- 1Department of Chemistry, Tshwane University of Technology, Pretoria, South Africa
- 2Department of Crop Sciences, Tshwane University of Technology, Pretoria, South Africa
The symbiotic interaction between rhizobia and legumes that leads to nodule formation is a complex chemical conversation involving plant release of nod-gene inducing signal molecules and bacterial secretion of lipo-chito-oligossacharide nodulation factors. During this process, the rhizobia and their legume hosts can synthesize and release various phytohormones, such as IAA, lumichrome, riboflavin, lipo-chito-oligossacharide Nod factors, rhizobitoxine, gibberellins, jasmonates, brassinosteroids, ethylene, cytokinins and the enzyme 1-aminocyclopropane-1-carboxylate (ACC) deaminase that can directly or indirectly stimulate plant growth. Whereas these attributes may promote plant adaptation to various edapho-climatic stresses including the limitations in nutrient elements required for plant growth promotion, tapping their full potential requires understanding of the mechanisms involved in their action. In this regard, several N2-fixing rhizobia have been cited for plant growth promotion by solubilizing soil-bound P in the rhizosphere via the synthesis of gluconic acid under the control of pyrroloquinoline quinone (PQQ) genes, just as others are known for the synthesis and release of siderophores for enhanced Fe nutrition in plants, the chelation of heavy metals in the reclamation of contaminated soils, and as biocontrol agents against diseases. Some of these metabolites can enhance plant growth via the suppression of the deleterious effects of other antagonistic molecules, as exemplified by the reduction in the deleterious effect of ethylene by ACC deaminase synthesized by rhizobia. Although symbiotic rhizobia are capable of triggering biological outcomes with direct and indirect effects on plant mineral nutrition, insect pest and disease resistance, a greater understanding of the mechanisms involved remains a challenge in tapping the maximum benefits of the molecules involved. Rather than the effects of individual rhizobial or plant metabolites however, a deeper understanding of their synergistic interactions may be useful in alleviating the effects of multiple plant stress factors for increased growth and productivity.
Introduction
Nitrogen is an essential component of all amino acids and nucleic acids, thus making it an important plant nutrient element. Although the atmosphere consists of 78.1% N2 gas, plants cannot use it unless it is converted into a usable form (Ferguson et al., 2010). Biological N2 fixation (BNF) is a free source of N that can be exploited by resource-poor farmers for increased crop yields (Giller and Cadisch, 1995), making it one of the most important microbiological processes on earth; globally, ~33–46 Tg of N year−1 is contributed by the legume-rhizobia symbiosis (Herridge, 2008). So far, 21 bacterial genera have been identified as nodule-forming microsymbionts (Wang et al., 2019a). These microsymbionts are distributed among major bacterial genera of the alpha-proteobacteria such as Rhizobium, Bradyrhizobium, Azorhizobium, Mesorhizobium, Ensifer (Sinorhizobium), Neorhizobium, Pararhizobium, and Allorhizobium, collectively termed “rhizobia”, which can form symbiotic associations with diverse legumes. Similarly, species of the beta-rhizobia Cupriavidus, Paraburkholderia, and Trinickia can also form symbiotic relationships with members of the Papilionoideae and Caesalpinioideae (LPWG, 2017; Sprent et al., 2017).
The formation of root nodules in symbiotic legumes involves a complex molecular signaling between the legume host and the rhizobial microsymbiont (Oldroyd et al., 2011). At the onset of nodule organogenesis, the rhizodeposition of flavonoid compounds from legume seed coats or root exudates induce the synthesis of NodD proteins which activate the transcription of genes needed to produce rhizobial lipo-chito oligosaccharide Nod factors (Andrews and Andrews, 2017). Upon perception of Nod factors by the plant, nodule organogenesis commences via the curling of the root hair tips leading to formation of an infection thread, mitotic division of root cortical cells, and consequently the formation of a nodule primordium (Oldroyd et al., 2011). Rhizobia penetrate the cortical cells via the infection thread and are eventually released into the nodule primordium housed in host-derived cells known a symbiosomes (Okubo et al., 2012). Rhizobia undergo differentiation into N2-fixing bacteroids which carry out the conversion of atmospheric N2 into ammonia in a reaction catalyzed by the nitrogenase enzyme (Udvardi and Kahn, 1992). Whereas many rhizobia invade roots via the infection thread, others utilize either “crack entry” or root epidermal cells as points of infection (González-Sama et al., 2004; Ardley et al., 2013; Bianco, 2014). When N2 fixation commences, the bacteroids in root nodules supply the host legume with fixed N while receiving C compounds from host photosynthesis (Udvardi and Kahn, 1992).
Besides the provision of symbiotic N, some rhizobia also exhibit physiologically desirable traits such as the production of plant growth-promoting phytohormones, which include indole-3-acetic acids (IAA), cytokinins, gibberellins, riboflavin, lumichrome, Nod factors, etc (Table 1, Figure 1), all of which play diverse roles in enhancing plant growth and productivity (Dakora and Phillips, 2002; Berg, 2009). Thus, rhizobia confer several advantages on plants in addition to the provision of N from symbiotic interactions. The beneficial effects of rhizobia are mediated by the production of diverse metabolites and enzymes that are directly or indirectly elicited by rhizobia and plants during nodule formation (Table 1). Of the plant growth-promoting molecules, the secretion of IAA, 1-aminocyclopropane-1-carboxylate (ACC) deaminase, lumichrome, riboflavin and protons for phosphate solubilization have been suggested as important PGPR mechanisms underlying plant growth promotion (Figure 1) (Li et al., 2000; Matiru and Dakora, 2005; Gravel et al., 2007; Bal et al., 2013; Dakora et al., 2015). ACC deaminase is an enzyme that can decrease deleterious amounts of ethylene in higher plants, leading to increased productivity. Lumichrome and riboflavin are novel molecules from rhizobial exudates that are known to stimulate plant growth. On the other hand, IAA is an important member of the auxin family that is responsible for controlling plant physiological processes, including cell enlargement and division, tissue differentiation and light/gravity responses (Teale et al., 2006; Shokri and Emtiazi, 2010) (Table 1).
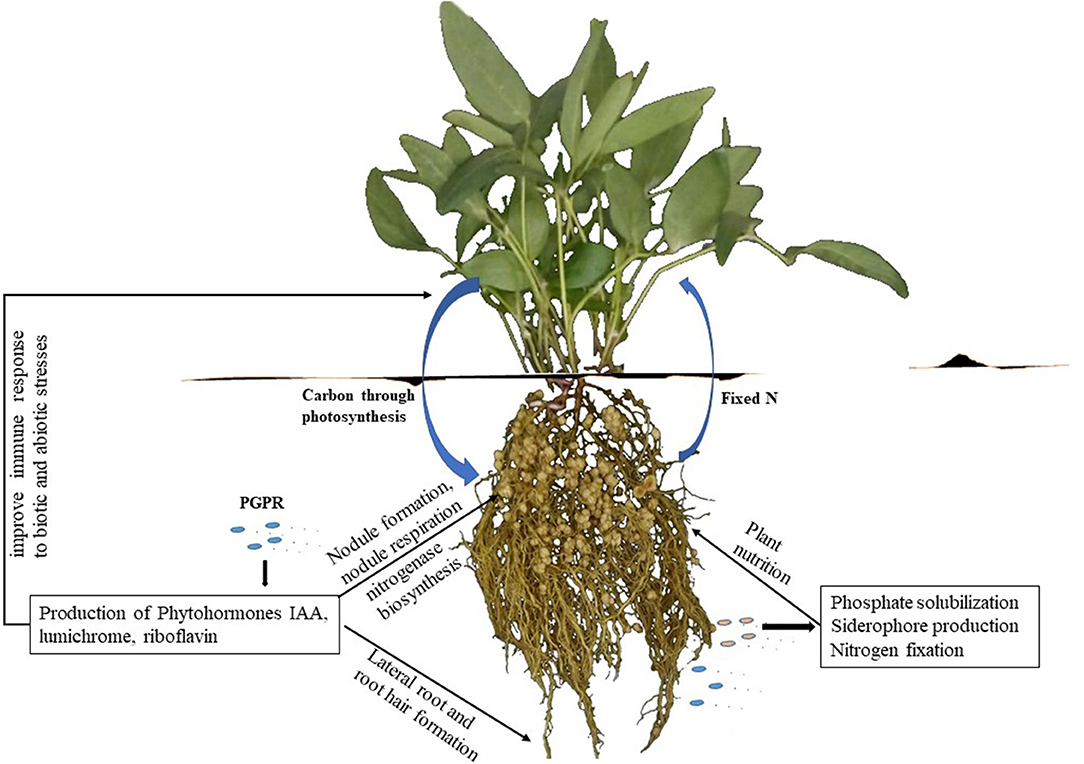
Figure 1. Plant growth promoting activities in the rhizosphere. Microsymbionts are activated by rhizodeposition of carbon and other signal molecules by the plant. After establishment of the PGPR in the rhizosphere, they promote plant growth by releasing growth substances and alleviating the effects of biotic stresses.
Plant growth promoting rhizobacteria (PGPR) comprise a diverse group of bacteria that present growth benefits to plants through several mechanisms. The rhizosphere soil of plants tends to contain PGPR that are capable of releasing protons to solubilize soil-bound phosphorus for plant use, and are usually referred to as phosphorus-solubilizing bacteria (PSB) (Chaiharn and Lumyong, 2009). The detailed mechanisms by which microsymbionts alter plant growth have remained elusive. Nevertheless, efforts at identifying highly effective rhizobial strains that combine adaptation to their environment with plant-growth promoting traits could improve plant growth and inoculation response under field conditions. This review addresses the roles played by the secretion of siderophore, IAA, ACC deaminase, lumichrome, riboflavin and protons for P solubilization in plant growth promotion, especially during the legume-rhizobia symbiosis. The secretion of these molecules by non-rhizobial plant growth promoting bacteria leading to increased plant development is also discussed.
RHIZOBIAL SYMBIONTS AS PROMOTERS OF LEGUME PLANT GROWTH
Legumes that harbor efficient rhizobia in their root or stem nodules often meet their N requirements from N2 fixation (Belane et al., 2011; Mohale et al., 2014). It is this symbiotic trait that gives legumes a superior survival advantage over their non-legume counterparts in N-depleted soils where other plant growth requirements are optimal. Nodulation and N2 fixation in legumes are an interactive process which involve the action of rhizobial Nod factors; and during the process, some rhizobia may produce phytohormones such as IAA, gibberellic acid and cytokinins which present plant growth promoting effects (Bottini et al., 1989; Hayashi et al., 2014) (Table 1). Aside serving as signal molecules in the early stages of nodule formation, Nod factors isolated from Rhizobium leguminosarum were also found to increase seed germination, nodulation and plant growth in pea and vetch, as well as pod yield in pea (Kidaj et al., 2012). Similarly, Nod factors from Bradyrhizobium japonicum strain 532C increased germination and early plant growth in soybean and other non-leguminous crops; moreover, the parent culture of Bradyrhizobium japonicum strain 532C, but not its mutant deficient in Nod factor synthesis, was also found to induce similar growth effects on plants (Prithiviraj et al., 2003). These observations clearly stress the multiple roles of rhizobial Nod factors as both signal molecules for nodulation and plant growth promoters in diverse crops. On the other hand, an IAA overproducing mutant of Sinorhizobium meliloti increased nodulation in Medicago sp. when compared to the parent strain, a report that stresses the involvement of this auxin in nodule formation aside its role in plant growth enhancement (Pii et al., 2007). Rhizobia can also increase nutrient availability in the root zone, especially N and P (Chebotar et al., 2001; Argaw, 2012), produce molecules that inhibit pathogens (Tavares et al., 2018), as well as alter rhizosphere chemistry involving the regulation of ethylene levels (Nascimento et al., 2018). Moreover, aside the growth promoting effects of cytokinin from either plant or bacterial origin, they are also involved in the nodulation process of legumes as well as plant response to pathogens (Giron et al., 2013; Gauthier-Coles et al., 2019). In pea plants, deficiencies in gibberellins and brassinosteroids were associated with impaired nodulation, pointing to their significant role in the symbiotic process and subsequent plant growth (Ferguson et al., 2005; Foo et al., 2016). The significant roles of the legume-rhizobia symbiosis in enhancing plant growth and productivity has been demonstrated in several legumes including soybean (Glycine max), mung bean (Vigna radiata), chickpea (Cicer aerietinum), common bean (Phaseolus vulgaris), cowpea (Vigna unguiculata), Bambara groundnut (Vigna subterranea) and Kersting's groundnut (Dashti et al., 1998; Shaharoona et al., 2006; Chihaoui et al., 2015; Gyogluu et al., 2018; Mohammed et al., 2018; Abdiev et al., 2019; Dabo et al., 2019; Ibny et al., 2019). However, a major limitation to tapping the maximum benefit of the symbiosis is related to its susceptibility to diverse environmental stress factors. For example, even when rhizobial strains exhibit high N2-fixing efficiency, their wider utilization in the field can be unpredictable, further hampering their adoption (Ulzen et al., 2016). Thus, tapping the maximum benefit of rhizobia for improved plant performance will require selection and/or engineering strains with multiple adaptive and plant growth promoting traits.
RHIZOBIAL PRODUCTION OF IAA AND N2 FIXATION IN LEGUMES
Indole acetic acid (IAA) is an important member of the auxin family responsible for controlling plant physiological processes, including cell division and enlargement, tissue differentiation, and light/gravity response (Teale et al., 2006; Shokri and Emtiazi, 2010). Rhizobia together with other bacteria, fungi and algae are producers of auxins, especially IAA (Shokri and Emtiazi, 2010), a common by-product of L-tryptophan metabolism in several microorganisms, including rhizobia (Datta and Basu, 2000; Ghosh and Basu, 2006; Mandal et al., 2007). The level of IAA production can vary among rhizobial isolates; and endogenous phenolic acids such as protocatechuic acid, 4-hydroxybenzaldehyde and p-coumaric acid in root nodules could increase IAA synthesis by rhizobial isolates from Vigna mungo (Mandal et al., 2009). Microbes and plants can mutualistically benefit from each other, with the microbes releasing plant growth-promoting substances in return for photosynthate from the plant. For example, the N-fixed by bacteroids in root nodules is transported to the aerial parts of plants in exchange for the carbon compounds from photosynthesis (Udvardi and Kahn, 1992; Kaiser et al., 2015).
IAA production by cowpea-nodulating rhizobia from South Africa and Mozambique differed, with about 80% of the rhizobial symbionts producing from 0.64 to 56.46 μg/ml of IAA (Dabo et al., 2019). Similar variations in the levels of IAA synthesis were also reported for rhizobial symbionts of Bambara groundnut in South African and Malian soils (Ibny et al., 2019). However, there is so far, no assessment of the relationship between rhizobial secretion of IAA and plant growth and/or grain yield. Nevertheless, Ali et al. (2008) showed that exogenous supply of IAA increased nitrogenase activity and leghemoglobin concentration in root nodules just as an engineered high IAA producing rhizobial strain showed significantly higher nitrogenase activity than the parent strain (Defez et al., 2019). Outside the legume symbiosis, bacterial production of IAA also presents growth promoting effects on non-legumes. In wheat for instance, root growth was reduced in plants treated with mutants of Azospirillum brasilense exhibiting low IAA synthesis than the wild-type (Spaepen et al., 2008). Moreover, whereas IAA synthesis is known for its plant-growth promoting effects, the mechanism seems to involve its degradation by bacteria; and was earlier demonstrated in Arabidopsis thaliana where an IAA degradation mutant of Burkholderia phytofirmans PsJN failed to increase root elongation when compared to the wild-type in the presence of exogenous IAA supply (Zúñiga et al., 2013).
IAA production by rhizobia is controlled by specific genes, and the overproduction of this auxin has been reported in several bacterial mutants, including that of Ensifer (Sinorhizobium) meliloti RD64 when compared to its wild type (Defez et al., 2019). The fixJ gene which works as a regulator to switch on the nitrogen fixation genes was highly expressed at 42 days of inoculation in the mutant of Ensifer (Sinorhizobium) meliloti RD64 leading to abundant FixJ protein which is regulated by nifA, fixK1, and fixK genes (Defez et al., 2019; Alemneh et al., 2020). Additionally, the IAA-overproducing Ensifer meliloti RD64 also showed an enhanced expression of the fixNOQP1,2 operon genes (which code for haem-copper cbb3-type oxidases at 40 days after inoculation), with an upregulation of gltA, icd, and sucA genes (responsible for TCA cycle enzymes) in the bacteroids (Defez et al., 2019). The inoculation of soybean with IAA-producing bacteria also showed upregulation of the otsA gene, which encodes for trehalose 6-phosphate synthase, and plays a major role in nodule formation, nodule respiration and nitrogenase biosynthesis (Suárez et al., 2008; Bargaz et al., 2013). Moreover, IAA-producing rhizobia have also been shown to significantly increase free amino acids such as valine, alanine, aspartic acid and glutamic acids in host plants as well as photosynthetic products supplied to bacteroids in root nodules (Tsikou et al., 2013; Erice et al., 2014; Defez et al., 2019). These findings together suggest that IAA promotes an increase in nitrogen fixation via the upregulation of the genes involved in carbon transport to N2-fixing bacteroids (Fisher, 1994; Defez et al., 2019).
BACTERIAL SECRETION OF SIDEROPHORES ENHANCES FE NUTRITION, PROMOTES PHYTOREMEDIATION AND CONTROLS PLANT PATHOGENS
Beyond the reduction of N2 to NH3 for direct use by plants in the synthesis of macromolecules such as chlorophyll, Rubisco and nucleic acids, some rhizobial strains are known to improve the availability and uptake of iron via siderophore production. Iron is one of the essential elements required by plants for growth and is a component of critical macromolecules such as leghaemoglobin and nitrogenase, both required for the N2 fixation process (Paudyal et al., 2007). As a result, a deficiency in Fe limits the efficiency of N2 fixation through a reduction in nodule development and nitrogenase activity (Duhan et al., 1998; Stajković et al., 2011). Fe exists in soils as the divalent (Fe2+) and trivalent (Fe3+) cations, and its availability for plant uptake is governed by several factors including soil pH and the levels of other soil nutrients (Rajkumar et al., 2010). Whereas siderophores are known to have diverse chemical structures, most bacteria produce the catecholate-type while fungi together with some bacteria produce the hydroxamate-type siderophores (Carson et al., 2000; Schalk et al., 2011; Grobelak and Hiller, 2017). For example, bacteria isolated from the rhizoplane of Arabidopsis thaliana were shown to produce both catecholate and hydroxamate siderophores, which subsequently improved plant growth and phytoremediation by decreasing metal toxicity to plants (Grobelak and Hiller, 2017). Siderophores have a high affinity for Fe3+ where soil Fe is low and can reduce it to Fe2+ for uptake and utilization by legumes (Gopalakrishnan et al., 2015; Lebrazi and Fikri-Benbrahim, 2018). Identifying siderophore-producing rhizobia is therefore important for increased plant growth and crop yields, especially of nodulated legumes. Several species of rhizobia have been identified as siderophore producers with benefits beyond Fe3+ sequestration. Many of these rhizobia have been found to differ markedly in their secretion of siderophores, with consequences on host plant nodulation (Carson et al., 1992). For example, increased siderophore production by Rhizobium and Bradyrhizobium strains that nodulated pigeon pea revealed increases in nodule mass, shoot N and Fe content (Duhan et al., 1998). The co-inoculation of common bean with Rhizobium phaseoli and a siderophore-producing Pseudomonas sp. LG also resulted in greater N and P accumulation (Stajković et al., 2011). Although the mechanisms of growth promotion remain complex and elusive, several studies involving mutants also point to the plant growth promoting traits of siderophores. For example, a mutant of Pseudomonas fluorescence with greater capacity for siderophore synthesis was found to increase plant growth in mung bean over the wild-type counterpart (Katiyar and Goel, 2004). Similarly, a wild-type endophytic Streptomyces sp. GMKU 3100 markedly improved root and shoot growth in mung bean and rice when compared to its siderophore-deficient mutant (Rungin et al., 2012). To tap the benefits of this trait, siderophore-producing microsymbionts such as Sinorhizobium meliloti, Rhizobium leguminosarum, Rhizobium leguminosarum bv. trifoli, and Bradyrhizobium japonicum have been identified as potential strains for use as Fe-based biofertilizers for increased crop yields (Guerinot, 1991). In addition to enhancing Fe nutrition and promoting plant growth, siderophores have also been implicated in plant adaptation to high concentrations of heavy metals in agricultural soils. Apparently, bacterial siderophores can chelate heavy metals such as Al, Cd, Cu, Pb and Zn to form complexes that are not toxic to plants (Braud et al., 2009; Schalk et al., 2011). Rhizobial siderophores are therefore important in the reclamation of contaminated soils for agricultural use. Although the legume-rhizobia symbiosis is sensitive to Al toxicity, siderophores can bind with Al ions and alleviate their toxic effect (Jaiswal et al., 2018), thus enhancing phytoremediation. So far, however, little is known about the chemistry of siderophores' interaction with heavy metals. Similarly, little attempt has been made to identify legume-rhizobia symbioses with efficiency in reclaiming contaminated soils associated with mining.
The genetics of siderophore production is rather complex. However, some genes involved in siderophore formation have been characterized. Rhizobia need iron for their own use in growth and symbiotic functioning, just as many proteins involved in the N2 fixation process require iron for the synthesis of hemoglobin needed for transporting O2 to respiring bacteroids. There are different types of siderophores. Rhizobia have the ability to produce a catechol siderophore to acquire iron under iron-poor conditions in the soil (Datta and Chakrabartty, 2014). The gene for rhizobactin 1021, a hydroxamate siderophore, was expressed under iron stress in Sinorhizobium meliloti (Lynch et al., 2001). Six genes (rhbABCDEF) needed in the biosynthesis of the siderophore have been identified and are located on an operon that is repressed under iron-replete conditions, while the gene rhtA encodes the outer membrane receptor protein for rhizobactin 1021 siderophore (Lynch et al., 2001). The transcription of both rhbABCDEF and rhtA genes is regulated by the product of the eighth gene in the cluster, namely rhrA, which has the characteristics of an AraC-type transcriptional activator (Lynch et al., 2001). According to McRose et al. (2018), some bacteria use quorum sensing (QS) to regulate siderophore production, including its concentration and ability to promote Fe uptake. In B. japonicum strain 61A152, a simple molecule such as citric acid can act as a siderophore (Guerinot et al., 1990), a finding confirmed by Siqueira et al. (2014) who showed that B. japonicum (Bj CPAC15), B. diazoefficiens strains Bd CPAC7, Bd USDA 110T and Bj USDA 6T all had three genes encoding citrate synthases enzyme. However, they also found genes related to the biosynthesis of cathecolate siderophores in strains Bj CPAC 15 and Bd CPAC 7. So far, however, siderophores produced by rhizobia have been identified in only S. meliloti, B. japonicum, and B. diazoefficiense (Lynch et al., 2001; Siqueira et al., 2014). With the many new symbionts continuously being reported, it is important to also identify their siderophores, as a necessary step for their use as Fe biofertilizers. Furthermore, more studies are needed to ascertain the Fe-releasing efficiency of the different types of siderophores.
THE ROLE OF LUMICHROME AND RIBOFLAVIN IN PLANT GROWTH PROMOTION
Microorganisms such as rhizobia are crucial in the promotion of plant growth, as they can synthesize and release phytohormones that alter the rhizosphere chemistry in favor of plant growth (Table 1). Rhizobial metabolites such as riboflavin and lumichrome are reportedly involved in chemical cross-talks leading to plant growth promotion, as well as improved immune response to biotic and abiotic stresses (Dakora et al., 2015; Kanazawa et al., 2020). As a result, soil microbes such as rhizobia and non-rhizobial bacteria have been used as inoculants because of these additional benefits. Exogenous application of lumichrome to both monocots and dicots yielded varied results, pointing to a dose-dependent beneficial outcome which is likely to be influenced by the type of plant species and the environment (Matiru and Dakora, 2005). Thus, any anticipated use of bacterial metabolites as plant growth promoters in cropping systems would require a better understanding of their operational mechanisms. Riboflavin released by rhizobia into the rhizosphere can promote microsymbiont colonization of root hairs, leading to greater and more effective nodulation and N2 fixation. For example, mutants of Sinorhizobium meliloti containing additional copies of the ribBA genes were found to exhibit increased synthesis of riboflavin leading to greater root colonization in Medicago sativa (Yang et al., 2002). Conversely, a Rhizobium leguminosarum ribN mutant exhibited reduced nodule occupancy when compared to the wild-type, further pointing to the vital role of riboflavin in the establishment of the legume-rhizobia symbiosis (Angulo et al., 2013). Lumichrome, the degradation by-product of riboflavin, is also reported to promote plant growth through improved symbiotic and photosynthetic functioning in legumes (Table 1; Phillips et al., 1999; Matiru and Dakora, 2005). Although the genetic basis for the involvement of lumichrome on stomatal function is scanty, the study by Matiru and Dakora (2005) reported increases in leaf stomatal conductance and transpiration rates when compared to untreated plants, with rhizobial inoculation producing similar responses. Similarly, lumichrome treatment was reported to increase photosynthetic rates in corn and soybean when compared to untreated plants (Khan et al., 2008). At the molecular level, lumichrome can induce the expression of genes involved in cell differentiation and cell expansion leading to increased plant biomass accumulation (Pholo et al., 2018). Given their roles at different stages of the legume-rhizobia symbiosis, riboflavin and lumichrome released by rhizobia can maximize plant growth and increase crop productivity via several alterations in the plant's physiology, including enhanced symbiosis and photosynthetic functioning.
The genetic basis of plant growth promotion by lumichrome (chemically defined as 7,8 dimethylalloxazine) stems from its ability to induce the expression of genes responsible for cell growth and mitotic division, and appears to coordinate cell division and proliferation in developing leaves. Lumichrome is also reported to increase CO2 concentration in the rhizosphere (Phillips et al., 1999) which is needed for the growth of N2- fixing rhizobia and mycorrhizal fungi (Maier et al., 1979). Pholo et al. (2018) found that the enhancement of mitotic CYCD3.3, CYCA1.1, SP1L3, RSW7, and PDF1 transcripts in lumichrome-treated Arabidopsis thaliana plants resulted in high plant biomass from cell differentiation and cell expansion. Moreover, lumichrome also increased starch accumulation in soybean and tomato by increasing glyceraldehyde 3-phosphate denhydrogenase (GAPDH) transcripts and NAD-dependent enzyme activity (Gouws et al., 2012). Additionally, lumichrome can also reduce the levels of gene expression associated with ethylene metabolism such as Acc oxydase 1 (ACO1), and a C2H2 zinc finger protein, leading to a minimal effect of ethylene on plant growth. However, Pholo et al. (2018) found a synergistic ethylene-auxin cross-talk via a reciprocal over-expression of ACO1 and SAUR54 in which ethylene activated the auxin biosynthetic pathway and regulated Arabidopsis growth, in addition to suppressing the negative effects of methyl jasmonate (MeJa) on chlorophyll loss and decreases in Rubisco and photosynthesis (Pholo et al., 2018). For example, treating Arabidopsis thaliana or soybean with Methyl jasmonate caused a decrease in leaf photosynthetic rates due to impaired chlorophyll production (Jung, 2004; Anjum et al., 2011). Whereas, pre-incubation of Bradyrhizobium japonicum with jasmonates prior to inoculation led to enhanced nodulation and N2 fixation in soybean (Mabood and Smith, 2005), the possible indirect inhibitory effect of jasmonate on symbiosis via reduced photosynthetic functioning remains to be determined.
ACC DEAMINASE-PRODUCING RHIZOBIAL BACTERIA
ACC (1-aminocyclopropane-1-carboxylic acid) deaminase is a known precursor of ethylene (Penrose and Glick, 2003) and is a plant growth-promoting enzyme (Table 1) that uses pyridoxal 5-phosphate (PLP) as substrate (Honma and Shimomura, 1978). ACC deaminase enzyme localized in the cytoplasm, is encoded by acdS gene (Honma and Shimomura, 1978; Jacobson et al., 1994). In Mesorhizobium loti, this acdS gene is located in the symbiotic island and is regulated by the NifA2 gene (Nukui et al., 2006). When ACC produced by plants is exuded into the rhizosphere, rhizobacteria that express ACC deaminase activity take up the ACC and degrade it within the bacterial cytoplasm (Glick et al., 1998; Penrose and Glick, 2003). This enzyme is constitutively expressed during nodule initiation, and under stressed conditions in plant roots (Ligero et al., 1986; Spaink, 1997; Saleem et al., 2007). The plant exuded ACC is often used by bacteria as an N source; however, ACC deaminase can also degrade ACC to lower ethylene levels, especially in plants exposed to biotic and abiotic stresses, as well as during nodule initiation by legumes and rhizobia (Glick et al., 1998). Earlier studies have shown the harmful effect of ethylene in inhibiting nodule formation through interfering with root hair deformation, infection thread elongation into the inner cortex, calcium spiking and the proliferation of rhizobia in legumes such as Pisum sativum, Trifolium repens, and Medicago sativa (Goodlass and Smith, 1979; Peters and Crist-Estes, 1989; Lee and LaRue, 1992; Oldroyd et al., 2001; Tamimi and Timko, 2003; Lohar et al., 2009). However, co-inoculation of either Rhizobium tropici CIAT899 or Cupriavidus taiwanensis STM894 with the wild-type ACC deaminase-producing Pseudomonas fluorescens YsS6 was found to improve nodulation and plant growth in Phaseolus vulgaris and Mimosa pudica, respectively, when compared to its mutant defective in ACC deaminase production (Nascimento et al., 2019). Ma et al. (2004) also found that an ACC deaminase-producing Sinorhizobium meliloti mutant elicited 40% more nodulation in Madicago sativa than the parent strain. Thus, the mechanism by which ACC deaminase-producing bacteria increase plant nodulation seems to involve the regulation of both ACC oxidase activity and ethylene synthesis, as observed in pea plants treated with ACC deaminase-producing Arthrobacter protophormiae (Barnawal et al., 2014). Furthermore, a S. meliloti strain harboring the acdS gene and co-inoculated with P. putida UW4 increased nodule number in Medicago lupulina when compared to inoculation with the wild type (Kong et al., 2015). The important plant growth promoting traits of these bacteria-produced molecules points to the need to bioprospect for rhizobial strains that possess such traits for increased symbiotic performance, plant growth promotion and increased grain yield.
The PvACS gene in Phaseolus vulgaris encodes ACC synthase which causes the formation of ethylene responsible for nodule senescence (Nascimento et al., 2018; Serova et al., 2018). The PvGS(n-1), a gene controlling the transcription of glutamine synthetase and commonly found in senescent nodules, is needed for ammonium assimilation in legumes (Hungria and Kaschuk, 2014; da Silva et al., 2019). However, the expression of this gene was found to enhance nitrogenase activity and leghaemoglobin concentration, leading to delayed senescence which increased ammonia assimilation and N2 fixation (Lara et al., 1983; Alemneh et al., 2020). Another enzyme, Uricase II, is needed in the metabolism of N-fixed for export as ureides from root nodules to shoots and tends to decline in senescent nodules (Papadopoulou et al., 1995; Capote-Mainez and Sánchez, 1997) as found for leghemoglobin, which is a physiological marker for nodule senescence. ACC deaminase-producing rhizobia can stimulate nodule formation and function and thus increase the amount of N2-fixed needed for plant growth.
RHIZOBIA-AIDED ACQUISITION OF PHOSPHORUS BY NODULATED LEGUMES
To increase crop yields for meeting global food security would require major nutrient inputs, especially N and P. Plants take up P in the form of phosphate that comes from the 83% of the world's P reserves occurring as rock phosphate in only Morocco, China, South Africa and the USA (Vaccari, 2009). The world's vast 5.7 billion hectares of agricultural land are generally deficient in P that must be added as input to increase crop productivity (Mouazen and Kuang, 2016). In Africa, most smallholder farmers grow their crops with insufficient or no P input. However, plant growth-promoting bacteria and fungi can influence plant development directly or indirectly by facilitating the supply and uptake of mineral nutrients from the soil. Solubilization of unavailable soil P compounds by symbiotic rhizobia in the rhizosphere is one strategy for enhancing P availability for plant growth and yield (Marra et al., 2012). Although P is an important macronutrient for plant development, about 95 to 99% of the soil P occurs in insoluble form which is not useable by plants (Vassilev et al., 2001). Some rhizobial bacteria are however capable of solubilizing unavailable soil P for plant uptake and growth. Aside plants, bacteroids in root nodules also require P for their metabolism; and the high-affinity phosphate transporter PstSCAB is known to promote the efficiency of the Sinorhizobium fredii-soybean symbiosis (Hu et al., 2018), just as the uptake of inorganic P leading to enhanced N2 fixation during P starvation is facilitated by the phoCDET genes which encode the ABC-type transport system in Sinorhizobium meliloti (Bardin et al., 1996; Yuan et al., 2006). Thus, given the vital roles of P in both plant and bacterial metabolism, screening for P-solubilizing traits in N2-fixing rhizobia can be a cheaper and useful strategy to ameliorate the negative effects of soil P stress on plants for improved crop yields and food security.
Immobilization of P can occur in inorganic and organic forms (Rodriguez and Fraga, 1999). During microbial P transformation, the carboxyl and hydroxyl ion containing organic acids release their protons and bind to cations (Bergkemper et al., 2016), thus lowering the pH (Richardson and Simpson, 2011). The acidification caused by microbial activity can result in the release of P ions by substitution with H+ (Rodriguez and Fraga, 1999; Richardson and Simpson, 2011). In low-P soils, rhizobia can solubilize soil-bound P in the rhizosphere through acidification by synthesizing gluconic acid under the control of pyrroloquinoline quinone (PQQ) genes (Yadav et al., 2020). Of all the organic acids, gluconic acid is most potent in P solubilization and the oxidation of glucose to gluconic acid by rhizobia is an important step in the solubilization of soil P (Richardson et al., 2011). Gene gcd in rhizobia encodes quinoprotein glucose dehydrogenase (PQQGDH), which is involved in the release of organic anions to solubilize inorganic P (Rodriguez et al., 2000). In addition to organic acids, inorganic acids and mycorrhizal fungi in soil can also enhance phosphorus solubilization (Alori et al., 2017). Whereas rhizobia are largely known for their N2-fixing traits, mycorrhizal fungi are particularly known for their role in the acquisition of phosphorus and other nutrients required by plants (Bolan, 1991). A synergistic interaction was observed when faba bean was treated with Rhizobium leguminosarum bv. viciae and arbuscular mycorrhizal fungi which resulted in increased nodulation and nitrogenase activity probably through enhanced P acquisition by the mycorrhizal partner (Abd-Alla et al., 2014).
Rhizobia and other microbes habour genes such as phoD and phoA which encode alkaline phosphatase, the appA gene which encodes phytase, and the phn gene which encodes C-P lyase enzymes that can convert soil organic-P into available P for plant uptake (Rodríguez et al., 2006). The organic P however occupies about 30–50% of the total soil P pool in forms such as inositol phosphate (soil phytate), phosphomonoesters, phosphodiesters, phospholipids, nucleic acids, and phosphotriesters (Rodriguez and Fraga, 1999). The activity of microbial P-enzymes on the huge P reservoir in soils largely accounts for P supply to terrestrial plants. P assimilation under P-poor soil conditions is usually achieved using high-affinity P transporters, in contrast to P-rich soils where low-affinity P transporters are involved (Hsieh and Wanner, 2010). The expression of genes phoU, phoR, and phoB in soil microbes largely regulates the P-starvation response in cropping systems for increased use of external P sources (Eder et al., 1996). However, information is lacking from a systems approach that links P status of the soil to plant and bacterial gene expression for P-enzyme formation/release for increased P availability, uptake and utilization. For example, what are the cues for low-P sensing in soil by plants and microbes, and what is the timeframe required for biological processes such gene expression to occur? And what are the regulatory mechanisms underlying the build-up of P pool in response to its demand by plants?
ROLE OF SYMBIOTIC RHIZOBIA IN PLANT DEFENSE
The successful induction of root nodules and their subsequent colonization by rhizobia require the production of rhizobial Nod factors that allow for their recognition by the host legume (Via et al., 2016). Aside their growth promoting effects, rhizobia have been implicated in processes leading to induced systemic resistance (ISR) in host plants which is governed by complex mechanisms; for example, inoculating common bean with Rhizobium etli led to enhanced resistance to infection by Pseudomonas syringae pv. phaseolicola via the accumulation of reactive oxygen species, increased callose production and the activation of defense related genes (Díaz-Valle and Alvarez-Venegas, 2019). The response of host plants to pathogen infection can include a molecular cross-talk between salicylic acid and jasmonates, both of which play key roles in the activation of plant defense related genes (Pieterse et al., 2012). Upon attack by insects for instance, intricate processes which include the synthesis of salicylic acid and jasmonates occur leading to the activation of genes responsible for plant defense (Hettenhausen et al., 2015; Wang et al., 2019b). Moreover, siderophores produced by symbiotic rhizobia and other microbes do not only enhance Fe nutrition for healthy plant growth and grain yield in legumes, but also serve as biocontrol agents against pathogens (Table 1). For example, siderophores produced by Sinorhizobium meliloti were shown to suppress Macrophomina phaseolina, the causal agent of charcoal rot in groundnut (Arora et al., 2001). Similarly, co-inoculation of groundnut with Rhizobium and Trichoderma harzianum successfully inhibited infection by Sclerotium rolfsii, the fungal pathogen that causes stem rot disease (Ganesan et al., 2007). A Rhizobium species was also found to protect soybean from root rot caused by Phytophthora megasperma, while a Sinorhizobium sp. inhibited plant infection by Fusarium oxysporum (Deshwal et al., 2003). Rhizobitoxine-producing strains of Bradyrhizobium japonicum were also able to successfully block infection of soybean by Macrophomina phaseolina, the causal pathogen of charcoal rot (Deshwal et al., 2003).
In addition to their roles as signal molecules in the legume-rhizobia symbiosis, rhizobial metabolites such as riboflavin and lumichrome, as well as vitamins which include thiamine, biotin, niacin and ascorbic acid have been implicated in legume plant defense against pathogens (Mehboob et al., 2009; Palacios et al., 2014). For instance, spraying riboflavin on tobacco and Arabidopsis caused resistance to Peronospora parasitica, Pseudomonas syringae pv. tomato, the Tobacco mosaic virus and Alternaria alternata (Dong and Beer, 2000), possibly due to the expression of pathogenesis-related genes which induced systemic acquired resistance to the pathogens. In Arabidopsis, riboflavin was similarly found to induce the priming of plant defense response toward infection by Pseudomonas syringae pv. tomato and was linked to the expression of genes involved in plant defense responses (Zhang et al., 2009). Moreover, Mesorhizobium loti induced the expression of the Phenylalanine Ammonia lyase (LjPAL1) gene responsible for the synthesis of salicylic acid, and consequently altered the response of Lotus japonicum to infection by Pseudomonas syringae (Chen et al., 2017). Legumes are also reported to protect themselves against pathogens using isoflavonoids, phytoalexins and phytoanticipins (Dakora and Phillips, 1996). It is therefore possible that the health of a legume plant is dependent on a molecular cross-talk involving several defense molecules such as isoflavones, riboflavin, thiamine and other yet unknown molecules (Subramanian et al., 2004; Ahn et al., 2005; Yadav et al., 2020). In Arabidopsis for example, infection with Sclerotinia scleroticum increased the expression of the IFS1 gene that codes for isoflavone synthase and highlights the involvement of isoflavones as plant defense molecules (Subramanian et al., 2004). Also, a thiamine treatment led to the accumulation of hydrogen peroxide (H2O2) and a build-up of lignin in roots of rice following infection with the root-knot nematode (Meloidogyne graminicola); this was associated with the increased transcription of the OsPAL1 and OsC4H genes involved in the phenylpropanoid pathway (Huang et al., 2016). Clearly, plants have diverse ways of overcoming biotic and abiotic stress within their environments through the synthesis of novel molecules. For example, following the infection of Arabidopsis by Pieris rapae, one branch of the jasmonate signaling pathway regulated by the MYC2 gene was expressed (Santino et al., 2013). Clearly, a lot remains to be unraveled regarding the complex chemical cross-talks involved in plant adaptation to disease infection and pathogen attack.
Conclusions
Plant growth and productivity is dependent on multiple factors, which include mineral nutrition, resistance to insect pests and diseases. Fortunately, symbiotic rhizobia are capable of triggering biological pathways that cause outcomes with direct and indirect effects on plant growth promotion and protection. Studying the interlinkages of outcomes from the legume-rhizobia symbioses has the potential to identify microsymbionts for use as inoculants due to the multiplicity of functions that they elicit. For example, rhizobia can be identified that (i) produce greater symbiotic N for host plant growth and productivity (ii) elicit the synthesis of host-plant compounds for defense and increased plant growth, (iii) produce environmental cues that regulate stomatal function and (iv) emit vitamins as growth factors for plant defense and increased growth/productivity. Whereas, some of the outcomes triggered by rhizobia may be tied to their symbiotic interactions with legumes, the effects of some of the signal molecules produced often extends to non-legumes, thus indicating a wider distribution of these traits among diverse bacterial genera.
Author Contributions
SJ and MM drafted the manuscript. FI produced the photo used in Figure 1. The photo was shot by her from her glasshouse studies. Some of the ideas in the manuscript are also from her Master's thesis. FD conceived the idea, edited, and approved the final version of the paper. All authors contributed to the article and approved the submitted version.
Funding
FD was grateful to the NRF, Tshwane University of Technology (TUT) and the South African Research Chair in Agrochemurgy and Plant Symbiosis for continued funding of his research. SJ and MM also acknowledge the NRF and TUT for continued support of their postdoctoral fellowships through the Research Chair.
Conflict of Interest
The authors declare that the research was conducted in the absence of any commercial or financial relationships that could be construed as a potential conflict of interest.
References
Abd-Alla, M. H., El-Enany, A. W. E., Nafady, N. A., Khalaf, D. M., and Morsy, F. M. (2014). Synergistic interaction of Rhizobium leguminosarum bv. viciae and arbuscular mycorrhizal fungi as a plant growth promoting biofertilizers for faba bean (Vicia faba L.) in alkaline soil. Microbiol. Res. 169, 49–58. doi: 10.1016/j.micres.2013.07.007
Abdiev, A., Khaitov, B., Toderich, K., and Park, K. W. (2019). Growth, nutrient uptake and yield parameters of chickpea (Cicer arietinum L.) enhance by Rhizobium and Azotobacter inoculations in saline soil. J. Plant Nutr. 42, 2703–2714. doi: 10.1080/01904167.2019.1655038
Ahn, I. P., Kim, S., and Lee, Y. H. (2005). Vitamin B1 functions as an activator of plant disease resistance. Plant Physiol. 138, 1505–1515. doi: 10.1104/pp.104.058693
Alemneh, A. A., Zhou, Y., Ryder, M. H., and Denton, M. D. (2020). Mechanisms in plant growth-promoting rhizobacteria that enhance legume–rhizobial symbioses. J. Appl. Microbiol. 129, 1133–1156. doi: 10.1111/jam.14754
Ali, B., Hayat, S., Aiman Hasan, S., and Ahmad, A. (2008). A comparative effect of IAA and 4-Cl-IAA on growth, nodulation and nitrogen fixation in Vigna radiata (L.) Wilczek. Acta Physiol. Plant 30, 35–41. doi: 10.1007/s11738-007-0088-4
Alori, E. T., Glick, B. R., and Babalola, O. O. (2017). Microbial phosphorus solubilization and its potential for use in sustainable agriculture. Front. Microbiol. 8:971. doi: 10.3389/fmicb.2017.00971
Andrews, M., and Andrews, M. E. (2017). Specificity in legume-rhizobia symbioses. Int. J. Mol. Sci. 18:705. doi: 10.3390/ijms18040705
Angulo, V. A. G., Bonomi, H. R., Posadas, D. M., Serer, M. I., Torres, A. G., Zorreguieta, Á., et al. (2013). Identification and characterization of RibN, a novel family of riboflavin transporters from Rhizobium leguminosarum and other proteobacteria. J. Bacteriol. 195, 4611–4619. doi: 10.1128/JB.00644-13
Anjum, S. A., Xie, X., Farooq, M., Wang, L., Xue, L. I., Shahbaz, M., et al. (2011). Effect of exogenous methyl jasmonate on growth, gas exchange and chlorophyll contents of soybean subjected to drought. Afr. J. Biotechnol. 10, 9647–9656. doi: 10.5897/AJB10.2641
Ardley, J. K., Reeve, W. G., O'Hara, G. W., Yates, R. J., Dilworth, M. J., and Howieson, J. G. (2013). Nodule morphology, symbiotic specificity and association with unusual rhizobia are distinguishing features of the genus Listia within the southern African crotalarioid clade Lotononis sl. Ann. Bot. 112, 1–15. doi: 10.1093/aob/mct095
Argaw, A. (2012). Evaluation of co-inoculation of Bradyrhizobium japonicum and phosphate solubilizing Pseudomonas spp. effect on soybean (Glycine max L. (Merr.)) in Assossa area. J. Agr. Sci. Tech-Iran 14, 213–224. Available online at: http://jast.modares.ac.ir/article-23-5342-en.html
Arora, N. K., Kang, S. C., and Maheshwari, D. K. (2001). Isolation of siderophore-producing strains of Rhizobium meliloti and their biocontrol potential against Macrophomina phaseolina that causes charcoal rot of groundnut. Curr. Sci. 81, 673–677. Available online at: https://www.jstor.org/stable/24106362?seq=1
Bal, H. B., Das, S., Dangar, T. K., and Adhya, T. K. (2013). ACC deaminase and IAA producing growth promoting bacteria from the rhizosphere soil of tropical rice plants. J. Basic Microbiol. 53, 972–984. doi: 10.1002/jobm.201200445
Bardin, S., Dan, S., Osteras, M., and Finan, T. M. (1996). A phosphate transport system is required for symbiotic nitrogen fixation by Rhizobium meliloti. J. Bacteriol. 178, 4540–4547. doi: 10.1128/JB.178.15.4540-4547.1996
Bargaz, A., Lazali, M., Amenc, L., Abadie, J., Ghoulam, C., Farissi, M., et al. (2013). Differential expression of trehalose 6-P phosphatase and ascorbate peroxidase transcripts in nodule cortex of Phaseolus vulgaris and regulation of nodule O2 permeability. Planta 238, 107–119. doi: 10.1007/s00425-013-1877-1
Barnawal, D., Bharti, N., Maji, D., Chanotiya, C. S., and Kalra, A. (2014). ACC deaminase-containing Arthrobacter protophormiae induces NaCl stress tolerance through reduced ACC oxidase activity and ethylene production resulting in improved nodulation and mycorrhization in Pisum sativum. J. Plant Physiol. 171, 884–894. doi: 10.1016/j.jplph.2014.03.007
Bartwal, A., Mall, R., Lohani, P., Guru, S. K., and Arora, S. (2013). Role of secondary metabolites and brassinosteroids in plant defense against environmental stresses. J. Plant Growth Regul. 32, 216–232. doi: 10.1007/s00344-012-9272-x
Belane, A. K., Asiwe, J., and Dakora, F. D. (2011). Assessment of N2 fixation in 32 cowpea (Vigna unguiculata L. Walp) genotypes grown in the field at Taung in South Africa, using 15N natural abundance. 10, 11450–11458. doi: 10.5897/AJB11.674
Berg, G. (2009). Plant-microbe interactions promoting plant growth and health: perspectives for controlled use of microorganisms in agriculture. Appl. Microbiol. Biotechnol. 84, 11–18. doi: 10.1007/s00253-009-2092-7
Bergkemper, F., Schöler, A., Engel, M., Lang, F., Krüger, J., Schloter, M., et al. (2016). Phosphorus depletion in forest soils shapes bacterial communities towards phosphorus recycling systems. Environ. Microbiol. 18, 1988–2000. doi: 10.1111/1462-2920.13188
Bianco, L. (2014). Rhizobial infection in Adesmia bicolor (Fabaceae) roots. Arch. Microbiol. 196, 675–679. doi: 10.1007/s00203-014-1004-0
Bolan, N. S. (1991). A critical review on the role of mycorrhizal fungi in the uptake of phosphorus by plants. Plant Soil 134, 189–207. doi: 10.1007/BF00012037
Bottini, R., Cassán, F., and Piccoli, P. (2004). Gibberellin production by bacteria and its involvement in plant growth promotion and yield increase. Appl. Microbiol. Biotechnol. 65, 497–503. doi: 10.1007/s00253-004-1696-1
Bottini, R., Fulchieri, M., Pearce, D., and Pharis, R. P. (1989). Identification of gibberellins A1, A3, and iso-A3 in cultures of Azospirillum lipoferum. Plant Physiol. 90, 45–47. doi: 10.1104/pp.90.1.45
Braud, A., Hoegy, F., Jezequel, K., Lebeau, T., and Schalk, I. J. (2009). New insights into the metal specificity of the Pseudomonas aeruginosa pyoverdine–iron uptake pathway. Environ. Microbiol. 11, 1079–1091. doi: 10.1111/j.1462-2920.2008.01838.x
Camerini, S., Senatore, B., Lonardo, E., Imperlini, E., Bianco, C., Moschetti, G., et al. (2008). Introduction of a novel pathway for IAA biosynthesis to rhizobia alters vetch root nodule development. Arch. Microbiol. 190, 67–77. doi: 10.1007/s00203-008-0365-7
Capote-Mainez, N., and Sánchez, F. (1997). Characterization of the common bean uricase II and its expression in organs other than nodules. Plant Physiol. 115, 1307–1317. doi: 10.1104/pp.115.4.1307
Carson, K. C., Holliday, S., Glenn, A. R., and Dilworth, M. J. (1992). Siderophore and organic acid production in root nodule bacteria. Arch. Microbiol. 157, 264–271. doi: 10.1007/BF00245160
Carson, K. C., Meyer, J. M., and Dilworth, M. J. (2000). Hydroxamate siderophores of root nodule bacteria. Soil Biol. Biochem. 32, 11–21. doi: 10.1016/S0038-0717(99)00107-8
Chaiharn, M., and Lumyong, S. (2009). Phosphate solubilization potential and stress tolerance of rhizobacteria from rice soil in Northern Thailand. World J. Microbiol. Biotechnol. 25, 305–314. doi: 10.1007/s11274-008-9892-2
Chebotar, V. K., Asis, C. A., and Akao, S. (2001). Production of growth-promoting substances and high colonization ability of rhizobacteria enhance the nitrogen fixation of soybean when co-inoculated with Bradyrhizobium japonicum. Biol. Fert. Soils 34, 427–432. doi: 10.1007/s00374-001-0426-4
Chen, Y., Li, F., Tian, L., Huang, M., Deng, R., Li, X., et al. (2017). The phenylalanine ammonia lyase gene LJPAL1 is involved in plant defense responses to pathogens and plays diverse roles in Lotus japonicus-rhizobium symbioses. Mol. Plant Microbe Interact. 30, 739–753. doi: 10.1094/MPMI-04-17-0080-R
Chihaoui, S.-A., Trabelsi, D., Jdey, A., Mhadhbi, H., and Mhamdi, R. (2015). Inoculation of Phaseolus vulgaris with the nodule-endophyte Agrobacterium sp. 10C2 affects richness and structure of rhizosphere bacterial communities and enhances nodulation and growth. Arch. Microbiol. 197, 805–813. doi: 10.1007/s00203-015-1118-z
da Silva, H. A. P., Caetano, V. S., Pessoa, D. D. V., Pacheco, R. S., and Simoes-Araujo, J. L. (2019). Molecular and biochemical changes of aging-induced nodules senescence in common bean. Symbiosis 79, 33–48. doi: 10.1007/s13199-019-00618-2
Dabo, M., Jaiswal, S. K., and Dakora, F. D. (2019). Phylogenetic evidence of allopatric speciation of bradyrhizobia nodulating cowpea (Vigna unguiculata L. Walp) in South African and Mozambican soils. FEMS Microbiol. Ecol. 95, 1–14. doi: 10.1093/femsec/fiz067
Dakora, F. D. (2003). Defining new roles for plant and rhizobial molecules in sole and mixed plant cultures involving symbiotic legumes. New Phytologist 158, 39–49. doi: 10.1046/j.1469-8137.2003.00725.x
Dakora, F. D., Matiru, V. N., and Kanu, A. S. (2015). Rhizosphere ecology of lumichrome and riboflavin, two bacterial signal molecules eliciting developmental changes in plants. Front. Plant Sci. 6:700. doi: 10.3389/fpls.2015.00700
Dakora, F. D., and Phillips, D. A. (1996). Diverse functions of isoflavonoids in legumes transcend anti-microbial definitions of phytoalexins. Physiol. Mol. Plant Pathol. 49, 1–20. doi: 10.1006/pmpp.1996.0035
Dakora, F. D., and Phillips, D. A. (2002). Root exudates as mediators of mineral acquisition in low-nutrient environments. Plant Soil 245, 35–47. doi: 10.1023/A:1020809400075
Dashti, N., Zhang, F., Hynes, R., and Smith, D. (1998). Plant growth promoting rhizobacteria accelerate nodulation and increase nitrogen fixation activity by field grown soybean [Glycine max (L.) Merr.] under short season conditions. Plant Soil 200, 205–213. doi: 10.1023/A:1004358100856
Datta, B., and Chakrabartty, P. K. (2014). Siderophore biosynthesis genes of Rhizobium sp. isolated from Cicer arietinum L. 3 Biotech. 4, 391–401. doi: 10.1007/s13205-013-0164-y
Datta, C., and Basu, P. S. (2000). Indole acetic acid production by a Rhizobium species from root nodules of a leguminous shrub, Cajanus cajan. Microbiological Res. 155, 123–127. doi: 10.1016/S0944-5013(00)80047-6
Defez, R., Andreozzi, A., Romano, S., Pocsfalvi, G., Fiume, I., Esposito, R., et al. (2019). Bacterial IAA-delivery into medicago root nodules triggers a balanced stimulation of C and N metabolism leading to a biomass increase. Microorganisms 7:403. doi: 10.3390/microorganisms7100403
Deshwal, V. K., Pandey, P., Kang, S. C., and Maheshwari, D. K. (2003). Rhizobia as a biological control agent against soil borne plant pathogenic fungi. Indian J. Exp. Biol. 41, 1160–1164. Available online at: http://nopr.niscair.res.in/handle/123456789/23372
Díaz-Valle, A., and Alvarez-Venegas, R. (2019). Enhancement of pathogen resistance in common bean plants by inoculation with Rhizobium etli. Front. Plant Sci. 10:1317. doi: 10.3389/fpls.2019.01317
Dong, H., and Beer, S. V. (2000). Riboflavin induces disease resistance in plants by activating a novel signal transduction pathway. Phytopathology 90, 801–811. doi: 10.1094/PHYTO.2000.90.8.801
Dong, Z., and Layzell, D. B. (2001). H2 oxidation, O2 uptake and CO2 fixation in hydrogen treated soils. Plant Soil 229, 1–12. doi: 10.1023/A:1004810017490
Dubois, M., Van den Broeck, L., and Inzé, D. (2018). The pivotal role of ethylene in plant growth. Trends Plant Sci. 23, 311–323. doi: 10.1016/j.tplants.2018.01.003
Duhan, J. S., Dudeja, S. S., and Khurana, A. L. (1998). Siderophore production in relation to N2 fixation and iron uptake in pigeon pea-Rhizobium symbiosis. Folia Microbiol. 43, 421–426. doi: 10.1007/BF02818585
Eder, S., Shi, L., Jensen, K., Yamane, K., and Hulett, F. M. (1996). A Bacillus subtilis secreted phosphodiesterase/alkaline phosphatase is the product of a Pho regulon gene, phoD. Microbiology 142, 2041–2047. doi: 10.1099/13500872-142-8-2041
Erice, G., Sanz-Sáez, A., Aroca, R., Ruíz-Lozano, J. M., Avice, J.-C., Irigoyen, J. J., et al. (2014). Photosynthetic down-regulation in N2-fixing alfalfa under elevated CO2 alters rubisco content and decreases nodule metabolism via nitrogenase and tricarboxylic acid cycle. Acta Physiol. Plant 36, 2607–2617. doi: 10.1007/s11738-014-1631-8
Ferguson, B. J., Indrasumunar, A., Hayashi, S., Lin, M.-H., Lin, Y.-H., Reid, D. E., et al. (2010). Molecular analysis of legume nodule development and autoregulation. J. Integr. Plant Biol. 52, 61–76. doi: 10.1111/j.1744-7909.2010.00899.x
Ferguson, B. J., Ross, J. J., and Reid, J. B. (2005). Nodulation phenotypes of gibberellin and brassinosteroid mutants of pea. Plant Physiol. 138, 2396–2405. doi: 10.1104/pp.105.062414
Fisher, H. M. (1994). Genetic regulation of nitrogen fixation in rhizobia. Microbiol. Rev. 58, 352–386. doi: 10.1128/MMBR.58.3.352-386.1994
Foo, E., McAdam, E. L., Weller, J. L., and Reid, J. B. (2016). Interactions between ethylene, gibberellins, and brassinosteroids in the development of rhizobial and mycorrhizal symbioses of pea. J. Exp. Bot. 67, 2413–2424. doi: 10.1093/jxb/erw047
Ganesan, S., Kuppusamy, R. G., and Sekar, R. (2007). Integrated management of stem rot disease (Sclerotium rolfsii) of groundnut (Arachis hypogaea L.) using Rhizobium and Trichoderma harzianum (ITCC - 4572). Turkish J. Agric. For. 31, 103–108. doi: 10.3906/tar-0609-15
Gauthier-Coles, C., White, R. G., and Mathesius, U. (2019). Nodulating legumes are distinguished by a sensitivity to cytokinin in the root cortex leading to pseudonodule development. Front. Plant Sci. 9:1901. doi: 10.3389/fpls.2018.01901
Ghosh, S., and Basu, P. S. (2006). Production and metabolism of indole acetic acid in roots and root nodules of Phaseolus mungo. Microbiol. Res. 161, 362–366. doi: 10.1016/j.micres.2006.01.001
Giller, K. E., and Cadisch, G. (1995). “Future benefits from biological nitrogen fixation: an ecological approach to agriculture,” in Management of biological nitrogen fixation for the development of more productive and ustainable agricultural systems. Developments in Plant and Soil Sciences, Vol. 65, eds J. K. Ladha, and M. B. Peoples (Dordrecht: Springer), 255–277. doi: 10.1007/978-94-011-0053-3_13
Giron, D., Frago, E., Glevarec, G., Pieterse, C. M., and Dicke, M. (2013). Cytokinins as key regulators in plant–microbe–insect interactions: connecting plant growth and defence. Funct. Ecol. 27, 599–609. doi: 10.1111/1365-2435.12042
Glick, B. R., Cheng, Z., Czarny, J., and Duan, J. (2007). Promotion of plant growth by ACC deaminase-producing soil bacteria. Eur. J. Plant Pathol. 119, 329–339. doi: 10.1007/s10658-007-9162-4
Glick, B. R., Penrose, D. M., and Li, J. (1998). A model for the lowering of plant ethylene concentrations by plant growth-promoting bacteria. J. Theor. Biol. 190, 63–68. doi: 10.1006/jtbi.1997.0532
González-Sama, A., Lucas, M. M., De Felipe, M. R., and Pueyo, J. J. (2004). An unusual infection mechanism and nodule morphogenesis in white lupin (Lupinus albus). New Phytol. 163, 371–380. Available online at: https://www.jstor.org/stable/1514387
Goodlass, G., and Smith, K. (1979). Effects of ethylene on root extension and nodulation of pea (Pisum sativum L.) and white clover (Trifolium repens L.). Plant Soil 51, 387–395. doi: 10.1007/BF02197785
Gopalakrishnan, S., Sathya, A., Vijayabharathi, R., Varshney, R. K., Gowda, C. L. L., and Krishnamurthy, L. (2015). Plant growth promoting rhizobia: challenges and opportunities. 3 Biotech 5, 355–377. doi: 10.1007/s13205-014-0241-x
Gouws, L., Botes, E., Wiese, A. J., Trenkamp, S., Torres-Jerez, I., Tang, Y., et al. (2012). The plant growth promoting substance, lumichrome, mimics starch and ethylene-associated symbiotic responses in lotus and tomato roots. Front. Plant Sci. 3:120. doi: 10.3389/fpls.2012.00120
Gravel, V., Antoun, H., and Tweddell, R. J. (2007). Growth stimulation and fruit yield improvement of greenhouse tomato plants by inoculation with Pseudomonas putida or Trichoderma atroviride: possible role of indole acetic acid (IAA). Soil Biol. Biochem. 39, 1968–1977. doi: 10.1016/j.soilbio.2007.02.015
Grobelak, A., and Hiller, J. (2017). Bacterial siderophores promote plant growth: screening of catechol and hydroxamate siderophores. Int. J. Phytoremediation 19, 825–833. doi: 10.1080/15226514.2017.1290581
Guerinot, M. L. (1991). “Iron uptake and metabolism in the rhizobia/legume symbioses,” in Iron Nutrition and Interactions in Plants. Developments in Plant and Soil Sciences, Vol. 43, eds Y. Chen, and Y. Hadar (Dordrecht: Springer), 39–249. doi: 10.1007/978-94-011-3294-7_29
Guerinot, M. L., Meidl, E. J., and Plessner, O. (1990). Citrate as a siderophore in Bradyrhizobium japonicum. J. Bacteriol. 172, 3298–3303. doi: 10.1128/JB.172.6.3298-3303.1990
Gyogluu, C., Jaiswal, S. K., Kyei-Boahen, S., and Dakora, F. D. (2018). Identification and distribution of microsymbionts associated with soybean nodulation in mozambican soils. Syst. Appl. Microbiol. 41, 506–515. doi: 10.1016/j.syapm.2018.05.003
Hao, X., Taghavi, S., Xie, P., Orbach, M. J., Alwathnani, H. A., Rensing, C., et al. (2014). Phytoremediation of heavy and transition metals aided by legume-rhizobia symbiosis. Int. J. Phytoremediation 16, 179–202. doi: 10.1080/15226514.2013.773273
Hause, B., and Schaarschmidt, S. (2009). The role of jasmonates in mutualistic symbioses between plants and soil-born microorganisms. Phytochemistry 70, 1589–1599. doi: 10.1016/j.phytochem.2009.07.003
Hayashi, S., Gresshoff, P. M., and Ferguson, B. J. (2014). Mechanistic action of gibberellins in legume nodulation. J. Integr. Plant Biol. 56, 971–978. doi: 10.1111/jipb.12201
Herridge, D. (2008). “Inoculation technology for legumes,” in Nitrogen-Fixing Leguminous Symbioses, eds M. J. Dilworth, E. K. James, J. I. Sprent, and W. E. Newton (Dordrecht: Springer), 77–115. doi: 10.1007/978-1-4020-3548-7_4
Hettenhausen, C., Schuman, M. C., and Wu, J. (2015). MAPK signaling: a key element in plant defense response to insects. Insect Sci. 22, 157–164. doi: 10.1111/1744-7917.12128
Honma, M., and Shimomura, T. (1978). Metabolism of 1-aminocyclopropane-1-carboxylic acid. Agric. Biol. Chem. 42, 1825–1831. doi: 10.1080/00021369.1978.10863261
Hsieh, Y. J., and Wanner, B. L. (2010). Global regulation by the seven-component Pi signaling system. Curr. Opinion Microbiol. 13, 198–203. doi: 10.1016/j.mib.2010.01.014
Hu, Y., Jiao, J., Liu, L. X., Sun, Y. W., Chen, W. F., Sui, X. H., et al. (2018). Evidence for phosphate starvation of rhizobia without terminal differentiation in legume nodules. Mol. Plant Microbe Interact. 31, 1060–1068. doi: 10.1094/MPMI-02-18-0031-R
Huang, W. K., Ji, H. L., Gheysen, G., and Kyndt, T. (2016). Thiamine-induced priming against root-knot nematode infection in rice involves lignification and hydrogen peroxide generation. Mol. Plant Pathol. 17, 614–624. doi: 10.1111/mpp.12316
Hungria, M., and Kaschuk, G. (2014). Regulation of N2 fixation and NO3/ assimilation in nodulated and N-fertilized Phaseolus vulgaris L. exposed to high temperature stress. Environ. Exp. Bot. 98, 32–39. doi: 10.1016/j.envexpbot.2013.10.010
Ibny, F. Y. I., Jaiswal, S. K., Mohammed, M., and Dakora, F. D. (2019). Symbiotic effectiveness and ecologically adaptive traits of native rhizobial symbionts of Bambara groundnut (Vigna subterranea L. Verdc.) in Africa and their relationship with phylogeny. Sci. Rep. 9:12666. doi: 10.1038/s41598-019-48944-1
Jacobson, C. B., Pasternak, J. J., and Glick, B. R. (1994). Partial purification and characterization of ACC deaminase from the plant growth-promoting rhizobacterium Pseudomonas putida GR12-2. Can. J. Microbiol. 40, 1019–1025. doi: 10.1139/m94-162
Jaiswal, S. K., Naamala, J., and Dakora, F. D. (2018). Nature and mechanisms of aluminium toxicity, tolerance and amelioration in symbiotic legumes and rhizobia. Biol. Fertil. Soils 54, 1–10. doi: 10.1007/s00374-018-1262-0
Jung, S. (2004). Effect of chlorophyll reduction in Arabidopsis thaliana by methyl jasmonate or norflurazon on antioxidant systems. Plant Physiol. Biochem. 42, 225–231. doi: 10.1016/j.plaphy.2004.01.001
Kaiser, C., Kilburn, M. R., Clode, P. L., Fuchslueger, L., Koranda, M., Cliff, J. B., et al. (2015). Exploring the transfer of recent plant photosynthates to soil microbes: mycorrhizal pathway vs direct root exudation. New Phytologist 205, 1537–1551. doi: 10.1111/nph.13138
Kanazawa, H., Ozaki, S., Doi, Y., Masuo, S., and Takaya, N. (2020). Symbiotic riboflavin degradation by Microbacterium and Nocardioides bacteria. Biosci. Biotechnol. Biochem. 84, 1056–1061. doi: 10.1080/09168451.2020.1715783
Katiyar, V., and Goel, R. (2004). Siderophore mediated plant growth promotion at low temperature by mutant of fluorescent pseudomonad? Plant Growth Regul. 42, 239–244. doi: 10.1023/B:GROW.0000026477.10681.d2
Khan, W., Prithiviraj, B., and Smith, D. L. (2008). Nod factor [Nod Bj V (C18: 1, MeFuc)] and lumichrome enhance photosynthesis and growth of corn and soybean. J. Plant Physiol. 165, 1342–1351. doi: 10.1016/j.jplph.2007.11.001
Kidaj, D., Wielbo, J., and Skorupska, A. (2012). Nod factors stimulate seed germination and promote growth and nodulation of pea and vetch under competitive conditions. Microbiol. Res. 167, 144–150. doi: 10.1016/j.micres.2011.06.001
Kong, Z., Glick, B. R., Duan, J., Ding, S., Tian, J., McConkey, B. J., et al. (2015). Effects of 1- aminocyclopropane-1-carboxylate (ACC) deaminase-overproducing Sinorhizobium meliloti on plant growth and copper tolerance of Medicago lupulina. Plant Soil 391, 383–398. doi: 10.1007/s11104-015-2434-4
Lara, M., Cullimore, J. V., Lea, P. J., Miflin, B. J., Johnston, A. W. B., and Lamb, J. W. (1983). Appearance of a novel form of plant glutamine synthetase during nodule development in Phaseolus vulgaris L. Planta 157, 254–258. doi: 10.1007/BF00405190
Lebrazi, S., and Fikri-Benbrahim, K. (2018). “Rhizobium-legume symbioses: heavy metal effects and principal approaches for bioremediation of contaminated soil,” in Legumes for Soil Health and Sustainable Management, eds R. S. Meena, A. D. G. S. Yadav, and R. Lal (Singapore: Springer), 539. doi: 10.1007/978-981-13-0253-4
Lee, K. H., and LaRue, T. A. (1992). Exogenous ethylene inhibits nodulation of Pisum sativum L. cv sparkle. Plant Physiol. 100, 1759–1763. doi: 10.1104/pp.100.4.1759
Li, J., Ovakim, D. H., Charles, T. C., and Glick, B. R. (2000). An ACC deaminase minus mutant of Enterobacter cloacae UW4 no longer promotes root elongation. Curr. Microbiol. 41, 101–105. doi: 10.1007/s002840010101
Ligero, F., Lluch, C., and Olivares, J. (1986). Evolution of ethylene from roots of Medicago sativa plants inoculated with Rhizobium meliloti. J. Plant Physiol. 125, 361–365. doi: 10.1016/S0176-1617(86)80158-4
Lohar, D., Stiller, J., Kam, J., Stacey, G., and Gresshoff, P. M. (2009). Ethylene insensitivity conferred by a mutated Arabidopsis ethylene receptor gene alters nodulation in transgenic Lotus japonicus. Ann. Bot. 104, 277–285. doi: 10.1093/aob/mcp132
LPWG (2017). A new subfamily classification of the Leguminosae based on a taxonomically comprehensive phylogeny. Taxon 66, 44–77. doi: 10.12705/661.3
Lynch, D., O'Brien, J., Welch, T., Clarke, P., ÓCuiv, P., Crosa, J. H., et al. (2001). Genetic organization of the region encoding regulation, biosynthesis, and transport of rhizobactin 1021, a siderophore produced by Sinorhizobium meliloti. J. Bacteriol. 183, 2576–2585. doi: 10.1128/JB.183.8.2576-2585.2001
Ma, W., Charles, T. C., and Glick, B. R. (2004). Expression of an exogenous 1-aminocyclopropane-1-carboxylate deaminase gene in Sinorhizobium meliloti increases its ability to nodulate alfalfa. Appl. Environ. Microbiol. 70, 5891–5897. doi: 10.1128/AEM.70.10.5891-5897.2004
Ma, W., Guinel, F. C., and Glick, B. R. (2003). Rhizobium leguminosarum biovar viciae 1-aminocyclopropane-1-carboxylate deaminase promotes nodulation of pea plants. Appl. Environ. Microbiol. 69, 4396–4402. doi: 10.1128/AEM.69.8.4396-4402.2003
Mabood, F., and Smith, D. L. (2005). Pre-incubation of Bradyrhizobium japonicum with jasmonates accelerates nodulation and nitrogen fixation in soybean (Glycine max) at optimal and suboptimal root zone temperatures. Physiol. Plant 125, 311–323. doi: 10.1111/j.1399-3054.2005.00559.x
Maier, R. J., Hanus, F. J., and Evans, H. J. (1979). Regulation of hydrogenase in Rhizobium japonicum. J. Bacteriol. 137, 825–829. doi: 10.1128/JB.137.2.825-829.1979
Mandal, S., Mandal, M., Das, A., Pati, B., and Ghosh, A. (2009). Stimulation of indoleacetic acid production in a Rhizobium isolate of Vigna mungo by root nodule phenolic acids. Arch. Microbiol. 191, 389–393. doi: 10.1007/s00203-008-0455-6
Mandal, S. M., Mondal, K. C., Dey, S., and Pati, B. R. (2007). Optimization of cultural and nutritional conditions for indole-3-acetic acid (IAA) production by a Rhizobium sp. isolated from root nodules of Vigna mungo (L.) Hepper. Res. J. Microbiol. 2, 239–246. doi: 10.3923/jm.2007.239.246
Marra, L. M., Soares, C. R. F. S., de Oliveira, S. M., Ferreira, P. A. A., Soares, B. L., de Fráguas Carvalho, R., et al. (2012). Biological nitrogen fixation and phosphate solubilization by bacteria isolated from tropical soils. Plant Soil. 357, 289–307. doi: 10.1007/s11104-012-1157-z
Matiru, V. N., and Dakora, F. D. (2005). Xylem transport and shoot accumulation of lumichrome, a newly recognized rhizobial signal, alters root respiration, stomatal conductance, leaf transpiration and photosynthetic rates in legumes and cereals. New Phytol. 165, 847–855. doi: 10.1111/j.1469-8137.2004.01254.x
McGuiness, P. N., Reid, J. B., and Foo, E. (2019). The role of gibberellins and brassinosteroids in nodulation and arbuscular mycorrhizal associations. Front. Plant Sci. 10:269. doi: 10.3389/fpls.2019.00269
McRose, D. L., Baars, O., Seyedsayamdost, M. R., and Morel, F. M. (2018). Quorum sensing and iron regulate a two-for-one siderophore gene cluster in Vibrio harveyi. Proc. Nat. Acad. Sci. U.S.A. 115, 7581–7586. doi: 10.1073/pnas.1805791115
Mehboob, I., Naveed, M., and Zahir, Z. A. (2009). Rhizobial association with non-legumes: mechanisms and applications. Critic. Rev. Plant Sci. 28, 432–456. doi: 10.1080/07352680903187753
Mohale, K. C., Belane, A. K., and Dakora, F. D. (2014). Symbiotic N nutrition, C assimilation, and plant water use efficiency in Bambara groundnut (Vigna subterranea L. Verdc) grown in farmers' fields in South Africa, measured using 15N and 13C natural abundance. Boil. Fertil. Soils 50, 307–319. doi: 10.1007/s00374-013-0841-3
Mohammed, M., Jaiswal, S. K., and Dakora, F. D. (2018). Distribution and correlation between phylogeny and functional traits of cowpea (Vigna unguiculata L. Walp.)-nodulating microsymbionts from Ghana and South Africa. Sci. Rep. 8:18006. doi: 10.1038/s41598-018-36324-0
Mouazen, A. M., and Kuang, B. (2016). On-line visible and near infrared spectroscopy for in-field phosphorous management. Soil Till. Res. 155, 471–477. doi: 10.1016/j.still.2015.04.003
Nascimento, F. X., Rossi, M. J., and Glick, B. R. (2018). Ethylene and 1-Aminocyclopropane-1-carboxylate (ACC) in plant–bacterial interactions. Front. Plant Sci. 9:114. doi: 10.3389/fpls.2018.00114
Nascimento, F. X., Tavares, M. J., Franck, J., Ali, S., Glick, B. R., and Rossi, M. J. (2019). ACC deaminase plays a major role in Pseudomonas fluorescens YsS6 ability to promote the nodulation of alpha-and betaproteobacteria rhizobial strains. Arch. Microbiol. 201, 817–822. doi: 10.1007/s00203-019-01649-5
Nukui, N., Minamisawa, K., Ayabe, S.-I., and Aoki, T. (2006). Expression of the 1-aminocyclopropane-1-carboxylic acid deaminase gene requires symbiotic nitrogen-fixing regulator gene nifA2 in Mesorhizobium loti MAFF303099. Appl. Environ. Microbiol. 72, 4964–4969. doi: 10.1128/AEM.02745-05
Okubo, T., Fukushima, S., and Minamisawa, K. (2012). Evolution of Bradyrhizobium–Aeschynomene mutualism: living testimony of the ancient world or highly evolved state?. Plant Cell Physiol. 53, 2000–2007. doi: 10.1093/pcp/pcs150
Oldroyd, G. E., Murray, J. D., Poole, P. S., and Downie, J. A. (2011). The rules of engagement in the legume-rhizobial symbiosis. Annu. Rev. Genet. 45, 119–144. doi: 10.1146/annurev-genet-110410-132549
Oldroyd, G. E. D., Engstrom, E. M., and Long, S. R. (2001). Ethylene inhibits the Nod factor signal transduction pathway of Medicago truncatula. Plant Cell 13, 1835–1849. doi: 10.1105/tpc.13.8.1835
Palacios, O. A., Bashan, Y., and de-Bashan, L. E. (2014). Proven and potential involvement of vitamins in interactions of plants with plant growth-promoting bacteria—an overview. Biol. Fertil. Soils 50, 415–432. doi: 10.1007/s00374-013-0894-3
Papadopoulou, K., Roussis, A., Kuin, H, and Katinakis, P. (1995). Expression pattern of uricase II gene during root nodule development in Phaseolus vulgaris. Experientia 51, 90–94. doi: 10.1007/BF01964927
Paudyal, S. P., Aryal, R. R., Chauhan, S. V. S., and Maheshwari, D. K. (2007). Effect of heavy metals on growth of Rhizobium strains and symbiotic efficiency of two species of tropical legumes. Sci. World 5, 27–32. doi: 10.3126/sw.v5i5.2652
Penrose, D. M., and Glick, B. R. (2003). Methods for isolating and characterizing ACC deaminase-containing plant growth-promoting rhizobacteria. Physiol. Plant 118, 10–15. doi: 10.1034/j.1399-3054.2003.00086.x
Peters, N. K., and Crist-Estes, D. K. (1989). Nodule formation is stimulated by the ethylene inhibitor aminoethoxyvinylglycine. Plant Physiol. 91, 690–693. doi: 10.1104/pp.91.2.690
Phillips, D. A., Joseph, C. M., Yang, G. P., Martínez-Romero, E., Sanborn, J. R., and Volpin, H. (1999). Identification of lumichrome as a Sinorhizobium enhancer of alfalfa root respiration and shoot growth. Proc. Natl. Acad. Sci. U.S.A. 96, 12275–12280. doi: 10.1073/pnas.96.22.12275
Pholo, M., Coetzee, B., Maree, H. J., Young, P. R., Lloyd, J. R., Kossmann, J., et al. (2018). Cell division and turgor mediate enhanced plant growth in Arabidopsis plants treated with the bacterial signalling molecule lumichrome. Planta 248, 477–488. doi: 10.1007/s00425-018-2916-8
Pieterse, C. M., Van der Does, D., Zamioudis, C., Leon-Reyes, A., and Van Wees, S. C. (2012). Hormonal modulation of plant immunity. Annu. Rev. Cell Dev. Biol. 28, 489–521. doi: 10.1146/annurev-cellbio-092910-154055
Pii, Y., Crimi, M., Cremonese, G., Spena, A., and Pandolfini, T. (2007). Auxin and nitric oxide control indeterminate nodule formation. BMC Plant Biol. 7:21. doi: 10.1186/1471-2229-7-1
Ping, L., and Boland, W. (2004). Signals from the underground: bacterial volatiles promote growth. Arab. Trends Plant Sci. 9, 263–266. doi: 10.1016/j.tplants.2004.04.008
Prithiviraj, B., Zhou, X., Souleimanov, A., Kahn, W., and Smith, D. (2003). A host-specific bacteria-to-plant signal molecule (Nod factor) enhances germination and early growth of diverse crop plants. Planta 216, 437–445. doi: 10.1007/s00425-002-0928-9
Rajkumar, M., Ae, N., Prasad, M. N. V., and Freitas, H. (2010). Potential of siderophore-producing bacteria for improving heavy metal phytoextraction. Trends Biotechnol. 28, 142–149. doi: 10.1016/j.tibtech.2009.12.002
Richardson, A. E., Lynch, J. P., Ryan, P. R., Delhaize, E., Smith, F. A., Smith, S. E., et al. (2011). Plant and microbial strategies to improve the phosphorus efficiency of agriculture. Plant Soil 349, 121–156. doi: 10.1007/s11104-011-0950-4
Richardson, A. E., and Simpson, R. J. (2011). Soil microorganisms mediating phosphorus availability update on microbial phosphorus. Plant Physiol. 156, 989–996. doi: 10.1104/pp.111.175448
Rodriguez, H., and Fraga, R. (1999). Phosphate solubilizing bacteria and their role in plant growth promotion. Biotechnol. Adv. 17, 319–339. doi: 10.1016/S0734-9750(99)00014-2
Rodríguez, H., Fraga, R., Gonzalez, T., and Bashan, Y. (2006). Genetics of phosphate solubilization and its potential applications for improving plant growth-promoting bacteria. Plant Soil. 287, 15–21. doi: 10.1007/s11104-006-9056-9
Rodriguez, H., Gonzalez, T., and Selman, G. (2000). Expression of a mineral phosphate solubilizing gene from Erwinia herbicola in two rhizobacterial strains. J. Biotechnol. 84, 155–161. doi: 10.1016/S0168-1656(00)00347-3
Rungin, S., Indananda, C., Suttiviriya, P., Kruasuwan, W., Jaemsaeng, R., and Thamchaipenet, A. (2012). Plant growth enhancing effects by a siderophore-producing endophytic streptomycete isolated from a Thai jasmine rice plant (Oryza sativa L. cv. KDML105). Antonie Van Leeuwenhoek 102, 463–472. doi: 10.1007/s10482-012-9778-z
Saleem, M., Arshad, M., Hussain, S., and Bhatti, A. S. (2007). Perspective of plant growth promoting rhizobacteria (PGPR) containing ACC deaminase in stress agriculture. J. Ind. Microbiol. Biot. 34, 635–648. doi: 10.1007/s10295-007-0240-6
Santino, A., Taurino, M., De Domenico, S., Bonsegna, S., Poltronieri, P., Pastor, V., et al. (2013). Jasmonate signaling in plant development and defense response to multiple (a) biotic stresses. Plant Cell Rep. 32, 1085–1098. doi: 10.1007/s00299-013-1441-2
Schalk, I. J., Hannauer, M., and Braud, A. (2011). New roles for bacterial siderophores in metal transport and tolerance. Environ. Microbiol. 13, 2844–2854. doi: 10.1111/j.1462-2920.2011.02556.x
Serova, T. A., Tsyganova, A. V., and Tsyganov, V. E. (2018). Early nodule senescence is activated in symbiotic mutants of pea (Pisum sativum L.) forming ineffective nodules blocked at different nodule developmental stages. Protoplasma 255, 1443–1459. doi: 10.1007/s00709-018-1246-9
Shaharoona, B., Arshad, M., and Zahir, Z. A. (2006). Effect of plant growth promoting rhizobacteria containing ACC-deaminase on maize (Zea mays L.) growth under axenic conditions and on nodulation in mung bean (Vigna radiata L.). Lett. Appl. Microbiol. 42, 155–159. doi: 10.1111/j.1472-765X.2005.01827.x
Shokri, D., and Emtiazi, G. (2010). Indole-3-acetic acid (IAA) production in symbiotic and non-symbiotic nitrogen-fixing bacteria and its optimization by Taguchi design. Curr. Microbiol. 61, 217–225. doi: 10.1007/s00284-010-9600-y
Siqueira, A. F., Ormeño-Orrillo, E., Souza, R. C., Rodrigues, E. P., Almeida, L. G. P., Barcellos, F. G., et al. (2014). Comparative genomics of Bradyrhizobium japonicum CPAC 15 and Bradyrhizobium diazoefficiens CPAC 7: elite model strains for understanding symbiotic performance with soybean. BMC Genomics 15:420. doi: 10.1186/1471-2164-15-420
Spaepen, S., Dobbelaere, S., Croonenborghs, A., and Vanderleyden, J. (2008). Effects of Azospirillum brasilense indole-3-acetic acid production on inoculated wheat plants. Plant Soil 312, 15–23. doi: 10.1007/s11104-008-9560-1
Spaink, H. P. (1997). Ethylene as a regulator of Rhizobium infection. Trends Plant Sci. 6, 203–204. doi: 10.1016/S1360-1385(97)01042-X
Sprent, J. I., Ardley, J., and James, E. K. (2017). Biogeography of nodulated legumes and their nitrogen-fixing symbionts. New Phytol. 215, 40–56. doi: 10.1111/nph.14474
Stajković, O., Delić, D., Jošić, D., Kuzmanović, D., Rasulić, N., and KneŽević-Vukčević, J. (2011). Improvement of common bean growth by co-inoculation with Rhizobium and plant growth-promoting bacteria. Rom. Biotechnol. Lett. 16, 5919–5926. Available online at: https://e-repository.org/rbl/vol.16/iss.1/11.pdf
Sturtevant, D. B., and Taller, B. J. (1989). Cytokinin production by Bradyrhizobium japonicum. Plant Physiol. 89, 1247–1252. doi: 10.1104/pp.89.4.1247
Suárez, R., Wong, A., Ramírez, M., Barraza, A., Orozco Mdel, C., Cevallos, M. A., et al. (2008). Improvement of drought tolerance and grain yield in common bean by overexpressing trehalose-6-phosphate synthase in rhizobia. Mol. Plant Microbe Interact. 21, 958–966. doi: 10.1094/MPMI-21-7-0958
Subramanian, S., Hu, X., Lu, G., Odelland, J. T., and Yu, O. (2004). The promoters of two isoflavone synthase genes respond differentially to nodulation and defense signals in transgenic soybean roots. Plant Mol. Biol. 54, 623–639. doi: 10.1023/B:PLAN.0000040814.28507.35
Tamimi, S. M., and Timko, M. P. (2003). Effects of ethylene and inhibitors of ethylene synthesis and action on nodulation in common bean (Phaseolus vulgaris L.). Plant Soil 257, 125–131. doi: 10.1023/A:1026280517660
Tavares, M. J., Nascimento, F. X., Glick, B. R., and Rossi, M. J. (2018). The expression of an exogenous ACC deaminase by the endophyte Serratia grimesii BXF1 promotes the early nodulation and growth of common bean. Lett. Appl. Microbiol. 66, 252–259. doi: 10.1111/lam.12847
Teale, W. D., Paponov, I. A., and Palme, K. (2006). Auxin in action: signalling, transport and the control of plant growth and development. Nat. Rev. Mol. Cell Biol. 7, 847–859. doi: 10.1038/nrm2020
Tsikou, D., Kalloniati, C., Fotelli, M. N., Nikolopoulos, D., Katinakis, P., Udvardi, M. K., et al. (2013). Cessation of photosynthesis in Lotus japonicus leaves leads to reprogramming of nodule metabolism. J. Exp. Bot. 64, 1317–1332. doi: 10.1093/jxb/ert015
Udvardi, M. K., and Kahn, M. L. (1992). Review article evolution of the (Brady) rhizobium-legume symbiosis: why do bacteroids fix nitrogen? Symbiosis 14, 87–101.
Ulzen, J., Abaidoo, R. C., Mensah, N. E., Masso, C., and Abdel Gadir, A. H. (2016). Bradyrhizobium inoculants enhance grain yields of soybean and cowpea in Northern Ghana. Front. Plant Sci. 7:1770. doi: 10.3389/fpls.2016.01770
Vaccari, D. A. (2009). Phosphorus: a looming crisis. Sci. Am. 300, 54–59. doi: 10.1038/scientificamerican0609-54
Vassilev, N., Vassileva, M., Fenice, M., and Federici, F. (2001). Immobilized cell technology applied in solubilization of insoluble inorganic (rock) phosphates and P plant acquisition. Biores. Technol. 79, 263–271. doi: 10.1016/S0960-8524(01)00017-7
Via, V. D., Zanetti, M. E., and Blanco, F. (2016). How legumes recognize rhizobia. Plant Signal. Behav. 11:e1120396. doi: 10.1080/15592324.2015.1120396
Wang, E. T., Tian, C. F., Chen, W. F., Young, J. P. W., and Chen, W. X. (2019a). Ecology and Evolution of Rhizobia: Principles and Applications. Singapore: Springer Nature. doi: 10.1007/978-981-32-9555-1
Wang, J., Wu, D., Wang, Y., and Xie, D. (2019b). Jasmonate action in plant defense against insects. J. Exp. Bot. 70, 3391–3400. doi: 10.1093/jxb/erz174
Yadav, A., Singh, R. P., Singh, A. L., and Singh, M. (2020). Identification of genes involved in phosphate solubilization and drought stress tolerance in chickpea symbiont Mesorhizobium ciceri Ca181. Arch. Microbiol. 1–8. doi: 10.1007/s00203-020-02109-1
Yang, G., Bhuvaneswari, T. V., Joseph, C. M., King, M. D., and Phillips, D. A. (2002). Roles for riboflavin in the Sinorhizobium-alfalfa association. Mol. Plant Microbe Interact. 15, 456–462. doi: 10.1094/MPMI.2002.15.5.456
Yuan, Z. C., Zaheer, R., and Finan, T. M. (2006). Regulation and properties of PstSCAB, a high-affinity, high-velocity phosphate transport system of Sinorhizobium meliloti. J. Bacteriol. 188, 1089–1102. doi: 10.1128/JB.188.3.1089-1102.2006
Zhang, S., Yang, X., Sun, M., Sun, F., Deng, S., and Dong, H. (2009). Riboflavin-induced priming for pathogen defense in Arabidopsis thaliana. J. Integr. Plant Biol. 51, 167–174. doi: 10.1111/j.1744-7909.2008.00763.x
Zúñiga, A., Poupin, M. J., Donoso, R., Ledger, T., Guiliani, N., Gutiérrez, R. A., et al. (2013). Quorum sensing and indole-3-acetic acid degradation play a role in colonization and plant growth promotion of Arabidopsis thaliana by Burkholderia phytofirmans PsJN. Mol. Plant Microbe Interact. 26, 546–553. doi: 10.1094/MPMI-10-12-0241-R
Keywords: rhizobia, legume, PGPR, symbiosis, sustainable agriculture, siderophores, indole-3-acetic acid
Citation: Jaiswal SK, Mohammed M, Ibny FYI and Dakora FD (2021) Rhizobia as a Source of Plant Growth-Promoting Molecules: Potential Applications and Possible Operational Mechanisms. Front. Sustain. Food Syst. 4:619676. doi: 10.3389/fsufs.2020.619676
Received: 20 October 2020; Accepted: 31 December 2020;
Published: 27 January 2021.
Edited by:
Everlon Cid Rigobelo, Universidade Estadual Paulista, BrazilReviewed by:
Chang Fu Tian, China Agricultural University, ChinaDinesh Kumar Maheshwari, Gurukul Kangri Vishwavidyalaya, India
Copyright © 2021 Jaiswal, Mohammed, Ibny and Dakora. This is an open-access article distributed under the terms of the Creative Commons Attribution License (CC BY). The use, distribution or reproduction in other forums is permitted, provided the original author(s) and the copyright owner(s) are credited and that the original publication in this journal is cited, in accordance with accepted academic practice. No use, distribution or reproduction is permitted which does not comply with these terms.
*Correspondence: Felix D. Dakora, ZGFrb3JhZmQmI3gwMDA0MDt0dXQuYWMuemE=; Sanjay K. Jaiswal, c2FuamF5c2lzd2EmI3gwMDA0MDtnbWFpbC5jb20=