- Department of Agricultural Sciences, University of Helsinki, Helsinki, Finland
Water deficit limits cassava (Manihot esculenta Crantz) productivity in drought-prone areas and alters the nutritive quality of the crop. Potassium (K) may mitigate the effects of water deficit and improve the nutritional content of cassava, which would alleviate malnutrition among the human population in the tropics who depend on cassava as a staple food. Pot experiments were conducted under controlled glasshouse conditions to investigate the influence of deficit irrigation and K fertigation on the nutritive and anti-nutritive quality of biofortified cassava during the early growth phase. Treatments initiated at 30 days after planting were three irrigation doses (30, 60, 100% pot capacity) that were split to five K (0.01, 1, 4, 16, and 32 mM) concentrations. Plants were harvested at 90 days after planting, and the starch, energy, carotenoid, crude protein, fiber, minerals, and cyanide concentration of the leaves and roots were determined. Irrigation and K treatments showed significant (P < 0.05) interactions for starch, carotenoid, energy, and cyanide concentration. An irrigation dose of 30% together with 0.01 mM K resulted in the lowest starch, carotenoid, energy, and fiber content, but highest cyanide concentration, relative to full-irrigated (100%) plants together with 16 mM K. When the K application rate was 16 mM the best nutritional quality was obtained, with the lowest cyanide concentration, regardless of irrigation dose. Moreover, nutritional traits showed strong positive associations, whereas cyanide concentration correlated negatively with all the nutritional traits. Notably, an irrigation dose of 60% together with 16 mM K reduced the nutritional content the least and showed minimal increase in cyanide concentration. The results indicate that K fertigation with adjusted irrigation may improve the dietary quality of young cassava and reduce antinutrients, which could enhance the nutrient bioavailability of cassava grown in drought-prone areas.
Introduction
The main challenge of crop production in the tropics is water deficit, which not only limits yield but also alters the nutritive quality of crops (Cakmak, 2010). Consequently, this affects the nutritional status of the human population in the tropics, where malnutrition remains a major health challenge (FAO, 2019). Productivity of tropical soils is also declining, mainly due to intensive use and depletion of nutrients through harvested crop components without adequate nutrient additions to the soil (Gruhn et al., 2000). In tropical soils, depletion of potassium (K) is more rapid compared with other plant nutrients (Cakmak, 2010), even though the role of K in mitigating the effects of water deficit is known (Oosterhuis et al., 2014). Therefore, irrigation strategies not based on full crop water requirements, such as deficit irrigation, would be more effective in the arid tropics when combined with K nutrition to ensure a sustainable quantity and quality production for staple crops such as cassava (Manihot esculenta Crantz).
Cassava is mainly grown in the tropical and sub-tropical regions, where its leaves and roots constitute a major portion of the diet of humans and animals (Lancaster and Brooks, 1983; Ravindra, 1993). The total human calory intake from cassava exceeds 200 billion kcal day−1, making cassava the fourth most important source of dietary energy in the world (El-Sharkawy, 2003). Leaves are rich in minerals, proteins, vitamins, and fiber, comparing favorably with most green vegetables. The roots are valued for their high starch content (Montagnac et al., 2009). In addition, the leaves and roots of biofortified (yellow-rooted) cassava cultivars are an excellent source of provitamin A carotenoid, which humans absorb and convert to retinol, vitamin A (Talsma et al., 2016). Despite the nutritional value of cassava, the human population in the arid tropics still experience mineral and vitamin A deficiencies, even in areas where cassava is grown (Gegios et al., 2010; Talsma et al., 2013). Previous studies on carotenoids in cassava have focused on roots as opposed to leaves, yet leaves have higher carotenoid content (Chávez et al., 2005; Oliveira et al., 2010). Low bioavailability of minerals and proteins from consumption of cassava leaves and roots also remains a challenge in the tropics (Gegios et al., 2010); yet, the nutritional value of cassava is comparable to most cereals, legumes and vegetables. Furthermore, various anti-nutrients, such as tannins, polyphenols, phytic acid, and cyanogenic glucosides, in cassava leaves and roots restrict its use as food and feed in the tropics (Oresegun et al., 2016). Cyanogenic glucosides are considered the principal anti-nutrient factor in cassava due to their toxic effects when they break down to release toxic hydrogen cyanide (HCN) (Rosling, 1994). Chronic cyanide intake from cassava can cause hyperthyroidism, tropical ataxic neuropathy (Osuntokun, 1994), and may cause paralytic disease konzo—characterized by irreversible paralysis of the legs particularly in children and young women (Howlett, 1994). Acute cyanide intoxication can lead to mental confusion, muscular paralysis, respiratory distress, and death (Rosling, 1994). Rural households use processing techniques, such as drying, pounding, boiling, and fermentation, which can reduce cyanide concentration by half (Essers, 1995), but are inefficient in eliminating all the cyanide, which can remain at lethal levels (Ngudi et al., 2003). Additionally, these processing methods degrade total carotenoid content (Oliveira et al., 2010), reduce starch content (Cuvaca et al., 2015), and result in loss of minerals (Achidi et al., 2008; Latif and Müller, 2015) leading to dietary deficiencies.
Previously, it has been shown that water deficit reduces starch content in cassava (Santisopasri et al., 2000) as well as mineral content (Helal et al., 2013), but increases the cyanide concentration (El-Sharkawy, 1993). K fertilization has been found to increase cassava starch content (Howeler, 1998), increase the uptake of other minerals (Howeler, 2014), and reduce cyanide concentration (El-Sharkawy and Cadavid, 2000). However, previous studies have not simultaneously assessed the effect of water deficit and K nutrition on the nutritive quality of cassava, and most studies have concentrated on cassava roots rather than leaves. In addition, there has been little focus on changes in total carotenoid content and crude protein content in cassava in relation to water deficit and K nutrition. Agronomic management practices that improve the nutritive quality of cassava and maximize its yield using less irrigation water with balanced K nutrition are desirable and would alleviate malnutrition, vitamin A deficiency, and cyanide toxicity experienced in the tropical areas where cassava is grown. The objective of this investigation is to assess the influence of the interaction between deficit irrigation and K fertigation on the nutritive and anti-nutritive quality of biofortified cassava during the early growth phase.
Materials and Methods
Four pot experiments were conducted with single-stem cuttings (25 cm) of yellow cassava “Mutura” cultivar [Kenya Agricultural and Livestock Research Organization (KALRO), Nairobi, Kenya] under controlled conditions in a greenhouse at the University of Helsinki, Finland, as described previously by Wasonga et al. (2020). In short, cuttings were planted in 5-L pots containing 1.7 kg of pre-fertilized potting mix and the plants were watered every second day on the soil surface until drainage for 30 days. Irrigation (30, 60, 100% pot capacity) and K fertigation [0.01, 1, 4, 16, 32 mM; potassium chloride (KCl), Sigma-Aldrich Chemie GmbH, Munich, Germany] treatments were initiated at 30 days after planting (DAP) and lasted 60 days. Plants were watered every second day with full-strength Hoagland solution (Hoagland and Arnon, 1950) in which the K concentration was modified. The experiments were arranged in a completely randomized design with four (EXP. I, in total 36 pots) to eight (EXP. II, III, IV, in total 72 pots each) replicates. During the experiment, plants were grown at day/night temperatures of 28/20°C, relative humidity of 55 ± 5%, photoperiod of 12-h light/dark with a photosynthetic photon flux density of 600 μmol m−2 s−1 of photosynthetically active radiation (PAR) at the top of the canopy. Detailed descriptions of the treatments, experimental design, growing media, and plant management are provided in Wasonga et al. (2020).
Measurement and Analyses
The plants were harvested at 90 DAP by cutting the stems at the soil surface. Leaves were detached from the plants, and the roots were carefully washed with water to remove soil. The fresh weight (FW) of leaves (leaf blade and petioles) and roots (fine and thickened-starchy roots) was recorded. Leaves were split into green and senescent. The green leaves and roots were divided into two subsamples. One subsample of each was snap-frozen in liquid N2 and stored at −20°C until further analysis. The other subsample was dried in a forced-air chamber at 70°C for 72 h, weighed, and ground into fine powder using a centrifugal mill (0.5 mm sieve; ZM200, Retsch, Haan, Germany) and stored at room temperature for further analysis.
The mineral content of the leaves and roots was determined for calcium (Ca), magnesium (Mg), phosphorus (P), sulfur (S), sodium (Na), copper (Cu), manganese (Mn), iron (Fe), and zinc (Zn). A ground sample of 250 mg was digested in 6 mL of 15.2 M nitric acid (68% w/v; VWR International BVBA, Leuven, Belgium) and 1 mL of 9.8 M hydrogen peroxide (30% w/v; Merck KGaA, Darmstadt, Germany) with a microwave (MARS 240/50; MARSXpress, CEM). The digested samples were filtered, and mineral analysis was conducted with an Inductively Coupled Plasma-Optical Emission Spectrometer (iCAP 6200, Thermo Fisher Scientific, Cambridge, UK) with every 20th sample a standard as described previously by Seleiman et al. (2012). Chloride (Cl) content was analyzed by mixing 500 mg of ground leaf sample with 10 mL of water and shaking for 3 h in a water bath at 60°C, then measuring the extract with a chloridometer (Sherwood M926 chloride analyser, Sherwood Scientific Ltd., Cambridge, UK) according to Mäkelä et al. (2003). Total carbon (C) and nitrogen (N) content was analyzed from 250 mg of ground leaf and root samples by the Dumas combustion method (VarioMAX CN, Elementar Analysensysteme GmbH, Hanau, Germany). Crude protein content was obtained by multiplying the percentage of N by the conversion factor 6.25 (Horwitz, 1980; Ndung'u et al., 2012).
Starch content was analyzed using the K-TSTA kit (Megazyme, Bray, Ireland) according to the manufacturer's protocol based on AOAC method 996.11 and AACC method 76-13.01. In short, ground leaf and root samples (100 mg) were incubated with ethanol (80% v/v) for 10 min, centrifuged, and the pellets formed were suspended in 2 M potassium hydroxide for 20 min. Approximately 8 mL of 1.2 M sodium acetate buffer was added to each tube followed by addition of 0.1 mL thermostable α-amylase, and 0.1 mL amyloglucosidase, and incubated for 30 min. Duplicate aliquots of the solution were taken and 3.0 mL GOPOD reagent was added. The absorbance was measured at 510 nm using a UV-spectrophotometer (Model UV-1800 240V IVDD, Shimadzu Inc., Kyoto, Japan). Total carotenoid content was determined from 10 g frozen leaf and root samples according to Oliveira et al. (2010). The samples were extracted with acetone (80% v/v), followed by partitioning in petroleum ether (HPLC grade, J.T. Baker, Gliwice, Poland), and the absorbance was measured at 450 nm using a UV spectrophotometer (Model UV-1800 240V IVDD, Shimadzu Inc., Kyoto, Japan).
The biomass energy content (MJ kg−1) was analyzed from ground leaves and roots by compressing 500 mg samples into pellets using a pellet press (Parr Instrument Co., Moline, IL, USA) followed by complete combustion with excess O2 at 3.04 MPa in a sealed steel adiabatic bomb calorimeter (Parr 1241EA, Parr Instrument Co., Moline, IL, USA). Benzoic acid pellets (1.0 g, Parr Instrument Co., Moline, IL, USA) were used as a standard. Total dietary fiber was analyzed from ground leaf and root samples using a K-TDFR kit (Megazyme, Bray, Ireland) according to the manufacturer's protocol based on AOAC Method 991.43 and AACC Method 32-07.01. Approximately 1.0 g samples were incubated with heat-stable α-amylase followed by incubation with protease and a further incubation with amyloglucosidase. Thereafter, pre-heated ethanol (95% v/v) was added to the mixture and the precipitate was filtered using a cold extraction unit (Fibertec 1021, Foss, Hillerød, Denmark), and the residue was dried overnight in an oven at 105°C. Total dietary fiber was calculated as: residue weight—protein weight—ash weight.
Total cyanide (HCN) concentration was analyzed on a wet mass basis from leaves and roots following the alkaline titration method according to Williams (1990). Approximately 20 g of frozen leaf and root samples were thawed and ground using a micro-grinder hand mill (A10 basic, IKA GmbH, Staufen, Germany). Ground samples were incubated with 250 mL of distilled water at 37°C for 16 h. The solution was steam-distilled until 200 mL of distillate was collected in a round-bottomed flask containing 20 mL of 0.625 M sodium hydroxide, which was diluted to 250 mL. Duplicate aliquots of 100 mL were taken, and 8 mL of 6 M ammonium hydroxide and 2 mL of 5% potassium iodide were added. Samples were titrated with 0.02 M silver nitrate until a yellowish turbid color appeared. Total cyanide concentration was then calculated as: 1 ml of 0.02 M silver nitrate = 1.08 mg HCN.
Data Analysis
Data on the nutritive content of the four experiments were combined and analyzed as one experiment, after subjecting to contrast analysis for experimental differences. A two-way analysis of variance (ANOVA) was performed using the R program (version 3.5.2; R Development Core Team, Vienna, Austria) to show the effects of irrigation dose, K rate, and their interactions as fixed effects on the nutritive contents measured. Differences were considered significant when P-values were < 0.05, and means were compared using Tukey's multiple range test. A two-tailed Pearson correlation was performed to identify the association patterns of nutritive contents. In addition, principal component analysis (PCA) was conducted on the combined leaves and roots data to identify the nutritive contents accounting for most of the variation. Only those components with Eigenvalues >1.0 were selected.
Results
Nutritional Traits
Starch content in leaves and roots was affected by irrigation, K treatments, and their interactions (Table 1). The highest starch content in leaves (46.1 mg g−1 DM) and roots (435 mg g−1 DM) was obtained with full-irrigated (100%) plants together with 16 mM K. In comparison, an irrigation dose of 30% together with 0.01 mM K reduced starch content in leaves by 79% and in roots by 82%; however, the reduction in starch content was only 37% in both leaves and roots when the K application rate was 32 mM. The smallest reduction in starch content in leaves, 20%, and roots, 18%, was observed with an irrigation dose of 60% together with 16 mM or 32 mM K. Moreover, the starch content in roots was 9 times higher than in leaves regardless of the irrigation and K treatments.
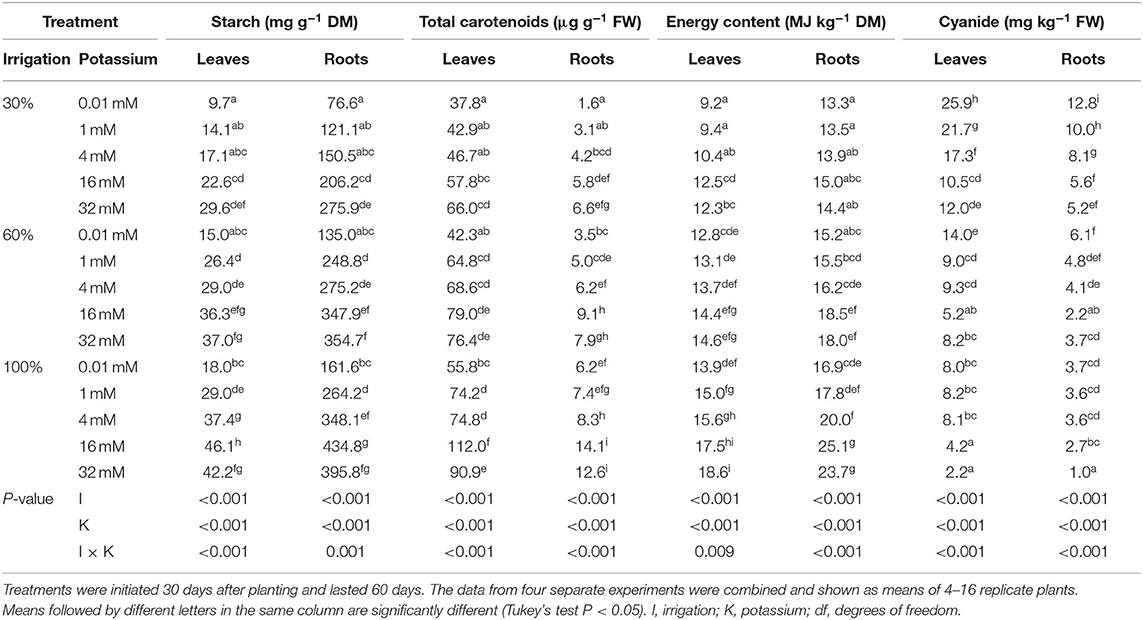
Table 1. Starch, total carotenoids, energy, and cyanide concentration in leaves and roots of 90-day-old cassava plants in response to deficit irrigation and potassium fertigation.
Irrigation and K treatments affected (P < 0.05) crude protein content in leaves and roots of cassava (Figure 1) but showed no treatment interaction (I × K) in leaves (P = 0.901) and roots (P = 0.121). Compared with full-irrigated plants, an irrigation dose of 30% reduced crude protein content in leaves by 24%, whereas an irrigation dose of 60% reduced the crude protein content in leaves by 10%. There was no difference in crude protein content in roots between irrigation dose 30% and irrigation dose 60%, when compared to full-irrigated plants. Notably, 16 mM K fertigation resulted in the highest crude protein content in leaves [198 g kg−1 dry matter (DM)] and roots (87 g kg−1 DM) compared with fertigation with 32 mM K or low-K treatments. In general, crude protein was 56% higher in leaves than in roots.
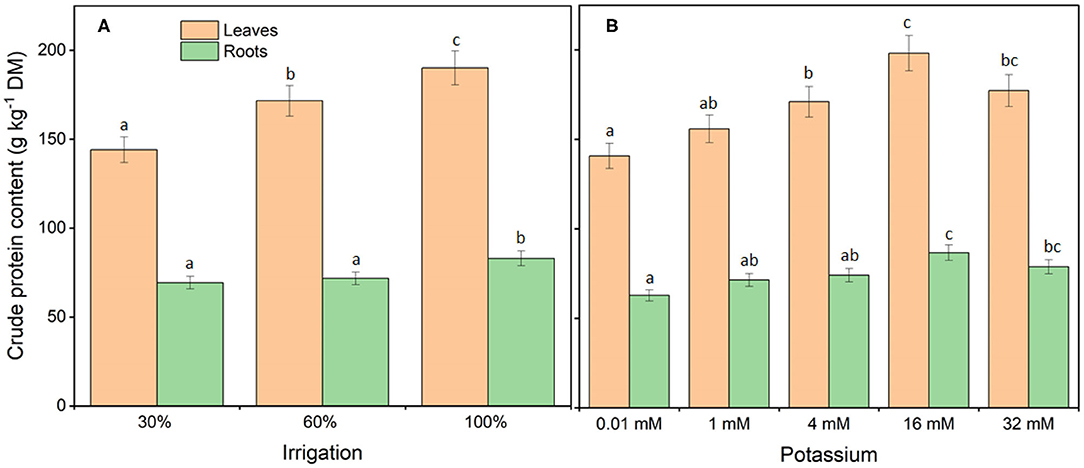
Figure 1. Crude protein content of young cassava plants in response to deficit irrigation (A) and potassium fertigation (B). Crude protein content in leaves and roots were both affected by irrigation (P < 0.001) and K (P < 0.001) but no treatment interactions (I × K) were observed in leaves (P = 0.901) and roots (P = 0.121). Treatments were initiated 30 days after planting and lasted 60 days. The data from four separate experiments were combined and shown as means ± standard errors; n = 4–16 replicate plants. Means followed by different letters in the same graph are significantly different (Tukey's test P < 0.05).
The energy content of leaves and roots was affected by the irrigation and K treatments and their interactions (P < 0.05) (Table 1). The highest energy content in leaves (18.6 MJ kg−1 DM) was obtained with full-irrigated plants together with 32 mM K. In contrast, an irrigation dose of 30% together with 0.01 mM K reduced energy content in leaves by 50%, but the reduction was 33% when the K application rate was 16 mM. It was noted that an irrigation dose of 60% together with 16 mM K showed the least (23%) reduction in energy content in leaves when compared with full-irrigated plants together with 32 mM K. The highest energy content in roots (25.1 MJ kg−1 DM) was obtained with full-irrigated plants together with 16 mM K. In comparison, the energy content in roots was reduced by 47% when the irrigation dose was 30% together with 0.01 mM K, but the reduction was 40% when the K application rate was 16 mM. Likewise, an irrigation dose of 60% together with 16 mM showed the least (26%) reduction in energy content in roots compared with full-irrigated plants together with 16 mM K. In all, the energy content in roots was 21% higher than in leaves.
Irrigation and K treatments and their interaction affected carotenoid content in leaves and roots (Table 1). The highest carotenoid content in leaves (112.0 μg g−1 FW) and roots (14.1 μg g−1 FW) were obtained in full-irrigated plants together with 16 mM K. In contrast, an irrigation dose of 30% together with 0.01 mM K reduced leaf carotenoid content to 37.8 μg g−1 FW, but when the K application rate was 32 mM leaf carotenoid content was reduced to 66.0 μg g−1 FW. Nevertheless, an irrigation dose of 60% together with 16 mM K showed the least reduction (79.0 μg g−1 FW) in leaf carotenoid content. Likewise, an irrigation dose of 30% together with 0.01 mM K reduced root carotenoid content to 1.6 μg g−1 FW, but reduced to 6.6 μg g−1 FW when the K application rate was 32 mM, compared to full-irrigated plants together with 16 mM K. Interestingly, an irrigation dose of 60% together with 16 mM K resulted in the least reduction (9.1 μg g−1 FW) in root carotenoid content. Total carotenoid content was 9.7-fold higher in leaves than in roots. Further, it seems that low K rates under full irrigation affected carotenoid content more than starch content.
Irrigation and K treatments affected (P < 0.05) the total dietary fiber in leaves and roots of cassava (Figure 2) but showed no treatment interaction (I × K) in leaves (P = 0.816) and roots (P = 0.382). An irrigation dose of 30% reduced the total dietary fiber in leaves by 15% whereas an irrigation dose of 60% reduced total dietary fiber in leaves by 8%, when compared with full-irrigated plants. Compared with full-irrigated plants, an irrigation dose of 30% reduced total dietary fiber in roots by 33% whereas an irrigation dose of 60% reduced total dietary fiber in roots by 23%. Low-K treatments resulted in low total dietary fiber content, whereas a K rate of 16 mM resulted in the highest total dietary fiber content in leaves and roots, but was not significantly different from 32 mM K.
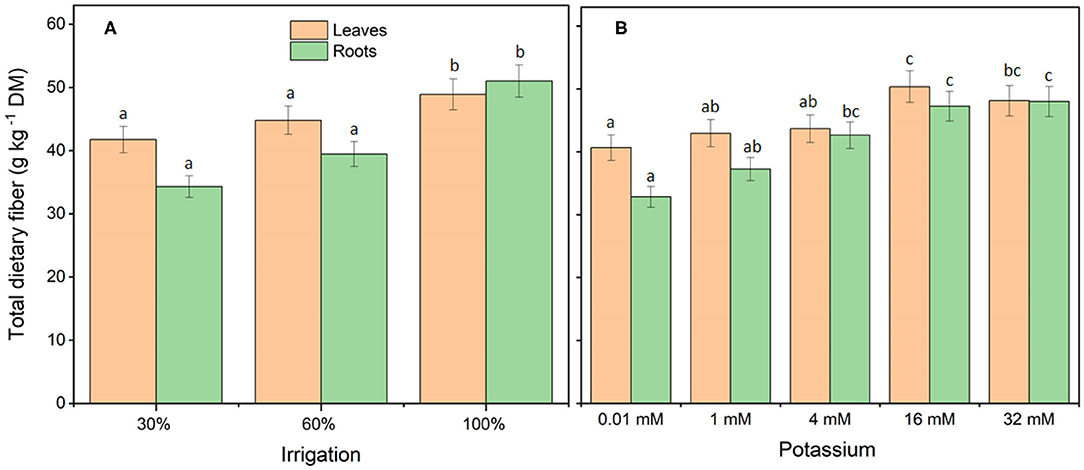
Figure 2. Total dietary fiber content of young cassava plants in response to deficit irrigation (A) and potassium fertigation (B). Total dietary fiber content in leaves and roots were both affected by irrigation (P < 0.001) and K (P < 0.001) but no treatment interactions (I × K) were observed in leaves (P = 0.816) and roots (P = 0.382). Treatments were initiated 30 days after planting and lasted 60 days. The data from four separate experiments were combined and shown as means ± standard errors; n = 4–16 replicate plants. Means followed by different letters in the same graph are significantly different (Tukey's test P < 0.05).
Irrigation and K treatments showed a significant (P < 0.05) interaction effect on Ca, S, and Mg content in both leaves and roots, as well as Fe and Cl content in leaves, and P, Zn, Mn, and Cu content in roots (Table 2). However, there was no interaction effect between irrigation and K treatments on leaf P, Na, Mn, and Cu content and on root Na and Fe content. Full irrigation (100%) together with 16 mM K resulted in the highest content for Ca, S, and Mg in both leaves and roots, as well as for Fe in leaves and Cu and Mn in roots. The highest leaf Cl content, as well as root P and Zn content, were obtained in full-irrigated plants together with 32 mM K. In contrast, an irrigation dose of 30% together with 0.01 mM K reduced the mineral content in leaves and roots the most. The mineral content in leaves and roots was the lowest with 0.01 mM K, except for Na, which was highest in leaves and roots, regardless of the irrigation dose. The major mineral content in leaves were highest to lowest in the order of Ca > Mg > P > S, whereas the minor minerals content followed the order of Na > Mn > Fe > Zn > Cl > Cu. Likewise, the major mineral content in roots followed the order of Ca > P > Mg > S, whereas the minor mineral content was in the order of Na > Fe > Mn > Zn > Cu. Notably, the mineral content was in general higher in leaves than roots except for Fe, which was higher in roots than leaves.
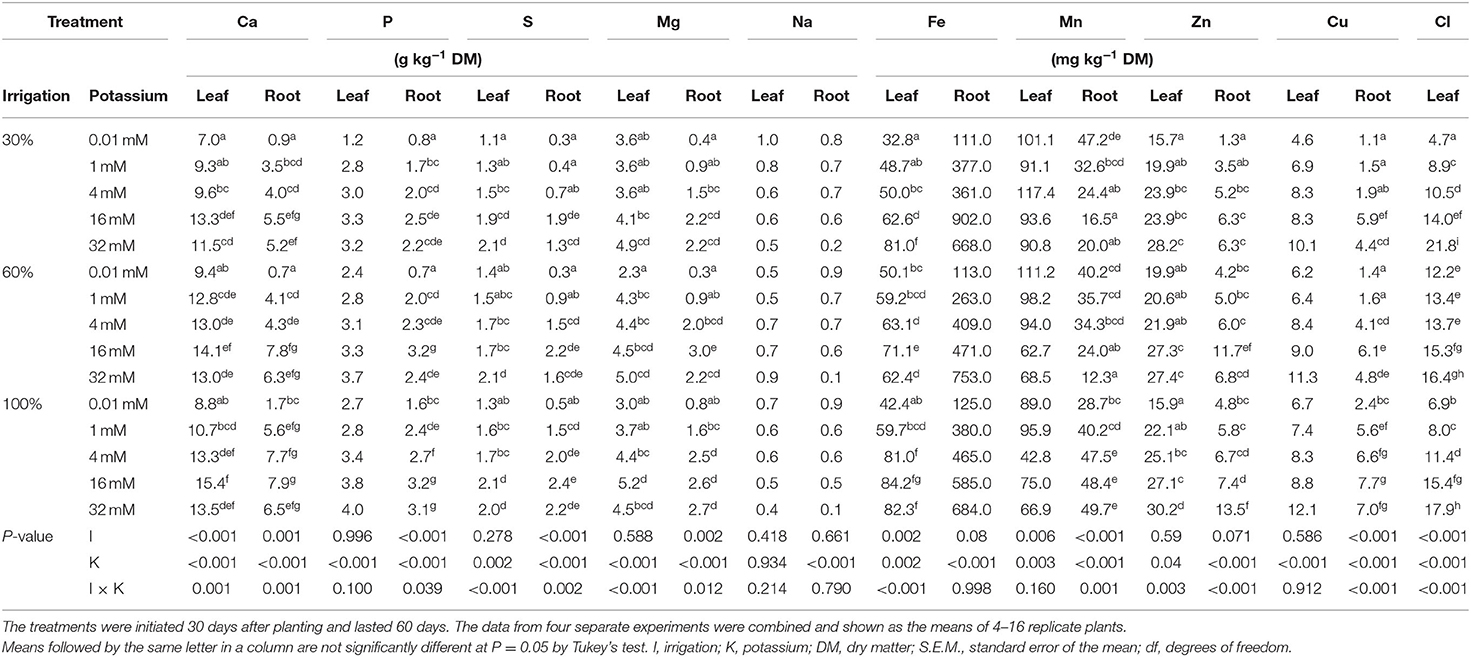
Table 2. Mineral content of young cassava plants in response to deficit irrigation and potassium fertigation.
Antinutritional Trait
The cyanide concentration in leaves and roots was affected by the irrigation and K treatments and their interaction (Table 1). The lowest cyanide concentration in leaves (2.2 mg kg−1 FW) and roots (1.0 mg kg−1 FW) were obtained in full-irrigated plants with 32 mM K. An irrigation dose of 30% together with 0.01 mM K increased cyanide concentration in leaves (25.9 mg kg−1 FW) and roots (12.8 mg kg−1 FW), but when the K application rate was 16 mM the cyanide concentration substantially decreased in leaves (10.5 mg kg−1 FW) and roots (5.6 mg kg−1 FW). An irrigation dose of 60% together with 16 mM K resulted in the lowest cyanide concentration in leaves (5.2 mg kg−1 FW) and roots (2.2 mg kg−1 FW) when compared with full-irrigated plants together with 32 mM K. In general, leaf cyanide concentration was double the root cyanide concentration regardless of the treatment.
Principal Component and Correlation Analyses
The Kaiser-Meyer-Olkin (KMO) test for the PCA of the combined leaves and roots data showed a value of 0.870, indicating that distinct and reliable components had been generated. The PCA showed 72.1% of the total variance in the first five components with Eigenvalues higher than 1.0 for each variable in the analyzed minerals. Moreover, the PCA separated the nutritive traits into clear clusters in the first two components (Figure 3). Most of the separation was achieved by PC1, which accounted for 44.5% of the total variance observed, whereas PC2 accounted for only 8.9%. Starch, total dietary fiber, total carotenoid content, and energy content were clustered together. Crude protein, Ca, Mg, Fe, P, S, and K consistently formed a separate cluster. Moreover, cyanide, Na and Mn content showed a negative relationship in the first principal component. The correlation analyses of the combined leaves and roots data confirmed the relationship between the nutrient contents (Table 3). Strong (P ≤ 0.01) positive associations (r > 0.5) were obtained between Ca, P, K, and Fe, as well as between crude protein, total carotenoid, starch, total dietary fiber, and energy content. The K content associated positively with Fe (r = 0.575), total carotenoid (r = 0.449), starch (r = 0.475), crude protein (r = 0.488), total dietary fiber (r = 0.467), and energy content (r = 0.417). Conversely, the K content had a strong negative association with cyanide concentration (r = −0.557), and a weak negative association with Cu and Mn. Cyanide concentration associated negatively with all the analyzed nutrients except for Mn.
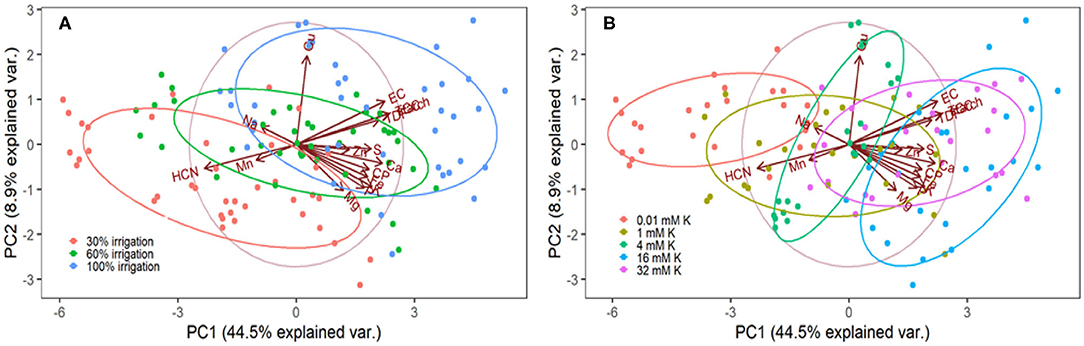
Figure 3. Biplot of the first two components of the Principal Component Analysis of combined nutrient content in leaves and roots of young cassava plants, as affected by irrigation (A) and potassium fertigation (B).
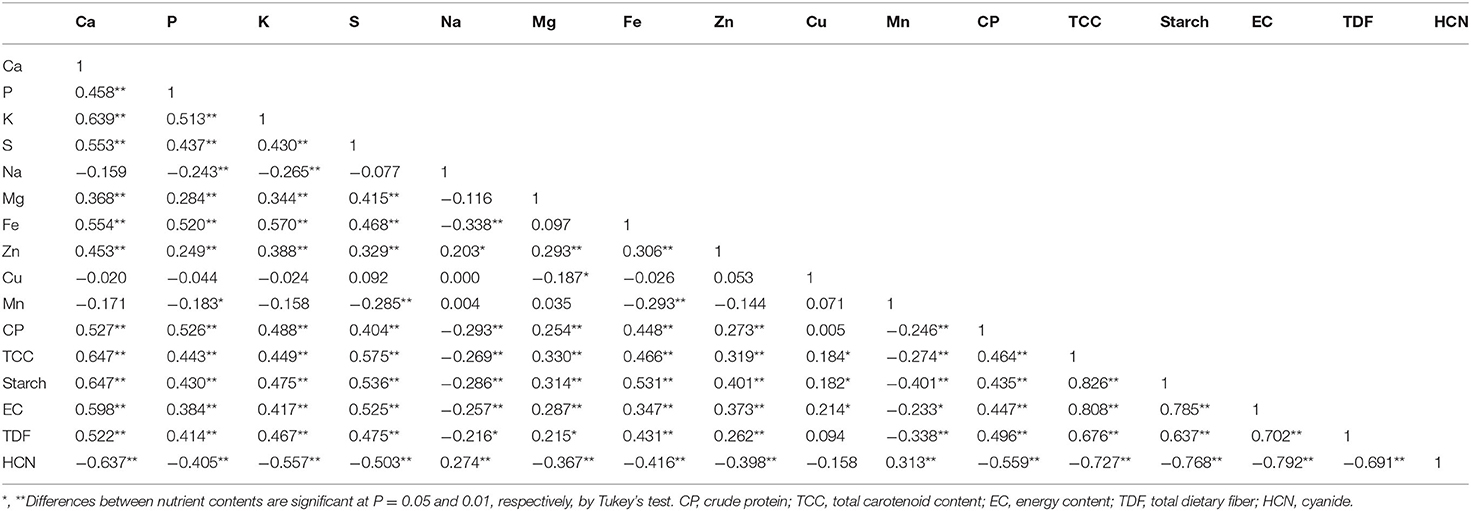
Table 3. Pearson correlation matrix for the combined nutrient contents in shoots and roots of young cassava plants in response to deficit irrigation and potassium fertigation.
Discussion
Nutritional Value of Cassava During the Early Growth Phase
The low starch content in young cassava leaves and roots obtained with a combination of 30% irrigation dose and 0.01 mM K could be related to reduced net photosynthesis, as demonstrated earlier by Wasonga et al. (2020). When net photosynthesis declines due to water deficit, the activity of ADP-glucose pyrophosphorylase—a key regulatory enzyme in starch synthesis—also declines (Kaur et al., 2017). Hence, inhibition of starch synthesis in leaves and storage of starch in roots results. The observed increased starch content in leaves and roots following the 30% irrigation dose combined with 16 mM K is attributed to the increased K supply. K is essential in carbohydrate translocation from photosynthesizing leaves to storage roots (Armengaud et al., 2009), and is known to stimulate the activity of ADP-glucose pyrophosphorylase and starch synthase enzymes involved in starch synthesis (Oosterhuis et al., 2014). The starch content in roots was 9 times higher than in leaves, demonstrating that cassava roots are richer in starch even in young plants. The higher starch content in roots than leaves could also explain the observed higher energy content in roots than leaves, given the strong positive correlation (r = 0.785) between energy content and starch content. Santisopasri et al. (2000) also reported lower starch content (1.2–3.5% DM) in cassava that was subjected to water deficit in the first 6 months after planting compared to higher starch content (20.4–25.9% DM) in control plants. Notably, cassava starch is an important source of energy in the diet of humans, and its gluten-free flour is suitable for individuals with an intolerance to gluten (Balagopalan, 2002). Consuming gluten-free cassava would avert celiac disease which affects ~1% of the global population (Catassi and Yachha, 2008).
Crude protein content was lowest in both leaves and roots with an irrigation dose of 30%. This suggests there were lower amounts and activity of photosynthesis-related enzymes in the leaves, resulting in reduced protein synthesis. Dhindsa and Cleland (1975) also reported a general reduction in protein synthesis in plants that were subjected to water deficit. The marked increase in crude protein content of leaves and roots with 16 mM K as opposed to low-K treatments may be due to the increased availability of N. K is involved in N metabolism (Marschner, 1995), and N is an essential macronutrient in protein synthesis (Fernandes et al., 2017). Moreover, K is known to activate the enzyme nitrate reductase which catalyzes the formation of proteins (Khanna-Chopra et al., 1980). The crude protein content of leaves was twice that of roots. According to Yeoh and Truong (1996), cassava roots are lower in protein than leaves. The crude protein content of leaves obtained in this investigation exceeds the FAO reference protein minimum of 0.55 g kg−1 day−1 (Harper, 1981). Thus, the young cassava leaves would serve as an ideal source of protein when consumed in diets; more so, since the protein digestibility from young leaves is often higher than the protein digestibility of older leaves (Lancaster and Brooks, 1983). Irrigation and K treatment combinations resulting in high leaf protein content is desirable for alleviating the protein-energy undernutrition common among humans in the tropics, even in cassava growing areas. Employing irrigation and K treatments for high protein productivity would allow cassava to better compete with other protein-based vegetable crops, such as cowpea (Vigna unguiculata L.). This would cut household expenses for protein-based foods and strengthen markets for cassava products, leading to sustainable nutrition.
The low carotenoid content in leaves and roots obtained with an irrigation dose of 30% together with 0.01 mM K could be attributed to limited carotenoid synthesis. Since carotenoids are crucial for the assembly of photosystems and harvesting light for photosynthesis (Nisar et al., 2015), the reduction in net photosynthesis due to water deficit (Duque and Setter, 2013) implies that carotenoid synthesis was limited. The limited carotenoid synthesis in leaves in turn resulted in minimal translocation of carotenoids to roots. Nevertheless, a K rate of 16 mM enhanced carotenoid synthesis, resulting in increased leaf carotenoid content. K is an essential co-factor required by pyruvic kinase and acetic thiokinase—two enzymes involved in the isoprenoid pathway of carotenoid synthesis (Trudel and Ozbun, 1970). Furthermore, low K limits the availability of geranyl-geranyl pyrophosphate, a precursor to carotenoids (Taber et al., 2008). The higher carotenoid content in leaves than in roots is consistent with an earlier finding that cassava leaves are richer in carotenoids (Montagnac et al., 2009). Thus, the leaves obtained with 16 mM or 32 mM K across the irrigation doses would provide valuable vitamin A when consumed, as the carotenoid contents were above the average daily requirement of carotene in children (2.4 mg), lactating women (5.0 mg), and adults (3.5 mg) (WHO., 1995). Humans cannot synthesize provitamin A carotenoids and their intake in the diet offers protection against night blindness, UV skin damage, and age-related degenerative diseases (Toti et al., 2018).
Polysaccharides make up the total dietary fiber content, and their synthesis depends mostly on photosynthetic activity in leaves. Photosynthetic activity is affected, in turn, by water deficit and potassium deficiency (Wasonga et al., 2020). This explains the low total dietary fiber content of leaves and roots obtained with an irrigation dose of 30% with 0.01 mM K as opposed to with 16 mM K fertigation. The total dietary fiber content obtained in this investigation would provide the adequate fiber intake of 25 g day−1 for women and 38 g day−1 for men as recommended by the panel on dietary reference intakes for macronutrients (Slavin, 2005). Diets rich in total dietary fiber are considered healthy and can reduce problems with constipation, obesity, and diabetes, and help prevent cancer and heart disease (Montagnac et al., 2009). However, consuming excess fiber can reduce protein digestibility (Ravindra, 1993).
The mineral content of cassava leaves and roots are important parameters for guiding its consideration for human consumption. In the present investigation, the mineral content of Fe and Cl in leaves, Cu, P, S, and Mg in roots, and Ca, K, Zn, and Mn in leaves and roots, were reduced when the irrigation dose was 30% but were highest in full-irrigated plants. This can be ascribed to the effect of water deficit, which likely reduced the uptake of the minerals. Water deficit reduces mineral uptake by the roots due to decreased rate of mineral diffusion from the soil matrix to the absorbing root surface (Hu et al., 2007). Nutrient transport from the roots to the shoots is also limited by a water deficit-induced decrease in the transpiration rate. For example, Merhaut (2007) reported that Mg is not physically or physiologically available to plants under conditions of water deficit. Increasing the K application rate resulted in an increase of Ca, P, Mg, Zn, Fe, and S content in both leaves and roots, and of Cl in leaves, regardless of the irrigation dose. An increased K supply to plants decreases the solute potential, which in turn causes a decrease in total water potential, creating a solute potential gradient which stimulates uptake of nutrients and water (Thompson and Zwieniecki, 2005). The Ca, S, and Mg content in leaves and roots were highest with 16 mM K but then reduced with 32 mM K. This suggests that 32 mM K fertigation was beyond optimum and possibly caused physiological antagonism. The ions of K, Ca, Mg, and S are quite similar in size, but the binding strength of K is usually much stronger and easily out-competes Ca, Mg, and S at exchange sites in the soil (Agbenin and Yakubu, 2006). Thus, the 32 mM K fertigation possibly reduced the plant's ability to absorb Ca, S, and Mg. In agreement, Howeler (2014) discovered reduced Ca, Mg, and S content in cassava that were over supplied with K. The Cl content was highest in full-irrigated plants which suggests that the abundant water likely mediated the increased Cl uptake by the plant compared to when the irrigation dose was 30%. Moreover, the Cl content in leaves was highest with 32 mM K, mainly because the Cl was supplied together with K as KCl and an increase in the K application rate likely increased the Cl content. Most crops are negatively affected only when plant Cl content exceeds 400 mg kg−1 DM (Marschner, 1995). For this reason, the Cl content obtained in this investigation was below toxic levels for the plant.
Mineral content was higher in cassava leaves than roots. Thus, consuming the leaves would provide vital minerals, especially Ca, Fe, Zn, S, and Mg, and improve the diets of humans in the tropics. The Ca content obtained with the various irrigation and K treatments would be sufficient in the diet as it was above the human requirement range of 1,000–1,500 mg day−1 (Bailey et al., 2010). Ca serves an important role in skeletal mineralization for maintaining healthy teeth and bones (Miller et al., 2001). The Fe content of leaves obtained with the different irrigation and K treatments would meet the minimum adult Fe requirement of 1.8 mg day−1 (Hurrell and Egli, 2010), which is important for the synthesis of hemoglobin and myoglobin, and for preventing anemia. Besides being a key component of proteins, dietary intake of S helps humans to detoxify cyanide by enzymic conversion of rhodanese to thiocyanate using S originating from dietary S amino acids (Cliff et al., 1985). The recommended daily allowance for Mg for adults is 310–420 mg [Institute of Medicine (US) Standing Committee on the Scientific Evaluation of Dietary Reference Intakes, 1997], which would be provided by the leaves in this investigation regardless of the irrigation dose and K rate. The human body maintains Mg content at 1,000 mmol, which is vital for normal nerve and muscle function, heart rhythm, blood pressure, the immune system, bone integrity, blood glucose levels, promoting calcium absorption, and fighting hypomagnesium (Laires et al., 2004). The leaves in this investigation would provide the estimated human Zn requirement of 15 mg day−1 (King and Turnlund, 1989). Zn is essential for transport and metabolism of vitamin A and curbs stunted growth and development common in Zn deficient persons (Christian and West, 1998). Thus, nutrition-driven agricultural production within environmental limits could solve global malnutrition problems.
Anti-nutrient Value of Cassava During the Early Growth Phase
Cassava leaves contain the cyanogenic glucosides, linamarin (95%) and lotaustralin (5%) (Conn, 1994), which on hydrolysis by the enzyme linamarase yield cyanohydrins that release toxic hydrogen cyanide (HCN) when hydrolyzed by the enzyme hydroxynitrile lyase (Cardoso et al., 2005). The cyanide concentration in the present investigation was two times higher in leaves than in roots, which is ascribed to linamarin levels more than 20 times higher in leaves than in roots (Siritunga and Sayre, 2004; Jørgensen et al., 2005). The cyanide concentration in leaves and roots was highest with an irrigation dose of 30% together with 0.01 mM K. This suggests that water deficit could have triggered an increase in synthesis of cyanogenic glucosides in leaves, given that the cyanide concentration was minimal with an irrigation dose of 60% together with 0.01 mM K and was lowest in full-irrigated plants. The effect of reduced cassava yields due to water deficit might also create a concentration effect (increased cyanide per mass) to further increase cyanide concentration (Burns et al., 2010). When the K application rate was 16 mM the leaf and root cyanide concentration substantially reduced, even when the irrigation dose was 30%, compared to with lower K application rates. This observation could be linked to the effect of K in moderating the synthesis of the linamarase enzyme responsible for cyanogenic glucoside production in cassava (Susan John et al., 2016). El-Sharkawy and Cadavid (2000) also found reduced root cyanide concentration in cassava plants that were grown with K fertilizer under rain-fed field conditions. The increased cyanide concentration with 32 mM K fertigation, especially when the irrigation dose was either 30 or 60%, could be associated with the reduced content of Ca, Mg, and S—whose uptake was restricted by 32 mM K. Like K, adequate uptake of Ca, Mg, Zn, and S is beneficial for reducing cyanogenic glucosides in cassava (Susan John et al., 2016). The harvested cassava leaves and roots would be safe for consumption as the cyanide concentrations were less than the FAO maximum recommended level of 50 mg kg−1 HCN in FW (Codex Alimentarius Commission, 2013). Cassava cultivars are classified as “sweet” when they contain <100 mg kg−1 HCN in FW, or “bitter” when they contain 100–500 mg kg−1 HCN in FW (Alves, 2002). Cyanide concentration also varies depending on plant maturity status, whereby young plants have lower cyanide concentration than older plants (Wobeto et al., 2007). Consuming more than 50 to 100 mg of HCN within 24 h can cause acute toxicity, as cyanide inhibits cytochrome oxidase in respiratory electron transport leading to oxygen starvation at cellular level and eventual death (Rosling, 1994).
Conclusions
Affordable technologies that improve the nutritional traits of cassava, such as K fertigation with deficit irrigation, could be crucial for millions of smallholder farmers in the tropics who struggle to produce quality cassava to meet their dietary requirements. An irrigation dose of 30% together with 0.01 mM K lowered the nutritional quality and increased the anti-nutritional quality of young cassava plants, whereas full irrigation with 16 mM K gave the highest nutritional quality with the least anti-nutritional quality. However, an irrigation dose of 60% together with 16 mM K produced a good quality yield and caused the least reduction in nutritional quality compared to full-irrigated plants together with 16 mM K. In addition, fertigation with 16 mM K markedly increased the nutritional quality but reduced the anti-nutrient quality regardless of the irrigation dose. Though these findings were obtained under controlled conditions with pot-grown plants, they still give a good indication that the nutritive quality of cassava growing in drought-prone areas could be improved by optimizing both irrigation and K application rates. Further testing in the field with more cultivars is necessary to develop knowledge-based K fertigation programs for cassava-growing areas.
Data Availability Statement
The original contributions presented in the study are included in the article/supplementary materials, further inquiries can be directed to the corresponding author.
Author Contributions
DW and PM proposed the framework of the study. DW, PM, and JK designed the experiment. DW conducted the experiments, organized the database, analyzed the data, and wrote the first draft of the manuscript. PM, JK, and LA provided revisions for the manuscript. All authors read and approved the submitted version.
Funding
The authors were grateful to CIMO Finland (TM-17-10552) for providing the financial support for this research. DW had a research grant by the Ella and Georg Ehrnrooth Foundation. Open access publication fee was covered by University of Helsinki. The funders had no role in study design, data collection and analysis, preparation of the manuscript, or decision to publish.
Conflict of Interest
The authors declare that the research was conducted in the absence of any commercial or financial relationships that could be construed as a potential conflict of interest.
Acknowledgments
We acknowledge Marjo Kilpinen and Markku Tykkyläinen for assistance with laboratory analyses, the Teppo Mattson for assistance with statistical interpretations. The Kenya Agricultural and Livestock Research Organization (KALRO) is acknowledged for providing the cassava cuttings used in this investigation.
References
Achidi, A. U., Ajayi, O. A., Maziya-Dixon, B., and Bokanga, M. (2008). The effect of processing on the nutrient content of cassava (Manihot esculenta Crantz) leaves. J. Food Process. Preserv. 32, 486–502. doi: 10.1111/j.1745-4549.2007.00165.x
Agbenin, J. O., and Yakubu, S. (2006). Potassium-calcium and potassium-magnesium exchange equilibria in an acid savanna soil from northern Nigeria. Geoderma 136, 542–554. doi: 10.1016/j.geoderma.2006.04.008
Alves, A. A. C. (2002). “Cassava botany and physiology,” in Cassava: Biology, Production and Utilization, eds R. J. Hillocks, J. M. Tres, and A. C. Bellotti (Wallingford, CT: CABI Publishing), 67–89.
Armengaud, P., Sulpice, R., Miller, A. J., Stitt, M., Amtmann, A., and Gibon, Y. (2009). Multilevel analysis of primary metabolism provides new insights into the role of potassium nutrition for glycolysis and nitrogen assimilation in Arabidopsis roots. Plant Physiol. 150, 772–785. doi: 10.1104/pp.108.133629
Bailey, R. L., Dodd, K. W., Goldman, J. A., Gahche, J. J., Dwyer, J. T., Moshfegh, A. J., et al. (2010). Estimation of total usual calcium and vitamin D intake in the United States. J. Nutr. 140, 817–822. doi: 10.3945/jn.109.118539
Balagopalan, C. (2002). “Cassava utilization in food, feed and industry,” in Cassava: Biology, Production and Utilization, eds R. J. Hillock, J. M. Thresh, and A. C. Bellotti (Oxfordshire: CABI Wallingford), 301–318.
Burns, A., Gleadow, R., Cliff, J., Zacarias, A., and Cavagnaro, T. (2010). Cassava: the drought, war and famine crop in a changing world. Sustainability 2, 3572–3607. doi: 10.3390/su2113572
Cakmak, I. (2010). Potassium for better crop production and quality. Plant Soil. 335, 1–2. doi: 10.1007/s11104-010-0534-8
Cardoso, A. P., Mirione, E., Ernesto, M., Massaza, F., Haque, M. R., Bradbury, J. H., et al. (2005). Processing of cassava roots to remove cyanogens. J. Food Compos. Anal. 18, 451–460. doi: 10.1016/j.jfca.2004.04.002
Catassi, C., and Yachha, S. K. (2008). The global village of celiac disease. Front. Celiac Dis. 12, 23–31. doi: 10.1159/000128610
Chávez, A. L., Sánchez, T., Jaramillo, G., Bedoya, J. M., Echeverry, J., Bolaños, E. A., et al. (2005). Variation of quality traits in cassava roots evaluated in landraces and improved clones. Euphytica 143, 125–133. doi: 10.1007/s10681-005-3057-2
Christian, P., and West, K. P. (1998). Interactions between zinc and vitamin A: an update. Am. J. Clin. Nutr. 68, 435S−441S. doi: 10.1093/ajcn/68.2.435S
Cliff, J., Martensson, J., Lundqvist, P., Rosling, H., and Sorbo, B. (1985). Association of high cyanide and low sulfur intake in cassava-induced spastic paraparesis. Lancet 2, 1211–1213. doi: 10.1016/S0140-6736(85)90742-1
Codex Alimentarius Commission (CAC) (2013). Proposed Draft for Maximum Levels for Hydrocyanic Acid in Cassava and Cassava Products. Moscow: Joint FAO/WHO Food Standards Programme, 34. Available online at: http://www.fao.org/tempref/codex/Meetings/CCCF/cccf7/cf07_10e.pdf
Conn, E. (1994). Cyanogenesis - a personal perspective. Acta Hortic. 375, 31–43. doi: 10.17660/ActaHortic.1994.375.1
Cuvaca, I. B., Eash, N. S., Zivanovic, S., Lambert, D. M., Walker, F., and Rustrick, B. (2015). Cassava (Manihot esculenta Crantz) tuber quality as measured by starch and cyanide (HCN) affected by nitrogen, phosphorus, and potassium fertilizer rates. J Agric Sci. 7, 36–49. doi: 10.5539/jas.v7n6p36
Dhindsa, R. S., and Cleland, R. E. (1975). Water stress and protein synthesis: differential inhibition of protein synthesis. Plant Physiol. 55, 778–781. doi: 10.1104/pp.55.4.778
Duque, L. O., and Setter, T. L. (2013). Cassava response to water deficit in deep pots: root and shoot growth, ABA, and carbohydrate reserves in stems, leaves and storage roots. Trop. Plant Biol. 6, 199–209. doi: 10.1007/s12042-013-9131-3
El-Sharkawy, M. A. (1993). Drought-tolerant cassava for Africa, Asia, and Latin America. BioScience 43, 441–451. doi: 10.2307/1311903
El-Sharkawy, M. A. (2003). Cassava biology and physiology. Plant Mol. Biol. 53, 621–641. doi: 10.1023/B:PLAN.0000019109.01740.c6
El-Sharkawy, M. A., and Cadavid, L. F. (2000). Genetic variation within cassava germplasm in response to potassium. Exp. Agr. 36, 323–334. doi: 10.1017/S0014479700003045
Essers, A. J. A. (1995). Removal of Cyanogens from cassava roots: studies in domestic sun-drying and solid -substrate fermentation in rural Africa (Ph.D. thesis). Wageningen Agricultural University, Wageningen, Netherlands.
FAO (2019). The State of Food Security and Nutrition in the World. Safeguarding Against Economic Slowdowns and Downturns. Rome: FAO. Available online at: http://www.fao.org/state-of-food-security-nutrition
Fernandes, A. M., Gazola, B., Nunes, J. G., Garcia, E. L., and Leonel, M. (2017). Yield and nutritional requirements of cassava in response to potassium fertilizer in the second cycle. J. Plant Nutr. 40, 2785–2796. doi: 10.1080/01904167.2017.1382520
Gegios, A., Amthor, R., Maziya-Dixon, B., Egesi, C., Mallowa, S., Nungo, R., et al. (2010). Children consuming cassava as a staple food are at risk for inadequate zinc, iron, and vitamin A intake. Plant Foods Hum. Nutr. 65, 64–70. doi: 10.1007/s11130-010-0157-5
Gruhn, P., Goletti, F., and Yudelman, M. (2000). Integrated Nutrient Management, Soil Fertility, and Sustainable Agriculture: Current Issues and Future Challenges. Food, Agriculture, and the Environment Discussion Paper 32 (Washington, DC: International Food Policy Research Institute).
Harper, A. E. (1981). “Task force II report,” in Protein Quality in Humans: Assessment and In Vitro Estimation eds C. E. Bodwell, J. S. Adkins, and D. T. Hopkins (Westport, CT: AVI Publishing Inc.,), 417–20.
Helal, N. A. S., Eisa, S. S., and Attia, A. (2013). Morphological and chemical studies on influence of water deficit on cassava. World J. Agric. Sci. 9, 369–376. doi: 10.5829/idosi.wjas.2013.9.5.1801
Hoagland, D. R., and Arnon, D. I. (1950). The water-culture method for growing plants without soil. Calif. Agric. Exp. Stat. Cir. 347, 1–39.
Horwitz, W. (1980). Official Methods of Analysis. Washington, DC: The Association of Official Analytical Chemists.
Howeler, R. (2014). Sustainable Soil and Crop Management of Cassava in Asia: A Reference Manual. Cali: Centro Internacional de Agricultura Tropical (CIAT), 280.
Howeler, R. H. (1998). “Cassava agronomy research in Asia – an overview, 1993–1996,” in Cassava Breeding, Agronomy and Farmer Participatory Research in Asia. Proceedings of the 5th Regional Workshop, ed R. H. Howeler (Danzhou), 355–375. Available online at: http://ciat-library.ciat.cgiar.org/
Howlett, W. P. (1994). Konzo a new human disease entity. Acta Hortic. 375, 323–329. doi: 10.17660/ActaHortic.1994.375.32
Hu, Y., Burucs, Z., Tucher, S. V., and Schmidhalter, U. (2007). Short-term effects of drought and salinity on mineral nutrient distribution along growing leaves of maize seedlings. Environ. Exp. Bot. 60, 268–275. doi: 10.1016/j.envexpbot.2006.11.003
Hurrell, R., and Egli, I. (2010). Iron bioavailability and dietary reference values. Am. J. Clin Nutr. 91, 1461S−1467S. doi: 10.3945/ajcn.2010.28674F
Institute of Medicine (US) Standing Committee on the Scientific Evaluation of Dietary Reference Intakes (1997). Dietary Reference Intakes for Calcium, Phosphorus, Magnesium, Vitamin D, and Fluoride. Washington, DC: National Academies Press.
Jørgensen, K., Bak, S., Busk, P., Sørensen, C., Olsen, C., Puonti-Kaerlas, J., et al. (2005). Cassava plants with a depleted cyanogenic glucoside content in leaves and tubers. Distribution of cyanogenic glucosides, their site of synthesis and transport, and blockage of the biosynthesis by RNA interference technology. Plant Physiol. 139, 363–374. doi: 10.1104/pp.105.065904
Kaur, V., Madaan, S., and Behl, R. K. (2017). ADP-glucose pyrophosphorylase activity in relation to yield potential of wheat: Response to independent and combined high temperature and drought stress. Cereal Res. Commun. 45, 181–191. doi: 10.1556/0806.45.2017.003
Khanna-Chopra, R., Chaturverdi, G. S., Aggarwal, P. K., and Sinha, S. K. (1980). Effect of potassium on growth and nitrate reductase during water stress and recovery in maize. Physiol. Plant 49, 495–500. doi: 10.1111/j.1399-3054.1980.tb03340.x
King, J. C., and Turnlund, J. R. (1989). “Human zinc requirements,” in Zinc in Human Biology. ILSI Human Nutrition Reviews, ed C. F. Mills (London: Springer), 335–350.
Laires, M. J., Monteiro, C. P., and Bicho, M. (2004). Role of cellular magnesium in health and human disease. Front. Biosci. 9, 262–276. doi: 10.2741/1223
Lancaster, P. A., and Brooks, J. E. (1983). Cassava leaves as human food. Econ. Bot. 37, 331–348. doi: 10.1007/BF02858890
Latif, S., and Müller, J. (2015). Potential of cassava leaves in human nutrition: a review. Trends Food Sci. Technol. 44, 147–158. doi: 10.1016/j.tifs.2015.04.006
Mäkelä, P., Munns, R., Colmer, T. D., and Peltonen-Sainio, P. (2003). Growth of tomato and an ABA-deficient mutant (sitiens) under saline conditions. Physiol. Plantarum. 117, 58–63. doi: 10.1034/j.1399-3054.2003.1170107.x
Merhaut, D. J. (2007). “Magnesium,” in Handbook of Plant Nutrition, 1st Edn., eds A. B. V. Barker and D. J. Pilbeam (New York, NY: CRC Taylor and Francis), 145–181.
Miller, G. D., Jarvis, J. K., and McBean, L. D. (2001). The importance of meeting calcium needs with foods. J. Am. Coll. Nutr. 20, 168S−185S. doi: 10.1080/07315724.2001.10719029
Montagnac, J. A., Davis, C. R., and Tanumihardjo, S. A. (2009). Nutritional value of cassava for use as a staple food and recent advances for improvement. Compr. Rev. Food Sci. Food Saf. 8, 181–194. doi: 10.1111/j.1541-4337.2009.00077.x
Ndung'u, J. N., Wachira, F. N., Kinyua, M. G., Lelgut, D. K., Okwaro, H., Njau, P., et al. (2012). Influence of the environment on cassava quality traits in central rift valley of Kenya. Am J Plant Sci. 3, 1504–1512. doi: 10.4236/ajps.2012.310181
Ngudi, D. D., Kuo, Y.-H., and Lambein, F. (2003). Amino acid profiles and protein quality of cooked cassava leaves or ‘saka saka'. J. Sci. Food Agric. 83, 529–534. doi: 10.1002/jsfa.1373
Nisar, N., Li, L., Lu, S., Khin, N. C., and Pogson, B. J. (2015). Carotenoid metabolism in plants. Mol. Plant 8, 68–82. doi: 10.1016/j.molp.2014.12.007
Oliveira, R. G. A., de Carvalho, M. J. L., Nutti, R. M., de Carvalho, L. V. J., and Fukuda, W. G. (2010). Assessment and degradation study of total carotenoid and ß-carotene in bitter yellow cassava (Manihot esculenta Crantz) varieties. Afr. J. Food Sci. 4, 148–155.
Oosterhuis, D. M., Loka, D. A., Kawakami, E. M., and Pettigrew, W. T. (2014). The physiology of potassium in crop production. Adv. Agron. 126, 203–233. doi: 10.1016/B978-0-12-800132-5.00003-1
Oresegun, A., Fagbenro, O. A., Ilona, P., and Bernard, E. (2016). Nutritional and anti-nutritional composition of cassava leaf protein concentrate from six cassava varieties leaf for in aqua feed. Cogent Food Agric. 2:1147323. doi: 10.1080/23311932.2016.1147323
Osuntokun, B. (1994). Chronic cyanide intoxication of dietary origin and a degenerative neuropathy in Nigerians. Acta Hortic. 75, 311–321. doi: 10.17660/ActaHortic.1994.375.31
Ravindra, V. (1993). Cassava leaves as animal feed: potential and limitations. J. Sci. Food Agric. 61, 141–150. doi: 10.1002/jsfa.2740610202
Rosling, H. (1994). Measuring effects in humans of dietary cyanide exposure from cassava. Acta Hortic. 375, 271–283. doi: 10.17660/ActaHortic.1994.375.27
Santisopasri, V., Kurotjanawong, K., Chotineeranat, S., Piyachomkwan, K., Sriroth, K., and Oates, C. G. (2000). Impact of water stress on yield and quality of cassava starch. Ind. Crops Prod. 13, 115–129. doi: 10.1016/S0926-6690(00)00058-3
Seleiman, M. F., Santanen, A., Stoddard, F. L., and Mäkelä, P. (2012). Feedstock quality and growth of bioenergy crops fertilized with sewage sludge. Chemosphere 89, 1211–1217. doi: 10.1016/j.chemosphere.2012.07.031
Siritunga, D., and Sayre, R. (2004). Engineering cyanogen synthesis and turnover in cassava (Manihot esculenta). Plant Mol. Biol. 56, 661–669. doi: 10.1007/s11103-004-3415-9
Slavin, J. L. (2005). Dietary fiber and body weight. Nutrition 21, 411–418. doi: 10.1016/j.nut.2004.08.018
Susan John, K., George, J., Shanida Beegum, S. U., and Shivay, Y. S. (2016). Soil fertility and nutrient management in tropical tuber crops. an overview. Ind. J. Agron. 61, 263–273. Available online at: https://www.researchgate.net/publication/318259681_Soil_fertility_and_nutrient_management_in_tropical_tuber_crops_-_An_overview
Taber, H., Perkins-Veazie, P., Li, S., White, W., Rodermel, S., and Xu, Y. (2008). Enhancement of tomato fruit lycopene by potassium is cultivar dependent. HortScience 43, 159–165. doi: 10.21273/HORTSCI.43.1.159
Talsma, E., Brouwer, I., Verhoef, H., Mbera, G., Mwangi, A., Demir, A., et al. (2016). Biofortified yellow cassava and vitamin A status of Kenyan children: a randomized controlled trial. Am. J. Clin. Nutr. 103, 258–267. doi: 10.3945/ajcn.114.100164
Talsma, E. F., Melse-Boonstra, A., Kok, B. P., Mbera, G. N. K., Mwangi, A. M., and Brouwer, I. D. (2013). Biofortified cassava with provitamin A is sensory and culturally acceptable for consumption by primary school children in Kenya. PLoS ONE 8:e73433. doi: 10.1371/journal.pone.0073433
Thompson, M. V., and Zwieniecki, M. A. (2005). “The role of potassium in long distance transport in plants,” in Physiological Ecology, Vascular Transport in Plants, eds N. Michele Holbrook and M. A. Zwieniecki (Burlighton: Academic Press), 22–240. doi: 10.1016/B978-012088457-5/50013-7
Toti, E., Chen, C. O., Palmery, M., Villaño, V. D., and Peluso, I. (2018). Non-provitamin A and provitamin A carotenoids as immunomodulators: recommended dietary allowance, therapeutic index, or personalized nutrition? Oxid. Med. Cell. Longev. 2018:4637861. doi: 10.1155/2018/4637861
Trudel, M. J., and Ozbun, J. L. (1970). Relationship between chlorophylls and carotenoids of ripening tomato fruit as influenced by potassium nutrition. J. Exp. Bot. 21, 881–886. doi: 10.1093/jxb/21.4.881
Wasonga, D., Kleemola, J., Alakukku, L., and Mäkelä, P. (2020). Growth response of cassava to deficit irrigation and potassium fertigation during the early growth phase. Agronomy 10, 321–335. doi: 10.3390/agronomy10030321
WHO. (1995). Global Prevalence of Vitamin A Deficiency. Micronutrient Deficiency Information System Working Paper No. 2. Geneva.
Williams, S., (ed.). (1990). “Hydrocyanic acid in beans, alkaline titration method,” in Official Method of Analysis of the Association of Official Analytical Chemists (Arlington, VA: AOAC Inc), 1213.
Wobeto, C., Corrêa, A. D., Abreu, C. M. P., Santos, C., and Do Pereira, H. V. (2007). Antinutrients in the cassava (Manihot esculenta Crantz) leaf powder at three ages of the plant. Cienc. Technol. Aliment. 27, 108–112. doi: 10.1590/S0101-20612007000100019
Keywords: starch, fiber, cyanide, energy, crude protein, carotenoid
Citation: Wasonga DO, Kleemola J, Alakukku L and Mäkelä PSA (2020) Potassium Fertigation With Deficit Irrigation Improves the Nutritive Quality of Cassava. Front. Sustain. Food Syst. 4:575353. doi: 10.3389/fsufs.2020.575353
Received: 23 June 2020; Accepted: 12 October 2020;
Published: 30 October 2020.
Edited by:
Kathleen L. Hefferon, Cornell University, United StatesReviewed by:
Roslyn Gleadow, Monash University, AustraliaChiedozie Egesi, Cornell University, United States
Copyright © 2020 Wasonga, Kleemola, Alakukku and Mäkelä. This is an open-access article distributed under the terms of the Creative Commons Attribution License (CC BY). The use, distribution or reproduction in other forums is permitted, provided the original author(s) and the copyright owner(s) are credited and that the original publication in this journal is cited, in accordance with accepted academic practice. No use, distribution or reproduction is permitted which does not comply with these terms.
*Correspondence: Daniel O. Wasonga, daniel.wasonga@helsinki.fi