- 1Department of Biological Sciences, School of Natural Sciences (SONAS), Masinde Muliro University of Science and Technology, Kakamega, Kenya
- 2Centre for African Medicinal and Nutritional Flora and Fauna, Masinde Muliro University of Science and Technology, Kakamega, Kenya
- 3Department of Biochemistry, College of Health Sciences, University of Nairobi, Nairobi, Kenya
- 4Department of Pure and Applied Chemistry, School of Natural Sciences (SONAS), Masinde Muliro University of Science and Technology, Kakamega, Kenya
- 5Department of Agriculture and Land Use Management, School of Agriculture, Veterinary Sciences and Technology (SAVET), Masinde Muliro University of Science and Technology, Kakamega, Kenya
Rhizobia are bacteria that exhibit both endophytic and free-living lifestyles. Endophytic rhizobial strains are widely known to infect leguminous host plants, while some do infect non-legumes. Infection of leguminous roots often results in the formation of root nodules. Associations between rhizobia and host plants may result in beneficial or non-beneficial effects. Such effects are linked to various biochemical changes that have far-reaching implications on relationships between host plants and the dependent multitrophic biodiversity. This paper explores relationships that exist between rhizobia and various plant species. Emphasis is on nutritional and phytochemical changes that occur in rhizobial host plants, and how such changes affect diverse consumers at different trophic levels. The purpose of this paper is to bring into context various aspects of such interactions that could improve knowledge on the application of rhizobia in different fields. The relevance of rhizobia in sustainable food systems is addressed in context.
Introduction
Rhizobium is a group of bacteria that were first described in the year 1889 by the German botanist Dr. Albert Bernhard Frank (1839-1900) (Hassen et al., 2020). Such bacteria are part of complex microbiomes that exist endophytically in root nodules of leguminous plants (Martínez-Hidalgo and Hirsch, 2017; Hansen et al., 2020). With the advent of modern molecular identification tools (Young and Haukka, 1996; Deng et al., 2011), root nodulating rhizobia have currently been sub-classified into at least 15 genera including the alphaproteobacteria Rhizobium, Ensifer (syn. Sinorhizobium), Allorhizobium, Pararhizobium, Neorhizobium, Shinella (Rhizobiaceae), Mesorhizobium, Aminobacter, Phyllobacterium (Phyllobacteriaceae), Ochrobactrum (Brucellaceae), Methylobacterium, Microvirga (Methylobacteriaceae), Bradyrhizobium (Bradyrhizobiaceae), Azorhizobium (Xanthobacteraceae), and Devosia (Hyphomicrobiaceae), as well as the betaproteobacterial genera Paraburkholderia, Cupriavidus, and Trinickia (Burkholderiaceae) (De Lajudie et al., 2019; Hassen et al., 2020). The term “Rhizobium” has mostly been retained for general reference and as a common name for these genera. However, apart from these root nodulating rhizobia, diverse strains of non-nodulating rhizobia do exist endophytically and in the rhizospheres of various leguminous plants (Wu et al., 2011; Gano-Cohen et al., 2016; Martínez-Hidalgo and Hirsch, 2017).
Symbiotic Rhizobium species associated within root nodule symbiosomes of leguminous plants endophytically fix molecular nitrogen (N2) through reactions catalyzed by nitrogenase enzyme (Flores-Tinoco et al., 2020; Jangir et al., 2020). The biologically fixed nitrogen is assimilated by legumes to meet the nutritional demands especially under N-limited conditions (Basu and Kumar, 2020). In return, the autotroph legumes (macrosymbionts) provide the heterotrophic Rhizobium bacteroids (microsymbionts) with organic carbon for respiration derived from photosynthetic reactions (Mitsch et al., 2018; Flores-Tinoco et al., 2020). Therefore, the root nodule is the point of convergence of two very important reactions namely, biological nitrogen fixation through nitrogenase machinery (Lindström and Mousavi, 2019; Signorelli et al., 2020), and carbon fixation in the photosynthetic machinery (Pinnola and Bassi, 2018; Vanlerberghe et al., 2020) (Figure 1). The union of Rhizobium and leguminous plants through nitrogen-fixing root nodules is an efficient nutrient cycling component in biogeochemical cycles of various ecosystems.
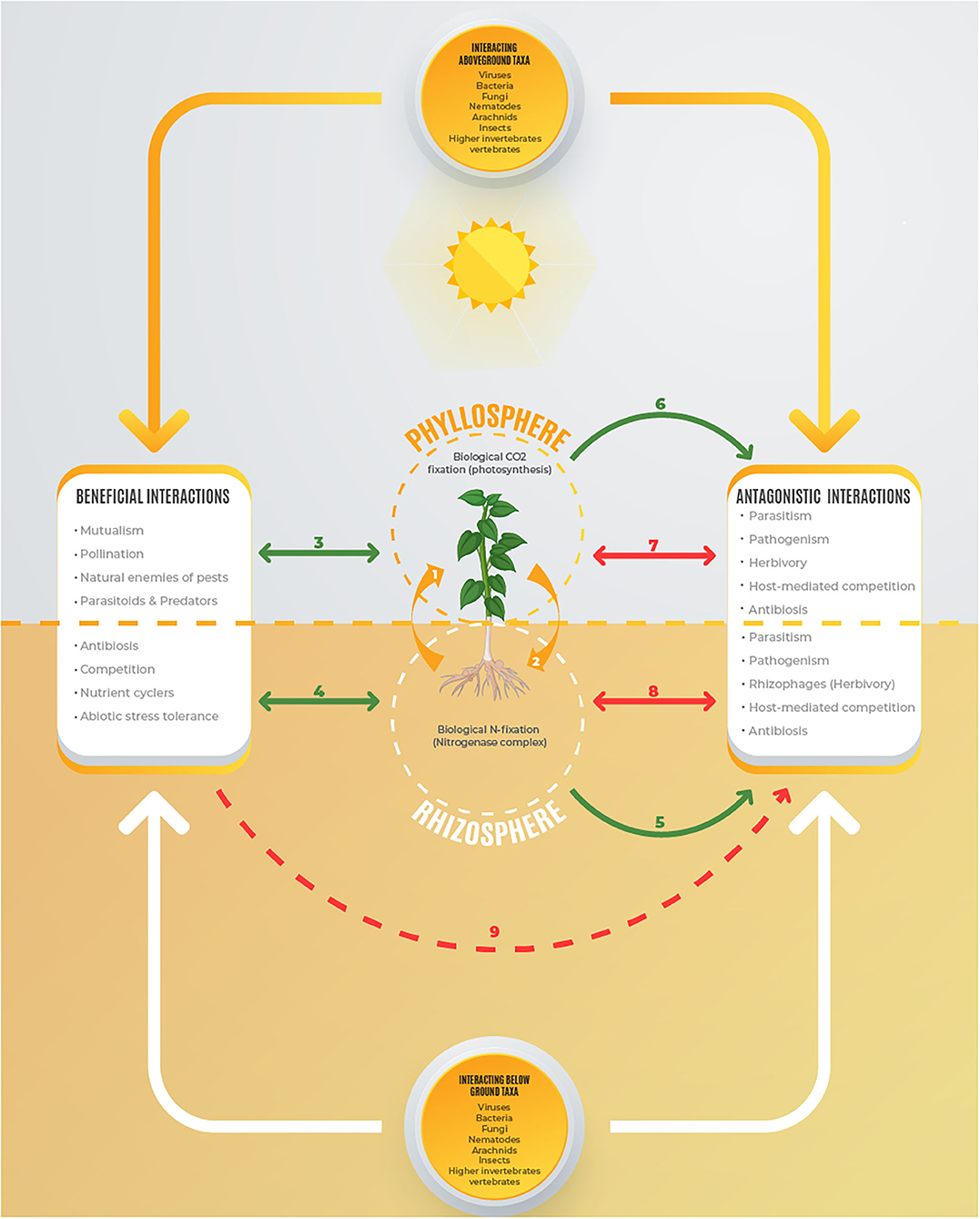
Figure 1. Leguminous plant with Rhizobium-nodulated roots. (1) The aboveground foliar system obtains gaseous CO2 from the phyllosphere and fixes it into organic compounds through photosynthetic machinery and translocate them to the heterotrophic rhizobia in the root nodules; (2) Rhizobium obtains nitrogen from the rhizosphere and fixes it into amino acids that are transmitted to the leguminous leaves for protein and chlorophyll synthesis; (3, 4) Beneficial mutualistic interactions between rhizobial plants with aboveground and belowground taxa with the autotroph providing organic carbon derived from photosynthesis and nitrogen fixation. (5, 6) The rhizobia plants providing food to antagonistic organisms under a situation of warfare (7, 8) and (9) beneficial biota help the rhizobial plant to suppress the antagonistic organisms.
The rhizobium-legume symbiosis supports aboveground and belowground networks of consumers at various trophic levels (Grunseich et al., 2020; Karoney et al., 2020). By fixing nitrogen, Rhizobium redefines autotrophy in leguminous plants and their interactions with various consumers. This is because root infection by rhizobia triggers variations in chemical composition of host plants that may be expressed in gaseous forms such as volatile organic compounds (VOCs) (Ballhorn et al., 2013), aqueous form including cellular fluids and root exudates (Karoney et al., 2020; Tian et al., 2020), as well as solid forms like cell walls (Fournier et al., 2015; Gigli-Bisceglia et al., 2020). Such chemical changes are linked with suitability of legumes as host plants in terms of nutrient content that promotes tolerance (Karoney et al., 2020), or expression of defensive compounds in terms of antibiosis and antixenosis (Cai et al., 2017). Rhizobium-legume symbiosis therefore determines biodiversity and the function of various ecosystems including drylands, wetlands, savannahs, tropical rain forests, and the human-managed agroecosystems.
Currently, the world is struggling to meet food demand for a fast-growing human population projected to reach 9.7 billion by the year 2050 (UN DESA, 2017). Global demand for protein-rich food sources including legumes for human food and animal feed will continue rising (Foyer et al., 2016). Intensification of food production to meet the rising food demand is associated with depletion of soil fertility (Kopittke et al., 2019), which results in the misuse of fertilizers and pesticides (Warra and Prasad, 2020), that serves the unsustainable goal of eliminating competition from pests and pathogens (Karoney et al., 2020), while trying to attain dominance in food markets (Gonzalez, 1999; Chalam et al., 2020). The result of such unsustainable practices is increased costs of production (Adolph et al., 2020), amidst a resource depleted and polluted agroecosystem that is toxic to human health and biodiversity (Mahmud et al., 2020; Warra and Prasad, 2020). These are among the reasons behind the constitution of the United Nations Sustainable Development Goals (SDGs), and in particular, to end hunger, achieve food security and improved nutrition, and promote sustainable agriculture (SDG2) (ICSU, 2017).
Rhizobia and their leguminous host plants are crucial components in attaining the SDGs through nitrogen and carbon dioxide fixation in agroecosystems (Saha and Bauddh, 2020). In-depth understanding of rhizobium-legume symbioses, especially the phytochemical mechanisms and changes associated with the nitrogenase-photosynthesis reactions, lays better ground for addressing some important issues regarding Sustainable Food Systems in agroecosystems. The present article focuses on symbiotic relationships between root nodulating Rhizobium species and leguminous plants in biological nitrogen fixation. Phytochemical changes associated with root infection by rhizobia, and how such changes affect various microbial and invertebrate consumers have been addressed in multitrophic contexts. Such interactions have been put into the perspective of Sustainable Food Systems in agroecosystems.
Carbon and Nitrogen Acquisition by Plants
Photosynthetic Machinery and Carbon Fixation in Host Plants
Plants are autotrophs equipped with two photosynthetic machineries in the thylakoid membrane inside the chloroplasts (Nelson and Junge, 2015), with the light reaction occurring in the grana of chloroplasts producing energy molecules (Mullineaux, 2005), and the dark reaction located in the stroma of chloroplasts (Poolman et al., 2003). The light reaction in the grana has two systems known as Photosystem II (PSII) (water-plastoquinone oxidoreductase) (Wydrzynski and Satoh, 2005; Freeman, 2006) and Photosystem I (PSI) (plastocyanin-ferredoxin oxidoreductase) (Golbeck, 2006). Photosystem I is located on the outer surface of the thylakoid membrane with a reaction center called P700 that absorbs light at 700 nm to reduce NADP+ to NADPH (Webber and Lubitz, 2001). Photosystem II (PSII) is located on the inner surface of the thylakoid membrane with a reaction center called P680 that absorbs light energy at 680 nm to provide energy for photo-splitting of water molecules into protons (H+) and oxygen gas (O2) (Renger and Renger, 2008; Herbstová et al., 2012). Electrons produced in PSII are used to replace those donated by PSI to reduce NADP+ into NADPH (Haldrup et al., 2001; Roach and Krieger-Liszkay, 2014). During photosynthetic reactions, ATP is synthesized from ADP and inorganic P through the process of phosphorylation (Jagendorf, 2002), which can either be cyclic phosphorylation involving only PSI (Allen, 2003; Johnson, 2011), or non-cyclic photophosphorylation involving both PSI and PSII that reduces NADP+ and O2− to NADPH and O2, respectively (Shimazaki and Zeiger, 1985; Allen, 2003).
The ATP and NADPH produced in the light reaction provide energy for the dark (carbon) reaction in the stroma that involves biofixation of carbon dioxide (Hopkins and Hüner, 2009; Buchanan, 2016). Carbon dioxide fixation can either be via the Calvin cycle in C3 plants (Raines, 2011), which includes leguminous hosts of Rhizobium species (Archimède et al., 2011), or the Hatch-Slack pathway for carbon dioxide fixation found in C4 plants (Osborne and Beerling, 2006). Crassulacean acid metabolism (CAM) functions in CAM plants (Males and Griffiths, 2017).
In the carbon reaction of C3 plants (Calvin cycle) that includes leguminous hosts of Rhizobium, CO2 that gets into plants cells via stomata is fixed into ribulose-1,5-diphosphate (RuBP) (C5H12O11P2) under the catalyzing effect of ribulose bisphosphate carboxylase (rubisco) to form two molecules of glyceric acid-3-phosphate (Wang and Lan, 2010). Glyceric acid-3-phosphate reacts with ATP to form two molecules of glyceraldehyde-3-phosphate while releasing ADP (Raines, 2003; Wang and Lan, 2010). Glyceraldehyde-3-phosphate can be synthesized into ribulose-1,5-biphosphate (RuBP) for continuation of the CO2 biofixation in the Calvin cycle (Raines, 2003; Wang and Lan, 2010), or converted into fructose-1,6-bisphosphate as a precursor for biosynthesis of glucose, sucrose, starch, or other energy-rich carbohydrates (Strand et al., 2000; Lv et al., 2017). The carbohydrates are used for generation of energy through the respiratory TCA cycle in the mitochondria (Raghavendra et al., 1994; Plaxton and Podestá, 2006). Besides foliar acquisition of carbon in the form of CO2 for photosynthesis, plants also do acquire carbon in the forms like CO2, carbonate and organic compounds through their roots (Raven et al., 1988; Farrar and Jones, 2000).
Nitrogen Acquisition in Host Plants
Plants require nitrogen to synthesize proteins and other complex compounds that are very important for their growth and reproduction. Nitrogen is key for the synthesis of chlorophyll and the function of photosystem I and II (Lu et al., 2001; Bassi et al., 2018). For instance, glutamate (C5H9NO4) is a nitrogenous compound that is a precursor for chlorophyll synthesis in developing leaves (Forde and Lea, 2007). Glutamate is the key compound involved in the acquisition of nitrogen by plants (Temple et al., 1998). This compound is primarily biosynthesized from pyruvate generated from the glycolytic pathway (Chesworth et al., 1998), through the breakdown of photosynthates arising from the Calvin cycle (Michelet et al., 2013). Pyruvate (C3H4O3) is converted to 2-oxoglutarate (α-ketoglutarate) through the action of glutamate dehydrogenase (GDH) in the tricarboxylic acid (TCA) cycle (Qiu et al., 2019). Glutamate is also synthesized through the proline (Pro)/pyrroline 5-carboxylate (P5C) cycle in the plant cytoplasm (Miller et al., 2009; Qiu et al., 2019). Glutamate is then used in nitrogen acquisition systems.
Plants have two nitrogen acquisition systems, the root low-affinity transport system that functions when soil N is adequate, and a high-affinity transport system that functions when N is low (Kraiser et al., 2011; Kiba and Krapp, 2016). Nitrogen is mainly acquired in the form of ammonium () in plants growing under low pH conditions and as nitrate () adapted to high pH conditions (Masclaux-Daubresse et al., 2010). Whereas ammonium is assimilated directly into amino acids, nitrate has to first be reduced to ammonium in a reaction catalyzed by nitrate reductase and nitrite reductase (Chamizo-Ampudia et al., 2017). Nitrogen in the form of ammonium () is assimilated via the glutamate synthase (GS)/glutamine oxoglutarate aminotransferase (GOGAT) synthetase pathway (Masclaux-Daubresse et al., 2006; Zhang Z. et al., 2017). In this pathway, ammonium from soil reacts with glutamate to form glutamine (C5H10N2O3) (Forde and Lea, 2007). Glutamate dehydrogenase (GDH) is another enzyme that catalyzes the incorporation of ammonium into glutamate just like glutamate synthase (Grabowska et al., 2012). However, glutamate dehydrogenase has lower affinity for ammonium than glutamate synthase (Zhang Z. et al., 2017).
When soil nitrogen supply is limited, the biosynthesis and function of PS I and PS II compounds is constrained (Nunes et al., 1993; Bassi et al., 2018), and hence the provision of energy for CO2 fixation into glutamate and other products (Bascuñán-Godoy et al., 2018). Such conditions require alternative ways of acquiring nitrogen. This is whereby diazotrophic Rhizobium species become of significance to the plants by symbiotic nitrogen fixation through nitrogenase-catalyzed reactions.
Acquisition of Carbon and Nitrogen by Rhizobium
Being heterotrophs, free-living rhizobia saprophytically acquire organic carbon and nitrogen in the rhizosphere (Poole et al., 2018). When soil fertility is low and the supply of organic carbon and nitrogen are limited, chances of survival of rhizobia diminish amidst intensifying competition and predation in the rhizosphere (Gabasawa, 2020) (Figure 1). Saprophytic processes that require more investment in breaking down organic compounds are weakened amidst scarcity of substrates. Scarcity of organic carbon and nitrogen in the rhizosphere favor the establishment of endophytic populations of symbiotic rhizobia in root nodules of host plants (Coba de la Peña et al., 2018). Besides providing shelter for rhizobia, root nodules also supply the symbiotic bacteria with organic carbon and other nutrients (Brewin, 2010). Rhizobium in the root nodules fix atmospheric N2 with the help of nitrogenase enzyme. Root nodules are facilitated with leghemoglobin and mitochondria that scavenge for oxygen to provide microaerobic conditions that protect nitrogenase from inhibition by O2 (Bergersen, 1997).
Carbon Acquisition by Endophytic Rhizobium Species
Rhizobia require organic carbon to generate energy in the form of ATP within the bacteroid Tricarboxylic acid cycle (TCA cycle) (Lodwig and Poole, 2003). The TCA cycle of the host plant is the source of organic carbon to rhizobia in root nodules (Andersen, 2020). Endophytic rhizobia acquire carbon from host plants in the form of C4-dicarboxylates (fumarate, malate, and succinate), which can easily penetrate peribacteroid membranes of root nodules (Mitsch et al., 2018). Specifically, L-malate (C4H6O5) is the key C4-dicarboxylate that supplies carbon to symbiotic rhizobia in root nodules (Haaker et al., 1996; Poole and Allaway, 2000; Mitsch et al., 2018). Rhizobial TCA cycle functions aerobically in free-living cells (Maier, 2004), and microaerobically involving anaplerotic enzymatic pathways in endophytic bacteroids (Dunn, 1998). The ATP produced is utilized as the energy molecule for nitrogen fixation by rhizobia (Duval et al., 2013). However, there is growing information on the existence of some photosynthetic rhizobia that colonize nitrogen fixing-stem nodules (Fleischman et al., 1995; Zhang et al., 2019).
Nitrogen Acquisition by Endophytic Rhizobium Species
Endophytic rhizobia acquire dinitrogen molecules (N2) through the gas diffusion pathway in the intercellular air spaces of root nodule cortical cells (Zeng et al., 1989; Hunt and Layzell, 1993). Nitrogen fixation reaction is catalyzed by a group of enzymes known as “nitrogenase” (Hoffman et al., 2009, 2014), which comprises of three main genetically distinct types namely Nif, Vnf, and Anf that either have molybdenum (Mo), vanadium (V), or iron (Fe) as their respective active-site central metals (Zhao et al., 2006; McGlynn et al., 2013). Mo-nitrogenase is found in all N2 fixing bacteria besides being the most widely studied (Newton, 1997; Garcia et al., 2020). Nitrogen is fixed via two distinct biochemical pathways arising from Janus reactions (Harris et al., 2018), with the “distal” (D) pathway being associated with the Chatt-Schrock cycle (Husch and Reiher, 2017), and the “alternating” (A) pathway (Hoffman et al., 2014). In the diazotrophic reaction, nitrogen gas (N2) is reduced to ammonium (NH3) with the supply of energy from ATP and catalyzed by nitrogenase (N2 + 8e− + 16ATP 8H+ → 2NH3 + H2 + 16ADP + 16Pi) (Hoffman et al., 2014; Ghebreamlak and Mansoorabadi, 2020). The resulting ammonium () diffuses into root cells (Patriarca et al., 2002), and assimilated through the glutamate synthetase (GS)/glutamine oxoglutarate aminotransferase (GOGAT) pathway (Zhang Z. et al., 2017; Lea and Miflin, 2018). Other rhizobia infect non-leguminous plants to provide plant growth promoting services through processes that do not rely on nitrogenase-catalyzed reactions (Mehboob et al., 2009).
Phytochemicals in Rhizobium-Legume Symbiosis and Their Influence on Consumers
The photosynthesis-nitrogen fixation machinery of leguminous plants supports complex rhizosphere (belowground) and phyllosphere (aboveground) food webs comprising of organisms from various taxonomic groups (Kempel et al., 2009; Katayama et al., 2011a,b; Zhao et al., 2014; Wu et al., 2017; Karoney et al., 2020) (Figure 1). Such trophic interactions primarily relate to the demand for nutrients, shelter, and reproductive space. Underlying such relationships is energy flow from the sun through complex biochemical reactions that have far-reaching effects, including the formation of subsequent generations of the organisms. Phytochemical composition of leguminous host plants exhibits variations, right from the process of rhizobial infection, multiplication into bacteroids, nitrogen fixation to senescence (Irmer et al., 2015). Such chemical compounds include chlorophylls, enzymes, photosynthates and their nutritional derivatives, plant secondary metabolites, hormones, and other signaling molecules as well as inorganic compounds (Wink, 2013; Sánchez-Chino et al., 2015; Šibul et al., 2016; Karoney et al., 2020). Various published works have linked rhizobium infection to nutritional and phytochemical changes that affect consumers in multitrophic systems (Tables 1, 2). Nutritional suitability of host plants is therefore influenced by rhizobium infection (Naluyange et al., 2014, 2016; Karoney et al., 2020), while prevention of overexploitation by the consumers depends on the expression of such compounds in terms of host plant resistance and tolerance (Enneking and Wink, 2000; Joosten and van Veen, 2011; Goyal et al., 2012; Goyal, 2013; Karoney et al., 2020).
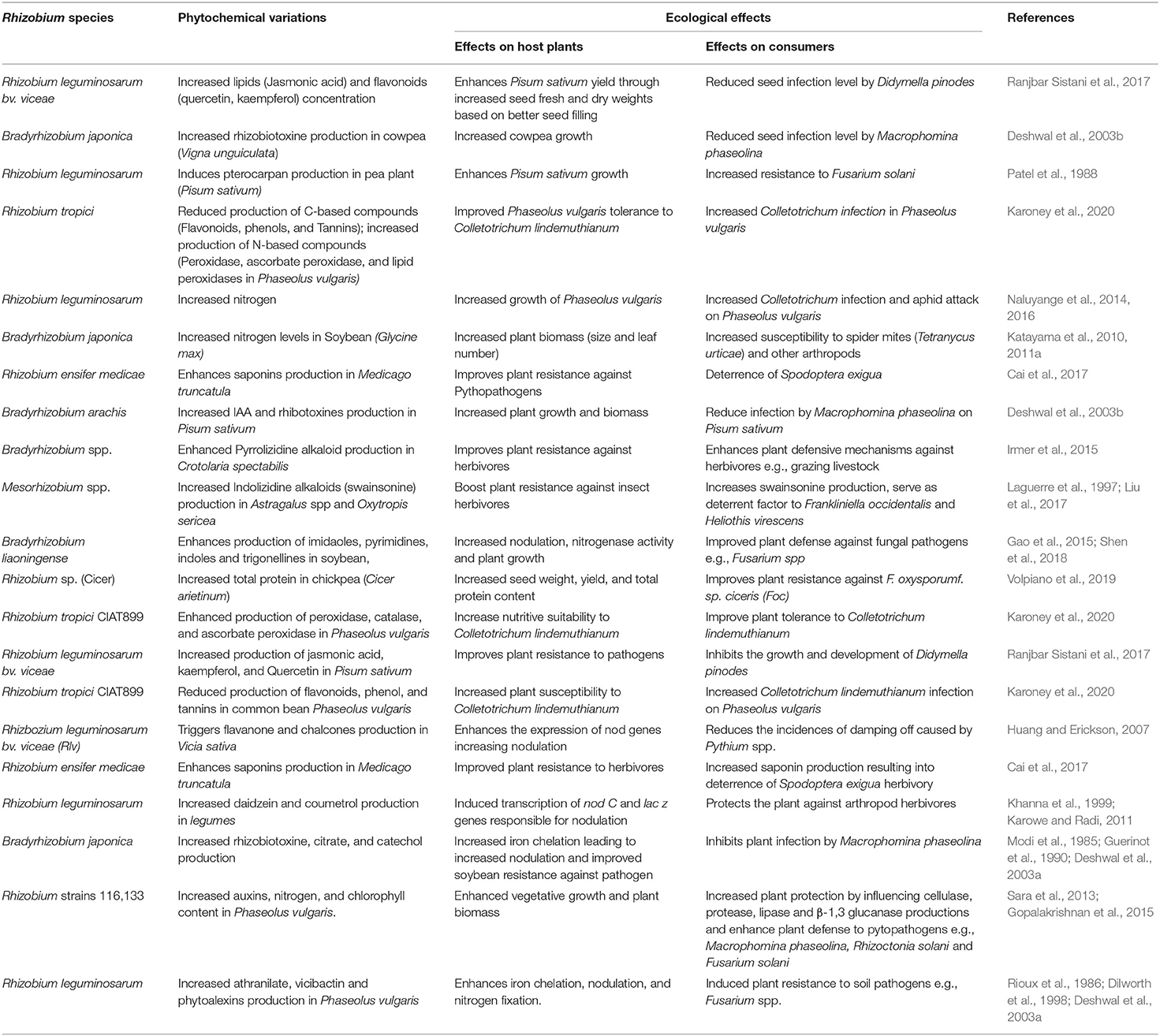
Table 1. Phytochemical variations associated with Rhizobium species and their effects on host plants and herbivorous consumers.
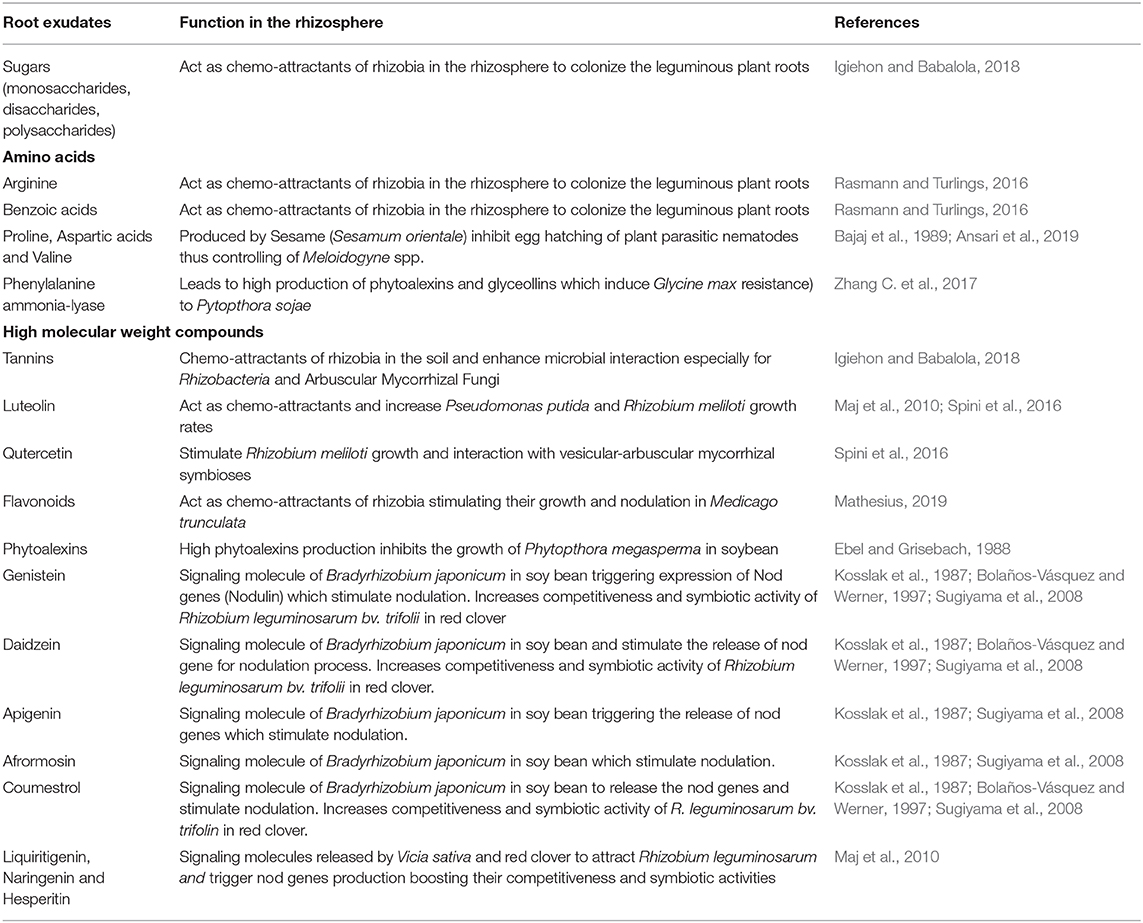
Table 2. Leguminous root exudates and their role in rhizosphere interactions with Rhizobium and other organisms.
Leguminous root nodules are microecosystems that host unique microbiomes consisting of consumers that benefit from resources provided by the photosynthesis-nitrogen fixation machinery. Success of root nodule symbiosis depends on how abiotic and biotic factors that determine compatibility between host plants and rhizobia take prominence over those related to antagonism. Leguminous host plants provide conducive environment that is characterized by production of resistance factors that are not harmful to mutualistic rhizobia. The leguminous root nodule also accommodates other microbes that benefit the host plant and cooperate with rhizobia. Together, the host plant, rhizobia, and other beneficial microbes within the root nodule microbiome promote their fitness over other endophytic and exophytic organisms that interact negatively through processes such as parasitism, pathogenism, predation, and competition. Organic carbon and nitrogen produced by the photosynthesis-nitrogen fixation machinery of legumes and rhizobia supports consumers at various trophic levels. Consumers are affected by compatible relationships between host plants and Rhizobium species (Dean et al., 2014; Naluyange et al., 2014, 2016; Pulido et al., 2019; Karoney et al., 2020; Xu et al., 2020). Consumers are also affected by antagonisms arising from incompatible relationship between host plants and rhizobium species (Gourion et al., 2015; Clúa et al., 2018; Benezech et al., 2020a). The function of both compatible and non-compatible relationships between host plants and rhizobia may still be affected by other beneficial or antagonistic biota and abiotic factors (Pugashetti et al., 1982; Parker, 2001; Mehboob et al., 2013; Haldar and Sengupta, 2015; Han et al., 2020). The photosynthesis-nitrogen fixation linkage of leguminous host plants and rhizobium, shapes the chemical profile and hence the community structures of both endophytic and exophytic organisms in the rhizosphere and phyllosphere, respectively. Therefore, a functional leguminous root nodule could be equated to stable and self-sufficient institution that provisions for its diverse citizens and protects its interests.
Compatibility of Rhizobium With Host Plants
The term “symbiosis” was coined in the year 1879 by the Father of Plant Pathology, the German botanist and mycologist Professor Heinrich Anton de Bary (1831-1888), to imply “the living together of unlike organisms” (Oulhen et al., 2016). Relationships between rhizobia and leguminous plants are among the most widely known examples of symbiosis. Compatibility of rhizobium with host plants is genetically determined and highly specific (Mergaert et al., 1997; Clúa et al., 2018; Sachs et al., 2018). The key determinants of specificity in rhizobium-legume relationships are lipo-chitooligosaccharide compounds known as Nod factors secreted by rhizobium strains (Mergaert et al., 1997; Geurts and Bisseling, 2002; Soto et al., 2006), in response to flavonoid signaling compounds in root exudates (Clúa et al., 2018). Flavonoid compounds such as the flavonoid aglycones (apigenin, daidzein, kaempferol, luteolin, myricetin, and quercetin) and the flavonoid glycosides (daidzin, genistin, hesperidin, hyperoside, kaempferol-7-O-glucoside, naringin, and rutin) have been detected in root exudates of the leguminous plants Melilotus indicus, Trifolium alexandrinum, and T. resupinatum (Gomaa et al., 2015). Compatible interactions based on plant flavonoids and rhizobial Nod factors trigger a series of events that lead to successful infection and development of nodules that can fix nitrogen (Wang et al., 2018). Such nodules can be determinate (nodules with determinate meristematic activity) or indeterminate (nodules with indeterminate meristematic activity) (Prell and Poole, 2006; Terpolilli et al., 2012; Mao et al., 2013).
Incompatible interactions between plants and Rhizobium may either result in non-formation of nodules (Wu et al., 2011; Gano-Cohen et al., 2016), or if formed, the nodules cannot fix nitrogen (Oono et al., 2009; Wang et al., 2018). Such groups of “freeloaders who do not pay rent” cannot be considered to be mutualistic, but they are parasitic Rhizobium species (Lewin, 1982; Taha, 1993; Denison and Kiers, 2004; Oono et al., 2009; Ballhorn et al., 2016). The main factors underlying incompatible interactions between plants and Rhizobium are genetically expressed in the form of non-complementary Nod factors (Mergaert et al., 1997; Geurts and Bisseling, 2002; Wang et al., 2018). Rhizobium species having genetic symbiotic defects are incompatible with host plants (Long, 1989; Nadler et al., 1990). Besides this, the legume host plant may produce defensive compounds including reactive oxygen species (ROS) that may inhibit Rhizobium nodulation (Gourion et al., 2015; Tóth and Stacey, 2015), or even the plant may be genetically non-nodulating (Matthews and Davis, 1990; Ali et al., 2014).
Antagonistic and Beneficial Rhizosphere Biota to Rhizobium-Legume Symbiosis
Antagonistic Rhizosphere Biota to Rhizobium-Legume Symbiosis
The leguminous rhizosphere is characterized by root exudation of organic compounds, enzymes, and ion exchange (Kidd et al., 2018; Preece and Peñuelas, 2020), supporting complex food webs of heterotrophic biota from various taxa (Bonkowski et al., 2009; De-la-Peña and Loyola-Vargas, 2014; Taylor et al., 2020) (Table 2). Rhizobium has to survive both in the rhizosphere and host tissues where they still encounter diverse endophytic biota inside the root nodule microbiome (Taha, 1993; Omar and Abd-Alla, 2000; Sharaf et al., 2019; Taylor et al., 2020). Such rhizosphere and endophytic biota have different influences on Rhizobium-legume symbiosis (Checcucci et al., 2017).
Competitors of Rhizobium Symbiosis
Competition is the contentious interaction for limited resources between organisms that is characterized by efficient resource consumption (exploitation) or harmful effects to the adversary (interference) (Ghoul and Mitri, 2016). Competition for nutrients and space is a key form of antagonistic interaction that affects rhizobia and host plants (Postma et al., 1990; Poole et al., 2018), in both independent and symbiotic states of the two organisms (Mendoza et al., 2016; Hortal et al., 2017; Lardi et al., 2017). Non-nodulating strains of Rhizobium leguminosarum compete with the nodulating types for infection sites, causing delay in development and function of root nodules (Lie et al., 1988), which reduces the performance of leguminous host plants (Gano-Cohen et al., 2016). In the root nodule microbiome of Medicago sativa, endophytic non-rhizobium bacteria engage in competitive interactions, with Brevibacillus brevis to diminish the benefits of cooperation between Sinorhizobium meliloti with the non-rhizobial Pseudomonas sp. and Paenibacillus sp. (Hansen et al., 2020). Apart from competing for infection sites on leguminous roots (Spaink, 1995; Mendoza-Suárez et al., 2020), free-living rhizobia, and other rhizosphere microbes compete for organic carbon in root exudates (Olanrewaju et al., 2019). Sources of organic carbon in root exudates include simple and complex sugars (e.g., fructose, mannose, glucose, maltose, arabinose, and oligosaccharides), amino acids (e.g., aspartate, asparagine, glutamine, arginine, and cysteine), organic acids (e.g., ascorbic, acetic, benzoic, ferulic, and malic acids), phenolic compounds, flavonoids, enzymes, fatty acids, auxins, gibberellins, nucleotides, tannins, steroids, terpenoids, alkaloids, polyacetylenes, and vitamins (Gunina and Kuzyakov, 2015; Hayat et al., 2017; Olanrewaju et al., 2019) (Table 2). Exudation of carbon-rich compounds by leguminous plants becomes more intense under conditions of phosphorus and nitrogen deficiency in the soil (Yoneyama et al., 2012; Tawaraya et al., 2014). Such nutrient-deficient conditions instigate intensive competition for N and P between leguminous roots and rhizosphere biota (Kuzyakov and Xu, 2013). Besides helping plants fix limited nitrogen (Liu et al., 2018), rhizobia are phosphate solubilizing microbes capable of enhancing phosphorus acquisition by leguminous host plants in P-deficient rhizospheres (Qin et al., 2011; Verma et al., 2020). As endophytes, the multiplying Rhizobium bacteroids require organic compounds in forms such as fumarate (C4H4O4), malate (C4H6O5), and succinate (C4H6O4) (Mitsch et al., 2018), as well as various mineral elements especially molybdenum (Mo), vanadium (V), iron (Fe), and phosphorus (P) that are required for nitrogenase synthesis within the O2 limited endonodular space (Rüttimann-Johnson et al., 1999; O'Hara, 2001; Rubio and Ludden, 2008; Brear et al., 2013; Hu and Ribbe, 2016). Whether intraspecific or interspecific, in planta competition for such nutrients is expected to occur under nutrient-deficient conditions (Oono et al., 2009).
Pathogenic and Non-pathogenic Parasites of Rhizobium Symbiosis
Pathogenic interactions are those that involve parasitic microbes that infect and reduce the performance of their hosts (Ochieno, 2020). Rhizobium symbioses exist within a situation of exploitation by various forms of parasites that can either be pathogenic or non-pathogenic (Brader et al., 2017). The main pathogens that directly affect Rhizobium cells are bacteriophage viruses (Werquin et al., 1988; Santamaría et al., 2014; Cubo et al., 2020). Phytopathogenic viruses infect leguminous shoots and root nodules resulting in reduced nodule biomass, premature nodule decay, low leghemoglobin content, and hence impaired nitrogen fixation (Orellana and Fan, 1978; Taha, 1993; Ismail and Atef, 1998; Huang, 2001; Mangeni et al., 2020). Other phytopathogens that infect root nodules include diverse bacterial species such as Ralstonia solanacearum in Medicago truncatula and Mimosa pudica (Benezech et al., 2020b; Moura et al., 2020). Fungal species such as Cladosporium cladosporioides, Fusarium moniliforme, Fusarium oxysporum, Fusarium solani, Macrophominia phaseolina, and Rhizoctonia solani are known plant pathogens (Walker et al., 2016; Batnini et al., 2020; Chen et al., 2020; Lakhran and Ahir, 2020; Poveda et al., 2020). However, non-pathogenic strains of the previously mentioned fungi with plant growth promoting effects have been isolated from leguminous root nodules of faba bean (Vicia faba) (Omar and Abd-Alla, 2000). While currently there is scarcity of published works on pathogenic endophytic fungi infection of leguminous root nodules, establishment of host range, and non-pathogenicity of some endophytic fungi remains a contentious subject (Ochieno, 2010, 2020; Avedi et al., 2014; Zarafi et al., 2015; Oula et al., 2020).
Root endoparasitic nematodes are pathogenic microinvertebrates that exploit resources from rhizobium-legume symbioses (Taha, 1993; Weerasinghe et al., 2005). Root knot nematodes of Meloidogyne spp. are examples of plant parasitic nematodes that cause galls on leguminous roots that affect rhizobial nodulation (El-Bahrawy and Salem, 1989; Wood et al., 2018; Yergaliyev et al., 2020). Meloidogyne incognita produces chemical factors that elicit signal transduction events that result in root knots, in a similar manner that roots nodules are formed through Rhizobium Nod factors (Weerasinghe et al., 2005).
Non-pathogenic parasites of Rhizobium-legume symbioses comprise a wide range of microbes that draw resources from symbiotic systems without causing disease to rhizobia or the host plant. These include non-pathogenic non-symbiotic bacteria and fungi that live endophytically in root nodules and other plant tissues without causing disease (Omar and Abd-Alla, 2000; Mrabet et al., 2006; Selvakumar et al., 2013; De Meyer et al., 2015; Martínez-Hidalgo and Hirsch, 2017; Hassen et al., 2018; Muresu et al., 2019). Besides the non-pathogenic bacteria, root nodules of leguminous plants such as Hedysarum species and Vicia faba host human pathogens such as Enterobacter cloacae, Enterobacter kobei, Escherichia vuneris, Pantoea agglomerans, and Leclercia adecarboxylata (Muresu et al., 2010; Saïdi et al., 2013; Selvakumar et al., 2013). These are non-pathogenic parasites to rhizobial host plants.
Predation of Rhizobium-Legume Symbiosis
Predation is a form of biointeraction in which one organism (predator) eats all or part of another organisms (prey). Herbivory is a form of predation in which the prey is a plant such as legumes, while microbivory is where the prey is a microbe like Rhizobium. Predation of the Rhizobium-plant symbiosis comes both in the form of microbivory and herbivory (Ramirez and Alexander, 1980). Direct predation on Rhizobium cells occurs in the rhizosphere by microbivore protozoans and nematodes (Ramirez and Alexander, 1980; Postma et al., 1990; Verhagen et al., 1993; Trap et al., 2016; Jiang et al., 2017). When ingested, Rhizobium induces DNA damage in the intestinal cells of the bacterivorous nematode Caenorhabditis elegans (Kniazeva and Ruvkun, 2019). Herbivory by rhizophages belonging to various taxa that feed on leguminous roots determines the establishment and function of Rhizobium-legume symbiosis within the root nodule microbiome. These include nematodes, arthropods, molluscs, and vertebrates that feed on roots of various leguminous plants (Brooks et al., 1988; Douglas and Tooker, 2012; Pereira et al., 2018; Gilarte et al., 2020). Herbivory on Rhizobium-plant symbiosis involves damage to root nodules and adjacent tissues by mandibulate coleopterous insects such as larval Cerotoma arcuata (Chrysomelidae) and Sitona lepidus (Curculionidae) that feed on root nodules of leguminous crops (Teixeira et al., 1996; Johnson et al., 2005; Evenden, 2018; Pereira et al., 2018). Sap-sucking organisms such as the root parasitic nematode Meloidogyne incognita and bean root aphid Smynthurodes betae (Aphididae) also fall into this category of belowground herbivores (Stevenson et al., 2007; Sikora et al., 2018). Molluscs such as slugs engage in rhizophagy through feeding by scraping of leguminous roots of medicago sativa among other plants (Douglas and Tooker, 2012). Rhizophagy extends to vertebrate species that interfere with leguminous roots while accessing edible belowground structures (Brooks et al., 1988).
Allelopathy and Rhizobium-Legume Symbiosis
Allelopathy (Greek: allelon=mutual, pathos=harm) is a biointeraction concept that was conceived by Professor Hans Molisch (1856-1937) around the year 1935 (Chou, 2006). Allelopathy is a mutual relationship between organisms that involves the release of secondary metabolites known as allelochemicals (Vokou et al., 2006; Farooq et al., 2020). Allelochemicals may have stimulatory (positive allelopathy) or inhibitive (negative allelopathy) effects among plants, microbes, invertebrates, and other interacting organisms (Cheng and Cheng, 2015; Mahdhi et al., 2018; Schandry and Becker, 2020). Positive allelopathy can also be referred to as “probiosis” while negative allelopathy is termed as “antibiosis” (Yunes, 2019). Allelochemicals produced by the interacting organisms are referred to as “probiotics” and “antibiotics,” respectively (Selleck, 1972; Yunes, 2019; Schandry and Becker, 2020).
Release of allelochemicals by leguminous plants can increase their vulnerability to antagonists thereby interfering with nitrogen fixing symbiosis with Rhizobium. For instance, cowpea Vigna unguiculata and faba bean Vicia faba are leguminous hosts of Rhizobium that release strigolactone allelochemicals via the carotenoid biosynthetic pathway (Matusova et al., 2005; Miyakawa et al., 2020), which stimulate germination and infection by the parasitic weeds Striga gesnerioides and Broomrapes Orobanche spp. (Bouraoui et al., 2016; Miyakawa et al., 2020). Flavonoids and strigolactones stimulate the germination of fungal pathogen spores and attraction of parasitic nematodes increasing chances of root infection (Steinkellner et al., 2007; Chin et al., 2018). Antagonistic plants can also release allelochemicals that interfere with leguminous host plants and symbiotic Rhizobium species in the rhizosphere (Rice, 1992; Kluson, 1995). For instance, the weed Sonchus oleraceus (Asteraceae) produces allelochemicals that inhibit the production of flavonoid compounds and root nodulation in the leguminous weeds Melilotus indicus and Trifolium resupinatum (Gomaa et al., 2015).
Leguminous host plants and rhizobia also produce allelochemicals that have detrimental effects on other plants, microbes, and herbivores through negative allelopathy (antibiosis). For instance, velvetbean (Mucuna deeringiana), jackbean (Canavalia ensiformis), jumbiebean (Leucaena leucocephala), and wild tamarind (Lysiloma latisiliquum) produce phytotoxic allelochemicals that are suppressive to weeds (Caamal-Maldonado et al., 2001). The leguminous weeds Trigonella polycerata, Vicia sativa, Lathyrus aphaca, and Medicago polymorpha produce allelochemicals that are suppressive to the growth of rice (Oryza sativa) (Zohaib et al., 2017). Forage legumes of the genus Desmodium release allelochemicals that are suppressive to the parasitic weeds Striga spp. (Pickett et al., 2013). Furthermore, M. deeringiana and C. ensiformis allelochemicals were found to be inhibitive to plant parasitic nematodes (Caamal-Maldonado et al., 2001). Leguminous plants also release antimicrobial allelochemicals including quercetin, luteolin and other substituted flavones that inhibit pathogens in the rhizosphere (Weston and Mathesius, 2013). Rhizobia on the other hand release allelochemicals that inhibit the activities of other organisms. Such allelochemicals include anti-rhizobial peptides produced by strains of Rhizobium, Mesorhizobium, and Sinorhizobium associated with their enhanced nodulation capacity and competitiveness (Triplett, 1999; Naamala et al., 2016).
Leguminous host plants and rhizobia engage in positive allelopathy (probiosis) in establishing root nodule symbiosis. Leguminous root exudates contain flavonoids that are the main allelochemical attractants for Rhizobium symbiosis (Hassan and Mathesius, 2012; Makoi and Ndakidemi, 2012). On the other side, Rhizobium secretes lipo-chitooligosaccharide compounds known as Nod factors that induce root nodulation in leguminous host plants (D'haeze and Holsters, 2002; Nandhini et al., 2018). Besides flavonoids, legumes also produce strigolactone allelochemicals that enhance Rhizobium activities on host roots (Peláez-Vico et al., 2016; McAdam et al., 2017), while stimulating the germination of arbuscular mycorrhizal fungi that facilitate phosphorus acquisition for improved nitrogen fixation (Püschel et al., 2017; Kafle et al., 2019). Positive allelopathy also exists in the leguminous crop Trifolium alexandrinum, which is induced to release high level of flavonoid compounds as a resistance response toward phytotoxic allelochemicals produced by the weed Sonchus oleraceus (Gomaa et al., 2015).
It is worth noting that the root words of the “allelopathy” terminology, denote harmful interactions. Therefore, “positive allelopathy” should be limited to situations whereby an organism releases allelochemicals that stimulate activities of the enemy. A good example is the stimulatory effect of plant allelochemicals to parasitic weeds and microbial pathogens. Cases whereby allelochemicals are harmful to other organisms should be considered as “negative allelopathy” or “antibiosis.” Stimulatory effects of plant metabolites to symbiotic beneficial microbes such as rhizobium and arbuscular mycorrhizal fungi should not be considered to be any form of allelopathy. These should be termed as “probiosis,” a term that would better be delinked from allelopathy.
Beneficial Rhizosphere Biota to Rhizobium-Legume Symbiosis
Rhizobium-legume symbioses are associated with rhizosphere organisms that favor their establishment and performance. The most common is the tripartite symbiotic relationship of Rhizobium and legumes with endophytic arbuscular mycorrhizal fungi such as Glomus intraradices (Scheublin et al., 2004; Scheublin and Van Der Heijden, 2006; Kaschuk et al., 2009, 2010), which enhance the acquisition of phosphorus (Tajini et al., 2012; Meng et al., 2015), while protecting the leguminous roots from attacks by microbial pathogens and root parasitic nematodes (Harrier and Watson, 2004; Wille et al., 2019). The rhizosphere also hosts a range of plant growth promoting microbes including non-nodulating bacterial species that provide services such as nitrogen fixation, nutrient cycling, growth hormone and siderophore production, and biological control while improving soil texture and water holding capacity (Martínez-Hidalgo and Hirsch, 2017; Mishra et al., 2017; Backer et al., 2018; Naik et al., 2019). For instance, apart from biological nitrogen fixation by endophytic rhizobia, other rhizosphere microbes play the roles of ammonification, nitrification, and denitrification that are part of the nitrogen cycle (Pajares and Bohannan, 2016; Kakraliya et al., 2018). Rhizosphere microbes involved in nitrification include the ammonia-oxidizing bacteria (e.g. Nitrosomonas, Micrococcus, Europaea, Nitrosococcus, Nitrosospira, Briensis, Nitrosovibrio, and Nitrocystis), nitrite-oxidizing bacteria (Nitrobacter winogradskyi, Nitrosococcus mobilis, Nitrocystis, Nitrospina gracilis), and nitrite-oxidizing fungi (e.g., Penicillium, Aspergillus) and actinomycetes (e.g., Streptomyces, Nocardia) (Paungfoo-Lonhienne et al., 2017; Kakraliya et al., 2018). Legume crops such as peanut (Arachis hypogaea) and soybean (Glycine max) increase the abundance of soil bacteria and archaea, but they suppress ammonia oxidizers dominated by archaea (Paungfoo-Lonhienne et al., 2017). This is probably due to competition for ammonium between plant roots and nitrifying bacteria (Verhagen et al., 1993). Mineral phosphate solubilizing microbes include bacteria in the genus Bacillus and Pseudomonas and the fungal genera Aspergillus and Penicillium (Khan et al., 2007). Biological control by beneficial rhizosphere microbes is offered through mechanisms such as antibiosis, competition for iron, parasitism, production of extracellular cell wall degrading enzymes, and induced resistance (Whipps, 2001). Furthermore, non-nodulating bacterial species that exist in root nodules help the nodulating rhizobia in extending their host range (Wu et al., 2011). Rhizobium-legume symbioses may also benefit from rhizosphere invertebrates through phoretic transfer of bacterial cells by nematodes like Caenorhabditis elegans that are attracted by plant volatiles to enhance root nodulation (Horiuchi et al., 2005).
Rhizobium-Legume Symbiosis and Aboveground Interactions
Increase in Abundance of Aboveground Consumers
Indirect interactions exist between Rhizobium in root nodules and consumers in the phyllosphere through host-mediated processes (Kempel et al., 2009). Rhizobium infection of leguminous roots has been linked with increased colonization of common bean leaves by the fungal pathogen Colletotrichum lindemuthianum (Naluyange et al., 2014, 2016; Karoney et al., 2020). Also, the presence of Rhizobium in leguminous roots is associated with increased abundance of arthropods such as aphids and mites (Katayama et al., 2011a,b; Dean et al., 2014; Naluyange et al., 2014, 2016). Besides this, aboveground insects such as clover root weevil (Sitona lepidus) prefer ovipositing at the base of Rhizobium-infected white clover (Trifolium repens) to ensure food availability and hence survival of their larvae (Johnson et al., 2006). Increase in abundance of foliar consumers is due to improved nutritive suitability of rhizobial plants through symbiotic nitrogen fixation (Karoney et al., 2020). Plants infected with root nodulating nitrogen fixing rhizobia become tolerant to herbivory (Naluyange et al., 2014; Ballhorn et al., 2017; Karoney et al., 2020).
Decrease in Abundance of Aboveground Consumers
Rhizobium infection of roots has been associated with inhibition of aboveground consumers through induced resistance mechanisms. Leguminous plants may either exhibit an increase in production of toxic compounds (antibiosis) (Clement et al., 1994; Soundararajan et al., 2013), or may affect the behaviors of the consumers through less attractive volatile emissions and unpleasant tastes (antixenosis) (Clement et al., 1994; Soundararajan et al., 2013). Rhizobium-induced antibiosis has been reported in Lima bean (Phaseolus lunatus) inoculated with Bradyrhizobium elkanii characterized by enhanced cyanogenesis that inhibits the Mexican bean beetle Epilachna varivestis (Coccinellidae) (Thamer et al., 2011; Godschalx et al., 2017). Nodulation of Crotalaria roots by Bradyrhizobium induces the biosynthesis of pyrrolizidine alkaloids that are toxic to grazers (Irmer et al., 2015). Rhizobium-induced antixenosis has been reported in Lima bean (P. lunatus) inoculated with B. elkanii that exhibits reduction in attractiveness to E. varivestis (Ballhorn et al., 2013), while the extrafloral nectar is less preferred by the pavement ant Tetramorium caespitum (Formicidae) (Godschalx et al., 2015). Apart from resistance mechanisms, rhizobia may establish dense endophytic and rhizosphere populations that compete with host plants for nutrients, and hence interfering with food supply to aboveground consumers. Such a situation may occur in plants growing under nutrient-deficient conditions (Ochieno, 2010, 2020), or when light energy for photosynthesis is not sufficient (Ballhorn et al., 2016).
Suppression of Rhizobium by Aboveground Consumers
Aboveground organisms influence symbiotic activities of Rhizobium in leguminous roots. Infection of common bean leaves by the fungal pathogen Colletotrichum gloeosporioides induces plant defense responses that inhibit root nodulation by Rhizobium and colonization by arbuscular mycorrhizal fungi (Ballhorn et al., 2014). In this interaction, C. gloeosporioides in the leaves enhances root activity of polyphenol oxidase (PPO) (Ballhorn et al., 2014), an enzyme associated with plant resistance to microbial pathogens (Constabel and Barbehenn, 2008; Taranto et al., 2017). Rhizobium usually evades such plant defenses in successful infections to induce root nodulation (Tóth and Stacey, 2015; Cao et al., 2017). However, polyphenol oxidase may not be responsible for suppression of Rhizobium nodulation, because the enzyme improves the development, structure, and function of root nodules (Webb et al., 2014). Rhizobium inoculation results in high concentration of nitrogen-based compounds including polyphenol oxidase, while reducing the concentration of phenolic compounds and other carbon-based metabolites (Karoney et al., 2020). The absence of polyphenol oxidase results in the accumulation of phenolic compounds (Webb et al., 2014), which are not viable sources of organic carbon for Rhizobium development and function compared to the C4 compounds (fumarate, malate and succinate) (Mitsch et al., 2018).
An alternative explanation is that, aboveground consumers destroy leaves and interfere with photosynthesis while consuming sugars and other nutrients that are required by Rhizobium leading to poor root nodulation (Katayama et al., 2014). This is similar to conditions of inadequate light for photosynthesis that constrains symbiosis transforming Rhizobium and other mutualists into parasites (Ballhorn et al., 2016). However, there is evidence that herbivory of red alder Alnus rubra (Betulaceae) seedlings induces compensatory growth with enhanced root nodulation by the nitrogen-fixing Frankia bacteria (Ballhorn et al., 2017). This is a form of Rhizobium-induced tolerance to consumers in host plants (Karoney et al., 2020).
Rhizobium in Sustainable Food Systems
Sustainable food systems (SFS) are currently being emphasized globally to improve food production in terms of quality and quantity in line with Sustainable Development Goals (SDGs) (ICSU, 2017). This can be achieved through methods and processes that ensure continuous profitability (economic sustainability), having broad-based benefits for society (social sustainability), with positive or neutral impacts on the natural environment (environmental sustainability) (FAO, 2018). Nitrogen-fixing Rhizobium species are part of plant growth promoting microbes being developed into bio-inoculants (Giller and Ronner, 2019), as part of Sustainable Food Systems (SFS) (FAO, 2018). The application of Rhizobium for biological nitrogen fixation (BNF) restores the function of the nitrogen and carbon cycles (Thornton et al., 2007), which are key nutrient deficiencies in human-disturbed agroecosystems (Morrow et al., 2016; Smith et al., 2016). Rhizobia symbiotically fix approximately 40 million tons of nitrogen in agroecosystems annually (Udvardi and Poole, 2013). Bio-inoculants based on symbiotic nitrogen fixing Rhizobium species could help minimize the misuse of synthetic nitrogenous fertilizers (Zahran, 1999), which have been linked to phytotoxicity (Naluyange et al., 2014; Delgado et al., 2016), soil acidification (Sha et al., 2020), and eutrophication of water bodies resulting in algal blooms and water hyacinth invasiveness (Naluyange et al., 2014; Onyango et al., 2020). Besides, the misuse of synthetic nitrogenous fertilizers is a public health concern (Wang and Lu, 2020), as the unassimilated salts in consumed plant tissues and the environment have been linked with health problems including respiratory ailments, cardiac diseases, and cancers (Townsend et al., 2003; Lu et al., 2015).
The coupling of rhizobial nitrogenase activity to leguminous photosynthesis improves carbon dioxide fixation and hence carbon sequestration (Kou-Giesbrecht and Menge, 2019). Nitrogen-fixing legume crops contribute to reduced emission of greenhouse gases associated with global warming and vulnerability to the effects of climate change (Stagnari et al., 2017). Mitigation of the negative effects of atmospheric carbon dioxide can be achieved through legume-based intercropping, agroforestry, conservation agriculture, and organic farming that integrate biological nitrogen fixation by various Rhizobium species (Khan et al., 2011; Stagnari et al., 2017). Furthermore, Rhizobium species are biological control agents in Integrated Pest Management systems (IPM) (Khan et al., 2011), which act directly on pests through processes such as antibiosis and competition (Deshwal et al., 2003b; Kawaguchi et al., 2012), and indirectly by enhancing tolerance of leguminous plants to pests (Naluyange et al., 2014; Karoney et al., 2020). Biological nitrogen fixation also improves food availability and hence species richness of beneficial organisms that provide ecosystem services such as parasitoids, predators, and pollinators (Mattson Jr, 1980; Barber and Soper Gorden, 2015; Tao et al., 2017).
Future Prospects
The world human population grew past 7.7 billion in the year 2019 and is expected to rise to 9.7 billion by the year 2050 (UN DESA, 2017). Feeding such a fast-growing population requires the intensification of food production systems (Corbeels et al., 2020). The Green Revolution started in the 1960s by Professor Norman E. Borlaug (1914-2009) was meant to ensure increased food production (Borlaug, 2002; Sumberg et al., 2012). However, this Green Revolution was characterized by excessive use of agrochemicals that polluted the environment besides being a threat to food security (van Emden and Peakall, 1996; Carson, 2002; Arora et al., 2020). The development of modern biotechnology industries and their marketing strategies (McCullum et al., 2003; Paarlberg, 2009), whose emphasis is perceived to require more focus on the importance of agroecological approaches (Herren et al., 2015; D'Annolfo et al., 2017), have brought up complex dimensions on the concept of Sustainable Food Systems (Zollitsch et al., 2007; Schütte et al., 2017; Ochieno, 2020). For instance, without adequate agroecological conceptualization, it may be a contentious matter to suggest that, destruction of leguminous biodiversity through herbicide-based weed management (Norsworthy et al., 2010; Corbeels et al., 2020), destroys nitrogen fixing and carbon-fixing machinery that sustain life on earth (Khan et al., 2006; Druille et al., 2015). This is because biodiversity of leguminous plants is associated with complexes of symbiotic nitrogen fixing Rhizobium species (Athar and Shabbir, 2008; Marwat et al., 2009).
There is need to establish agroecological management systems that support biological nitrogen fixation as part of the nitrogen cycle, as well as photosynthesis within the carbon cycle, in order to attain sustainable food systems (Tully and Ryals, 2017; Shah et al., 2020). In this case, Rhizobium-legume symbioses need to be an integral part of agroecosystems management strategies in the Next Green Revolution (Conway and Barbie, 1988; Arora et al., 2020). Integrated approaches that promote inflow and sufficient utilization of energy and nutrients while limiting the loss of such resources in agroecosystems need to be encouraged. This comprises Integrated Food Energy Systems (IFES) (Sachs and Silk, 1991; Bogdanski et al., 2011), especially those that include leguminous crops (Germaine et al., 2010; Bogdanski, 2012; Orr et al., 2015). Evidence already exists in yield improvement under mixed-cropping systems that include leguminous crops (Ofori and Stern, 1987; Duchene et al., 2017). For instance, agroecological technologies such as Push-Pull that rely on leguminous crops to promote cereal production while suppressing the parasitic weed Striga hermonthica and insect pests like the fall armyworm Spodoptera frugiperda and the stemborers Chilo partellus and Busseola fusca (Hailu et al., 2018). The use of organic matter such as compost and mulch to improve soil carbon, nitrogen and other soil properties that benefit Rhizobium-legume symbiosis need to be encouraged (Naluyange et al., 2014). Informed and better methods for the application of synthetic fertilizers and pesticides, such as those based on precision agriculture need to be developed for the promotion of Rhizobium symbioses (Thilakarathna and Raizada, 2018). There is need to re-examine IPM decision models such as Economic Threshold Levels (ETLs) (Capinera, 1981; Knight and Cammell, 1994), especially in situations whereby herbivore populations are boosted by Rhizobium-induced improved nutritive suitability and tolerance in leguminous host plants (Dean et al., 2014; Karoney et al., 2020). This will help in preventing the misuse of pest control products. This should be extended to other beneficial rhizobacteria whose interactions with herbivores have been associated with induced susceptibility in various host plants (Katayama et al., 2010, 2011a,b; Pineda et al., 2012). Resources need to be allocated toward research and innovation in microbial genetic resources (Sharma et al., 2018), so as to identify and develop competitive and efficient Rhizobium strains (Irisarri et al., 2019). This should follow guidelines that deliver endophytic plant growth promoting microbes and identifying non-beneficial ones for the bio-inoculant and biofertilizer industry (Avedi et al., 2014; Ochieno, 2020). This should be complemented with research on plant genetic resources to improve Rhizobium-legume symbiosis with nutrient use efficiency. Regardless of the technology used to develop such plants and microbes, their function as biotic factors in agroecosystems need to be well-integrated into Sustainable Food Systems conceptual frameworks (Hansen et al., 2017; Afzal et al., 2020). There is need to address disparities in research partnerships related to Rhizobium-legume symbioses and other similar plant and soil associated technologies (Giller, 2020; Minasny et al., 2020; Ochieno, 2020). Investment in research on various aspects of Rhizobium-legume interactions is necessary for the application of such plant growth promoting microbes for Sustainable Food Systems. Emphasis should be on the application of modern technologies in unraveling the composition and function of root nodule microbiomes in relation to rhizosphere microbiomes of various ecosystems.
Author Contributions
DO conceptualization and drafting the manuscript. EK drafting the manuscript and tables. EM, EN, and VN drafting the manuscript and imaging. DB and SS drafting the manuscript. All authors contributed to the article and approved the submitted version.
Funding
This review is associated with work that was supported by the International Foundation for Science, IFS [C5681-1, 2015] and co-funding from the Association of African Universities (AAU) [REF. PC/6].
Conflict of Interest
The authors declare that the research was conducted in the absence of any commercial or financial relationships that could be construed as a potential conflict of interest.
References
Adolph, B., Allen, M., Beyuo, E., Banuoku, D., Barrett, S., Bourgou, T., et al. (2020). Supporting smallholders' decision making: managing trade-offs and synergies for sustainable agricultural intensification. Int. J. Agric. Sustain. 1–18. doi: 10.1080/14735903.2020.1786947
Afzal, M., Alghamdi, S. S., Migdadi, H. H., Khan, M. A., Mirza, S. B., and El-Harty, E. (2020). Legume genomics and transcriptomics: from classic breeding to modern technologies. Saudi J. Biol. Sci. 27, 543–555. doi: 10.1016/j.sjbs.2019.11.018
Ali, L., Madrid, E., Varshney, R. K., Azam, S., Millan, T., Rubio, J., et al. (2014). Mapping and identification of a Cicer arietinum NSP2 gene involved in nodulation pathway. Theor. Appl. Genet. 127, 481–488. doi: 10.1007/s00122-013-2233-3
Allen, J. F. (2003). Cyclic, pseudocyclic and noncyclic photophosphorylation: new links in the chain. Trends Plant Sci. 8, 15–19. doi: 10.1016/S1360-1385(02)00006-7
Andersen, T. G. (2020). How to catch the N–An inter-species exchange with the right chemistry. Mol. Syst. Biol. 16:e9514. doi: 10.15252/msb.20209514
Ansari, S., Charehgani, H., and Ghaderi, R. (2019). Resistance of ten common medicinal plants to the root-knot nematode Meloidogyne javanica. Hell. Plant Prot. J. 12, 6–11. doi: 10.2478/hppj-2019-0002
Archimède, H., Eugène, M., Magdeleine, C. M., Boval, M., Martin, C., Morgavi, D. P., et al. (2011). Comparison of methane production between C3 and C4 grasses and legumes. Anim. Feed Sci. Technol. 166, 59–64. doi: 10.1016/j.anifeedsci.2011.04.003
Arora, N. K., Fatima, T., Mishra, I., and Verma, S. (2020). “Microbe-based inoculants: role in next green revolution,” in Environmental Concerns and Sustainable Development, eds V. Shukla and N. Kumar (Singapore: Springer), 191–246. doi: 10.1007/978-981-13-6358-0_9
Athar, M., and Shabbir, S. M. (2008). Nodulating leguminous weeds of some major crops of Pakistan. Phytologia 90, 246–251.
Avedi, E. K., Ochieno, D. M., Ajanga, S., Wanyama, C., Wainwright, H., Elzein, A., et al. (2014). Fusarium oxysporum f. sp. strigae strain Foxy 2 did not achieve biological control of Striga hermonthica parasitizing maize in Western Kenya. Biol. Control 77, 7–14. doi: 10.1016/j.biocontrol.2014.05.012
Backer, R., Rokem, J. S., Ilangumaran, G., Lamont, J., Praslickova, D., Ricci, E., et al. (2018). Plant growth-promoting rhizobacteria: context, mechanisms of action, and roadmap to commercialization of biostimulants for sustainable agriculture. Front. Plant Sci. 9:1473. doi: 10.3389/fpls.2018.01473
Bajaj, Y. P. S., Atwal, A. S., and Tanda, A. S. (1989). In vitro inhibition of root-knot nematode Meloidogyne incognita by sesame root exudate and its amino acids. Nematologica 35, 115–124. doi: 10.1163/002825989X00124
Ballhorn, D. J., Elias, J. D., Balkan, M. A., Fordyce, R. F., and Kennedy, P. G. (2017). Colonization by nitrogen-fixing Frankia bacteria causes short-term increases in herbivore susceptibility in red alder (Alnus rubra) seedlings. Oecologia 184, 497–506. doi: 10.1007/s00442-017-3888-2
Ballhorn, D. J., Kautz, S., and Schädler, M. (2013). Induced plant defense via volatile production is dependent on rhizobial symbiosis. Oecologia 172, 833–846. doi: 10.1007/s00442-012-2539-x
Ballhorn, D. J., Schädler, M., Elias, J. D., Millar, J. A., and Kautz, S. (2016). Friend or foe-light availability determines the relationship between mycorrhizal fungi, rhizobia and Lima Bean (Phaseolus lunatus L.). PLoS ONE 11:e0154116. doi: 10.1371/journal.pone.0154116
Ballhorn, D. J., Younginger, B. S., and Kautz, S. (2014). An aboveground pathogen inhibits belowground rhizobia and arbuscular mycorrhizal fungi in Phaseolus vulgaris. BMC Plant Biol, 14:321. doi: 10.1186/s12870-014-0321-4
Barber, N. A., and Soper Gorden, N. L. (2015). How do belowground organisms influence plant–pollinator interactions? J. Plant Ecol. 8, 1–11. doi: 10.1093/jpe/rtu012
Bascuñán-Godoy, L., Sanhueza, C., Hernández, C. E., Cifuentes, L., Pinto, K., Álvarez, R., et al. (2018). Nitrogen supply affects photosynthesis and photoprotective attributes during drought-induced senescence in quinoa. Front. Plant Sci. 9:994. doi: 10.3389/fpls.2018.00994
Bassi, D., Menossi, M., and Mattiello, L. (2018). Nitrogen supply influences photosynthesis establishment along the sugarcane leaf. Sci. Rep. 8, 1–13. doi: 10.1038/s41598-018-20653-1
Basu, S., and Kumar, G. (2020). “Nitrogen fixation in a legume-rhizobium symbiosis: the roots of a success story,” in Plant Microbe Symbiosis, eds A. Varma, S. Tripathi, and R. Prasad (Cham: Springer), 35–53. doi: 10.1007/978-3-030-36248-5_3
Batnini, M., Lopez-Gomez, M., Palma, F., Haddoudi, I., Kallala, N., Zribi, K., et al. (2020). Sinorhizobium spp inoculation alleviates the effect of Fusarium oxysporum on Medicago truncatula plants by increasing antioxidant capacity and sucrose accumulation. Appl. Soil Ecol. 150, 103458. doi: 10.1016/j.apsoil.2019.103458
Benezech, C., Berrabah, F., Jardinaud, M. F., Le Scornet, A., Milhes, M., Jiang, G., et al. (2020b). Medicago-Sinorhizobium-Ralstonia co-infection reveals legume nodules as pathogen confined infection sites developing weak defenses. Curr. Biol. 30, 351–358. doi: 10.1016/j.cub.2019.11.066
Benezech, C., Doudement, M., and Gourion, B. (2020a). Legumes tolerance to rhizobia is not always observed and not always deserved. Cell. Microbiol. 22:e13124. doi: 10.1111/cmi.13124
Bergersen, F. J. (1997). Regulation of nitrogen fixation in infected cells of leguminous root nodules in relation to O2 supply. Plant Soil 191, 189–203. doi: 10.1023/A:1004236922993
Bogdanski, A. (2012). Integrated food–energy systems for climate-smart agriculture. Agric. Food Secur. 1, 1–10. doi: 10.1186/2048-7010-1-9
Bogdanski, A., Dubois, O., Jamieson, C., and Krell, R. (2011). Making Integrated Food-Energy Systems Work for People and Climate: An Overview. Rome: Food and Agriculture Organization of the United Nations (FAO).
Bolaños-Vásquez, M. C., and Werner, D. (1997). Effects of Rhizobium tropici, R. etli, and R. leguminosarum bv. phaseoli on nod gene-inducing flavonoids in root exudates of Phaseolus vulgaris. Mol. Plant Microbe Interact. 10, 339–346. doi: 10.1094/MPMI.1997.10.3.339
Bonkowski, M., Villenave, C., and Griffiths, B. (2009). Rhizosphere fauna: the functional and structural diversity of intimate interactions of soil fauna with plant roots. Plant Soil 321, 213–233. doi: 10.1007/s11104-009-0013-2
Borlaug, N. E. (2002). The Green Revolution Revisited and the Road Ahead. Stockholm: Nobelprize. org.
Bouraoui, M., Abbes, Z., Rouissi, M., Abdi, N., Hemissi, I., Kouki, S., et al. (2016). Effect of rhizobia inoculation, N and P supply on Orobanche foetida parasitising faba bean (Vicia faba minor) under field conditions. Biocontrol Sci. Technol. 26, 776–791. doi: 10.1080/09583157.2016.1157137
Brader, G., Compant, S., Vescio, K., Mitter, B., Trognitz, F., Ma, L. J., et al. (2017). Ecology and genomic insights into plant-pathogenic and plant-nonpathogenic endophytes. Annu. Rev. Phytopathol. 55, 61–83. doi: 10.1146/annurev-phyto-080516-035641
Brear, E. M., Day, D. A., and Smith, P. M. C. (2013). Iron: an essential micronutrient for the legume-rhizobium symbiosis. Front. Plant Sci. 4:359. doi: 10.3389/fpls.2013.00359
Brewin, N. J. (2010). Root Nodules (legume–rhizobium symbiosis). eLS. doi: 10.1002/9780470015902.a0003720.pub2
Brooks, J. E., Ahmad, E., and Hussain, I. (1988). “Characteristics of damage by vertebrate pests to groundnuts in Pakistan,” in Proceedings of the Vertebrate Pest Conference (Vol. 13). Available online at: https://digitalcommons.unl.edu/cgi/viewcontent.cgi?article=1026andcontext=vpcthirteen
Buchanan, B. B. (2016). The carbon (formerly dark) reactions of photosynthesis. Photosynth. Res. 128, 215–217. doi: 10.1007/s11120-015-0212-z
Caamal-Maldonado, J. A., Jiménez-Osornio, J. J., Torres-Barragán, A., and Anaya, A. L. (2001). The use of allelopathic legume cover and mulch species for weed control in cropping systems. Agron. J. 93, 27–36. doi: 10.2134/agronj2001.93127x
Cai, F., Watson, B. S., Meek, D., Huhman, D. V., Wherritt, D. J., Ben, C., et al. (2017). Medicago truncatula oleanolic-derived saponins are correlated with caterpillar deterrence. J. Chem. Ecol. 43, 712–724. doi: 10.1007/s10886-017-0863-7
Cao, Y., Halane, M. K., Gassmann, W., and Stacey, G. (2017). The role of plant innate immunity in the legume-rhizobium symbiosis. Annu. Rev. Plant Biol. 68, 535–561. doi: 10.1146/annurev-arplant-042916-041030
Capinera, J. L. (1981). Some effects of infestation by bean aphid, Aphis fabae Scopoli, on carbohydrate and protein levels in sugarbeet plants, and procedures for estimating economic injury levels. Z. Angew. Entomol. 92, 374–384. doi: 10.1111/j.1439-0418.1981.tb01686.x
Chalam, V. C., Deepika, D. D., Abhishek, G. J., and Maurya, A. K. (2020). “Major seed-borne diseases of agricultural crops: International Trade of Agricultural Products and Role of Quarantine,” in Seed-Borne Diseases of Agricultural Crops: Detection, Diagnosis and Management, eds R. Kumar and A. Gupta (Singapore: Springer), 25–61. doi: 10.1007/978-981-32-9046-4_2
Chamizo-Ampudia, A., Sanz-Luque, E., Llamas, A., Galvan, A., and Fernandez, E. (2017). Nitrate reductase regulates plant nitric oxide homeostasis. Trends Plant Sci. 22, 163–174. doi: 10.1016/j.tplants.2016.12.001
Checcucci, A., DiCenzo, G. C., Bazzicalupo, M., and Mengoni, A. (2017). Trade, diplomacy, and warfare: the quest for elite rhizobia inoculant strains. Front. Microbiol. 8:2207. doi: 10.3389/fmicb.2017.02207
Chen, L., Wu, Q., He, T., Lan, J., Ding, L., Liu, T., et al. (2020). Transcriptomic and metabolomic changes triggered by Fusarium solani in Common Bean (Phaseolus vulgaris L.). Genes 11:177. doi: 10.3390/genes11020177
Cheng, F., and Cheng, Z. (2015). Research progress on the use of plant allelopathy in agriculture and the physiological and ecological mechanisms of allelopathy. Front. Plant Sci. 6:1020. doi: 10.3389/fpls.2015.01020
Chesworth, J. M., Stuchbury, T., and Scaife, J.R. (1998). “Glycolysis,” in An Introduction to Agricultural Biochemistry, eds J. M. Chesworth, T. Stuchbury, and J. R. Scaife (Dordrecht: Springer), 141–147. doi: 10.1007/978-94-009-1441-4
Chin, S., Behm, C. A., and Mathesius, U. (2018). Functions of flavonoids in plant–nematode interactions. Plants 7:85. doi: 10.3390/plants7040085
Chou, C. H. (2006). “Introduction to allelopathy,” in Allelopathy, eds M. Reigosa, N. Pedrol, and L. González (Dordrecht: Springer), 1–9. doi: 10.1007/1-4020-4280-9_1
Clement, S. L., El-Din, N. E. D. S., Weigand, S., and Lateef, S. S. (1994). “Research achievements in plant resistance to insect pests of cool season food legumes,” in Expanding the Production and Use of Cool Season Food Legumes, eds F. J. Muehlbauer and W. J. Kaiser (Dordrecht: Springer), 290–304. doi: 10.1007/978-94-011-0798-3_16
Clúa, J., Roda, C., Zanetti, M. E., and Blanco, F. A. (2018). Compatibility between legumes and rhizobia for the establishment of a successful nitrogen-fixing symbiosis. Genes 9:125. doi: 10.3390/genes9030125
Coba de la Peña, T., Fedorova, E., Pueyo, J. J., and Lucas, M. M. (2018). The symbiosome: legume and rhizobia co-evolution toward a nitrogen-fixing organelle?. Front. Plant Sci. 8:2229. doi: 10.3389/fpls.2017.02229
Constabel, C. P., and Barbehenn, R. (2008). “Defensive roles of polyphenol oxidase in plants,” in Induced Plant Resistance to Herbivory, ed A. Schaller (Dordrecht: Springer), 253–270. doi: 10.1007/978-1-4020-8182-8_12
Conway, G. R., and Barbie, E. B. (1988). After the green revolution: sustainable and equitable agricultural development. Futures 20, 651–670. doi: 10.1016/0016-3287(88)90006-7
Corbeels, M., Naudin, K., Whitbread, A. M., Kühne, R., and Letourmy, P. (2020). Limits of conservation agriculture to overcome low crop yields in sub-Saharan Africa. Nat. Food 1, 447–454. doi: 10.18167/DVN1/DLTQWR
Cubo, M. T., Alías-Villegas, C., Balsanelli, E., Mesa, D., de Souza, E., and Espuny, M. R. (2020). Diversity of Sinorhizobium (Ensifer) meliloti bacteriophages in the rhizosphere of Medicago marina: myoviruses, filamentous and N4-like podovirus. Front. Microbiol. 11:22. doi: 10.3389/fmicb.2020.00022
D'Annolfo, R., Gemmill-Herren, B., Graeub, B., and Garibaldi, L. A. (2017). A review of social and economic performance of agroecology. Int. J. Agr. Sustain. 15, 632–644. doi: 10.1080/14735903.2017.1398123
De Lajudie, P. M., Andrews, M., Ardley, J., Eardly, B., Jumas-Bilak, E., Kuzmanović, N., et al. (2019). Minimal standards for the description of new genera and species of rhizobia and agrobacteria. Int. J. Syst. Evol. Microbiol. 69, 1852–1863. doi: 10.1099/ijsem.0.003426
De Meyer, S. E., De Beuf, K., Vekeman, B., and Willems, A. (2015). A large diversity of non-rhizobial endophytes found in legume root nodules in Flanders (Belgium). Soil Biol. Biochem. 83, 1–11. doi: 10.1016/j.soilbio.2015.01.002
Dean, J. M., Mescher, M. C., and De Moraes, C. M. (2014). Plant dependence on rhizobia for nitrogen influences induced plant defenses and herbivore performance. Int. J. Mol. Sci. 15, 1466–1480. doi: 10.3390/ijms15011466
De-la-Peña, C., and Loyola-Vargas, V. M. (2014). Biotic interactions in the rhizosphere: a diverse cooperative enterprise for plant productivity. Plant Physiol. 166, 701–719. doi: 10.1104/pp.114.241810
Delgado, A., Quemada, M., and Villalobos, F. J. (2016). “Fertilizers,” in Principles of Agronomy for Sustainable Agriculture, eds F. Villalobos and E. Fereres (Cham: Springer), 321–339. doi: 10.1007/978-3-319-46116-8_23
Deng, Z. S., Zhao, L. F., Kong, Z. Y., Yang, W. Q., Lindström, K., Wang, E. T., et al. (2011). Diversity of endophytic bacteria within nodules of the Sphaerophysa salsula in different regions of Loess Plateau in China. FEMS Microbiol. Ecol. 76, 463–475. doi: 10.1111/j.1574-6941.2011.01063.x
Denison, R. F., and Kiers, E. T. (2004). Lifestyle alternatives for rhizobia: mutualism, parasitism, and forgoing symbiosis. FEMS Microbiol. Lett. 237, 187–193. doi: 10.1111/j.1574-6968.2004.tb09695.x
Deshwal, V. K., Dubey, R. C., and Maheshwari, D. K. (2003a). Isolation of plant growth-promoting strains of Bradyrhizobium (Arachis) sp. with biocontrol potential against Macrophomina phaseolina causing charcoal rot of peanut. Curr. Sci. 84, 43–448.
Deshwal, V. K., Pandey, P., Kang, S. C., and Maheshwari, D. K. (2003b). Rhizobia as a biological control agent against soil borne plant pathogenic fungi. Indian J. Exp. Biol. 41, 1160–1164.
D'haeze, W., and Holsters, M. (2002). Nod factor structures, responses, and perception during initiation of nodule development. Glycobiology 12, 79R−105R. doi: 10.1093/glycob/12.6.79R
Dilworth, M. J., Carson, K. C., Giles, R. G., Byrne, L. T., and Glenn, A. R. (1998). Rhizobium leguminosarum bv. viciae produces a novel cyclic trihydroxamate siderophore, vicibactin. Microbiology 144, 781–791. doi: 10.1099/00221287-144-3-781
Douglas, M. R., and Tooker, J. F. (2012). Slug (Mollusca: Agriolimacidae, Arionidae) ecology and management in no-till field crops, with an emphasis on the mid-Atlantic region. J. Integr. Pest Manage. 3, C1–C9. doi: 10.1603/IPM11023
Druille, M., Cabello, M. N., Parisi, P. G., Golluscio, R. A., and Omacini, M. (2015). Glyphosate vulnerability explains changes in root-symbionts propagules viability in pampean grasslands. Agric. Ecosyst. Environ. 202, 48–55. doi: 10.1016/j.agee.2014.12.017
Duchene, O., Vian, J. F., and Celette, F. (2017). Intercropping with legume for agroecological cropping systems: complementarity and facilitation processes and the importance of soil microorganisms. A review. Agric. Ecosyst. Environ. 240, 148–161. doi: 10.1016/j.agee.2017.02.019
Dunn, M. F. (1998). Tricarboxylic acid cycle and anaplerotic enzymes in rhizobia. FEMS Microbiol. Rev. 22, 105–123. doi: 10.1111/j.1574-6976.1998.tb00363.x
Duval, S., Danyal, K., Shaw, S., Lytle, A. K., Dean, D. R., Hoffman, B. M., et al. (2013). Electron transfer precedes ATP hydrolysis during nitrogenase catalysis. Proc. Natl. Acad. Sci. U.S.A. 110, 16414–16419. doi: 10.1073/pnas.1311218110
Ebel, J., and Grisebach, H. (1988). Defense strategies of soybean against the fungus Phytophthora megasperma f. sp. glycinea: a molecular analysis. Trends Biochem. Sci. 13, 23–27.
El-Bahrawy, S. A., and Salem, F. M. (1989). Interaction between Rhizobium leguminosarum and Meloidogyne javanica nematode in broad bean under nematicide application. Z. Mikrobiol. 144, 279–281. doi: 10.1016/S0232-4393(89)80091-5
Enneking, D., and Wink, M. (2000). “Towards the elimination of anti-nutritional factors in grain legumes,” in Linking Research and Marketing Opportunities for Pulses in the 21st Century, ed R. Knight (Dordrecht: Springer), 671–683. doi: 10.1007/978-94-011-4385-1_65
Evenden, M. L. (2018). Semiochemical-Based management of the pea leaf weevil (Coleoptera: Curculionidae). Ann. Entomol. Soc. Am. 111, 154–160. doi: 10.1093/aesa/say004
FAO (2018). Sustainable Food Systems, Concept and Framework. Food and Agriculture Organization of the United Nations. Available online at: http://www.fao.org/3/ca2079en/CA2079EN.pdf
Farooq, N., Abbas, T., Tanveer, A., and Jabran, K. (2020). “Allelopathy for weed management,” in Co-evolution of Secondary Metabolites, eds J. M. Mérillon and K. Ramawat (Cham: Springer), 505–519. doi: 10.1007/978-3-319-96397-6_16
Farrar, J. F., and Jones, D. L. (2000). The control of carbon acquisition by roots. New Phytol. 147, 43–53. doi: 10.1046/j.1469-8137.2000.00688.x
Fleischman, D. E., Evans, W. R., and Miller, I. M. (1995). “Bacteriochlorophyll-Containing Rhizobium Species,” in Anoxygenic Photosynthetic Bacteria. Advances in Photosynthesis and Respiration, eds R. E. Blankenship, M.T. Madigan, and C.E. Bauer (Dordrecht: Springer), 2, 123–136. doi: 10.1007/0-306-47954-0_7
Flores-Tinoco, C. E., Tschan, F., Fuhrer, T., Margot, C., Sauer, U., Christen, M., et al. (2020). Co-catabolism of arginine and succinate drives symbiotic nitrogen fixation. Mol. Syst. Biol. 16:e9419. doi: 10.15252/msb.20199419
Forde, B. G., and Lea, P. J. (2007). Glutamate in plants: metabolism, regulation, and signalling. J. Exp. Bot. 58, 2339–2358. doi: 10.1093/jxb/erm121
Fournier, J., Teillet, A., Chabaud, M., Ivanov, S., Genre, A., Limpens, E., et al. (2015). Remodeling of the infection chamber before infection thread formation reveals a two-step mechanism for rhizobial entry into the host legume root hair. Plant Physiol. 167, 1233–1242. doi: 10.1104/pp.114.253302
Foyer, C. H., Lam, H. M., Nguyen, H. T., Siddique, K. H., Varshney, R. K., Colmer, T. D., et al. (2016). Neglecting legumes has compromised human health and sustainable food production. Nat. Plants 2, 1–10. doi: 10.1038/nplants.2016.112
Freeman, J. A. (2006). Photosystem II: The Light-Driven Water: Plastoquinone Oxidoreductase, Vol. 22. Dordrecht: Springer Science and Business Media.
Gabasawa, A. I. (2020). “Prospects for developing effective and competitive native strains of Rhizobium inoculants in Nigeria,” in Current Microbiological Research in Africa, eds A. Abia and G. Lanza (Cham: Springer), 223–256. doi: 10.1007/978-3-030-35296-7_9
Gano-Cohen, K. A., Stokes, P. J., Blanton, M. A., Wendlandt, C. E., Hollowell, A. C., Regus, J. U., et al. (2016). Nonnodulating Bradyrhizobium spp. modulate the benefits of legume-Rhizobium mutualism. Appl. Environ. Microbiol. 82, 5259–5268. doi: 10.1128/AEM.01116-16
Gao, T. G., Xu, Y. Y., Jiang, F., Li, B. Z., Yang, J. S., Wang, E. T., et al. (2015). Nodulation characterization and proteomic profiling of Bradyrhizobium liaoningense CCBAU05525 in response to water-soluble humic materials. Sci. Rep. 5:10836. doi: 10.1038/srep10836
Garcia, A. K., McShea, H., Kolaczkowski, B., and Kaçar, B. (2020). Reconstructing the evolutionary history of nitrogenases: evidence for ancestral molybdenum-cofactor utilization. Geobiology 18, 394–411. doi: 10.1111/gbi.12381
Germaine, K. J., Chhabra, S., Song, B., Brazil, D., and Dowling, D. N. (2010). Microbes and sustainable production of biofuel crops: a nitrogen perspective. Biofuels 1, 877–888. doi: 10.4155/bfs.10.67
Geurts, R., and Bisseling, T. (2002). Rhizobium Nod factor perception and signalling. Plant Cell 14, S239–S249. doi: 10.1105/tpc.002451
Ghebreamlak, S. M., and Mansoorabadi, S. O. (2020). Divergent members of the nitrogenase Superfamily: tetrapyrrole biosynthesis and beyond. ChembioChem 21, 1723–1728. doi: 10.1002/cbic.201900782
Ghoul, M., and Mitri, S. (2016). The ecology and evolution of microbial competition. Trend. Microbiol. 24, 833–845. doi: 10.1016/j.tim.2016.06.011
Gigli-Bisceglia, N., Engelsdorf, T., and Hamann, T. (2020). Plant cell wall integrity maintenance in model plants and crop species-relevant cell wall components and underlying guiding principles. Cell. Mol. Life Sci. 7, 2049–2077. doi: 10.1007/s00018-019-03388-8
Gilarte, P., Plett, J., Pendall, E., et al. (2020). Direct and indirect trophic interactions of soil nematodes impact chickpea and oat nutrition. Plant Soil. 457, 255–268. doi: 10.1007/s11104-020-04735-6
Giller, K. E. (2020). Grounding the helicopters. Geoderma 373:114302. doi: 10.1016/j.geoderma.2020.114302
Giller, K. E., and Ronner, E. (2019). The story of N2Africa: Putting nitrogen fixation to work for smallholder farmers in Africa: a flavour of the excitement and the richness of learning from N2Africa. N2Africa. doi: 10.18174/527074
Godschalx, A. L., Schädler, M., Trisel, J. A., Balkan, M. A., and Ballhorn, D. J. (2015). Ants are less attracted to the extrafloral nectar of plants with symbiotic, nitrogen-fixing rhizobia. Ecology 96, 348–354. doi: 10.1890/14-1178.1
Godschalx, A. L., Tran, V., and Ballhorn, D. J. (2017). Host plant cyanotype determines degree of rhizobial symbiosis. Ecosphere 8:e01929. doi: 10.1002/ecs2.1929
Golbeck, J. H. (2006). Photosystem I: The Light-Driven Plastocyanin: Ferredoxin Oxidoreductase, Vol. 24. Dordrecht: Springer Science and Business Media.
Gomaa, N. H., Hassan, M. O., Fahmy, G. M., González, L., Hammouda, O., and Atteya, A. M. (2015). Flavonoid profiling and nodulation of some legumes in response to the allelopathic stress of Sonchus oleraceus L. Acta Bot. Bras. 29, 553–560. doi: 10.1590/0102-33062015abb0153
Gonzalez, R. H. (1999). “Pesticide residues in developing countries–A review of residues detected in food exports from the developing world,” in Pesticide Chemistry and Bioscience, eds G. T. Brooks and T. R. Roberts (Cambridge: Woodhead Publishing Ltd.), 386–401. doi: 10.1533/9781845698416.8.386
Gopalakrishnan, S., Sathya, A., Vijayabharathi, R., Varshney, R. K., Gowda, C. L., and Krishnamurthy, L. (2015). Plant growth promoting rhizobia: challenges and opportunities. 3 Biotech 5, 355–377. doi: 10.1007/s13205-014-0241-x
Gourion, B., Berrabah, F., Ratet, P., and Stacey, G. (2015). Rhizobium–legume symbioses: the crucial role of plant immunity. Trends Plant Sci. 20, 186–194. doi: 10.1016/j.tplants.2014.11.008
Goyal, S. (2013). “Ecological role of alkaloids,” in Natural Products, eds K. G. Ramawat and J. M. Mérillon (Berlin: Springer), 149–171. doi: 10.1007/978-3-642-22144-6
Goyal, S., Lambert, C., Cluzet, S., Merillon, J. M., and Ramawat, K. G. (2012). “Secondary metabolites and plant defence,” in Plant Defence: Biological Control, eds J. Mérillon and K. Ramawat (Dordrecht: Springer), 109–138. doi: 10.1007/978-94-007-1933-0_5
Grabowska, A., Kwinta, J., and Bielawski, W. (2012). Glutamine synthetase and glutamate dehydrogenase in triticale seeds: molecular cloning and genes expression. Acta Physiol. Plant. 34, 2393–2406. doi: 10.1007/s11738-012-1085-9
Grunseich, J. M., Thompson, M. N., Aguirre, N. M., and Helms, A. M. (2020). The role of plant-associated microbes in mediating host-plant selection by insect herbivores. Plants 9:6. doi: 10.3390/plants9010006
Guerinot, M. L., Meidl, E. J., and Plessner, O. (1990). Citrate as a siderophore in Bradyrhizobium japonicum. J. Bacteriol. 172, 3298–3303. doi: 10.1128/jb.172.6.3298-3303.1990
Gunina, A., and Kuzyakov, Y. (2015). Sugars in soil and sweets for microorganisms: review of origin, content, composition, and fate. Soil Biol. Biochem. 90, 87–100. doi: 10.1016/j.soilbio.2015.07.021
Haaker, H., Szafran, M., Wassink, H., Klerk, H., and Appels, M. (1996). Respiratory control determines respiration and nitrogenase activity of Rhizobium leguminosarum bacteroids. J. Bacteriol. 178, 4555–4562. doi: 10.1128/jb.178.15.4555-4562.1996
Hailu, G., Niassy, S., Zeyaur, K. R., Ochatum, N., and Subramanian, S. (2018). Maize–legume intercropping and push–pull for management of fall armyworm, stemborers, and striga in Uganda. Agron. J. 110, 2513–2522. doi: 10.2134/agronj2018.02.0110
Haldar, S., and Sengupta, S. (2015). Plant-microbe cross-talk in the rhizosphere: insight and biotechnological potential. Open Microbiol. J. 9, 1–7. doi: 10.2174/1874285801509010001
Haldrup, A., Jensen, P. E., Lunde, C., and Scheller, H. V. (2001). Balance of power: a view of the mechanism of photosynthetic state transitions. Trend. Plant Sci. 6, 301–305. doi: 10.1016/S1360-1385(01)01953-7
Han, Q., Ma, Q., Chen, Y., Tian, B., Xu, L., Bai, Y., et al. (2020). Variation in rhizosphere microbial communities and its association with the symbiotic efficiency of rhizobia in soybean. ISME J. 14, 1915–1928. doi: 10.1038/s41396-020-0648-9
Hansen, A. P., Choudhary, D. K., Agrawal, P. K., and Varma, A. (2017). Rhizobium Biology and Biotechnology, Vol. 50. Cham: Springer. doi: 10.1007/978-3-319-64982-5
Hansen, B. L., de Cassia Pessotti, R., Fischer, M. S., Collins, A., El-Hifnawi, L., Liu, M. D., et al. (2020). Cooperation, competition, and specialized metabolism in a simplified root nodule microbiome. Mbio 11:e01917-20. doi: 10.1128/mBio.01917-20
Harrier, L. A., and Watson, C. A. (2004). The potential role of arbuscular mycorrhizal (AM) fungi in the bioprotection of plants against soil-borne pathogens in organic and/or other sustainable farming systems. Pest Manage. Sci. 60, 149–157. doi: 10.1002/ps.820
Harris, D. F., Yang, Z. Y., Dean, D. R., Seefeldt, L. C., and Hoffman, B. M. (2018). Kinetic understanding of N2 reduction versus H2 evolution at the E4 (4H) Janus state in the three nitrogenases. Biochemistry 57, 5706–5714. doi: 10.1021/acs.biochem.8b00784
Hassan, S., and Mathesius, U. (2012). The role of flavonoids in root–rhizosphere signalling: opportunities and challenges for improving plant–microbe interactions. J. Exp. Bot. 63, 3429–3444. doi: 10.1093/jxb/err430
Hassen, A. I., Habig, J. H., and Lamprecht, S. C. (2018). Assessing root nodule microsymbionts in healthy and declined rooibos (Aspalathus linearis burm f.) at a plantation in South Africa. J. Plant Interact. 13, 277–279. doi: 10.1080/17429145.2018.1473514
Hassen, A. I., Lamprecht, S. C., and Bopape, F. L. (2020). Emergence of β-rhizobia as new root nodulating bacteria in legumes and current status of the legume–rhizobium host specificity dogma. World J. Microbiol. Biotechnol. 36, 1–13. doi: 10.1007/s11274-020-2811-x
Hayat, S., Faraz, A., and Faizan, M. (2017). “Root exudates: composition and impact on plant–microbe interaction,” in Biofilms Plant and Soil Health, eds I. Ahmad and M. F. Husain (Hoboken, NJ: Wiley Blackwell), 179–193.
Herbstová, M., Tietz, S., Kinzel, C., Turkina, M. V., and Kirchhoff, H. (2012). Architectural switch in plant photosynthetic membranes induced by light stress. Proc. Natl. Acad. Sci. U.S.A. 109, 20130–20135. doi: 10.1073/pnas.1214265109
Herren, H. R., Hilbeck, A., Hoffmann, U., Home, R., Levidow, L., Müller, A., et al. (2015). Feeding the People: Agroecology for Nourishing the World and Transforming the Agri-Food System. Brussels: IFOAM Organics Europe.
Hoffman, B. M., Dean, D. R., and Seefeldt, L. C. (2009). Climbing nitrogenase: toward a mechanism of enzymatic nitrogen fixation. Acc. Chem. Res. 42, 609–619. doi: 10.1021/ar8002128
Hoffman, B. M., Lukoyanov, D., Yang, Z. Y., Dean, D. R., and Seefeldt, L. C. (2014). Mechanism of nitrogen fixation by nitrogenase: the next stage. Chem. Rev. 114, 4041–4062. doi: 10.1021/cr400641x
Hopkins, W. G., and Hüner, N. P. A. (2009). Introduction to Plant Physiology, 2nd Edn. New York, NY: John Wiley and Sons, Inc.
Horiuchi, J. I., Prithiviraj, B., Bais, H. P., Kimball, B. A., and Vivanco, J. M. (2005). Soil nematodes mediate positive interactions between legume plants and rhizobium bacteria. Planta 222, 848–857. doi: 10.1007/s00425-005-0025-y
Hortal, S., Lozano, Y. M., Bastida, F., Armas, C., Moreno, J. L., Garcia, C., et al. (2017). Plant-plant competition outcomes are modulated by plant effects on the soil bacterial community. Sci. Rep. 7, 1–9. doi: 10.1038/s41598-017-18103-5
Hu, Y., and Ribbe, M. W. (2016). Biosynthesis of the metalloclusters of nitrogenases. Annu. Rev. Biochem. 85, 455–483. doi: 10.1146/annurev-biochem-060614-034108
Huang, H. C., and Erickson, R. S. (2007). Effect of seed treatment with Rhizobium leguminosarum on Pythium damping-off, seedling height, root nodulation, root biomass, shoot biomass, and seed yield of pea and lentil. J. Phytopathol. 155, 31–37. doi: 10.1111/j.1439-0434.2006.01189.x
Huang, J. S. (2001). “Rhizobium-legume symbiosis and the effects of diseases on nodulation and nitrogen fixation,” in Plant Pathogenesis and Resistance, ed J. S. Huang (Dordrecht: Springer), 175–236. doi: 10.1007/978-94-017-2687-0_4
Hunt, S., and Layzell, D. B. (1993). Gas exchange of legume nodules and the regulation of nitrogenase activity. Annu. Rev. Plant Physiol. Plant Mol. Biol. 44, 483–511.
Husch, T., and Reiher, M. (2017). Mechanistic consequences of chelate ligand stabilization on nitrogen fixation by Yandulov–Schrock-type complexes. ACS Sustaina. Chem. Eng. 5, 10527–10537. doi: 10.1021/acssuschemeng.7b02518
ICSU (2017). A Guide to SDG Interactions: From Science to Implementation, eds D. J. Griggs, M. Nilsson, A. Stevance, and D. McCollum. Paris: International Council for Science (ICSU). doi: 10.24948/2017.01
Igiehon, N. O., and Babalola, O. O. (2018). Below-ground-above-ground plant-microbial interactions: focusing on soybean, rhizobacteria and mycorrhizal fungi. Open Microbiol. 12:261. doi: 10.2174/1874285801812010261
Irisarri, P., Cardozo, G., Tartaglia, C., Reyno, R., Gutiérrez, P., Lattanzi, F. A., et al. (2019). Selection of competitive and efficient rhizobia strains for white clover. Front. Microbiol. 10:768. doi: 10.3389/fmicb.2019.00768
Irmer, S., Podzun, N., Langel, D., Heidemann, F., Kaltenegger, E., Schemmerling, B., et al. (2015). New aspect of plant–rhizobia interaction: alkaloid biosynthesis in Crotalaria depends on nodulation. Proc. Natl. Acad. Sci. U.S.A. 112, 4164–4169. doi: 10.1073/pnas.1423457112
Ismail, M. H., and Atef, N. M. (1998). Impact of bean yellow mosaic virus (BYMV) on Rhizobium nodulation in faba bean. Phytopathol. Mediter. 37, 58–68.
Jagendorf, A. T. (2002). Photophosphorylation and the chemiosmotic perspective. Photosynth. Res. 73, 233–241. doi: 10.1023/A:1020415601058
Jangir, H., Bhardwaj, A., and Das, M. (2020). Larger root nodules increased Fe, Mo, Mg, P, Ca, Mn, K in the roots and higher yield in chickpea grown from nano FeS 2 pre-treated seeds: emulating nitrogenase. Appl. Nanosci. 10, 445–454. doi: 10.1007/s13204-019-01238-4
Jiang, Y., Liu, M., Zhang, J., Chen, Y., Chen, X., Chen, L., et al. (2017). Nematode grazing promotes bacterial community dynamics in soil at the aggregate level. ISME J. 11, 2705–2717. doi: 10.1038/ismej.2017.120
Johnson, G. N. (2011). Physiology of PSI cyclic electron transport in higher plants. Biochim. Biophys. Acta 1807, 384–389. doi: 10.1016/j.bbabio.2010.11.009
Johnson, S. N., Birch, A. N. E., Gregory, P. J., and Murray, P. J. (2006). The ‘mother knows best'principle: should soil insects be included in the preference–performance debate? Ecol. Entomol. 31, 395–401. doi: 10.1111/j.1365-2311.2006.00776.x
Johnson, S. N., Gregory, P. J., Greenham, J. R., Zhang, X., and Murray, P. J. (2005). Attractive properties of an isoflavonoid found in white clover root nodules on the clover root weevil. J. Chem. Ecol. 31, 2223–2229. doi: 10.1007/s10886-005-6355-1
Joosten, L., and van Veen, J. A. (2011). Defensive properties of pyrrolizidine alkaloids against microorganisms. Phytochem. Rev. 10, 127–136. doi: 10.1007/s11101-010-9204-y
Kafle, A., Garcia, K., Wang, X., Pfeffer, P. E., Strahan, G. D., and Bücking, H. (2019). Nutrient demand and fungal access to resources control the carbon allocation to the symbiotic partners in tripartite interactions of Medicago truncatula. Plant Cell Environ. 42, 270–284. doi: 10.1111/pce.13359
Kakraliya, S. K., Singh, U., Bohra, A., Choudhary, K. K., Kumar, S., Meena, R. S., et al. (2018). “Nitrogen and legumes: a meta-analysis,” in Legumes for Soil Health and Sustainable Management, eds R. Meena, A. Das, G. Yadav, and R. Lal (Singapore: Springer), 277–314. doi: 10.1007/978-981-13-0253-4_9
Karoney, E. M., Ochieno, D. M. W., Baraza, D. L., Muge, E. K., Nyaboga, E. N., and Naluyange, V. (2020). Rhizobium improves nutritive suitability and tolerance of Phaseolus vulgaris to Colletotrichum lindemuthianum by boosting organic nitrogen content. Appl. Soil Ecol. 149:103534. doi: 10.1016/j.apsoil.2020.103534
Karowe, D. N., and Radi, J. K. (2011). Are the phytoestrogens genistein and daidzein anti-herbivore defenses? A test using the gypsy moth (Lymantria dispar). J. Chem. Ecol. 37:830. doi: 10.1007/s10886-011-9986-4
Kaschuk, G., Kuyper, T. W., Leffelaar, P. A., Hungria, M., and Giller, K. E. (2009). Are the rates of photosynthesis stimulated by the carbon sink strength of rhizobial and arbuscular mycorrhizal symbioses? Soil Biol. Biochem. 41, 1233–1244. doi: 10.1016/j.soilbio.2009.03.005
Kaschuk, G., Leffelaar, P. A., Giller, K. E., Alberton, O., Hungria, M., and Kuyper, T. W. (2010). Responses of legumes to rhizobia and arbuscular mycorrhizal fungi: a meta-analysis of potential photosynthate limitation of symbioses. Soil Biol. Biochem. 42, 125–127. doi: 10.1016/j.soilbio.2009.10.017
Katayama, N., Nishida, T., Zhang, Z. Q., and Ohgushi, T. (2010). Belowground microbial symbiont enhances plant susceptibility to a spider mite through change in soybean leaf quality. Popul. Ecol. 52, 499–506. doi: 10.1007/s10144-010-0207-8
Katayama, N., Silva, A. O., Kishida, O., Ushio, M., Kita, S., and Ohgushi, T. (2014). Herbivorous insect decreases plant nutrient uptake: the role of soil nutrient availability and association of below-ground symbionts. Ecol. Entomol. 39, 511–518. doi: 10.1111/een.12125
Katayama, N., Zhang, Z. Q., and Ohgushi, T. (2011a). Community-wide effects of below-ground rhizobia on above-ground arthropods. Ecol. Entomol. 36, 43–51. doi: 10.1111/j.1365-2311.2010.01242.x
Katayama, N., Zhang, Z. Q., and Ohgushi, T. (2011b). Belowground rhizobia positively affect abundances of aboveground sap feeding and leaf chewing herbivores. J. Plant Interact. 6, 173–174. doi: 10.1080/17429145.2010.536264
Kawaguchi, A., Kondo, K. I., and Inoue, K. (2012). Biological control of apple crown gall by nonpathogenic Rhizobium vitis strain VAR03-1. J. Gen. Plant Pathol. 78, 287–293. doi: 10.1007/s10327-012-0388-4
Kempel, A., Brandl, R., and Schädler, M. (2009). Symbiotic soil microorganisms as players in aboveground plant–herbivore interactions–the role of rhizobia. Oikos 118, 634–640. doi: 10.1111/j.1600-0706.2009.17418.x
Khan, M. S., Zaidi, A., and Rizvi, P. Q. (2006). Biotoxic effects of herbicides on growth, nodulation, nitrogenase activity, and seed production in chickpeas. Commun. Soil Sci. Plant Anal. 37, 1783–1793. doi: 10.1080/00103620600710645
Khan, M. S., Zaidi, A., and Wani, P. A. (2007). Role of phosphate-solubilizing microorganisms in sustainable agriculture — A review. Agron. Sustain. Dev. 27, 29–43. doi: 10.1051/agro:2006011
Khan, Z., Midega, C., Pittchar, J., Pickett, J., and Bruce, T. (2011). Push—pull technology: a conservation agriculture approach for integrated management of insect pests, weeds and soil health in Africa: UK government's Foresight Food and Farming Futures project. Int. J. Agr. Sustain. 9, 162–170. doi: 10.3763/ijas.2010.0558
Khanna, A. Q., Borowicz, V. A., and Jones, M. A. (1999). Effects of nitrogen fertilizer and defoliation on growth, foliar nitrogen and foliar coumestrol concentrations of soybean. Trans. Ill. State Acad. Sci. 92, 167–179.
Kiba, T., and Krapp, A. (2016). Plant nitrogen acquisition under low availability: regulation of uptake and root architecture. Plant Cell Physiol. 57, 707–714. doi: 10.1093/pcp/pcw052
Kidd, D. R., Ryan, M. H., Hahne, D., Haling, R. E., Lambers, H., Sandral, G. A., et al. (2018). The carboxylate composition of rhizosheath and root exudates from twelve species of grassland and crop legumes with special reference to the occurrence of citramalate. Plant Soil 424, 389–403. doi: 10.1007/s11104-017-3534-0
Kluson, R. A. (1995). “Intercropping allelopathic crops with nitrogen-fixing legume crops: a tripartite legume symbiosis perspective,” in Allelopathy - Organisms, Processes, and Applications, eds K. M. Inderjit, M. Dakshini, and F. A. Einhellig (Washington DC: ACS Publications), 193–210. doi: 10.1021/bk-1995-0582.ch015
Kniazeva, M., and Ruvkun, G. (2019). Rhizobium induces DNA damage in Caenorhabditis elegans intestinal cells. Proc. Natl. Acad. Sci. U.S.A. 116, 3784–3792. doi: 10.1073/pnas.1815656116
Knight, J. D., and Cammell, M. E. (1994). A decision support system for forecasting infestations of the black bean aphid, Aphis fabae Scop., on spring-sown field beans, Vicia faba. Comput. Electron. Agric. 10, 269–279. doi: 10.1016/0168-1699(94)90046-9
Kopittke, P. M., Menzies, N. W., Wang, P., McKenna, B. A., and Lombi, E. (2019). Soil and the intensification of agriculture for global food security. Environ. Int. 132:105078. doi: 10.1016/j.envint.2019.105078
Kosslak, R. M., Bookland, R., Barkei, J., Paaren, H. E., and Appelbaum, E. R. (1987). Induction of Bradyrhizobium japonicum common nod genes by isoflavones isolated from Glycine max. Proc. Natl. Acad. Sci. U.S.A. 84, 7428–7432. doi: 10.1073/pnas.84.21.7428
Kou-Giesbrecht, S., and Menge, D. (2019). Nitrogen-fixing trees could exacerbate climate change under elevated nitrogen deposition. Nat. Commun. 10, 1–8. doi: 10.1038/s41467-019-09424-2
Kraiser, T., Gras, D. E., Gutiérrez, A. G., González, B., and Gutiérrez, R. A. (2011). A holistic view of nitrogen acquisition in plants. J. Exp. Bot. 62, 1455–1466. doi: 10.1093/jxb/erq425
Kuzyakov, Y., and Xu, X. (2013). Competition between roots and microorganisms for nitrogen: mechanisms and ecological relevance. New Phytol. 198, 656–669. doi: 10.1111/nph.12235
Laguerre, G., van Berkum, P., Amarger, N., and Prévost, D. (1997). Genetic diversity of rhizobial symbionts isolated from legume species within the genera Astragalus, Oxytropis, and Onobrychis. Appl. Environ. Microbiol. 63, 4748–4758.
Lakhran, L., and Ahir, R. R. (2020). In-vivo evaluation of different fungicides, plant extracts, biocontrol agents and organics amendments for management of dry root rot of chickpea caused by Macrophomina phaseolina. Legume Res. 43, 140–145. doi: 10.18805/LR-3939
Lardi, M., de Campos, S. B., Purtschert, G., Eberl, L., and Pessi, G. (2017). Competition experiments for legume infection identify Burkholderia phymatum as a highly competitive β-rhizobium. Front. Microbiol. 8:1527. doi: 10.3389/fmicb.2017.01527
Lea, P. J., and Miflin, B. J. (2018). Nitrogen assimilation and its relevance to crop improvement. Annu. Plant Rev. 42, 1–40. doi: 10.1002/9781119312994.apr0448
Lewin, R. A. (1982). Symbiosis and parasitism: definitions and evaluations. Bioscience 32, 254–260. doi: 10.2307/1308530
Lie, T. A., Nijland, G. J., and Waluyo, S. H. (1988). “Competition between nodulating and non-nodulating Rhizobium strains: delay of nodulation,” in Physiological Limitations and the Genetic Improvement of Symbiotic Nitrogen Fixation, eds F. O'Gara, S. Manian, and J. J. Drevon (Dordrecht: Springer), 127–136. doi: 10.1007/978-94-009-1401-8_14
Lindström, K., and Mousavi, S. A. (2019). Effectiveness of nitrogen fixation in rhizobia. Microbiol. Biotechnol. 13, 1314–1335. doi: 10.1111/1751-7915.13517
Liu, A., Contador, C. A., Fan, K., and Lam, H. M. (2018). Interaction and regulation of carbon, nitrogen, and phosphorus metabolisms in root nodules of legumes. Front. Plant Sci. 9:1860. doi: 10.3389/fpls.2018.01860
Liu, X., Vrieling, K., and Klinkhamer, P. G. (2017). Interactions between plant metabolites affect herbivores: a study with pyrrolizidine alkaloids and chlorogenic acid. Front. Plant Sci. 8, p.903. doi: 10.3389/fpls.2017.00903
Lodwig, E., and Poole, P. (2003). Metabolism of Rhizobium bacteroids. Crit. Rev. Plant Sci. 22, 37–78. doi: 10.1080/713610850
Long, S. R. (1989). Rhizobium genetics. Annu. Rev. Genet. 23, 483–506. doi: 10.1146/annurev.ge.23.120189.002411
Lu, C., Zhang, J., Zhang, Q., Li, L., and Kuang, T. (2001). Modification of photosystem II photochemistry in nitrogen deficient maize and wheat plants. J. Plant Physiol. 158, 1423–1430. doi: 10.1078/0176-1617-00501
Lu, Y., Song, S., Wang, R., Liu, Z., Meng, J., Sweetman, A. J., et al. (2015). Impacts of soil and water pollution on food safety and health risks in China. Environ. Int. 77, 5–15. doi: 10.1016/j.envint.2014.12.010
Lv, G. Y., Guo, X. G., Xie, L. P., Xie, C. G., Zhang, X. H., Yang, Y., et al. (2017). Molecular characterization, gene evolution, and expression analysis of the fructose-1, 6-bisphosphate aldolase (FBA) gene family in wheat (Triticum aestivum L.). Front. Plant Sci. 8:1030. doi: 10.3389/fpls.2017.01030
Mahdhi, M., Tounekti, T., and Khemira, H. (2018). Invasive character of Prosopis juliflora facilitated by its allelopathy and a wide mutualistic interaction with soil microorganisms. J. Biol. Sci. 18, 115–123. doi: 10.3923/jbs.2018.115.123
Mahmud, K., Makaju, S., Ibrahim, R., and Missaoui, A. (2020). Current progress in nitrogen fixing plants and microbiome research. Plants 9:97. doi: 10.3390/plants9010097
Maier, R. J. (2004). “Nitrogen fixation and respiration: two processes linked by the energetic demands of nitrogenase,” in Respiration in Archaea and Bacteria. Advances in Photosynthesis and Respiration, ed D. Zannoni (Dordrecht: Springer), 101–120. doi: 10.1007/978-1-4020-3163-2_5
Maj, D., Wielbo, J., Marek-Kozaczuk, M., and Skorupska, A. (2010). Response to flavonoids as a factor influencing competitiveness and symbiotic activity of Rhizobium leguminosarum. Microbiol. Res. 165, 50–60. doi: 10.1016/j.micres.2008.06.002
Makoi, J. H., and Ndakidemi, P. A. (2012). Allelopathy as protectant, defence and growth stimulants in legume cereal mixed culture systems. New Zeal. J. Crop Hort. Sci. 40, 161–186. doi: 10.1080/01140671.2011.630737
Males, J., and Griffiths, H. (2017). Stomatal biology of CAM plants. Plant Physiol. 174, 550–560. doi: 10.1104/pp.17.00114
Mangeni B. C. Were H. K. Ndong'a M. Mukoye B. (2020). Incidence and severity of bean common mosaic disease and resistance of popular bean cultivars to the disease in western Kenya. J. Phytopathol. 168, 501–515. doi: 10.1111/jph.12928
Mao, G., Turner, M., Yu, O., and Subramanian, S. (2013). miR393 and miR164 influence indeterminate but not determinate nodule development. Plant Signal. Behav. 8:e26753. doi: 10.4161/psb.26753
Martínez-Hidalgo, P., and Hirsch, A. M. (2017). The nodule microbiome: N2-fixing rhizobia do not live alone. Phytobiomes 1, 70–82. doi: 10.1094/PBIOMES-12-16-0019-RVW
Marwat, S. K., Khan, M. A., Ahmad, M., Zafar, M., Ahmad, F., and Nazir, A. (2009). Taxonomic studies of nodulated leguminous weeds from the flora of North Western part (Dera Ismail Khan) of Pakistan. Afr. J. Biotechnol. 8, 2163–2168.
Masclaux-Daubresse, C., Daniel-Vedele, F., Dechorgnat, J., Chardon, F., Gaufichon, L., and Suzuki, A. (2010). Nitrogen uptake, assimilation, and remobilization in plants: challenges for sustainable and productive agriculture. Ann. Bot. 105, 1141–1157. doi: 10.1093/aob/mcq028
Masclaux-Daubresse, C., Reisdorf-Cren, M., Pageau, K., Lelandais, M., Grandjean, O., Kronenberger, J., et al. (2006). Glutamine synthetase-glutamate synthase pathway and glutamate dehydrogenase play distinct roles in the sink-source nitrogen cycle in tobacco. Plant Physiol. 140, 444–456. doi: 10.1104/pp.105.071910
Mathesius, U. (2019). “The role of the flavonoid pathway in Medicago truncatula in root nodule formation. A review,” in The Model Legume Medicago truncatula, ed F. de Bruijn (Hoboken, NJ: Wiley Press), 434–438. doi: 10.1002/9781119409144.ch54
Matthews, L. J., and Davis, T. M. (1990). Anatomical comparison of wild-type and non-nodulating mutant chickpea (Cicer arietinum). Can. J. Bot. 68, 1201–1207. doi: 10.1139/b90-152
Mattson, W. J. Jr (1980). Herbivory in relation to plant nitrogen content. Annu. Rev. Ecol. Syst. 11, 119–161. doi: 10.1146/annurev.es.11.110180.001003
Matusova, R., Rani, K., Verstappen, F. W., Franssen, M. C., Beale, M. H., and Bouwmeester, H. J. (2005). The strigolactone germination stimulants of the plant-parasitic Striga and Orobanche spp. are derived from the carotenoid pathway. Plant Physiol. 139, 920–934. doi: 10.1104/pp.105.061382
McAdam, E. L., Hugill, C., Fort, S., Samain, E., Cottaz, S., Davies, N. W., et al. (2017). Determining the site of action of strigolactones during nodulation. Plant Physiol. 175, 529–542. doi: 10.1104/pp.17.00741
McCullum, C., Benbrook, C., Knowles, L., Roberts, S., and Schryver, T. (2003). Application of modern biotechnology to food and agriculture: food systems perspective. J. Nutr. Educ. Behav. 35, 319–332. doi: 10.1016/S1499-4046(06)60347-3
McGlynn, S. E., Boyd, E. S., Peters, J. W., and Orphan, V. J. (2013). Classifying the metal dependence of uncharacterized nitrogenases. Front. Microbiol. 3:419. doi: 10.3389/fmicb.2012.00419
Mehboob, I., Naveed, M., and Zahir, Z. A. (2009). Rhizobial association with non-legumes: mechanisms and applications. Crit. Rev. Plant Sci. 28, 432–456. doi: 10.1080/07352680903187753
Mehboob, I., Naveed, M., Zahir, Z. A., and Sessitsch, A. (2013). “Potential of rhizosphere bacteria for improving Rhizobium-legume symbiosis,” in Plant microbe symbiosis: Fundamentals and Advances, eds V. Meena, B. Maurya, J. Verma, and R. Meena (New Delhi: Springer), 305–349. doi: 10.1007/978-81-322-1287-4_12
Mendoza, R., García, I., Depalma, D., and López, C. F. (2016). Competition and growth of a grass–legume mixture fertilised with nitrogen and phosphorus: effect on nutrient acquisition, root morphology and symbiosis with soil microorganisms. Crop Pasture Sci. 67, 629–640. doi: 10.1071/CP15257
Mendoza-Suárez, M. A., Geddes, B. A., Sánchez-Cañizares, C., Ramírez-González, R. H., Kirchhelle, C., Jorrin, B., et al. (2020). Optimizing Rhizobium-legume symbioses by simultaneous measurement of rhizobial competitiveness and N2 fixation in nodules. Proc. Natl. Acad. Sci. U.S.A. 117, 9822–9831. doi: 10.1073/pnas.1921225117
Meng, L., Zhang, A., Wang, F., Han, X., Wang, D., and Li, S. (2015). Arbuscular mycorrhizal fungi and rhizobium facilitate nitrogen uptake and transfer in soybean/maize intercropping system. Front. Plant Sci. 6:339. doi: 10.3389/fpls.2015.00339
Mergaert, P., Van Montagu, M., and Holsters, M. (1997). Molecular mechanisms of Nod factor diversity. Mol. Microbiol. 25, 811–817. doi: 10.1111/j.1365-2958.1997.mmi526.x
Michelet, L., Zaffagnini, M., Morisse, S., Sparla, F., Pérez-Pérez, M. E., Francia, F., et al. (2013). Redox regulation of the Calvin–Benson cycle: something old, something new. Front. Plant Sci. 4:470. doi: 10.3389/fpls.2013.00470
Miller, G., Honig, A., Stein, H., Suzuki, N., Mittler, R., and Zilberstein, A. (2009). Unraveling Δ1-pyrroline-5-carboxylate-proline cycle in plants by uncoupled expression of proline oxidation enzymes. J. Biol. Chem. 284, 26482–26492. doi: 10.1074/jbc.M109.009340
Minasny, B., Fiantis, D., Mulyanto, B., Sulaeman, Y., and Widyatmanti, W. (2020). Global soil science research collaboration in the 21st century: Time to end helicopter research. Geoderma 373:114299. doi: 10.1016/j.geoderma.2020.114299
Mishra, J., Singh, R., and Arora, N. K. (2017). “Plant growth-promoting microbes: diverse roles in agriculture and environmental sustainability,” in Probiotics and Plant Health, eds V. Kumar, M. Kumar, and S. Sharma (Singapore: Springer), 71–111. doi: 10.1007/978-981-10-3473-2_4
Mitsch, M. J., Cowie, A., and Finan, T. M. (2018). Succinate transport is not essential for symbiotic nitrogen fixation by Sinorhizobium meliloti or Rhizobium leguminosarum. Appl. Environ. Microbiol. 84:e01561–e01517. doi: 10.1128/AEM.01561-17
Miyakawa, T., Xu, Y., and Tanokura, M. (2020). Molecular basis of strigolactone perception in root-parasitic plants: aiming to control its germination with strigolactone agonists/antagonists. Cell.Mol. Life Sci. 77, 1103–1113. doi: 10.1007/s00018-019-03318-8
Modi, M., Shah, K. S., and Modi, V. V. (1985). Isolation and characterization of catechol-like siderophore from cowpea Rhizobium RA-1. Arch. Microbiol. 141, 156–158. doi: 10.1007/BF00423277
Morrow, J. G., Huggins, D. R., Carpenter-Boggs, L. A., and Reganold, J. P. (2016). Evaluating measures to assess soil health in long-term agroecosystem trials. Soil Sci. Soc. Am. J. 80, 450–462. doi: 10.2136/sssaj2015.08.0308
Moura, E. G., Carvalho, C. S., Bucher, C. P., Souza, J. L., Aguiar, A. C., Junior, A. S., et al. (2020). Diversity of rhizobia and importance of their interactions with legume trees for feasibility and sustainability of the tropical agrosystems. Diversity 12:206. doi: 10.3390/d12050206
Mrabet, M., Mnasri, B., Romdhane, S. B., Laguerre, G., Aouani, M. E., and Mhamdi, R. (2006). Agrobacterium strains isolated from root nodules of common bean specifically reduce nodulation by Rhizobium gallicum. FEMS Microbiol. Ecol. 56, 304–309. doi: 10.1111/j.1574-6941.2006.00069.x
Mullineaux, C. W. (2005). Function and evolution of grana. Trends Plant Sci. 10, 521–525. doi: 10.1016/j.tplants.2005.09.001
Muresu, R., Maddau, G., Delogu, G., Cappuccinelli, P., and Squartini, A. (2010). Bacteria colonizing root nodules of wild legumes exhibit virulence-associated properties of mammalian pathogens. Antonie Van Leeuwenhoek 97, 143–153. doi: 10.1007/s10482-009-9396-6
Muresu, R., Porceddu, A., Sulas, L., and Squartini, A. (2019). Nodule-associated microbiome diversity in wild populations of Sulla coronaria reveals clues on the relative importance of culturable rhizobial symbionts and co-infecting endophytes. Microbiol. Res. 221, 10–14. doi: 10.1016/j.micres.2019.01.004
Naamala, J., Jaiswal, S. K., and Dakora, F. D. (2016). Antibiotics resistance in Rhizobium: type, process, mechanism, and benefit for agriculture. Curr. Microbiol. 72, 804–816. doi: 10.1007/s00284-016-1005-0
Nadler, K. D., Johnston, A. W., Chen, J. W., and John, T. R. (1990). A Rhizobium leguminosarum mutant defective in symbiotic iron acquisition. J. Bacteriol. 172, 670–677. doi: 10.1128/jb.172.2.670-677.1990
Naik, K., Mishra, S., Srichandan, H., Singh, P. K., and Sarangi, P. K. (2019). Plant growth promoting microbes: potential link to sustainable agriculture and environment. Biocatal. Agric. Biotechnol. 21, 101326. doi: 10.1016/j.bcab.2019.101326
Naluyange, V., Ochieno, D. M., Maingi, J. M., Ombori, O., Mukaminega, D., Amoding, A., et al. (2014). Compatibility of Rhizobium inoculant and water hyacinth compost formulations in rosecoco bean and consequences on Aphis fabae and Colletotrichum lindemuthianum infestations. Appl. Soil Ecol. 76, 68–77. doi: 10.1016/j.apsoil.2013.12.011
Naluyange, V., Ochieno, D. M., Wandahwa, P., Odendo, M., Maingi, J. M., Amoding, A., et al. (2016). Belowground influence of Rhizobium inoculant and water hyacinth composts on yellow bean infested by Aphis fabae and Colletotrichum lindemuthianum under field conditions. J. Plant Stud. 5, 32–41. doi: 10.5539/jps.v5n2p32
Nandhini, D. U., Somasundaram, E., and Amanullah, M. M. (2018). Effect of rhizobial nod factors (lipo-chitooligosaccharide) on seedling growth of blackgram under salt stress. Legum. Res. 41, 159–162. doi: 10.18805/LR-3597
Nelson, N., and Junge, W. (2015). Structure and energy transfer in photosystems of oxygenic photosynthesis. Annu. Rev. Biochem. 84, 659–683. doi: 10.1146/annurev-biochem-092914-041942
Newton, W. E. (1997). “Molybdenum-nitrogenase: structure and function,” in Biological Fixation of Nitrogen for Ecology and Sustainable Agriculture, eds A. Legocki, H. Bothe, and A. Pühler (Berlin; Heidelberg: Springer), 9–12. doi: 10.1007/978-3-642-59112-9_2
Norsworthy, J. K., McClelland, M., Griffith, G., Bangarwa, S. K., and Still, J. (2010). Evaluation of legume cover crops and weed control programs in conservation-tillage, enhanced glyphosate-resistant cotton. Weed Technol. 24, 269–274. doi: 10.1614/WT-D-09-00037.1
Nunes, M. A., Ramalho, J. C., and Dias, M. A. (1993). Effect of nitrogen supply on the photosynthetic performance of leaves from coffee plants exposed to bright light. J. Exp. Bot. 44, 893–899. doi: 10.1093/jxb/44.5.893
Ochieno, D. M. (2020). Towards consensus on the transfer of Fusarium oxysporum V5w2-enhanced tissue culture banana technology to farmers through public-private partnerships in East Africa. Sci. Afr. 10:e00605. doi: 10.1016/j.sciaf.2020.e00605
Ochieno, D. M. W. (2010). Endophytic control of Cosmopolites sordidus and Radopholus similis using Fusarium oxysporum V5w2 in tissue culture banana (Ph.D. Thesis). Wageningen University, Wageningen, Netherlands. Available online at: https://library.wur.nl/WebQuery/wda/lang/1948566
Ofori, F., and Stern, W. R. (1987). Cereal–legume intercropping systems. Adv. Agron. 41, 41–90. doi: 10.1016/S0065-2113(08)60802-0
O'Hara, G. W. (2001). Nutritional constraints on root nodule bacteria affecting symbiotic nitrogen fixation: a review. Aust. J. Exp. Agric. 41, 417–433. doi: 10.1071/EA00087
Olanrewaju, O. S., Ayangbenro, A. S., Glick, B. R., and Babalola, O. O. (2019). Plant health: feedback effect of root exudates-rhizobiome interactions. Appl. Microbiol. Biotechnol. 103, 1155–1166. doi: 10.1007/s00253-018-9556-6
Omar, S. A., and Abd-Alla, M. H. (2000). Physiological aspects of fungi isolated from root nodules of faba bean (Vicia faba L.). Microbiol. Res. 154, 339–347. doi: 10.1016/S0944-5013(00)80008-7
Onyango, D. M., Orina, P. S., Ramkat, R. C., Kowenje, C., Githukia, C. M., Lusweti, D., et al. (2020). Review of current state of knowledge of microcystin and its impacts on fish in Lake Victoria. Lake. Reserv. Res. Manage. 25, 350–361. doi: 10.1111/lre.12328
Oono, R., Denison, R. F., and Kiers, E. T. (2009). Controlling the reproductive fate of rhizobia: how universal are legume sanctions? New Phytol. 183, 967–979. doi: 10.1111/j.1469-8137.2009.02941.x
Orellana, R. G., and Fan, F. F. (1978). Nodule infection by bean yellow mosaic virus in Phaseolus vulgaris. Appl. Environ. Microbiol. 36, 814–818.
Orr, A., Kambombo, B., Roth, C., Harris, D., and Doyle, V. (2015). Adoption of integrated food-energy systems: improved cookstoves and pigeonpea in southern Malawi. Exp. Agric. 51, 191–209. doi: 10.1017/S0014479714000222
Osborne, C. P., and Beerling, D. J. (2006). Nature's green revolution: the remarkable evolutionary rise of C4 plants. Philos. Trans. R. Soc. Lond. B. Biol. Sci. 361, 173–194. doi: 10.1098/rstb.2005.1737
Oula, D. A., Nyongesah, J. M., Odhiambo, G., and Wagai, S. (2020). The effectiveness of local strains of Fusarium oxysporum f. sp. strigae to control Striga hermonthica on local maize in western Kenya. Food Sci. Nutr. 8, 4352–4360. doi: 10.1002/fsn3.1732
Oulhen, N., Schulz, B. J., and Carrier, T. J. (2016). English translation of Heinrich Anton de Bary's 1878 speech, ‘Die Erscheinung der Symbiose' (‘De la symbiose'). Symbiosis 69, 131–139. doi: 10.1007/s13199-016-0409-8
Paarlberg, R. (2009). Starved for Science: How Biotechnology Is Being Kept Out of Africa. Cambridge: Harvard University Press.
Pajares, S., and Bohannan, B. J. (2016). Ecology of nitrogen fixing, nitrifying, and denitrifying microorganisms in tropical forest soils. Front. Microbiol. 7:1045. doi: 10.3389/fmicb.2016.01045
Parker, M. A. (2001). Mutualism as a constraint on invasion success for legumes and rhizobia. Divers. Distrib. 7, 125–136. doi: 10.1046/j.1472-4642.2001.00103.x
Patel, H. N., Chakraborty, R. N., and Desai, S. B. (1988). Isolation and partial characterization of phenolate siderophore from Rhizobium leguminosarum IARI 102. FEMS Microbiol. Lett. 56, 131–134. doi: 10.1111/j.1574-6968.1988.tb03164.x
Patriarca, E. J., Tatè, R., and Iaccarino, M. (2002). Key role of bacterial metabolism in Rhizobium-plant symbiosis. Microbiol. Mol. Biol. Rev. 66, 203–222. doi: 10.1128/MMBR.66.2.203-222.2002
Paungfoo-Lonhienne, C., Wang, W., Yeoh, Y. K., and Halpin, N. (2017). Legume crop rotation suppressed nitrifying microbial community in a sugarcane cropping soil. Sci. Rep. 7, 1–7. doi: 10.1038/s41598-017-17080-z
Peláez-Vico, M. A., Bernabéu-Roda, L., Kohlen, W., Soto, M. J., and López-Ráez, J. A. (2016). Strigolactones in the Rhizobium-legume symbiosis: stimulatory effect on bacterial surface motility and down-regulation of their levels in nodulated plants. Plant Sci. 245, 119–127. doi: 10.1016/j.plantsci.2016.01.012
Pereira, J. L., Galdino, T. V., Silva, G. A., Picanço, M. C., Silva, A. A., Corrêa, A. S., et al. (2018). Effects of glyphosate on the non-target leaf beetle Cerotoma arcuata (Coleoptera: Chrysomelidae) in field and laboratory conditions. J. Environ. Sci. Heal. B 53, 447–453. doi: 10.1080/03601234.2018.1455363
Pickett, J. A., Hooper, A. M., Midega, C. A. O., and Khan, Z. R. (2013). “Allelopathy,” in Parasitic Orobanchaceae, eds D. Joel, J. Gressel, and L. Musselman (Berlin; Heidelberg: Springer), 459–467. doi: 10.1007/978-3-642-38146-1_25
Pineda, A., Zheng, S. J., Van Loon, J. J. A., and Dicke, M. (2012). Rhizobacteria modify plant–aphid interactions: a case of induced systemic susceptibility. Plant Biol. 14, 83–90. doi: 10.1111/j.1438-8677.2011.00549.x
Pinnola, A., and Bassi, R. (2018). Molecular mechanisms involved in plant photoprotection. Biochem. Soc. Trans. 46, 467–482. doi: 10.1042/BST20170307
Plaxton, W. C., and Podestá, F. E. (2006). The functional organization and control of plant respiration. Crit. Rev. Plant Sci. 25, 159–198. doi: 10.1080/07352680600563876
Poole, P., and Allaway, D. (2000). Carbon and nitrogen metabolism in Rhizobium. Adv. Microbiol. Physiol. 43, 117–163. doi: 10.1016/S0065-2911(00)43004-3
Poole, P., Ramachandran, V., and Terpolilli, J. (2018). Rhizobia: from saprophytes to endosymbionts. Nat. Rev. Microbiol. 16:291. doi: 10.1038/nrmicro.2017.171
Poolman, M. G., Fell, D. A., and Raines, C. A. (2003). Elementary modes analysis of photosynthate metabolism in the chloroplast stroma. Eur. J. Biochem. 270, 430–439. doi: 10.1046/j.1432-1033.2003.03390.x
Postma, J., Hok-A-Hin, C. H., and Van Veen, J. A. (1990). Role of microniches in protecting introduced Rhizobium leguminosarum biovar trifolii against competition and predation in soil. Appl. Environ. Microbiol. 56, 495–502.
Poveda, J., Eugui, D., and Velasco, P. (2020). Natural control of plant pathogens through glucosinolates: An effective strategy against fungi and oomycetes. Phytochem. Rev. 19, 1045–1059. doi: 10.1007/s11101-020-09699-0
Preece, C., and Peñuelas, J. (2020). A return to the wild: root exudates and food security. Trends Plant Sci. 25, 14–21. doi: 10.1016/j.tplants.2019.09.010
Prell, J., and Poole, P. (2006). Metabolic changes of rhizobia in legume nodules. Trends Microbiol. 14, 161–168. doi: 10.1016/j.tim.2006.02.005
Pugashetti, B. K., Angle, J. S., and Wagner, G. H. (1982). Soil microorganisms antagonistic towards Rhizobium japonicum. Soil Biol. Biochem. 14, 45–49. doi: 10.1016/0038-0717(82)90075-X
Pulido, H., Mauck, K. E., De Moraes, C. M., and Mescher, M. C. (2019). Combined effects of mutualistic rhizobacteria counteract virus-induced suppression of indirect plant defences in soya bean. Proc. R. Soc. B 286:20190211. doi: 10.1098/rspb.2019.0211
Püschel, D., Janoušková, M., Voríšková, A., Gryndlerová, H., Vosátka, M., and Jansa, J. (2017). Arbuscular mycorrhiza stimulates biological nitrogen fixation in two Medicago spp. through improved phosphorus acquisition. Front. Plant Sci. 8:390. doi: 10.3389/fpls.2017.00390
Qin, L., Jiang, H., Tian, J., Zhao, J., and Liao, H. (2011). Rhizobia enhance acquisition of phosphorus from different sources by soybean plants. Plant Soil 349, 25–36. doi: 10.1007/s11104-011-0947-z
Qiu, X. M., Sun, Y. Y., Ye, X. Y., and Li, Z. G. (2019). Signaling role of glutamate in plants. Front. Plant Sci. 10:1743. doi: 10.3389/fpls.2019.01743
Raghavendra, A. S., Padmasree, K., and Saradadevi, K. (1994). Interdependence of photosynthesis and respiration in plant cells: interactions between chloroplasts and mitochondria. Plant Sci. 97, 1–14. doi: 10.1016/0168-9452(94)90101-5
Raines, C. A. (2003). The Calvin cycle revisited. Photosynth. Res. 75, 1–10. doi: 10.1023/A:1022421515027
Raines, C. A. (2011). Increasing photosynthetic carbon assimilation in C3 plants to improve crop yield: current and future strategies. Plant Physiol. 155, 36–42. doi: 10.1104/pp.110.168559
Ramirez, C., and Alexander, M. (1980). Evidence suggesting protozoan predation on Rhizobium associated with germinating seeds and in the rhizosphere of beans (Phaseolus vulgaris L.). Appl. Environ. Microbiol. 40, 492–499.
Ranjbar Sistani, N., Kaul, H. P., Desalegn, G., and Wienkoop, S. (2017). Rhizobium impacts on seed productivity, quality, and protection of Pisum sativum upon disease stress caused by Didymella pinodes: phenotypic, proteomic, and metabolomic traits. Front. Plant Sci. 8:1961. doi: 10.3389/fpls.2017.01961
Rasmann, S., and Turlings, T. C. (2016). Root signals that mediate mutualistic interactions in the rhizosphere. Curr. Opin. Plant Biol. 32, 62–68. doi: 10.1016/j.pbi.2016.06.017
Raven, J. A., Handley, L. L., MacFarlane, J. J., McInroy, S., McKenzie, L., and Richards, J. H. (1988). The role of CO2 uptake by roots and CAM in acquisition of inorganic C by plants of the isoetid life-form: a review, with new data on Eriocaulon decangulare L. New Phytol. 108, 125–148. doi: 10.1111/j.1469-8137.1988.tb03690.x
Renger, G., and Renger, T. (2008). Photosystem II: the machinery of photosynthetic water splitting. Photosynth. Res. 98, 53–80. doi: 10.1007/s11120-008-9345-7
Rice, E. L. (1992). “Allelopathic effects on nitrogen cycling,” in Allelopathy, eds S. J. H. Rizvi and V. Rizvi (Dordrecht: Springer), 31–58. doi: 10.1007/978-94-011-2376-1_4
Rioux, C. R., Jordan, D. C., and Rattray, J. B. (1986). Iron requirement of Rhizobium leguminosarum and secretion of anthranilic acid during growth on an iron-deficient medium. Arch. Biochem. Biophys. 248, 175–182. doi: 10.1016/0003-9861(86)90414-5
Roach, T., and Krieger-Liszkay, A. (2014). Regulation of photosynthetic electron transport and photoinhibition. Curr. Protein Pept. Sci. 15, 351–362.
Rubio, L. M., and Ludden, P. W. (2008). Biosynthesis of the iron-molybdenum cofactor of nitrogenase. Annu. Rev. Microbiol. 62, 93–111. doi: 10.1146/annurev.micro.62.081307.162737
Rüttimann-Johnson, C., Staples, C. R., Rangaraj, P., Shah, V. K., and Ludden, P. W. (1999). A vanadium and iron cluster accumulates on VnfX during iron-vanadium-cofactor synthesis for the vanadium nitrogenase in Azotobacter vinelandii. J. Biol. Chem. 274, 18087–18092. doi: 10.1074/jbc.274.25.18087
Sachs, I., and Silk, D. (1991). Final Report of the Food Energy Nexus Programme of the United Nations University, 1983-1987. UNU-FEN.
Sachs, J. L., Quides, K. W., and Wendlandt, C. E. (2018). Legumes versus rhizobia: a model for ongoing conflict in symbiosis. New Phytol. 219, 1199–1206. doi: 10.1111/nph.15222
Saha, L., and Bauddh, K. (2020). “Sustainable agricultural approaches for enhanced crop productivity, better soil health, and improved ecosystem services,” in Ecological and Practical Applications for Sustainable Agriculture, eds K. Bauddh, S. Kumar, R. Singh, and J. Korstad (Singapore: Springer), 1–23. doi: 10.1007/978-981-15-3372-3_1
Saïdi, S., Chebil, S., Gtari, M., and Mhamdi, R. (2013). Characterization of root-nodule bacteria isolated from Vicia faba and selection of plant growth promoting isolates. World J. Microbiol. Biotechnol. 29, 1099–1106. doi: 10.1007/s11274-013-1278-4
Sánchez-Chino, X., Jiménez-Martínez, C., Dávila-Ortiz, G., Álvarez-González, I., and Madrigal-Bujaidar, E. (2015). Nutrient and nonnutrient components of legumes, and its chemopreventive activity: a review. Nutr. Cancer 67, 401–410. doi: 10.1080/01635581.2015.1004729
Santamaría, R. I., Bustos, P., Sepúlveda-Robles, O., Lozano, L., Rodríguez, C., Fernández, J. L., et al. (2014). Narrow-host-range bacteriophages that infect Rhizobium etli associate with distinct genomic types. Appl. Environ. Microbiol. 80, 446–454. doi: 10.1128/AEM.02256-13
Sara, S., Morad, M., and Reza, C. M. (2013). Effects of seed inoculation by Rhizobium strains on chlorophyll content and protein percentage in common bean cultivars (Phaseolus vulgaris L.). Int. J. Biosci. 3, 1–8. doi: 10.12692/ijb/3.3.1-8
Schandry, N., and Becker, C. (2020). Allelopathic Plants: Models for Studying Plant–Interkingdom Interactions. Trends Plant Sci. 25, 176–185. doi: 10.1016/j.tplants.2019.11.004
Scheublin, T. R., Ridgway, K. P., Young, J. P. W., and Van Der Heijden, M. G. (2004). Nonlegumes, legumes, and root nodules harbor different arbuscular mycorrhizal fungal communities. Appl. Environ. Microbiol. 70, 6240–6246. doi: 10.1128/AEM.70.10.6240-6246.2004
Scheublin, T. R., and Van Der Heijden, M. G. (2006). Arbuscular mycorrhizal fungi colonize nonfixing root nodules of several legume species. New Phytol. 172, 732–738. doi: 10.1111/j.1469-8137.2006.01858.x
Schütte, G., Eckerstorfer, M., Rastelli, V., Reichenbecher, W., Restrepo-Vassalli, S., Ruohonen-Lehto, M., et al. (2017). Herbicide resistance and biodiversity: agronomic and environmental aspects of genetically modified herbicide-resistant plants. Environ. Sci. Eur. 29:5. doi: 10.1186/s12302-016-0100-y
Selleck, G. W. (1972). The antibiotic effects of plants in laboratory and field. Weed Sci. 20, 189–194.
Selvakumar, G., Panneerselvam, P., and Ganeshamurthy, A. N. (2013). “Legume root nodule associated bacteria,” in Plant Microbe Symbiosis: Fundamentals and Advances, ed N. Arora (New Delhi: Springer), 215–232. doi: 10.1007/978-81-322-1287-4_8
Sha, Z., Lv, T., Staal, M., Ma, X., Wen, Z., Li, Q., et al. (2020). Effect of combining urea fertilizer with P and K fertilizers on the efficacy of urease inhibitors under different storage conditions. J. Soils Sediments 20, 2130–2140. doi: 10.1007/s11368-019-02534-w
Shah, T., Lateef, S., and Noor, M. A. (2020). “Carbon and nitrogen cycling in agroecosystems: an overview,” in Carbon and Nitrogen Cycling in Soil, eds R. Datta, R. Meena, S. Pathan, and M. Ceccherini (Singapore: Springer), 1–15. doi: 10.1007/978-981-13-7264-3_1
Sharaf, H., Rodrigues, R. R., Moon, J., Zhang, B., Mills, K., and Williams, M. A. (2019). Unprecedented bacterial community richness in soybean nodules vary with cultivar and water status. Microbiome 7, 1–18. doi: 10.1186/s40168-019-0676-8
Sharma, S. K., Singh, S. K., Ramesh, A., Sharma, P. K., Varma, A., Ahmad, E., et al. (2018). “Microbial genetic resources: status, conservation, and access and benefit-sharing regulations,” in Microbial Resource Conservation, eds S. Sharma and A. Varma (Cham: Springer), 1–33. doi: 10.1007/978-3-319-96971-8_1
Shen, Q., Liu, L., Wang, L., and Wang, Q. (2018). Indole primes plant defense against necrotrophic fungal pathogen infection. PLoS ONE 13:e0207607. doi: 10.1371/journal.pone.0207607
Shimazaki, K. I., and Zeiger, E. (1985). Cyclic and noncyclic photophosphorylation in isolated guard cell chloroplasts from Vicia faba L. Plant Physiol. 78, 211–214. doi: 10.1104/pp.78.2.211
Šibul F. Orčić D. Vasić M. Anačkov G. Nadpal J. Savić A. . (2016). Phenolic profile, antioxidant and anti-inflammatory potential of herb and root extracts of seven selected legumes. Ind. Crops Prod. 83, 641–653. doi: 10.1016/j.indcrop.2015.12.057
Signorelli, S., Sainz, M., Tabares-da Rosa, S., and Monza, J. (2020). The role of nitric oxide in nitrogen fixation by legumes. Front. Plant Sci. 11:521. doi: 10.3389/fpls.2020.00521
Sikora, R., Coyne, D., Hallmann, J., and Timper, P. (2018). Plant Parasitic Nematodes in Subtropical and Tropical Agriculture, 3rd Edn. Wallingford, CT: CABI.
Smith, C. R., Blair, P. L., Boyd, C., Cody, B., Hazel, A., Hedrick, A., et al. (2016). Microbial community responses to soil tillage and crop rotation in a corn/soybean agroecosystem. Ecol. Evol. 6, 8075–8084. doi: 10.1002/ece3.2553
Soto, M. J., Sanjuan, J., and Olivares, J. (2006). Rhizobia and plant-pathogenic bacteria: common infection weapons. Microbiology 152, 3167–3174. doi: 10.1099/mic.0.29112-0
Soundararajan, R. P., Chitra, N., and Geetha, S. (2013). Host plant resistance to insect pests of grain legumes-A review. Agric. Rev. 34, 176–187. doi: 10.5958/j.0976-0741.34.3.002
Spaink, H. P. (1995). The molecular basis of infection and nodulation by rhizobia: the ins and outs of sympathogenesis. Annu. Rev. Phytopathol. 33, 345–368. doi: 10.1146/annurev.py.33.090195.002021
Spini, G., Decorosi, F., Cerboneschi, M., Tegli, S., Mengoni, A., Viti, C., et al. (2016). Effect of the plant flavonoid luteolin on Ensifer meliloti 3001 phenotypic responses. Plant Soil 399, 159–178. doi: 10.1007/s11104-015-2659-2
Stagnari, F., Maggio, A., Galieni, A., and Pisante, M. (2017). Multiple benefits of legumes for agriculture sustainability: an overview. Chem. Biol. Technol. Agric. 4:2. doi: 10.1186/s40538-016-0085-1
Steinkellner, S., Lendzemo, V., Langer, I., Schweiger, P., Khaosaad, T., Toussaint, J. P., et al. (2007). Flavonoids and strigolactones in root exudates as signals in symbiotic and pathogenic plant-fungus interactions. Molecules 12, 1290–1306. doi: 10.3390/12071290
Stevenson, P. C., Dhillon, M. K., Sharma, H. C., and Bouhssini, M. E. (2007). “Insect pests of lentil and their management,” in Lentil, eds S. S. Yadav, D. L. McNeil, and P. C. Stevenson (Dordrecht: Springer), 331–348. doi: 10.1007/978-1-4020-6313-8_20
Strand, Å., Zrenner, R., Trevanion, S., Stitt, M., Gustafsson, P., and Gardeström, P. (2000). Decreased expression of two key enzymes in the sucrose biosynthesis pathway, cytosolic fructose-1, 6-bisphosphatase and sucrose phosphate synthase, has remarkably different consequences for photosynthetic carbon metabolism in transgenic Arabidopsis thaliana. Plant J. 23, 759–770. doi: 10.1046/j.1365-313x.2000.00847.x
Sugiyama, A., Shitan, N., and Yazaki, K. (2008). Signaling from soybean roots to rhizobium: an ATP-binding cassette-type transporter mediates genistein secretion. Plant Signal Behav. 3, 38–40. doi: 10.4161/psb.3.1.4819
Sumberg, J., Keeney, D., and Dempsey, B. (2012). Public agronomy: Norman Borlaug as ‘brand hero'for the Green Revolution. J. Dev. Stud. 48, 1587–1600. doi: 10.1080/00220388.2012.713470
Taha, A. E. S. H.Y. (1993). “Nematode interactions with root-nodule bacteria,” in Nematode Interactions, ed M. W. Khan (Dordrecht: Springer), 55–78. doi: 10.1007/978-94-011-1488-2_4
Tajini, F., Trabelsi, M., and Drevon, J. J. (2012). Combined inoculation with Glomus intraradices and Rhizobium tropici CIAT899 increases phosphorus use efficiency for symbiotic nitrogen fixation in common bean (Phaseolus vulgaris L.). Saudi J. Biol. Sci. 19, 157–163. doi: 10.1016/j.sjbs.2011.11.003
Tao, L., Hunter, M. D., and de Roode, J. C. (2017). Microbial root mutualists affect the predators and pathogens of herbivores above ground: mechanisms, magnitudes, and missing links. Front. Ecol. Evol. 5:160. doi: 10.3389/fevo.2017.00160
Taranto, F., Pasqualone, A., Mangini, G., Tripodi, P., Miazzi, M. M., Pavan, S., et al. (2017). Polyphenol oxidases in crops: biochemical, physiological and genetic aspects. Int. J. Mol. Sci. 18:377. doi: 10.3390/ijms18020377
Tawaraya, K., Horie, R., Saito, S., Wagatsuma, T., Saito, K., and Oikawa, A. (2014). Metabolite profiling of root exudates of common bean under phosphorus deficiency. Metabolites 4, 599–611. doi: 10.3390/metabo4030599
Taylor, B. N., Simms, E. L., and Komatsu, K. J. (2020). More than a functional group: diversity within the legume–Rhizobia mutualism and its relationship with ecosystem function. Diversity 12:50. doi: 10.3390/d12020050
Teixeira, L. F. M., Coutinho, L. C. H., and Franco, A. A. (1996). Effects of Cerotoma arcuata (Coleoptera: Chrysomelidae) on predation of nodules and on N2 fixation of Phaseolus vulgaris. J. Econ. Entomol. 89, 165–169. doi: 10.1093/jee/89.1.165
Temple, S. J., Vance, C. P., and Gantt, J. S. (1998). Glutamate synthase and nitrogen assimilation. Trend. Plant Sci. 3, 51–56. doi: 10.1016/S1360-1385(97)01159-X
Terpolilli, J. J., Hood, G. A., and Poole, P. S. (2012). What determines the efficiency of N2-fixing Rhizobium-legume symbioses? Adv. Microb. Physiol. 60, 325–389. doi: 10.1016/B978-0-12-398264-3.00005-X
Thamer, S., Schädler, M., Bonte, D., and Ballhorn, D. J. (2011). Dual benefit from a belowground symbiosis: nitrogen fixing rhizobia promote growth and defense against a specialist herbivore in a cyanogenic plant. Plant Soil 341, 209–219. doi: 10.1007/s11104-010-0635-4
Thilakarathna, M. S., and Raizada, M. N. (2018). Challenges in using precision agriculture to optimize symbiotic nitrogen fixation in legumes: progress, limitations, and future improvements needed in diagnostic testing. Agronomy 8:78. doi: 10.3390/agronomy8050078
Thornton, P. E., Lamarque, J. F., Rosenbloom, N. A., and Mahowald, N. M. (2007). Influence of carbon-nitrogen cycle coupling on land model response to CO2 fertilization and climate variability. Glob. Biogeochem. Cycles 21, 1–5. doi: 10.1029/2006GB002868
Tian, T., Reverdy, A., She, Q., Sun, B., and Chai, Y. (2020). The role of rhizodeposits in shaping rhizomicrobiome. Environ. Microbiol. Rep. 12, 160–172. doi: 10.1111/1758-2229.12816
Tóth, K., and Stacey, G. (2015). Does plant immunity play a critical role during initiation of the legume-rhizobium symbiosis? Front. Plant Sci. 6:401. doi: 10.3389/fpls.2015.00401
Townsend, A. R., Howarth, R. W., Bazzaz, F. A., Booth, M. S., Cleveland, C. C., Collinge, S. K., et al. (2003). Human health effects of a changing global nitrogen cycle. Front. Ecol. Environ. 1, 240–246. doi: 10.1890/1540-9295(2003)001[0240:HHEOAC]2.0.CO;2
Trap, J., Bonkowski, M., Plassard, C., Villenave, C., and Blanchart, E. (2016). Ecological importance of soil bacterivores for ecosystem functions. Plant Soil 398, 1–24. doi: 10.1007/s11104-015-2671-6
Triplett, E. W. (1999). “Antibiosis as a means to enhance nodulation competitiveness by Rhizobium inoculum strains under agricultural conditions,” in Highlights of Nitrogen Fixation Research, eds E. Martlnez and G. Hernández (Boston, MA: Springer). doi: 10.1007/978-1-4615-4795-2_45
Tully, K., and Ryals, R. (2017). Nutrient cycling in agroecosystems: balancing food and environmental objectives. Agroecol. Sustain. Food Syst. 41, 761–798. doi: 10.1080/21683565.2017.1336149
Udvardi, M., and Poole, P. S. (2013). Transport and metabolism in legume-rhizobia symbioses. Annu. Rev. Plant Biol. 64, 781–805. doi: 10.1146/annurev-arplant-050312-120235
UN DESA (2017). World Population Projected to Reach 9.8 Billion in 2050, and 11.2 Billion in 2100. New York, NY: United Nations Department of Economic and Social Affairs.Available online at: https://www.un.org/development/desa/en/news/population/world-population-prospects-2017.html (accessed July 30, 2020).
van Emden, H. F., and Peakall, D. B. (1996). Beyond Silent Spring: Integrated Pest Management and Chemical Safety. London: Chapman and Hall Ltd.
Vanlerberghe, G. C., Dahal, K., Alber, N. A., and Chadee, A. (2020). Photosynthesis, respiration and growth: a carbon and energy balancing act for alternative oxidase. Mitochondrion 52, 197–211. doi: 10.1016/j.mito.2020.04.001
Verhagen, F. J., Duyts, H., and Laanbroek, H. J. (1993). Effects of grazing by flagellates on competition for ammonium between nitrifying and heterotrophic bacteria in soil columns. Appl. Environ. Microbiol. 59, 2099–2106.
Verma, M., Singh, A., Dwivedi, D. H., and Arora, N. K. (2020). Zinc and phosphate solubilizing Rhizobium radiobacter (LB2) for enhancing quality and yield of loose leaf lettuce in saline soil. Environ. Sustain. 3, 209–218. doi: 10.1007/s42398-020-00110-4
Vokou, D., Chalkos, D., and Karamanoli, K. (2006). “Microorganisms and allelopathy: a one-sided approach,” in Allelopathy, eds M. Reigosa, N. Pedrol, and L. González (Dordrecht: Springer), 341–371. doi: 10.1007/1-4020-4280-9_15
Volpiano, C. G., Lisboa, B. B., Granada, C. E., São José, J. F. B., de Oliveira, A. M. R., Beneduzi, A., et al. (2019). “Rhizobia for biological control of plant diseases,” in Microbiome in Plant Health and Disease, eds V. Kumar, R. Prasad, M. Kumar, and D. Choudhary (Singapore: Springer), 315–336. doi: 10.1007/978-981-13-8495-0_14
Walker, C., Muniz, M. F. B., Rolim, J. M., Martins, R. R. O., Rosenthal, V. C., Maciel, C. G., et al. (2016). Morphological and molecular characterization of Cladosporium cladosporioides species complex causing pecan tree leaf spot. Genet. Mol. Res. 15, 1–11. doi: 10.4238/gmr.15038714
Wang B. and Lan, C.Q. (2010). “Biofixation of carbon dioxide (CO2) by microorganisms,” in Developments and Innovation in Carbon Dioxide (CO2) Capture and Storage Technology, ed M. M. Maroto-Valer (Cambridge: Woodhead Publishing), 411–432. doi: 10.1533/9781845699581.4.411
Wang, Q., Liu, J., and Zhu, H. (2018). Genetic and molecular mechanisms underlying symbiotic specificity in legume-Rhizobium interactions. Front. Plant Sci. 9:313. doi: 10.3389/fpls.2018.00313
Wang, Y., and Lu, Y. (2020). Evaluating the potential health and economic effects of nitrogen fertilizer application in grain production systems of China. J. Clean. Prod. 264:121635. doi: 10.1016/j.jclepro.2020.121635
Warra, A. A., and Prasad, M. N. V. (2020). “African perspective of chemical usage in agriculture and horticulture—their impact on human health and environment,” in Agrochemicals Detection, Treatment and Remediation, eds M. Narasimha and V. Prasad (Butterworth-Heinemann), 401–436. doi: 10.1016/B978-0-08-103017-2.00016-7
Webb, K. J., Cookson, A., Allison, G., Sullivan, M. L., and Winters, A. L. (2014). Polyphenol oxidase affects normal nodule development in red clover (Trifolium pratense L.). Front. Plant Sci. 5:700. doi: 10.3389/fpls.2014.00700
Webber, A. N., and Lubitz, W. (2001). P700: the primary electron donor of photosystem I. Biochim. Biophys. Acta 1507, 61–79. doi: 10.1016/S0005-2728(01)00198-0
Weerasinghe, R. R., Bird, D. M., and Allen, N. S. (2005). Root-knot nematodes and bacterial Nod factors elicit common signal transduction events in Lotus japonicus. Proc. Natl. Acad. Sci. U.S.A. 102, 3147–3152. doi: 10.1073/pnas.0407926102
Werquin, M., Ackermann, H. W., and Levesque, R. C. (1988). A study of 33 bacteriophages of Rhizobium meliloti. Appl. Environ. Microbiol. 54, 188–196.
Weston, L. A., and Mathesius, U. (2013). Flavonoids: their structure, biosynthesis and role in the rhizosphere, including allelopathy. J. Chem. Ecol. 39, 283–297. doi: 10.1007/s10886-013-0248-5
Whipps, J. M. (2001). Microbial interactions and biocontrol in the rhizosphere. J. Exp. Bot. 52, 487–511. doi: 10.1093/jexbot/52.suppl_1.487
Wille, L., Messmer, M. M., Studer, B., and Hohmann, P. (2019). Insights to plant–microbe interactions provide opportunities to improve resistance breeding against root diseases in grain legumes. Plant Cell Environ. 42, 20–40. doi: 10.1111/pce.13214
Wink, M. (2013). Evolution of secondary metabolites in legumes (Fabaceae). S. Afr. J. Bot. 89, 164–175. doi: 10.1016/j.sajb.2013.06.006
Wood, C. W., Pilkington, B. L., Vaidya, P., Biel, C., and Stinchcombe, J. R. (2018). Genetic conflict with a parasitic nematode disrupts the legume–rhizobia mutualism. Evol. Lett. 2, 233–245. doi: 10.1002/evl3.51
Wu, G. L., Liu, Y., Tian, F. P., and Shi, Z. H. (2017). Legumes functional group promotes soil organic carbon and nitrogen storage by increasing plant diversity. Land Degrad. Dev. 28, 1336–1344. doi: 10.1002/ldr.2570
Wu, L. J., Wang, H. Q., Wang, E. T., Chen, W. X., and Tian, C. F. (2011). Genetic diversity of nodulating and non-nodulating rhizobia associated with wild soybean (Glycine soja Sieb. and Zucc.) in different ecoregions of China. FEMS Microbiol. Ecol. 76, 439–450. doi: 10.1111/j.1574-6941.2011.01064.x
Wydrzynski, T., and Satoh, K. (2005). Photosystem II: Light-Induced Water: Plastoquinone Oxidoreductase, Advances in Photosynthesis and Respiration, Vol. 22. Dordrecht: Springer.
Xu, H., Yang, Y., Tian, Y., Xu, R., Zhong, Y., and Liao, H. (2020). Rhizobium inoculation drives the shifting of rhizosphere fungal community in a host genotype dependent manner. Front. Microbiol. 10:3135. doi: 10.3389/fmicb.2019.03135
Yergaliyev, T., Alexander-Shani, R., Dimeretz, H., Pivonia, S., Bird, D. M., Rachmilevitch, S., et al. (2020). The bacterial community structure dynamics in Meloidogyne incognita infected roots and its role in worm-microbiome interactions. bioRxiv. 1–30. doi: 10.1101/2020.03.25.007294
Yoneyama, K., Xie, X., Kim, H. I., Kisugi, T., Nomura, T., Sekimoto, H., et al. (2012). How do nitrogen and phosphorus deficiencies affect strigolactone production and exudation? Planta 235, 1197–1207. doi: 10.1007/s00425-011-1568-8
Young, J. P. W., and Haukka, K. (1996). Diversity and phylogeny of rhizobia. New Phytol. 133:87–94. doi: 10.1111/j.1469-8137.1996.tb04344.x
Yunes, J. S. (2019). “Cyanobacterial toxins,” in Cyanobacteria. From Basic Science to Applications, eds A. K. Mishra, D. N. Tiwari, and A. N. Rai (London: Academic Press), 443–458. doi: 10.1016/B978-0-12-814667-5.00022-2
Zahran, H. H. (1999). Rhizobium-legume symbiosis and nitrogen fixation under severe conditions and in an arid climate. Microbiol. Mol. Biol. Rev. 63, 968–989. doi: 10.1128/MMBR.63.4.968-989.1999
Zarafi, A. B., Elzein, A., Abdulkadir, D. I., Beed, F., and Akinola, O. M. (2015). Host range studies of Fusarium oxysporum f. sp. strigae meant for the biological control of Striga hermonthica on maize and sorghum. Arch. Phytopathol. Plant Prot. 48, 1–9. doi: 10.1080/03235408.2014.880580
Zeng, S., Tjepkema, J. D., and Berg, R. H. (1989). Gas diffusion pathway in nodules of Casuarina cunninghamiana. Plant Soil 118, 119–123. doi: 10.1007/BF02232796
Zhang, C., Wang, X., Zhang, F., Dong, L., Wu, J., Cheng, Q., et al. (2017). Phenylalanine ammonia-lyase2. 1 contributes to the soybean response towards Phytophthora sojae infection. Sci. Rep. 7, 1–13. doi: 10.1038/s41598-017-07832-2
Zhang, Z., Li, Y., Pan, X., Shao, S., Liu, W., Wang, E. T., et al. (2019). Aeschynomene indica-nodulating rhizobia lacking Nod factor synthesis genes: diversity and evolution in Shandong Peninsula, China. Appl. Environ. Microbiol. 85, e00782–19. doi: 10.1128/AEM.00782-19
Zhang, Z., Xiong, S., Wei, Y., Meng, X., Wang, X., and Ma, X. (2017). The role of glutamine synthetase isozymes in enhancing nitrogen use efficiency of N-efficient winter wheat. Sci. Rep. 7, 1–12. doi: 10.1038/s41598-017-01071-1
Zhao, J., Wang, X., Wang, X., and Fu, S. (2014). Legume-soil interactions: legume addition enhances the complexity of the soil food web. Plant Soil 385, 273–286. doi: 10.1007/s11104-014-2234-2
Zhao, Y., Bian, S.-M., Zhou, H.-N., and Huang, J.-F. (2006). Diversity of nitrogenase systems in diazotrophs. J. Integr. Plant Biol. 48, 745–755. doi: 10.1111/j.1744-7909.2006.00271.x
Zohaib, A., Anjum, S. A., Jabbar, A., Tabassum, T., Abbas, T., and Nazir, U. (2017). Allelopathic effect of leguminous weeds on rate, synchronization and time of germination, and biomass partitioning in rice. Planta Daninha 35, e017160380. doi: 10.1590/s0100-83582017350100032
Keywords: multitrophic interactions, phytochemicals, Rhizobium, nitrogen, carbon, plant tolerance
Citation: Ochieno DMW, Karoney EM, Muge EK, Nyaboga EN, Baraza DL, Shibairo SI and Naluyange V (2021) Rhizobium-Linked Nutritional and Phytochemical Changes Under Multitrophic Functional Contexts in Sustainable Food Systems. Front. Sustain. Food Syst. 4:604396. doi: 10.3389/fsufs.2020.604396
Received: 09 September 2020; Accepted: 03 December 2020;
Published: 11 January 2021.
Edited by:
Saveetha Kandasamy, A&L Canada Laboratories, CanadaReviewed by:
Senthilkumar Murugaiyan, Tamil Nadu Agricultural University, IndiaGiovanna Frugis, National Research Council (CNR), Italy
Copyright © 2021 Ochieno, Karoney, Muge, Nyaboga, Baraza, Shibairo and Naluyange. This is an open-access article distributed under the terms of the Creative Commons Attribution License (CC BY). The use, distribution or reproduction in other forums is permitted, provided the original author(s) and the copyright owner(s) are credited and that the original publication in this journal is cited, in accordance with accepted academic practice. No use, distribution or reproduction is permitted which does not comply with these terms.
*Correspondence: Victoria Naluyange, dmljbHV5QGdtYWlsLmNvbQ==