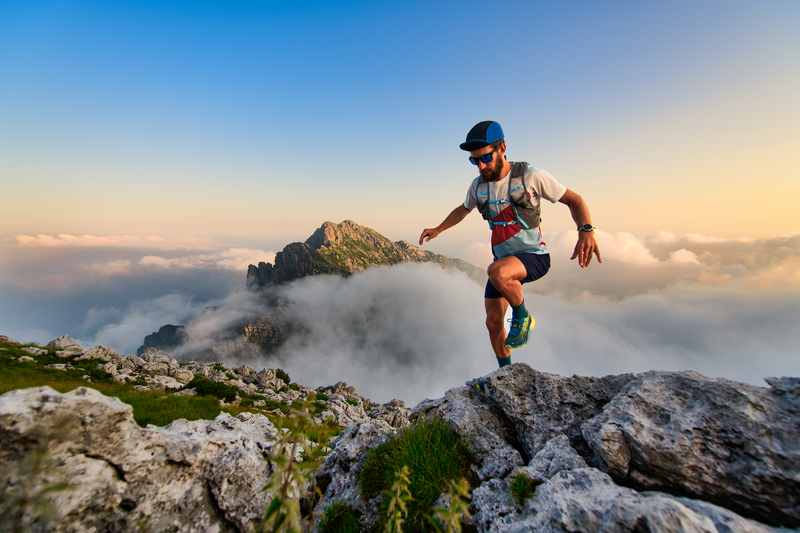
95% of researchers rate our articles as excellent or good
Learn more about the work of our research integrity team to safeguard the quality of each article we publish.
Find out more
ORIGINAL RESEARCH article
Front. Sustain. Food Syst. , 25 September 2020
Sec. Crop Biology and Sustainability
Volume 4 - 2020 | https://doi.org/10.3389/fsufs.2020.579976
This article is part of the Research Topic Plant Growth-Promoting Microorganisms for Sustainable Agricultural Production View all 50 articles
While the effects of lipopolysaccharides (LPS) of plant pathogenic bacteria in induction of plant defense responses have been characterized, the role of LPS of beneficial rhizobacteria on plant growth is less clear. In this study, we assessed the in vitro effects of LPS from the rhizobacterium Azospirillum brasilense Sp245 on early growth of wheat seedlings (Triticum aestivum) and on some biochemical responses related to growth, like peroxidase (POD) enzyme activity and Ca2+ availability. Four days after treating the seedlings with various concentrations of A. brasilense LPS (10 to 1,000 μg/mL), the growth of the seedlings was enhanced as evidenced by significant increase in leaf and root lengths as well as fresh weight. These increases were similar or even higher to those resulting from inoculation with the rhizobacteria. POD enzyme activity increased significantly in roots treated with LPS and was concentration dependent. Salicylhydroxamic acid, an inhibitor of peroxidase activity, decreased POD activity and plant growth promoted by LPS. Lanthanum, an inhibitor of calcium channels, and EGTA, inhibited plant growth and POD activity promoted by LPS, while the calcium ionophore A23187 alone was able to increase plant growth and POD activity. In summary, the results suggest that isolated LPS of A. brasilense have the capacity to promote early wheat seedling growth and that POD enzyme activity and Ca2+ levels are involved in the LPS-mediated biological activity.
Conventional agriculture is facing either reduced production or increased costs, or both, as a result in the weakening of general soil vitality, groundwater purity, and beneficial microbe (Singh et al., 2011). This have derived in the addition of increasing amount of fertilizers and pesticides to the soil. Although conventional methods enabled large increases in crop yields, their effects on soil and ecology health fail to be considered as an option for future agriculture (Singh et al., 2011). Sustainable agriculture is a broad based concept that involve agricultural management practices and technology. Numerous soil microorganisms contribute to the sustainability of the crop yield by improving the efficiency of nutrient acquisition and enhancing plant health.
Azospirillum belong to soil microorganisms that can be useful in the sustainable agriculture. They are Gram-negative free-living nitrogen-fixing bacteria grouped in plant growth-promoting rhizobacteria (PGPR) (Okon, 1994; Okon and Vanderleyden, 1997; Zawoznika and Groppa, 2019). Inoculation with Azospirillum stimulate important changes in plant root morphology, most likely due to the bacterial production of plant growth regulating substances such as auxin and gibberelins (Fibach-Paldi et al., 2012). Plant treatment with Azospirillum increases the number of lateral roots and root hairs length, which maximizes the surface area available for nutrient absorption, resulting in a greater capacity for nutrient uptake and improved water status. These factors significantly contribute to the plant growth promoting effect (Lin et al., 1983; Okon, 1994; Castro-Mercado and García-Pineda, 2019). In Arabidopsis thaliana, inoculation of A. brasilense similarly causes an increase in the number of lateral roots, root hairs, elevation in internal auxin concentrations, and significant changes in the root transcriptome (Spaepen et al., 2014).
It is known that the outer cell surface characteristics of Azospirillum are essential for the plant–bacterium interaction contributing to the ability of the cells to colonize the root surfaces and/or to promote plant growth (Fedonenko et al., 2005). Lipopolysaccharides (LPS) are cell surface components of Gram-negative bacteria that are associated with the outer membrane of the cell envelope. In bacterial pathogens, LPS play a number of important roles in the interactions with their eukaryotic hosts (Erbs and Newman, 2003). These molecules have been associated in the virulence of many Gram-negative pathogens as key molecules in mediating host-microbe associations (Munford, 2008). In plants, LPS from various plant pathogenic bacteria trigger plant innate immune responses by acting as microbe-associated molecular pattern (MAMP) (Erbs and Newman, 2012; Desaki et al., 2018). Recently, in rice a putative protein receptor recognizing LPS from different bacterial sources such as Pseudomonas aeruginosa, Salmonnela enterica, and Escherichia coli has been reported. This protein, named OsCERK1, is a multifunctional receptor-like kinase that contains lysin motifs (LysMs) and is essential for the perception of chitin and peptidoglycan, fungal and bacterial MAMPs, respectively. Moreover, it seems that the different structures from the LPS are sensed by this receptor because the lipooligosaccharide and the lipid A structures stimulated the production of reactive oxygen species (ROS) at similar level than whole LPS (Desaki et al., 2018).
In relation with non-pathogenic PGPR, LPS isolated from these bacteria prime plants to respond more rapidly and/or strongly to subsequent pathogen challenges (Van Loon et al., 1998; Coventry and Dubery, 2001), and increase the Ca2+ influx into the cytoplasm (Dow et al., 2000; Nürnberger and Brunner, 2002; Gerber et al., 2004).
While plant defense responses to the LPS of pathogenic bacteria have been well-characterized (Erbs and Newman, 2003), there is little information regarding the effects of LPS from beneficial bacteria on plant cells. In this way, wheat plants sprayed with A. brasilense LPS increased leaf length, and spike formation. Besides, the aging was accelerated and the dry weight of plants increased, suggesting that LPS affected some aspects of wheat development (Chavez-Herrera et al., 2018), however the molecular mechanism by which LPS affect plant grown remain unknown.
Responses stimulated by PGPR includes the increase in peroxidase (POD) enzyme activity, although the study of this biochemical response has been focused mostly to analyze the potential of rhizobacteria to inhibit pathogens growth (Sharma et al., 2007). POD are oxidoreductases that use H2O2 as an electron acceptor. They take electrons from various donors to reduce H2O2 to water (Penel and Dunand, 2009). Plant POD are grouped in class III (Welinder, 1992), and are encoded by multigene families; for example, Arabidopsis thaliana has 73 genes encoding for peroxidase (Tognolli et al., 2002) and Oryza sativa has 138 (Passardi et al., 2004). In plants, POD are mainly localized in the cell wall, the apoplast and in vacuoles. Some POD are involved in lignification, suberization, and oxidative cross-linking of proteins in the plant cell walls (Quiroga et al., 2000; Deepak et al., 2007; Mnich et al., 2020). Enzymatic activity of POD can be detected in all organs and almost all tissues, with a particularly high level in roots (Francoz et al., 2015).
The calcium ion (Ca2+) is a ubiquitous intracellular second messenger used extensively in all organisms to connect intracellular responses to extracellular stimuli and to coordinate a wide range of endogenous processes (Edel et al., 2017). It is well-known that POD activity is strongly dependent on the presence of calcium ions (Pintus et al., 2011) and they may switch a POD between different modes of action (Mura et al., 2006). Removal of calcium ions results in a considerable decrease in POD activity (Morishima et al., 1996), suggesting calcium ions function to maintain enzyme stability associated with high catalytic activity (Medda et al., 2003).
The balance of plant growth and cell expansion can be controlled by POD. They are able to either build a rigid wall by forming bonds by oxidizing aromatic cell wall compounds in the presence of H2O2, or loosen cell wall by regulating the concentration of H2O2 or generating hydroxyl radical (OH.) which break covalent bonds in cell wall polymers. They can also control cell elongation through their auxin oxidase activity (Francoz et al., 2015).
The aim of this study was to analyze the effect of LPS derived from A. brasilense on in vitro early plant growth of wheat seedlings, and on several biochemical responses related with plant growth, such as peroxidase activity and calcium mobilization.
The strain used in this study was the wild type A. brasilense Sp245 (Baldani et al., 1986). The strain was routinely maintained on solid LB medium (10 g/L tryptone, 5 g/L yeast extract, 5 g/L NaCl, 0.186 g/L MgSO4, 0.277 g/L CaCl2, 15 g/L agar).
Triticum aestivum seeds, cv Nana F2007, were kindly provided by Dr. Mario González-Chavira (Instituto Nacional de Investigaciones Forestales, Agrícolas y Pecuarias—Celaya, Guanajuato, México).
Lipopolysaccharide extraction was performed as previously described (Renukadevi et al., 2012), with some modifications. A culture of 50 mL A. brasilense was grown for 16 h in LB agar (28°C). Cells were washed (3,200 rpm, 5 min) in phosphate buffered saline (10 mM, pH 7.2). Pelleted cells were then resuspended in 10 mL 50 mM Tris-HCl and 2 mM ethylenediaminetetraacetic acid (EDTA) buffer (pH 8.5). The bacterial suspension was mixed with 90% phenol that was preheated to 65°C, and then incubated at 35°C in an orbital shaker (160 rpm) for 15 min, followed by centrifugation at 5,000 rpm for 20 min. The supernatant (water fraction) was used to purify LPS by extensive dialysis against deionized water for 4 days. LPS were concentrated by alcohol precipitation as follow: sodium acetate was added to a final concentration of 0.15 M followed by dropwise addition of ice-cold 96% ethanol to yield a final proportion of 1:4 (v/v). Then, the mixture was incubated for 24 h at −20°C. Pellets containing LPS were collected after centrifugation at 4,000 rpm and then resuspended in distilled water. Final purified LPS were lyophilized and stored at 4°C.
A pre-inoculum was first established taken a colony from a fresh culture and inoculating in 3 mL of liquid LB medium. The culture was incubated for 16 h (~ 1.0 OD600 nm) at 28 °C, with rotation at 140 rpm. After that, 150 μL of the pre-inoculum was transferred in 50 mL of liquid LB medium and incubated for 16 h (exponential phase) at 28°C, with rotation at 140 rpm. Bacterial cultures were centrifuged at 3,800 g for 12 min at room temperature. The pellet was washed with 0.9% NaCl, centrifuged at 4,300 × g, 10 min, 4°C and resuspended in 0.01 M MgSO4, and adjusted using sequential dilutions to yield the desired final concentration of colony-forming units (CFU)/mL for its use.
To germination, seeds were first shaking in 1% sodium dodecyl sulfate (SDS) for 3 min, and then surface sterilized with 1% sodium hypochlorite solution for 5 min. Seeds were washed four times with sterile distilled water, 5 min each time, and germinated on distilled water-wetted sterile filter paper in Petri dishes for 3 days in the dark at 28°C. The seedlings were aseptically transferred to assay tubes (15 cm length and 2.5 cm width) where only the roots were immersed in 5 mL liquid Murashige and Skoog (MS) medium (pH 5.7).
Lipopolysaccharide were added to the liquid MS medium at various concentrations (10, 100, 500, and 1,000 μg/mL). Inoculation with A. brasilense was performed by adding 106 CFU/mL in liquid MS medium. To analyze the role of peroxidase and calcium on plant growth, salicylhydroxamic acid (SHAM), a peroxidase inhibitor, lantanum chloride (LaCl3), a Ca2+ channel inhibitor, ethylene glycol-bis (β-aminoethyl ether)-N,N,N'N'-tetraacetic acid (EGTA), a calcium chelator, and A23187, a calcium ionophore, were used at 50, 1, 100, and 10 μM, respectively. Chemicals were added alone into MS medium or half hour before the addition of 100 μg/mL LPS. All chemicals were obtained from Sigma-Aldrich (St. Louis, MO, USA). Treated seedlings were incubated by 4 days into a growth chamber (Percival Scientific AR-95L) at 22°C with photoperiod of 16 h light, 8 h darkness and light intensity of 100 μmol m−2 s−1. Plant growth parameters were measured as leaf length, root length, number of lateral roots, total fresh weight and dry weight after different treatments.
To A. brasilense, inoculum was adjusted to a final concentration of 106 colony-forming units (CFU)/mL.
Soluble POD assay was performed according to Svalheim and Robertsen (1990). Seedlings were treated with LPS by 24 h and after that POD activity was assayed. Roots (~ 100 mg) from plants were ground in liquid nitrogen, re-suspended in 10 mM sodium phosphate, pH 6.0, and homogenized by vortex. Samples were centrifuged at 12,000 rpm for 5 min, and the suspension was assayed for peroxidase activity following the formation of tetraguaiacol as an increase in absorbance at 470 nm in a spectrophotometer (Beckman Instruments, Fullerton, CA). Each reaction mixture (1 mL) consisted of 10 μL root extract and 990 μL guaiacol solution containing 0.25% guaiacol (v/v) in 10 mM sodium phosphate buffer pH 6.0 and 0.125% H2O2 (v/v). The reaction was monitored for 1 min. Protein content of extracts was determined by Bradford assay 1976. Bovine serum albumin was used as the standard.
Wheat roots growing in MS liquid medium in presence of 100 μg/mL LPS alone or in combination with 50 μM SHAM for 4 days were transferred to solution consisting of 0.1 M Tris-acetate, 0.1 mM 2,6-dichloroindophenol (DCPIP), and 0.9 mM H2O2 at pH 5. After 30 min of incubation, a reddish color was developed, and root images were taken.
Experiments were repeated at least three times and data were expressed as mean ± standard error (SE). Statistical analyses were done with one-way analysis of variance (ANOVA) followed by Duncan's multiple range tests for independent samples. In all cases, the confidence coefficient was set at P < 0.05.
The effect of isolated LPS on the growth of wheat seedlings was analyzed. LPS were used at various concentrations (ranging from 10 to 1,000 μg/mL) to treat by 4 days wheat seedlings. The plant growth promotion of the seedlings was concentration-dependent (Figure 1A). Treatment of seedlings with 10–500 μg/mL LPS increased total fresh weight and total dry weight for 57 and 38%, respectively, whereas root length and leaf length were increased for 60 and 31%, respectively relative to untreated seedlings. Although all these concentrations stimulated plant growth, this biological activity was consistently observed with LPS at 100 μg/mL. Increasing the LPS concentration above 1,000 μg/mL produced no additional increase in plant growth (Table 1).
Figure 1. Effect of A. brasilense LPS on wheat seedlings growth. LPS were added to seedlings growing in liquid MS medium and after 4 days growth parameters were analyzed and compared with the inoculation of A. brasilense cells. (A) Image of wheat seedlings treated with increasing LPS concentrations. Barr = 1 cm. (B) Total fresh weight. (C) Root length. (D) Leaf length. C, Control (untreated seedlings); LPS, Lipopolysaccharides; Ab, Azospirillum brasilense. LPS in panels (B–D) were used at 100 μg/mL. A. brasilense was inoculated at 106 CFU/mL. Data are mean ± SE of three independent experiments (n = 10). Letters above bars indicate significant differences according to a Duncan test (p < 0.05).
The effect of LPS (100 μg/mL) on plant growth was compared with the inoculation of whole cells of A. brasilense (106 CFU/mL). The inoculation of wheat seedlings increased total fresh weight by ~44%, and root length and leaf length by ~26 and 20%, respectively, in relation to the non-inoculated control (Figures 1B–D). Thus, LPS shown similar or even better bioactivity to the rhizobacteria to stimulate plant growth.
Wheat seedlings were treated with two concentrations of LPS (10 and 100 μg/mL), and after 4 d of incubation, the POD activity was assayed on roots. Both LPS concentrations were able to increase POD activity (32 and 45% to 10 and 100 μg/mL), with an overall effect of increase observed to a higher concentration (Figure 2A).
Figure 2. Effect of A. brasilense LPS on POD enzyme activity. Seedlings were treated with LPS and the POD inhibitor SHAM alone or in combination with LPS. Plant growth parameters were analyzed 4 days after treatment. (A) Increasing LPS concentrations were used to assay POD activity in roots 4 days after treatment. (B) Effect of LPS and SHAM on POD activity. (C) Image of in vivo detection of POD activity with DCPIP in roots. (D) Leaf length. (E) Root length. (F) Total fresh weight. LPS, Lipopolysaccharides; SHAM, salicylhydroxamic acid. Data are mean ± SE of three independent experiments (n = 10). Letters above bars indicate significant differences according to a Duncan test (p < 0.05).
Next, we used SHAM, a POD inhibitor, to further assess the effect of the LPS on wheat seedlings. Seedlings were grown in presence of the inhibitor at 50 μM alone, or in combination with LPS at 100 μg/mL for 4 days. SHAM alone decreased POD activity in the roots by 55 % related to the untreated control. The bacterial LPS failed to increase POD activity in the presence of SHAM (Figure 2B).
Root seedlings treated with LPS (100 μg/mL) and staining with DCPIP to locate in vivo POD activity indicated a high increase in the enzyme activity in all root tissues, as well as, a high increase in hair root formation. In control roots, POD activity was mainly detected in the root tips and it was abolished with 50 μM SHAM. The addition of LPS to seedlings treated with SHAM not shown increase in root POD activity (Figure 2C). The plant growth parameters leaf length, root length, and total fresh weight also decreased with SHAM treatment, regardless of whether LPS were added (Figures 2D–F).
The role of calcium on wheat seedlings growth (total fresh weight, root length, and leaf length) promoted by LPS was analyzed using the calcium ionophore A23187 and the calcium channel inhibitor lanthanum chloride. The ionophore A23187 at 10 μM alone stimulated seedling growth at similar levels to those observed with LPS, suggesting that some of the plant growth promoting effects were mediated through Ca2+ signaling (Table 2). When the ionophore was added to seedlings in combination with LPS (100 μg/mL) not additional increase in growth was observed compared to untreated control.
An increase in POD activity (~32%) in presence of the ionophore, similar to treatment with LPS alone, was observed. The addition of A23187 (10 μM) in combination with LPS (100 μg/mL) did not stimulate an additional increase in POD activity.
The Ca2+ channel inhibitor La3+ alone (1 μM) inhibited the growth of seedlings. In addition, La3+ decreased POD activity by 53 % in relation to untreated control. The combination of LaCl3 (1 μM) with LPS (100 μg/mL) further decreased POD activity by 66%. EGTA use alone or plus LPS decreased at similar levels plant growth and POD activity, but not to degree than with LaCl3.
Lipopolysaccharides are molecules consisting of a lipid, a core oligosaccharide, and an O-polysaccharide moiety. The lipid moiety is called lipid A, and is embedded in the outer phospholipid bilayer. Lipid A is linked to a core oligosaccharide via the sugar 3-deoxy-D-manno-2-octulosonate (KDO) (Erbs and Newman, 2003). O-polysaccharide consists of a short series of repeating oligosaccharide units ending in the O-antigen. Variations in LPS structure reside mostly in the O-antigen, commonly used for serotype classification (Luderitz et al., 1971). Characterization of the O-polysaccharide moiety of some Azospirillum species LPS has shown that it consists of a polysaccharide with repeating units of that rarely contain more than five residues. Particularly, the polysaccharide of A. brasilense Sp245 consist of a linear pentasaccharide containing only D-Rhamnose residues (Fedonenko et al., 2002).
Despite the number of studies addressing the molecular mechanisms of interactions between Azospirillum and cereal plants, details about the importance of LPS in growth promotion have yet to be elucidated. The LPS cover almost 80% of the cell surface of Gram-negative bacteria, which likely underlies the critical role of these molecules in bacterial interactions with their eukaryotic hosts (Bedini et al., 2005). To date, most studies on the role of LPS during bacteria-plant interactions have focused on the LPS induction of plant defense responses. LPS from plant pathogenic bacteria function as general elicitors of plant defense responses triggering the production of reactive oxygen species (Meyer et al., 2001; Gerber et al., 2004; Bedini et al., 2005), the expression of pathogenesis-related proteins (Coventry and Dubery, 2001) and the systemic resistance in several plant species (Leeman et al., 1995; Van Wees et al., 1997; Reitz et al., 2000), among others.
In this study, we showed that LPS molecules isolated from A. brasilense directly mediate in vitro early wheat seedlings growth, a novel property of rhizobacteria LPS molecules. Also, our study supports previous observations that A. brasilense cell surface components, including LPS, are essential to stimulate plant growth (Bahat-Samet et al., 2004; Jofré et al., 2004).
Although the role of rhizobacterial LPS as a key component in the growth-promoting interactions between plants and bacteria awaits additional characterization, Evseeva et al. (2011) reported that treatment of wheat roots seedlings with 10 μg A. brasilense Sp245 LPS increased the mitotic index of cells to a degree that was comparable to that produced by inoculation with whole cells, suggesting that LPS-mediated effects on plant growth may be related to their ability to induce cell division.
Analyzing the LPS biochemical action mechanism in plant growth promotion, we found an increase in POD activity. This increase in activity could explain, in part, its effect on promoting plant growth, because SHAM, a POD inhibitor, abolished LPS-induced plant growth. Several plant development processes are regulated by POD enzymes, for example promoting root elongation (Passardi et al., 2006; Cosio et al., 2009), and lateral roots initiation and elongation processes (Mei et al., 2009). It has been reported that PGPR increased plant growth and POD activity in chile and tomato plants (Sharma et al., 2007), thus, both responses are associated during plant growth stimulated by rhizobacteria. Our results suggest that the LPS could be one of the molecules involved in the biological activity from rhizobacteria on plants because they are able to stimulate plant growth and POD activity. The effect of LPS on root morphology was particularly interesting because it caused a high increase in root hair formation concomitant with a high increase in in vivo POD activity; these two effects were inhibited by the addition of SHAM, suggesting that the effect of LPS on root morphology is mediated by POD activity.
The role of calcium was analyzed as a candidate molecule to mediate the cellular responses stimulated by LPS. Inhibiting the cellular availability of calcium with either LaCl3 or EGTA in presence of LPS, the observed effects with LPS on plant growth and POD activity were inhibited, clearly suggesting that calcium availability is required by LPS to stimulate plant growth. The calcium ionophore A23187 alone increased plant growth, thus supporting the hypothesis that intracellular calcium is needed for LPS biological activity. However, when the calcium ionophore A23187 was used in addition to LPS a decrease in plant growth was observed, perhaps due to an overstimulation of the biological system (Figure 3).
Figure 3. Scheme showing the relationship among LPS, calcium mobilization and POD activity, to regulate plant growth. LPS, lipopolysaccharides; A23187, ionophore A23187; SHAM, salicylhydroxamic acid; POD, peroxidase; LaCl3, lanthanum chloride.
Peroxidase activity is dependent on the presence of calcium ions (Mura et al., 2005a; Pintus et al., 2011) altering its activity bia a variety of mechanisms. Some POD enzyme have a calmodulin binding domain (Mura et al., 2005b; Pintus et al., 2011) suggesting that calcium signaling may directly affect POD activity in these enzymes. In general, Ca2+ ions have been linked to stabilizing POD catalytic activity (Longu et al., 2004), and in the case of horseradish peroxidase, the removal of calcium ions results a considerable decrease in POD activity. Cell wall matrix calcium-dependent binding to pectate enhances apoplastic POD activities (Shah et al., 2004). In addition, calcium may increase POD secretion in sugar beet (Penel et al., 1984). Thus, LPS might increase POD activity acting on changes in calcium levels stabilizing or stimulating the release of POD enzymes.
Overall, the results suggest that the plant growth promoted by LPS of A. brasilense could be mediated by POD activity and calcium mobilization.
In addition, the biological effects of bacterial LPS on plant response could be mediated through dedicated cellular receptors. Receptor to LPS seems to be present in plants, and some candidates have been reported in rice and in Arabidopsis (Ranf et al., 2015; Desaki et al., 2018), but the associated signal transduction cascades remain to be better characterized.
The elucidation of the molecular mechanisms implicated in LPS induced plant growth will reveal the relative contributions of these molecules to the plant growth promotion ability of rhizobacteria.
The raw data supporting the conclusions of this article will be made available by the authors, without undue reservation.
AAH-E conducted the experiments. EC-M contributed with technical assistance to experimental setup. EV-C and GA discussed the results and revised the manuscript redaction. EG-P was the author of project planning and wrote the manuscript. All authors contributed to the article and approved the submitted version.
This study was supported by funds from the Coordinación de la Investigación Científica, Universidad Michoacana de San Nicolás de Hidalgo, and with a Grant to AAH-E (No. 606506) from CONACYT, México.
The authors declare that the research was conducted in the absence of any commercial or financial relationships that could be construed as a potential conflict of interest.
Bahat-Samet, E., Castro-Sowinski, S., and Okon, Y. (2004). Arabinose content of extracellular polysaccharide plays a role in cell aggregation of Azospirillum brasilense. FEMS Microbiol. Lett. 237, 195–203. doi: 10.1111/j.1574-6968.2004.tb09696.x
Baldani, V. L. D., Alvarez, M. A., Baldani, J. I, and Dobereiner, J. D. (1986). Establishment of inoculated Azospirillum spp in the rhizosphere and in roots of field grown wheat and sorghum. Plant Soil. 90, 35–46. doi: 10.1007/BF02277385
Bedini, E., De Castro, C., Erbs, G., Mangoni, L., Dow, J. M., Newman, M. A., Parrilli, M., and Unverzag, C. (2005). Structure-dependent modulation of a pathogen response in plants by synthetic O-antigen polysaccharides. J. Am. Chem. Soc. 127, 2414–2416. doi: 10.1021/ja0452166
Bradford, M. (1976). A rapid and sensitive method for the quantification of microgram quantities of protein utilizing the principle of protein-dye binding. Ann. Biochem. 72, 48–254. doi: 10.1016/0003-2697(76)90527-3
Castro-Mercado, E., and García-Pineda, E. (2019). Phospholipase C activity is increased in wheat seedlings inoculated with the rhizobacteria Azospirillum brasilense Sp245. Acta Physiol. Plant. 41:152. doi: 10.1007/s11738-019-2949-z
Chavez-Herrera, E., Hernandez-Esquivel, A. A., Castro-Mercado, E., and Garcia-Pineda, E. (2018). Effect of Azospirillum brasilense Sp245 lipopolysaccharides on wheat plant development. J. Plant Growth Reg. 37, 859–866. doi: 10.1007/s00344-018-9782-2
Cosio, C., Vuillemin, L., De Meyer, M., Kevers, C., Penel, C., and Dunand, C. (2009). An anionic class III peroxidase from zucchini may regulate hypocotyl elongation through its auxin oxidase activity. Planta 229, 823–836. doi: 10.1007/s00425-008-0876-0
Coventry, H. S., and Dubery, I. A. (2001). Lipopolysaccharides from burkholderia cepacia contribute to an enhanced defense capacity and the induction of pathogenesis-related proteins in nicotiana tabacum. Physiol. Mol. Plant Pathol. 58, 149–158. doi: 10.1006/pmpp.2001.0323
Deepak, S., Shailasree, S., Sujeeth, N., Kini, R. K., Shetty, S. H., and Mithofer, A. (2007). Purification and characterization of proline/hydroxyproline-rich glycoprotein from pearl millet coleoptiles infected with downy mildew pathogen Sclerospora graminicola. Phytochemistry 68, 298–305. doi: 10.1016/j.phytochem.2006.10.024
Desaki, Y., Kouzai, Y., Ninomiya, Y., Iwase, R., Shimizu, Y., Seko, K., et al. (2018). OsCERK1 plays a crucial role in the lipopolysaccharide-induced immune response of rice. New Phytol. 217, 1042–1049. doi: 10.1111/nph.14941
Dow, M., Newman, M. A., and von Roepenack, E. (2000). The induction and modulation of plant defence responses by bacterial lipopolysaccharides. Annu. Rev. Phytopathol. 38, 241–261. doi: 10.1146/annurev.phyto.38.1.241
Edel, K. H., Marchadier, E., Brownlee, C., Kudla, J., and Hetherington, A. M. (2017). The evolution of calcium-based signalling in plants. Curr. Biol. 27, R667–R679. doi: 10.1016/j.cub.2017.05.020
Erbs, G., and Newman, M. A. (2003). The role of lipopolysaccharide in induction of plant defence responses. Mol. Plant Pathol. 4, 421–425. doi: 10.1046/j.1364-3703.2003.00179.x
Erbs, G., and Newman, M. A. (2012). The role of lipopolysaccharide and peptidoglycan, two glycosylated bacterial microbe-associated molecular patterns (MAMPs), in plant innate immunity. Mol. Plant Pathol. 13, 95–104. doi: 10.1111/j.1364-3703.2011.00730.x
Evseeva, N. V., Matora, L. Y., Burygin, G. L., Dmitrienko, V. V., and Shchyogolev, S. Y. (2011). Effect of Azospirillum brasilense Sp245 lipopolysaccharides on the functional activity of wheat root meristematic cells. Plant Soil. 346, 181–188. doi: 10.1007/s11104-011-0808-9
Fedonenko, P., Borisov, I. V., Konnova, O. N., Zdorovenko, E. L., Katsy, E. I., Konnova, S. A., et al. (2005). Determination of the structure of the repeated unit of the azospirillum brasilense SR75 O-specific polysaccharide and homology of the lps loci in the plasmids of azospirillum brasilense strains SR75 and Sp245. Microbiol 74, 542–548. doi: 10.1007/s11021-005-0101-0
Fedonenko, Y. P., Zatonsky, G. V., Konnova, S. A., Zdorovenko, E. L., and Ignatov, V. V. (2002). Structure of the O-specific polysaccharide of the lipopolysaccharide of azospirillum brasilense Sp245. Carbohydr. Res. 337, 9869–872. doi: 10.1016/S0008-6215(02)00061-7
Fibach-Paldi, S., Burdman, S., and Okon, Y. (2012). Key physiological properties contributing to rhizosphere adaptation and plant growth promotion abilities of Azospirillum brasilense. FEMS Microbiol. Lett. 326, 99–108. doi: 10.1111/j.1574-6968.2011.02407.x
Francoz, E., Ranocha, P., Nguyen-Kim, H., Jamet, E., Burlat, V., and Dunand, C. (2015). Roles of cell wall peroxidases in plant development. Phytochemistry 112, 15–21. doi: 10.1016/j.phytochem.2014.07.020
Gerber, I. B., Zeidler, D., Durner, J., and Dubery, I. A. (2004). Early perception responses of Nicotiana tabacum cells in response to lipopolysaccharides from Burkholderia cepacia. Planta 218, 647–657. doi: 10.1007/s00425-003-1142-0
Jofré, E., Lagares, A., and Mori, G. (2004). Disruption of dTDP rhamnose biosynthesis modifies lipopolysaccharide core, exopolisaccharide production, and root colonization in Azospirillum brasilense. FEMS Microbiol. Lett. 231, 267–275. doi: 10.1016/S0378-1097(04)00003-5
Leeman, M., Van Pelt, J., Hendrickx, M. J., Scheffer, R. J., Bakker, P., and Schippers, B. (1995). Biocontrol of Fusarium wilt of radish in commercial greenhouse trials by seed treatment with Pseudomonas fluorescens WCS374. J. Phytopathol. 85, 1301–1305. doi: 10.1094/Phyto-85-1301
Lin, W., Okon, Y., and Hardy, R. W. F. (1983). Enhanced mineral uptake by Zea mays and Sorghum bicolor roots inoculated with Azospirillum brasilense. Appl. Environ. Microbiol. 45, 1775–1779. doi: 10.1128/AEM.45.6.1775-1779.1983
Longu, S., Medda, R., Padiglia, A., Pedersen, J. Z., and Floris, G. (2004). The reaction mechanism of plant peroxidases. Ital. J. Biochem. 53, 42–46.
Luderitz, O., Westphal, O., Staub, A. M., and Nikaido, H. (1971). “Isolation and chemical and immunological characterization of bacterial lipopolysaccharides,” in Microbiology Toxins, Vol. IV Bacterial Endotoxins, eds. G. Weinbaum, S. Kadis, and S. Ajl (New York: Academic Press), 145–233. doi: 10.1016/B978-0-12-046504-0.50010-3
Medda, R., Padiglia, A., Longu, S., Bellelli, A., Arcovito, A., Cavallo, S., Pedersen, J. Z., and Floris, G. (2003). Critical role of Ca2+ ions in the reaction mechanism of Euphorbia characias peroxidase. Biochemistry 42, i8909–8918. doi: 10.1021/bi034609z
Mei, W., Qin, Y., Song, W., Li, J., and Zhu, Y. (2009). Cotton GhPOX1 encoding plant class III peroxidase may be responsible for the high level of reactive oxygen species production that is related to cotton fiber elongation. J. Gen. Genom. 36, 141–150. doi: 10.1016/S1673-8527(08)60101-0
Meyer, A., Puhler, A., and Niehaus, K. (2001). The lipopolysaccharides of the phytopathogen Xanthomonas campestris pv. campestris induce an oxidative burst reaction in cell cultures of Nicotiana tabacum. Planta 213, 214–222. doi: 10.1007/s004250000493
Mnich, E., Bjarnholt, N., Eudes, A., Harholt, J., Holland, C., Jørgensen, B., et al. (2020). Phenolic cross-links: building and de-constructing the plant cell wall. Nat. Prod. Rep. 37, 919–961. doi: 10.1039/C9NP00028C
Morishima, I., Kurono, M., and Shiro, Y. (1996). Presence of endogenous calcium ion in horseradish peroxidase. J. Biol. Chem. 261, 9391–9399.
Munford, R. S. (2008). Sensing gram-negative bacterial lipopolysaccharides: a human disease determinant? Infect. Immun. 76, 454–465. doi: 10.1128/IAI.00939-07
Mura, A., Longu, S., Padiglia, A., Rinaldi, A. C., Floris, G., and Medda, R. (2005a). Reversible thermal inactivation and conformational states in denaturant guanidinium of a calcium-dependent peroxidase from Euphorbia characias. Int. J. Biol. Macromol. 37, 205–11. doi: 10.1016/j.ijbiomac.2005.10.010
Mura, A., Medda, R., Longu, S., Floris, G., Rinaldi, A. C., and Padiglia, A. A. (2005b). A Ca2+/calmodulin-binding peroxidase from Euphorbia latex: novel aspects of calcium-hydrogen peroxide cross talk in the regulation of plant defenses. Biochemistry. 44, 14120–14130. doi: 10.1021/bi0513251
Mura, A., Pintus, F., Lai, P., Padiglia, A., Bellelli, A., Floris, G., and Medda, R. (2006). Catalytic pathways of Euphorbia characias peroxidase reacting with hydrogen peroxide. Biol. Chem. 387, 559–67. doi: 10.1515/BC.2006.072
Nürnberger, T., and Brunner, F. (2002). Innate immunity in plants and animals: emerging parallels between the recognition of general elicitors and pathogen-associated molecular patterns. Curr. Opin. Plant Biol. 5, 318–324. doi: 10.1016/S1369-5266(02)00265-0
Okon, Y., and Vanderleyden, J. (1997). Root-associated Azospirillum species can stimulate plants. ASM News. 63, 366–370.
Passardi, F., Longet, D., Penel, C., and Dunand, C. (2004). The class III peroxidase multigenic family in rice and its evolution in land plants. Phytochemistry 65, 1879–1893. doi: 10.1016/j.phytochem.2004.06.023
Passardi, F., Tognolli, M., De Meyer, M., Penel, C., and Dunand, C. (2006). Two cell wall associated peroxidases from Arabidopsis influence root elongation. Planta 223, 965–974. doi: 10.1007/s00425-005-0153-4
Penel, C., and Dunand, C. (2009). “Signaling via plant peroxidases,” in Signaling in Plants, Signaling and Communication in Plants, eds. F. Baluška and S. Mancuso (Berlin; Heidelberg: Springer-Verlag), 155–172. doi: 10.1007/978-3-540-89228-1_8
Penel, C., Sticher, L., Kevers, C., Gaspar, T., and Greppin, H. (1984). Calcium-controlled peroxidase secretion by sugar beet cells: effect of ionophores in relation to organogenesis. Biochem. Physiol. Pflanzen. 179, 173–180. doi: 10.1016/S0015-3796(84)80075-X
Pintus, F., Spanò, D., Medda, R., and Floris, G. (2011). Calcium ions and a secreted peroxidase in Euphorbia characias latex are made for each other. Protein J. 30, 115–23. doi: 10.1007/s10930-011-9310-8
Quiroga, M., Guerrero, C., Botella, M. A., Barcelo, A., Amaya, I., Medina, M. I., et al. (2000). A tomato peroxidase involved in the synthesis of lignin and suberin. Plant Physiol. 122, 1119–1127. doi: 10.1104/pp.122.4.1119
Ranf, S., Gisch, N., Schaffer, M., Illig, T., Westphal, L., Knirel, Y. A., et al. (2015). A lectin S-domain receptor kinase mediates lipopolysaccharide sensing in Arabidopsis thaliana. Nat. Immunol. 16, 426–433. doi: 10.1038/ni.3124
Reitz, M., Rudolph, K., Schroder, I., Hoffmann-Hergarten, S., Hallmann, J., and Sikora, R. A. (2000). Lipopolysaccharides of Rhizobium etli strain G12 act in potato roots as an inducing agent of systemic resistance to infection by the cyst nematode Globodera pallida. Appl. Environ. Microbiol. 66, 3515–3518. doi: 10.1128/AEM.66.8.3515-3518.2000
Renukadevi, K. P., Angayarkanni, J., and Karunakaran, G. (2012). Extraction and characterization of lipopolysaccharide from Serratia rubidaea and its cytotoxicity on lung cancer cell line-nci-h69. Acta Tech. Corviniensis. 2, 97–101.
Shah, K., Penel, C., Gagnon, J., and Dunand, C. (2004). Purification and identification of a Ca(2+)-pectate binding peroxidase from Arabidopsis leaves. Phytochemistry 65, 307–312. doi: 10.1016/j.phytochem.2003.11.019
Sharma, A., Pathak, A., Sahgal, M., Meyer, J. M., Wray, V., and Johri, B. N. (2007). Molecular characterization of plant growth promoting rhizobacteria that enhance peroxidase and phenylalanine ammonia-lyase activities in chile (Capsicum annuum L.) and tomato (Lycopersicon esculentum Mill.). Arch. Microbiol. 188, 483–494. doi: 10.1007/s00203-007-0270-5
Singh, J. S., Pandey, V. C., and Singh, D. P. (2011). Efficient soil microorganisms: a new dimension for sustainable agriculture and environmental development. Agric. Ecosyst. Environ. 140, 339–353. doi: 10.1016/j.agee.2011.01.017
Spaepen, S., Bossuyt, S., Engelen, K., Marchal, K., and Vanderleyden, J. (2014). Phenotypical and molecular responses of Arabidopsis thaliana roots as a result of inoculation with the auxin-producing bacterium Azospirillum brasilense. New Phytol. 201, 850–861. doi: 10.1111/nph.12590
Svalheim, O., and Robertsen, B. (1990). Induction of peroxidase in cucumber hypocotyls by wounding and fungal infection. Physiol. Plant. 78, 261–267. doi: 10.1111/j.1399-3054.1990.tb02090.x
Tognolli, M., Penel, C., Greppin, H., and Simon, P. (2002). Analysis and expression of the class III peroxidase large gene family in Arabidopsis thaliana. Gene. 288, 129–138. doi: 10.1016/S0378-1119(02)00465-1
Van Loon, L. C., Bakker, P. A. H. M., and Pieterse, C. M. J. (1998). Systemic resistance induced by rhizosphere bacteria. Annu. Rev. Phytopathol. 36, 453–483. doi: 10.1146/annurev.phyto.36.1.453
Van Wees, S. C. M., Pieterse, C. M. J., Trijssenaar, A., Van‘t Westende, Y. A. M., Hartog, F., and Van Loon, L. C. (1997). Differential induction of systemic resistance in Arabidopsis by biocontrol bacteria. Mol. Plant-Microbe Interact. 10, 716–724. doi: 10.1094/MPMI.1997.10.6.716
Welinder, K. G. (1992). Superfamily of plant, fungal and bacterial peroxidases. Curr. Opin. Struct. Biol. 2, 388–393. doi: 10.1016/0959-440X(92)90230-5
Keywords: Azospirillum, lipopolysaccharides, peroxidase, growth, wheat
Citation: Hernaández-Esquivel AA, Castro-Mercado E, Valencia-Cantero E, Alexandre G and García-Pineda E (2020) Application of Azospirillum brasilense Lipopolysaccharides to Promote Early Wheat Plant Growth and Analysis of Related Biochemical Responses. Front. Sustain. Food Syst. 4:579976. doi: 10.3389/fsufs.2020.579976
Received: 03 July 2020; Accepted: 24 August 2020;
Published: 25 September 2020.
Edited by:
Everlon Cid Rigobelo, São Paulo State University, BrazilReviewed by:
Gennady L. Burygin, Institute of Biochemistry and Physiology of Plants and Microorganisms (RAS), RussiaCopyright © 2020 Hernaández-Esquivel, Castro-Mercado, Valencia-Cantero, Alexandre and García-Pineda. This is an open-access article distributed under the terms of the Creative Commons Attribution License (CC BY). The use, distribution or reproduction in other forums is permitted, provided the original author(s) and the copyright owner(s) are credited and that the original publication in this journal is cited, in accordance with accepted academic practice. No use, distribution or reproduction is permitted which does not comply with these terms.
*Correspondence: Ernesto García-Pineda, ZWdwaW5lZGFAdW1pY2gubXg=
Disclaimer: All claims expressed in this article are solely those of the authors and do not necessarily represent those of their affiliated organizations, or those of the publisher, the editors and the reviewers. Any product that may be evaluated in this article or claim that may be made by its manufacturer is not guaranteed or endorsed by the publisher.
Research integrity at Frontiers
Learn more about the work of our research integrity team to safeguard the quality of each article we publish.