guevara (2016) describe that a mycorrhizal inoculation had a positive effect on stem mass, root mass, and leaf nitrogen content in Datura stramonium, but only when light was not a limiting factor. This is because, under limited light conditions, photosynthesis is reduced, consequently, carbohydrate production is also reduced, turning mutualistic microbes into parasites (Ballhorn et al., 2016).
As previously explained, during screening for PGPM, light conditions commonly experienced by the targeted plant species must be taken into account. This is because light intensity can interfere with the PGPM efficiency. For example, Brachiaria brizantha is a tropical forage grass grown either under full sun, or under moderate shade, as when cultivated in silvipastoral systems. Therefore, Lopes et al. (2018b) report that they tested the efficiency of rhizobacteria in promoting growth of this forage grass under both full sun and moderate shade. According to Lopes et al. (2018b), the PGPM efficiency varied with the type of microorganism and light intensity. When the bacteria were inoculated individually, plants under full sun showed the highest growth with Pseudomonas fluorescens, while under moderate shade Burkholderia pyrrocinia was more efficient in promoting plant growth (Lopes et al., 2018b).
High and low temperatures potentially caused by climatic change may become a major threat to global agriculture, reducing crop production, with drastic economic results (Ipek et al., 2019; Mukhtar et al., 2020). Beneficial microorganism inoculation is efficient in enhancing plat growth and mitigating adverse stresses caused by extreme temperature, as Pseudomonas putida in Triticum sp. (Ali et al., 2011) and Bacillus cereus in Solanum lycopersicum (Mukhtar et al., 2020) under high temperature. Fernandez et al. (2012) related that under low temperature Burkholderia sp. increased tolerance to low temperature by modification of carbohydrate metabolism and increased plant yield in Vitis vinifera.
Extreme temperatures are recognized stress in agriculture, reducing seed germination, seedling growth, yield and altering plant metabolism (Ipek et al., 2019; Mukhtar et al., 2020). Temperature impacts morphological, biochemical and physiological attributes of plants, interfering with plant-PGPM interaction by changing root exudation composition (Ali et al., 2011; Meena et al., 2015; Ipek et al., 2019). Therefore, for PGPM to be able to withstand environmental transformations that crop plants are exposed, it is necessary to isolate these microorganisms from different rhizosphere environments, under diverse environmental conditions, such as prevalent high and low temperatures (Etesami, 2020). This is because rhizobacteria that persist under change temperatures have the ability of improving plant growth and productivity on these adverse environmental conditions (Meena et al., 2015).
The use of PGPM inoculants is a potential tool to increase plant growth and crop yields in a more environmentally sustainable way, by reducing the need of chemical inputs and providing tolerance against abiotic stresses. The reviewed literature has shown that inoculation method and abiotic factors, associated climatic conditions, are essential for the success of the interaction between PGPM and plants.
Inoculation methods have a great effect on the establishment and persistence of microorganism in the rhizosphere and on their growth-promoting effects on host plants. Decision on which inoculation method to use must be based on knowledge of the plant growth stages, if they are produces the allelochemicals with negative allelopathic effects, and its morphological characteristics. For species, that exude more allelopathic composts in germination, and these compounds are reduced throughout the development of the plant, the ideal inoculation method would be in the root or soil. On the other hand, in species with pivoting roots, the cell wall of the roots is denser, this could hinder the adhesion and colonization of PGPM. In these species, the ideal inoculation method would be in seeds.
Climatic changes influence the abiotic factors, resulting in plant stresses, besides affecting the success of its interaction with PGPM. Abiotic factors such as soil (nutrient, heavy metal, pH, and salinity), water availability, light intensity, and temperature can influence the plant-microbe interactions, because they alter plant metabolism, root exudates composition and rhizosphere biology. This is because, if there is a change in the exudative pattern of the plant, the same isolate and the same plant genotype may interact differently.
In screening studies, it is recommended to use non-sterile soil, test different inoculation methods and different abiotic stresses, and even select microorganisms from plants under abiotic stress. Therefore, in screening experiments for the selection of PGPM it is necessary to know about the environmental requirements of the target plant and the environmental conditions on which it will be cultivated. In addition, as the PGPM depend on the exudates provided by the plant, this causes a specific relationship, so, the ideal scenario is using, in selection tests, microorganisms from the rhizosphere site-specific plant associations.
In this respect, it is worth noting that in addition to auxin, ACC-deaminase, nitrogen fixation capacity, phosphate solubilization, and siderophore production, we suggest that the ability of the microorganisms to produce catalase should also be considered in screening tests. Microbial production of catalase is a simple test, low-cost and indicates the potential of the microorganism to reduce oxidative stress in the plant.
The future perspectives of microbial applications must include the improvement of screening techniques, such as the quantification of antioxidant enzymes and others that can benefit plant development. Also, screening tests should be carried out under different temperatures, soil nutrient, water and pH. In addition, it is important to evaluate the ability of PGPM to promote plant growth under contrasting light intensities. Future studies must include improved methods for inoculation, detailing the rhizosphere microbiome of each species studied, and the interaction between plants and PGPM. In the future, it is expected the development of software to indicate the ideal PGPM to benefit a specific plant species, the best inoculation method and its action under different abiotic factors. Thus, there will be a more efficient selection of microorganisms, resulting in increased plant growth, leading to agricultural sustainability and environmental preservation.
ML designed the project. ML, MD-F, and EG wrote the manuscript. ML and MD-F edited the manuscript. All authors contributed to the article and approved the submitted version.
The authors declare that the research was conducted in the absence of any commercial or financial relationships that could be construed as a potential conflict of interest.
We thank Museu Paraense Emílio Goeldi—MPEG/MCTIC/CNPq (301341/2020-6).
Abhilash, P. C., Dubey, R. K., Tripathi, V., Gupta, V. K., and Singh, H. B. (2016). Plant growth-promoting microorganisms for environmental sustainability. Trends Biotechn. 34, 847–850. doi: 10.1016/j.tibtech.2016.05.005
Afzal, M., Khan, S., Iqbal, S., Sajjad, M., Qaiser, M., and Khan, M. (2013). Inoculation method affects colonization and activity of Burkholderia phytofirmans PsJN during phytoremediation of diesel-contaminated soil. Int. Biodeterior. Biodegrad. 85, 331–336. doi: 10.1016/j.ibiod.2013.08.022
Aguilar-Chama, A., and Guevara, R. (2016). Resource allocation in an annual herb: effects of light, mycorrhizal fungi, and defoliation. Acta Oecol. 71, 1–7. doi: 10.1016/j.actao.2015.12.011
Ahemad, M., and Kibret, M. (2014). Mechanisms and applications of plant growth promoting rhizobacteria: current perspective. J. King Saud Univ. 26, 1–20. doi: 10.1016/j.jksus.2013.05.001
Ali, S. K. Z., Sandhya, V., Grover, M., Linga, V. R., and Bandi, V. (2011). Effect of inoculation with a thermotolerant plant growth promoting Pseudomonas putida strain AKMP7 on growth of wheat (Triticum spp.) under heat stress. J. Plant Interact. 6, 239–246. doi: 10.1080/17429145.2010.545147
Ammor, M. S., Michaelidis, C., and Nychas, G. J. (2008). Insights into the role of quorum sensing in food spoilage. J. Food Prot. 71, 1510–1525. doi: 10.4315/0362-028X-71.7.1510
Arora, N. K., Fatima, T., Mishra, I., and Verma, S. (2020). “Microbe-based inoculants: role in next green revolution,” in Environmental Concerns and Sustainable Development, eds V. Shukla and N. Kumar (Singapore: Springer), 191–245. doi: 10.1007/978-981-13-6358-0_9
Asghari, B., Khademian, R., and Sedaghati, B. (2020). Plant growth promoting rhizobacteria (PGPR) confer drought resistance and stimulate biosynthesis of secondary metabolites in pennyroyal (Mentha pulegium L.) under water shortage condition. Sci. Hort. 263, 1–10. doi: 10.1016/j.scienta.2019.109132
Bakhshandeh, E., Gholamhosseini, M., Yaghoubian, Y., and Pirdashti, H. (2020). Plant growth promoting microorganisms can improve germination, seedling growth and potassium uptake of soybean under drought and salt stress. Plant Growth Regul. 90, 123–136. doi: 10.1007/s10725-019-00556-5
Ballhorn, D. J., Schädler, M., Elias, J. D., Millar, J. A., and Kautz, S. (2016). Friend or foe—light availability determines the relationship between mycorrhizal fungi, rhizobia and lima bean (Phaseolus lunatus L.). PLoS ONE 11:e0154116. doi: 10.1371/journal.pone.0154116
Bhat, M. A., Rasool, R., and Ramzan, S. (2019). Plant growth promoting rhizobacteria (PGPR) for sustainable and eco-friendly agriculture. Acta Sci. Agric. 3, 23–25.
Bhattacharjya, S., and Chandra, R. (2013). Effect of inoculation methods of Mesorhizobium ciceri and PGPR in chickpea (Cicer areietinum L.) on symbiotic traits, yields, nutrient uptake and soil properties. Legum. Res. 36, 331–337.
Cely, M. V. T., Siviero, M. A., Emiliano, J., Spago, F. R., Freitas, V. F., Barazetti, A. R., et al. (2016). Inoculation of Schizolobium parahyba with mycorrhizal fungi and plant growth-promoting rhizobacteria increases wood yield under field conditions. Front. Plant Sci. 7:1708. doi: 10.3389/fpls.2016.01708
Czarnes, S., Mercier, P.-E., Lemoine, D. G., Hamzaoui, J., and Legendre, L. (2020). Impact of soil water content on maize responses to the plant growth-promoting rhizobacterium Azospirillum lipoferum CRT1. J. Agro. Crop Sci. 206, 1–12. doi: 10.1111/jac.12399
Danish, S., Hye, M. Z., Hussain, S., Riaz, M., and 2 Qayyum, M. F. (2020). Mitigation of drought stress in maize through inoculation with drought tolerant acc deaminase containing pgpr under axenic conditions. Pak. J. Bot. 52, 49–60. doi: 10.30848/PJB2020-1(7)
Doornbos, R. F., Loon, L. C., and Bakker, P. A. H. M. (2012). Impact of root exudates and plant defense signaling on bacterial communities in the rhizosphere. A review. Agron. Sustain. Dev. 32, 227–243. doi: 10.1007/s13593-011-0028-y
Dutta, J., and Bora, U. (2019). “Role of PGPR for alleviating aluminum toxicity in acidic soil,” in Plant Growth Promoting Rhizobacteria for Sustainable Stress Management. Microorganisms for Sustainability, Vol 12, eds R. Sayyed, N. Arora and M. Reddy (Singapore: Springer). doi: 10.1007/978-981-13-6536-2_14
Egamberdiyeva, D. (2007). The effect of plant growth promoting bacteria on growth and nutrient uptake of maize in two different soils. Appl. Soil Ecol. 36, 184–189. doi: 10.1016/j.apsoil.2007.02.005
El-Sayed, S. Y. S., and Hagab, R. H. (2020). Effect of organic acids and plant growth promoting rhizobacteria (PGPR) on biochemical content and productivity of wheat under saline soil conditions. Middle East J. Agric. Res. 9:2, 227–242. doi: 10.36632/mejar/2020.9.2.20
Enebe, M. C., and Babalola, O. O. (2018). The influence of plant growth-promoting rhizobacteria in plant tolerance to abiotic stress: a survival strategy. ssAppl. Microbiol. Biotechnol. 102, 7821–7835. doi: 10.1007/s00253-018-9214-z
Etesami, H. (2020). Plant–microbe interactions in plants and stress tolerance. Plant Life Under Changing Environ. 2020, 355–396. doi: 10.1016/B978-0-12-818204-8.00018-7
Fernandez, O., Theocharis, A., Bordiec, S., Feil, R., Jacquens, L., and Clément, C. (2012). Burkholderia phytofirmans PsJN acclimates grapevine to cold by modulating carbohydrate metabolism. MPMI 5, 496–504. doi: 10.1094/MPMI-09-11-0245
Fleming, T. R., Fleming, C. C., Levy, C. C. B., Repiso, C., Hennequart, F., Nolasco, J. B., et al. (2019). Biostimulants enhance growth and drought tolerance in Arabidopsis thaliana and exhibit chemical priming action. Ann. Appl. Biol. 174, 1–13. doi: 10.1111/aab.12482
Fu, J., Luo, Y., Sun, P., Gao, J., Zhao, D., Yang, P., et al. (2020). Effects of shade stress on turfgrasses morphophysiology and rhizosphere soil bacterial communities. BMC Plant Biol. 20:92. doi: 10.1186/s12870-020-2300-2
Gouda, S., Kerry, R. G., Das, G., Paramithiotis, S., Shin, H., and Patra, J. K. (2018). Revitalization of plant growth promoting rhizobacteria for sustainable development in agriculture. Microbiol. Res. 206, 131–140. doi: 10.1016/j.micres.2017.08.016
Hartman, K., and Tringe, S. G. (2019). Interactions between plants and soil shaping the root microbiome under abiotic stress. Biochem. J. 476, 2705–2724. doi: 10.1042/BCJ20180615
Hashem, A., Tabassum, B., and Allah, E. F. A. (2019). Bacillus subtilis: a plant-growth promoting rhizobacterium that also impacts biotic stress. Saudi J. Biol. Sci. 26, 1291–1297. doi: 10.1016/j.sjbs.2019.05.004
Hernández-Montiel, L. G., Chiquito-Contreras, C. J., Murillo-Amador, B., Vidal-Hernández, L., Quiñones-Aguilar, E. E., and Chiquito-Contreras, R. G. (2017). Efficiency of two inoculation methods of Pseudomonas putida on growth and yield of tomato plants. Soil Sci. Plant Nutr. 17, 1003–1012. doi: 10.4067/S0718-95162017000400012
Ipek, M., Arikan, S, Pirlak, L., and Eşitken, A. (2019). “Sustainability of crop production by PGPR.” in Plant Growth Promoting Rhizobacteria for Agricultural Sustainability, eds A. Kumar and V. Meena (Singapore: Springer), 293–314. doi: 10.1007/978-981-13-7553-8_15
Khan, N., Bano, A., Ali, S., and Babar, M.d. A. (2020). Crosstalk amongst phytohormones from planta and PGPR under biotic and abiotic stresses. Plant Growth Regul. 90, 189–203. doi: 10.1007/s10725-020-00571-x
Khan, N., Bano, A., Rahman, M. A., Guo, J., Kang, Z., Babar, M.d., et al. (2019). Comparative physiological and metabolic analysis reveals a complex mechanism involved in drought tolerance in chickpea (Cicer arietinum L.) induced by PGPR and PGRs. Sci. Rep. 9:2097. doi: 10.1038/s41598-019-38702-8
Khoshru, B., Mitra, D., Khoshmanzar, E., Myo, E. M., Uniyal, N., Mahakur, B., et al. (2020). Current scenario and future prospects of plant growth-promoting rhizobacteria: an economic valuable resource for the agriculture revival under stressful conditions. J. Plant Nutr. 43, 3062–3092. doi: 10.1080/01904167.2020.1799004
Kim, A. Y., Shahzad, R., Kang, S. M., Seo, C. W., Park, Y. G., Park, H. J., and Lee, I. J. (2017). IAA-producing Klebsiella variicola AY13 reprograms soybean growth during flooding stress. J. Crop Sci. Biotech. 20, 235–242. doi: 10.1007/s12892-017-0041-0
Kloepper, J. W., Gutiérrez-Estrad, A., and McInroy, J. A. (2007). Photoperiod regulates elicitation of growth promotion but not induced resistance by plant growth-promoting rhizobacteria. Can. J. Microbiol. 53, 159–167. doi: 10.1139/w06-114
Lopes, M. J., Filho, M. B. D., Reis Castro, T. H., Filippi, M. C. C., and Silva, G. B. (2018a). Effect of Pseudomonas fluorescens and Burkholderia pyrrocinia on the growth improvement and physiological responses in Brachiaria brizantha. Am. J. Plant Sci. 9, 250–265. doi: 10.4236/ajps.2018.92021
Lopes, M. J., Filho, M. B. D., Reis Castro, T. H., and Silva, G. B. (2018b). Light and plant growth-promoting rhizobacteria effects on Brachiaria brizantha growth and phenotypic plasticity to shade. Grass. Forage. Sci. 73, 493–499. doi: 10.1111/gfs.12336
Mahmood, I., Rizvi, R., Sumbul, A., and Ansari, R. A. (2019). “Potential role of plant growth promoting rhizobacteria in alleviation of biotic stress,” in Plant Health Under Biotic Stress, eds R. A. Ansari and I. Mahmood (Singapore: Springer), 177–188. doi: 10.1007/978-981-13-6040-4_9
Martínez-Viveros, O., Jorquera, M. A., Crowley, D. E., Gajardo, G., and Mora, M. L. (2010). Mechanisms and practical considerations involved in plant growth promotion by rhizobacteria. J. Soil Sci. Plant Nutr. 10, 293–319. doi: 10.4067/S0718-95162010000100006
Mathimaran, N., Jegan, S., Thimmegowda, M. N., Prabavathy, V. R., Yuvaraj, P., Kathiravan, R., et al. (2020). Intercropping transplanted pigeon pea with finger millet: arbuscular mycorrhizal fungi and plant growth promoting rhizobacteria boost yield while reducing fertilizer input. Front. Sustain. Food Syst. 4:88. doi: 10.3389/fsufs.2020.00088
Meena, R. K., Singh, R. K., Singh, N. P., Meena, S. K., and Meena, V. S. (2015). Isolation of low temperature surviving plant growth – promoting rhizobacteria (PGPR) from pea (Pisum sativum L.) and documentation of their plant growth promoting traits. Biocatal. Agric. Biotechnol. 4, 806–811. doi: 10.1016/j.bcab.2015.08.006
Mimmo, T., Pii, Y., Valentinuzzi, F., Astolfi, S., Lehto, N., Robinson, B., et al. (2018). Nutrient availability in the rhizosphere: a review. Acta Hortic. 1217, 13–28. doi: 10.17660/ActaHortic.2018.1217.2
Mirzaei, M., Moghadam, A. L., Hakimi, L., and Danaee, E. (2020). Plant growth promoting rhizobacteria (PGPR) improve plant growth, antioxidant capacity, and essential oil properties of lemongrass (Cymbopogon citratus) under water stress. Iran. J. Plant Physiol. 10, 3155–3166. doi: 10.22034/IJPP.2020.672574
Moeller, R., Horneck, G., Facius, R., and Stackebrandt, E. (2005). Role of pigmentation in protecting Bacillus sp. endospores against environmental UV radiation. FEMS Microbiol. Ecol. 51, 231–236. doi: 10.1016/j.femsec.2004.08.008
Msimbira, L. A., and Smith, D. L. (2020). The roles of plant growth promoting microbes in enhancing plant tolerance to acidity and alkalinity stresses. Front. Sustain. Food Syst. 4, 1–14. doi: 10.3389/fsufs.2020.00106
Mukhtar, T., Rehman, S., Smith, D., Sultan, T., Seleiman, M. F., Alsadon, A. A., et al. (2020). Mitigation of heat stress in Solanum lycopersicum L. by ACC-deaminase and exopolysaccharide producing Bacillus cereus: effects on biochemical profiling. Sustainability 12:2159. doi: 10.3390/su12062159
Ndeddy Aka, R. J., and Babalola, O. O. (2016). Effect of bacterial inoculation of strains of Pseudomonas aeruginosa, Alcaligenes feacalis and Bacillus subtilis on germination, growth and heavy metal (Cd, Cr, and Ni) uptake of Brassica juncea. Int. J. Phytoremed. 18, 200–209. doi: 10.1080/15226514.2015.1073671
Oosten, M. J. V., Pepe, O., Pascale, S., Silletti, S., and Maggio, A. (2017). The role of biostimulants and bioeffectors as alleviators of abiotic stress in crop plants. Chem. Biol. Technol. Agric. 4:5. doi: 10.1186/s40538-017-0089-5
Prisa, D. (2020). Optimised fertilisation with zeolitites containing plant growth promoting rhizobacteria (PGPR) in Ranunculus asiaticus. GSC Biol. Pharmaceut. Sci. 10, 96–102. doi: 10.30574/gscbps.2020.10.1.0011
Romeiro, R. S. (2007). Controle biológico de doenças de plantas: procedimentos. Viçosa, MG: Universidade Federal de Viçosa. 172p.
Salwan, R., Sharma, A., and Sharma, V. (2019). Microbes mediated plant stress tolerance in saline agricultural ecosystem. Plant Soil. 1–22. doi: 10.1007/s11104-019-04202-x
Samaddar, S., Chatterjee, P., Choudhury, A. R., Ahmed, S., and Sa, T. (2019). Interactions between Pseudomonas spp. and their role in improving the red pepper plant growth under salinity stress. Microbiol. Res. 219, 66–73. doi: 10.1016/j.micres.2018.11.005
Saravanakumar, D. (2012). “Rhizobacterial ACC deaminase in plant growth and stress amelioration,” in Bacteria in Agrobiology: Stress Management, eds D. Maheshwari (Berlin, Heidelberg: Springer), 187–204. doi: 10.1007/978-3-662-45795-5_9
Saravanakumar, D., Kavino, M., Raguchander, T., Subbian, P., and Samiyappan, R. (2011). Plant growth promoting bacteria enhance water stress resistance in green gram plants. Acta Physiol. Plant. 33, 203–209. doi: 10.1007/s11738-010-0539-1
Sharma, P., Jha, A. B., Dubey, R. S., and Pessarakli, M. (2012). Reactive oxygen species, oxidative damage, and antioxidative defense mechanism in plants under stressful conditions. J. Bot. 2012, 1–26. doi: 10.1155/2012/217037
Souza, R., de Ambrosini, A., and Passaglia, L. M. P. (2015). Plant growth-promoting bacteria as inoculants in agricultural soils. Genet. Mol. Biol. 38, 401–419. doi: 10.1590/S1415-475738420150053
Strigul, N. S., and Kravchenko, L. V. (2006). Mathematical modeling of PGPR inoculation into the rhizosphere. Environ. Model. Softw. 21, 1158–1171. doi: 10.1016/j.envsoft.2005.06.003
Subramanian, P., Kim, K., Krishnamoorthy, R., Mageswari, A., Selvakumar, G., et al. (2016). Cold stress tolerance in psychrotolerant soil bacteria and their conferred chilling resistance in tomato (Solanum lycopersicum Mill.) under low temperatures. PLOS ONE. 11:e0161592. doi: 10.1371/journal.pone.0161592
Taiz, L., and Zeiger, E. (2017). Fisiologia e Desenvolvimento Vegetal, 6th Edn. Porto Alegre: Artmed, 888p.
Ullah, M. A., Mahmood, I. A., Ali, A., Nawaz, Q., Sultan, T., and Zaman, B. U. (2017). Effect of inoculation methods of biozote-max (plant growth promoting rhizobacteria-pgpr) on growth and yield of rice under naturally salt-affected soil. Res. Plant Bio. 7, 24–26. doi: 10.25081/ripb.2017.v7.3602
Upadhyay, S. K., Singh, J. S., Saxena, A. K., and Singh, D. P. (2011). Impact of PGPR inoculation on growth and antioxidant status of wheat under saline conditions. Plant Bio. 14, 605–611. doi: 10.1111/j.1438-8677.2011.00533.x
Varma, A., Tripathi, S., and Prasad, R. (Eds.). (2019). Plant Biotic Interactions, 2019. Cham: Springer. doi: 10.1007/978-3-030-26657-8
Venturi, V., and Keel, C. (2016). Signaling in the Rhizosphere. Trends Plant Sci. 21, 187–198. doi: 10.1016/j.tplants.2016.01.005
Zerrouk, I. Z., Rahmoune, B., Khelifi, L., Mounir, H., Baluska, F., and Ludwig-Müller, J. (2019). Algerian Sahara PGPR confers maize root tolerance to salt and aluminum toxicity via ACC deaminase and IAA. Acta Physiol Plant. 41, 1–10. doi: 10.1007/s11738-019-2881-2
Keywords: PGPM, PGPR, soil, light, pH, temperature, water
Citation: Lopes MJS, Dias-Filho MB and Gurgel ESC (2021) Successful Plant Growth-Promoting Microbes: Inoculation Methods and Abiotic Factors. Front. Sustain. Food Syst. 5:606454. doi: 10.3389/fsufs.2021.606454
Received: 15 September 2020; Accepted: 04 February 2021;
Published: 25 February 2021.
Edited by:
Duraisamy Saravanakumar, The University of the West Indies St. Augustine, Trinidad and TobagoReviewed by:
Shalini Tiwari, National Botanical Research Institute (CSIR), IndiaCopyright © 2021 Lopes, Dias-Filho and Gurgel. This is an open-access article distributed under the terms of the Creative Commons Attribution License (CC BY). The use, distribution or reproduction in other forums is permitted, provided the original author(s) and the copyright owner(s) are credited and that the original publication in this journal is cited, in accordance with accepted academic practice. No use, distribution or reproduction is permitted which does not comply with these terms.
*Correspondence: Monyck Jeane dos Santos Lopes, bW9ueWNrbG9wZXNAbXVzZXUtZ29lbGRpLmJy
Disclaimer: All claims expressed in this article are solely those of the authors and do not necessarily represent those of their affiliated organizations, or those of the publisher, the editors and the reviewers. Any product that may be evaluated in this article or claim that may be made by its manufacturer is not guaranteed or endorsed by the publisher.
Research integrity at Frontiers
Learn more about the work of our research integrity team to safeguard the quality of each article we publish.