- 1Department of Entomology, Rutgers University, New Brunswick, NJ, United States
- 2USDA-ARS, Southeastern Fruit and Nut Laboratory, Byron, GA, United States
- 3Agroscope, Entomology in Field Crops and Viticulture, Plant Protection Strategic Research Division, Nyon, Switzerland
The emphasis of this review is on the use and potential of entomopathogenic nematodes (EPNs) as biological control agents in sustainable food production across a wide range of agricultural and other commodities. To aid with the understanding of the potential of EPNs in sustainable food production, this review also provides overviews on EPN biology and ecology, mass production and application technology, and interactions with other management tools. First discovered in the 1920s, their commercialization as biopesticides in the 1980s was accompanied and followed by an exponential growth in research on their application, biology, and ecology, followed by a further expansion in more basic research areas since the mid-2000s. This review summarizes significant progress made in the research and application of EPN in insect pest management in important food crops including orchards, small fruit, maize, vegetables, tuber crops, greenhouses, and mushrooms. Significant factors affecting the success of EPN commercialization are also discussed. A growing interest in alternatives to synthetic insecticides and in organic agriculture opens opportunities for EPNs, but EPNs will need to be further improved with respect to efficacy, reduced costs, and ease of use. Moreover, their potential to recycle in host populations beckons to be further exploited for long term pest suppression.
Introduction
First discovered in the 1920s, entomopathogenic nematodes (EPNs) received increasing interest starting in the 1950, and their commercialization started in the 1980s. They have been largely excluded from pesticide registration requirements in many countries due to their high level of safety to humans, non-target organisms, and the environment (Ehlers, 2005; Piedra Buena et al., 2015). This in turn has aided EPN commercialization leading to the commercial development of at least five Heterorhabditis species and eight Steinernema species (Table 1). Research on the application, biology, and ecology of EPNs saw exponential growth starting in the 1990s and further expanded into major basic research in the 2000s. The extensive body of literature resulting from this research has been reviewed comprehensively and extensively in the chapters of several seminal books (Gaugler and Kaya, 1990; Bedding et al., 1993; Gaugler, 2002; Grewal et al., 2005; Campos-Herrera, 2015) which will be used as our primary references. Additional references will be listed for important and relevant studies or more specific reviews that were not or only briefly described in these chapters.
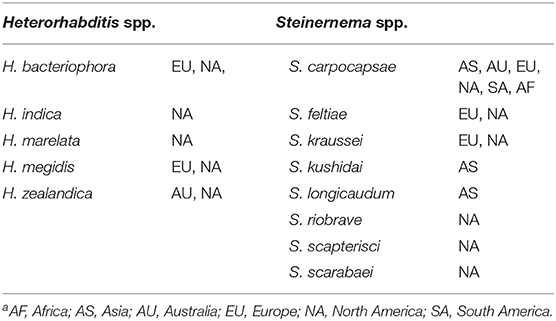
Table 1. Currently or recently commercialized entomopathogenic nematode species and where they are or have been commercializeda.
EPN Biology and Ecology
Biology, Host Range, and Virulence
EPNs have been recovered from soils around the world. At least 96 Steinernema, 1 Neosteinernema, and 21 Heterorhabditis species have been described to date (Lewis and Clarke, 2012; Shapiro-Ilan et al., 2017, 2018). They are mostly classified in the not closely related families Heterorhabditidae and Steinernematidae that share a number of characteristics through convergent evolution. Several species of nematodes in the genus Oscheius in the family Rhabditidae also meet the criteria to be categorized as EPNs (Zhang et al., 2009; Torres-Barragan et al., 2011; Dillman et al., 2012). Some Oscheius species appear to be associated with only one species of bacterial symbionts while other members of the genus are associated with multiple bacterial species. However, these species are not as well characterized ecologically and are not used in biological control applications. This review therefore focusses on the Steinernematidae and Heterorhabditidae.
EPNs are parasites of arthropods in nature that are only horizontally transmitted and possess an infective juvenile (IJ) stage that actively invades the insect host. They are always associated with symbiotic bacteria that play an important role in host infection. The biology of the EPN-symbiotic bacteria complex has been extensively reviewed in Burnell and Stock (2000), Griffin et al. (2005), Lewis and Clarke (2012), Stock (2015), and Shapiro-Ilan et al. (2017, 2018).
All known EPN species share a similar biology. The only stage that survives outside of a host is the non-feeding, non-developing third stage infective juvenile (IJ) or dauer juvenile. IJs use environmental and host cues to locate, recognize and accept insects as potential hosts. They enter a host through natural openings (mouth, anus, spiracles) or directly penetrate through thin parts of the cuticle. After establishing in the hemocoel, IJs molt and release their symbiotic bacteria through defecation or regurgitation. Nematodes and bacteria cooperate to kill a susceptible host insect within 24–48 h. IJs of Steinernema spp. develop into male and female adults, and following generations in the host also produce males and females (with the exception of Steinernema hermaphroditum). Heterorhabditis spp. IJs develop to hermaphroditic adults in the first generation, but subsequent generations include males, females and hermaphroditic individuals (Figure 1). The nematodes feed on bacteria cells and host tissues digested by the bacteria, and over 1–4 weeks go through one to three generations until host resources are depleted. At that point, 100s to 100s of thousands new IJs with symbiotic bacteria cells in their nonfunctional digestive system leave the host cadaver to forage for new insect hosts (Figure 1). Steinernema spp. IJs harbor the bacterial cells in a specialized vesicle in their anterior gut whereas Heterorhabditis spp. carry them attached to their pre-intestinal valve.
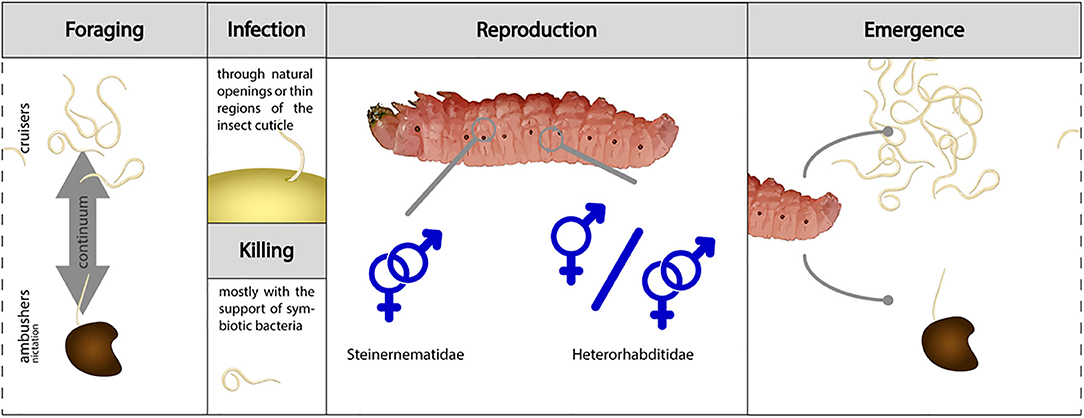
Figure 1. The life cycles of Heterorhabditis and Steinernema entomopathogenic nematodes. Foraging infective juveniles (IJs) can adopt various behaviors spanning from active search for a host (cruiser) to the passive strategy of nictation (ambusher). Once a host has been located and accepted, infection can occur through natural openings or by piercing thin regions of the cuticle. In the hemolymph, IJs release their symbiotic bacteria and the host dies within 24-48 h by toxemia and septicemia. EPNs go through up to three generations inside the host cadaver. In most Steinernema spp., adults are always male and females; in Heterorhabditis spp., adults of the first generation are hermaphrodites, whereas following generations contain hermaphrodites, females, and males. When space and food resources become depleted, a new cohort of IJs leaves the host cadaver and starts foraging for a new host.
The symbiotic bacteria of EPNs fall in the genera Photorhabdus (Heterorhabditis spp.) and Xenorhabdus (Steinernema spp.). Once members of the family Enterobacteriaceae, Photorhabdus and Xenorhabdus are now seated within the family Morganellaceae (Enterobacteriales) along with the type genus Morganella and five other genera (Adeolu et al., 2016). Currently, there are 19 species of Photorhabdus and 26 species of Xenorhabdus. The association between nematode and bacterium is highly specific. In nature, the bacteria have no infective capabilities and cannot persist outside the nematodes or insect host and rely on the nematode to vector them from host to host. However, the bacteria play a major role in killing the insect host through suppression of the immune system causing toxemia and septicemia. They also produce antibiotics that prevent secondary host invasions, a deterrent factor that discourages scavengers from feeding on the host cadaver (Photorhabdus spp. only), and serve as a food resource for the nematodes. The exact role of bacteria and nematodes in overcoming the immune response has only been studied in depth in a few nematode-bacteria combinations and in a few host species. Based on those studies, in Photorhabdus-Heterorhabditis combinations, the bacteria play the major role in killing the host whereas in Steinernema-Xenorhabdus combinations, the nematodes play a more active role in contributing to the virulence of the nematode-bacterium complex (Lu et al., 2017).
The host range of most known EPN species remains mostly unknown to date (Peters, 1996; Shapiro-Ilan et al., 2017, 2018) because most species have been isolated from soil samples using the highly susceptible wax moth, Galleria mellonella, larvae as a bait insect. Many EPN species may infect a wide range of insect species in laboratory assays (e.g., S. carpocapsae > 200 insects across 10 orders). But after field applications and especially in nature, the host range is much narrower due to the ecology of the nematodes and its potential hosts. Some species that have been isolated from natural hosts in the field are particularly well adapted to a narrow group of hosts species but show poor infection of other hosts (i.e., S. scapterisci is adapted to Orthoptera and S. kushidai and S. scarabaei to larvae of Scarabaeidae).
Host defenses and immune reactions in response to EPN infection have been studied only in a few EPN species-insect species combinations (reviewed in Lewis and Clarke, 2012; Shapiro-Ilan et al., 2017, 2018). Behavioral defenses may include intensive grooming behavior when in contact with IJs to prevent infection and evasion of areas with high numbers of IJs (scarabaeid white grubs). Physical barriers to prevent IJs from reaching the hemocoel may include reduced access to the hemocoel via the mouth through forward projecting hairs in the preoral cavity (elaterid wireworms) or a thick peritrophic membrane protecting the midgut epithelium (white grubs). Narrow, slit-like openings of the spiracles (wireworms) or fine sieve-like plates covering the spiracles (white grubs) may limit access to the hemocoel via the tracheal system.
Once inside the host, IJs may overcome or evade the host's immune response (reviewed in Lewis and Clarke, 2012; Shapiro-Ilan et al., 2017, 2018) by shedding of the second-stage-juvenile cuticle (sheath), depositing de-novo produced or host-sequestered immune factors as a camouflage, interfering with the host immune system by secreting putative proteins (S. carpocapsae), and releasing proteases (Steinernema spp.). Both Xenorhabdus and Photorhabdus symbionts play complimentary roles in overcoming the insect defenses and actively suppressing the immune response. They also produce and release several toxins lethal to the insect host as well as antibiotics to prevent secondary infections of the cadaver by other pathogens or scavengers.
Ecology
To locate a potential host, IJs employ various dispersal and foraging behaviors which are a key component in their ecology and their use in biological control (reviewed in Griffin et al., 2005; Lewis and Clarke, 2012; Griffin, 2015; Shapiro-Ilan et al., 2017, 2018). Some species (e.g., S. carpocapsae, S. scapterisci) are sit-and-wait strategists or ambushers that tend to stay near the soil surface where specialized foraging behaviors (nictation, jumping) facilitate infection of mobile hosts. At the other extreme are species (e.g., S. glaseri, H. bacteriophora) that are widely searching foragers or cruisers that distribute themselves actively throughout the soil profile and are well adapted to infecting less mobile hosts. Most species appear to be situated somewhere along a continuum between the extremes. Foraging behaviors may be modulated by various factors. Thus, some species may adopt an ambusher behavior in sandy soils but more actively disperse in more complex substrates (Wilson et al., 2012; Hiltpold and Hibbard, 2018). IJ populations of the ambusher S. carpocapsae contain a low proportion of individuals which actively disperse from the cadaver they emerged from (i.e., “sprinters”) (Bal et al., 2014). Moreover, EPN species have been observed to display group behavior and seem to disperse as packs (Shapiro-Ilan et al., 2014). Active dispersal is generally limited to 90 cm horizontally and vertically within 30 days even for the highly mobile S. glaseri (Schroeder and Beavers, 1987; Kaya, 1990). EPNs are also passively dispersed through phoresy (e.g., on earthworms, isopods) or mobile hosts that take several days to die after initial IJ attachment. Greater distances are covered through movement of substrate or surface water containing IJs.
IJs respond to a variety of cues and signals to navigate through the substrate and find hosts (Shapiro-Ilan et al., 2018). They respond to volatile cues from either their host (Baiocchi et al., 2017) or plants damaged by herbivorous insects and releasing alarm cues in the ground (Rasmann et al., 2005; Ali et al., 2010; Hiltpold et al., 2010; Turlings et al., 2012). Recent contributions to the quickly evolving field highlight the importance of a good understanding of these finely tuned interactions when considering EPNs as a pest management strategy (e.g., Chiriboga et al., 2017; Stelinski et al., 2019). Interestingly, cues emitted by IJs appear to negatively impact the performance of root insect herbivores (Helms et al., 2019) adding dual benefits of using EPNs in pest management strategies. IJs can also differentiate between already infected hosts and non-infected host. A preference to invade already infected hosts is strongest during the initial stages of the infection but eventually reverts to repulsion (Grewal et al., 1996). Invasion of a recently infected host offers the advantages of a weakened immune system and the presence of conspecifics that may be necessary for reproduction. Invasion of advanced infections may be avoided because of food depletion in the cadaver and a high level of conspecific competition.
Factors Affecting Survival and Efficacy
When matching the best EPN species or strain to the target host and environment, innate virulence as well as suitability of environmental conditions have to be considered. The persistence of EPN populations is determined by the longevity of individual IJs and the recycling of populations in host cadavers and numerous factors that can affect both mechanisms (reviewed in Griffin et al., 2005; Lewis and Clarke, 2012; Griffin, 2015; Stuart et al., 2015; Shapiro-Ilan et al., 2017, 2018). IJs of different EPN species differ in their innate longevity between a few months to over 1 year. After soil applications, losses can reach 50% within hours until IJs have settled in the soil. Thereafter, losses may be in the range of 5–10% per day until after 1–6 weeks often only around 1% of the original inoculum survives. To compensate for these losses, the general rule of thumb for application rates is 25 IJs/cm2 of treated area, but some target pests or cropping systems may require higher or (rarely) lower rates. As a result, in soil or similar substrates IJ populations generally remain high enough to provide effective control for 2–8 weeks. Recycling after application often occurs but usually not at a level sufficient to achieve multi-season control because IJ distribution becomes too patchy over time.
Within minutes after application, IJs can be inactivated and killed by UV radiation, but the impact varies among EPN strains and species (Gaugler et al., 1992). IJ loss can be minimized if applications are made early in the morning or in the evening, by adding UV protectants to the IJ suspension, and by making soil applications in high carrier volumes combined with immediate rinsing in with sufficient amounts of water. Most EPN species show optimal performance between 20 and 30°C (Grewal et al., 1994), become sluggish below 10–15°C, and are inactivated above 30–40 °C. Various Steinernema spp. have been isolated from cold regions and other EPN species from hot semi-arid or even arid regions and may hold promise for use in extreme environments.
In soil, IJs move through the water film that coats the interstitial spaces. Moderate substrate moisture levels are essential for good IJ activity. IJ activity is limited in dry conditions, but if moisture removal is gradual, IJs can enter a state of quiescence and persist. Desiccation-intolerant H. bacteriophora IJs will actively seek out soil layers with higher moisture content whereas more desiccation-tolerant S. carpocapsae IJs can survive better in drier conditions. In water-saturated soil, anoxic conditions and low surface tension can be detrimental to movement and even survival of IJs. IJ movement and survival are generally more restricted in fine textured soils than in sandy soils (Portillo-Aguilar et al., 1999) but sandy soils can dry out more quickly which reduces IJ activity. EPNs are negatively affected by pH levels <4 and > 8.
Various biotic factors can also affect the survival of IJs or EPN populations in the soil. Numerous species of arthropods and other invertebrates prey on IJs (e.g., mites, collembolans, tardigrades, predatory nematodes, nematophagous fungi) or scavenge on the EPN-infected hosts while other insect pathogens (e.g., entomopathogenic fungi, bacteria, or viruses) or parasitoids compete with EPNs for hosts (summarized in Shapiro-Ilan et al., 2018). However, competition with other pathogens does not always have negative effects on EPN populations and can in some cases result in synergistic effects on host mortality without significant negative effects on IJ reproduction in the hosts (see section Combinations With Other Control Agents; Shapiro-Ilan et al., 2018).
EPN Use in Different Food Production Systems
This review is restricted to insect groups or species and crops or commodities in which EPNs have been studied more intensely and where at least several field studies have shown good control potential by EPNs (see also Table 2). References were mostly restricted to relevant chapters in the comprehensive books by Grewal et al. (2005), Lacey (2017), and Campos-Herrera (2015). Additional references were listed for important and relevant studies or more specific reviews that were not or only briefly described in these chapters. For additional, relevant chapters that may elaborate on some of the older studies see Bedding et al. (1993) and Gaugler and Kaya (1990).
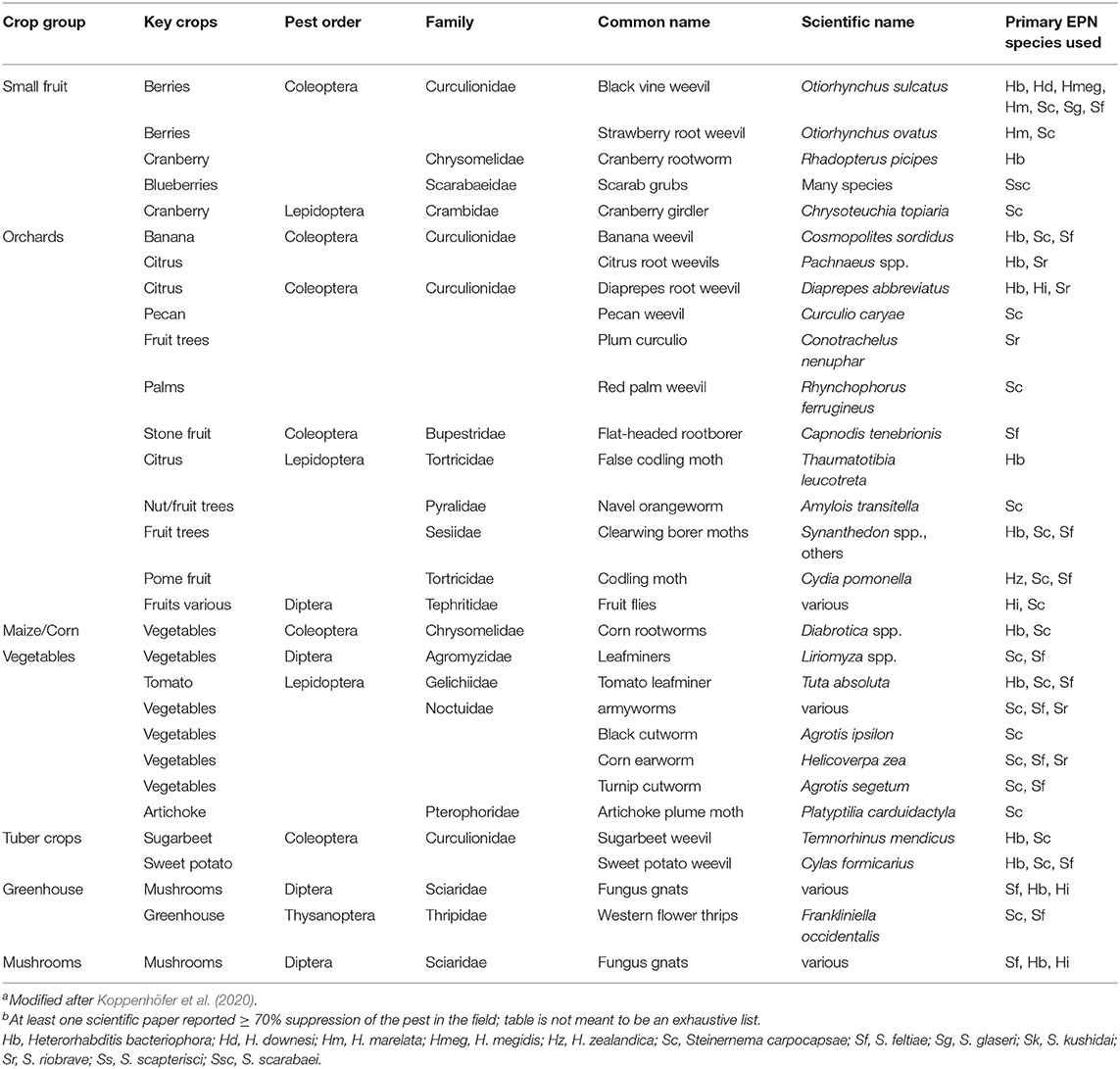
Table 2. Food crops with major target pests on which entomopathogenic nematodes have been used commercially or have shown promisea, b.
Orchards
Research on the use of EPNs against insect pests of orchards has been extensively summarized in Shapiro-Ilan et al. (2005, 2017), Maniania et al. (2017), and Moore and Duncan (2017) (see also references therein). EPNs can be effective against soil dwelling stages of various orchard insect pests. Larvae of the false codling moth, Thaumatotibia leucotreta, an important pest of citrus in South Africa, develop in the fruit but fall to the ground to pupate at a very shallow depth. Single H bacteriophora (10–20 IJs/cm2) applications to the soil reduced fruit infestation by up to 81%, but monthly applications at 5–10 IJs/cm2 provided more consistent control. Larvae of the plum curculio, Conotrachelus nenuphar, a key pest of pome and stone fruit in eastern North America, cause infested fruit to fall to the ground. The mature larvae exit the fruit and burrow in the ground (1–8 cm depth) to pupate. The most effective EPN species to control the stages in the soil has been S. riobrave, consistently providing 80–100% at 0.4–4.0 × 106 IJs/m2 treated area in peach, apple, and cherry orchards across the eastern USA (e.g., Shapiro-Ilan et al., 2013). Larvae of the pecan weevil, Curculio caryae, cause significant damage to pecan nuts in the southeastern USA. After developing inside the nut, mature larvae drop to the ground to burrow to soil depths of 8–25 cm not to emerge as adults until 2–3 years later. One-time applications of S. carpocapsae (2.5–5.0 × 109 IJs/ha) targeting emerging adults provided short-lived and insufficient control (60%). However, three applications of S. carpocapsae (5–10 × 109 IJs/ha) per season over a 2-year-period reduced C. caryae survival by 99% (Shapiro-Ilan and Gardner, 2012). Larvae of the guava weevil, Conotrachelus psidii, a major pest of guava in the Americas, cause infested fruit to drop. The mature larvae exit the fruit and bury into the ground to pupate. Applications of H. bacteriophora provided 79–85% control and low rates of H. indica (1 and 10 IJs/cm2) caused 33–50% mortality. The larvae of various species of fruit flies, that are important pests of a variety of fruit crops around the world, leave the fruit and pupate in the ground. High rates of S. carpocapsae provided 100% control of Mediterranean fruit fly, Ceratitis capitata, prepupae in a papaya field (500 IJs/cm2) and 86% control of oriental fruit fly, Bactrocera dorsalis (300 IJs/cm2) in a guava field. However, H. indica caused 66–93% mortality at much lower rates (1 and 10 IJs/cm2).
EPNs have been particularly well studied and widely used to manage citrus root weevils, especially the Diaprepes root weevil, Diaprepes abbreviatus, an important pest of citrus in Florida, the Caribbean, and California. Larval feeding on the roots, particularly girdling of large roots, causes severe damage. There are continuous and overlapping generations per year. Several EPN species have been commercialized since the 1990 for weevil control, including S. carpocapsae, H. bacteriophora, H. indica, and S. riobrave with the latter being the most effective. In some studies, single applications of S. riobrave provided up to 90% control, and twice-yearly applications through micro-sprinkler irrigation systems at 20–40 IJs/cm2 under the tree canopies have reduced emerging adult weevil populations by about 50% (e.g., Duncan et al., 2007; Bender et al., 2014). S. riobrave products were discontinued in 2011 due to insufficient sales related to the advent of Asian citrus greening disease and the intensive chemical insecticide use for its vector. However, production resumed in 2016 after it was recognized that weevils feeding on the roots is even more damaging to top tree health in presence of the greening disease. Conservation and manipulation of natural EPN populations has been well studied in citrus orchards in Florida (see section Environmental Manipulation; Campos-Herrera et al., 2015a).
Nematodes can also control various pests in cryptic habitats that can be hard to reach with insecticides. Larvae of the peachtree borer, Synanthedon exitiosa, bore into the trunk of stone fruit trees near the soil surface and tunnel toward the roots to feed on crown and roots. S. carpocapsae applied around the tree base (0.3–1.5 × 106 IJs/tree ~ 0.75–3.75 × 108 IJs/ha), preventively during the egg-laying period or curatively to existing infestations, has provided 78–100% control (e.g., Shapiro-Ilan et al., 2015b, 2016a). Lesser peach borer, Synanthedon pictipes, larvae bore into the inner bark and cambium of the trunk and scaffolding limbs of Prunus spp. in the eastern USA. Application of S. carpocapsae (106 IJs/infested tree wound) combined with a diluted formulation of a fire gel (Barricade) to enhance IJ survival provided 70–100% control of S. pictipes (Shapiro-Ilan et al., 2010). Larvae of the banana weevil, Cosmopolites sordidus, cause severe damage to bananas and plantains worldwide by tunneling in the rhizome and the base of the pseudostem. Application of S. carpocapsae with a water thickener into cuts or holes made in residual rhizomes provided 43–68% larval control. Most adult weevils attracted to multiple bait holes cut into residual rhizomes were killed if holes were treated with 2.5 × 105 S. carpocapsae IJs/hole (Treverrow and Bedding, 1993). Larvae of the red palm weevil, Rhynchophorus ferrugineus, a major pest of palms, tunnel in the trunk of various palm species. Application to the top of the palm stipe of a combination of S. carpocapsae (5 × 106 IJs/palm) and chitosan provided 99% control and improved palm survival by 57% (Dembilio et al., 2015).
Larvae overwintering in cryptic habitats may be major sources of reinfestation in the following spring. Larvae of the codling moth, Cydia pomonella, a significant pest of pears, quince, walnuts and especially apples worldwide, overwinter as fifth instars under tree bark, prop piles, or in wooden fruit bins and reinfest orchards in the following year. Applications of S. carpocapsae, S. feltiae, and H. zealandica in orchards (≥ 70% at 106 IJs/tree ~ 109 IJs/ha) (e.g., Lacey et al., 2006; De Waal et al., 2011) and in fruit bins (up to 97% at 10–25 IJs/ml with adjuvant and humectant gel added) have provided effective control of overwintering larvae (e.g., Lacey et al., 2005; De Waal et al., 2010). Larvae of the Navel orangeworm, Amyelois transitella, a major pest of pistachio and almond in California, that overwinter in nuts left after harvest on the tree or ground and reinfest orchards the following season, were controlled (75%) with high-spray-volume (1,870 L water/ha) applications of S. carpocapsae (109 IJs/ha) followed by irrigation (Siegel et al., 2006).
Small Fruit
Research on the use of EPNs against insect pests of small fruit has been extensively summarized in Cowles et al. (2005) and Dara (2017) (see also references therein). Best studied among these pests are root weevil, Otiorhynchus spp., larvae which cause severe damage to the roots of field-grown small fruit and are especially well suited for management with EPNs (Cowles et al., 2005; Dara, 2017). Different Otiorhynchus species have been successfully controlled with various EPN species (e.g., H. bacteriophora, H. marelata, H. megidis, S. carpocapsae, S. feltiae, S. glaseri) in strawberries, cranberries, raspberries, or black currants (e.g., Shanks and Agudelo-Silva, 1990; Booth et al., 2002). In cranberries, effective control with EPNs has also been observed against cranberry girdler, Chrysoteuchia topiaria, larvae (44–92% with S. carpocapsae at 4.9 × 109 IJs/ha) and cranberry rootworm, Rhadopterus picipes, larvae (54–79% with H. bacteriophora at 5 × 109 IJs/ha). In highbush blueberries, S. scarabaei (0.75–1.5 × 109 IJs/ha) provided 86–95% control within 21 days of oriental beetle, Anomala orientalis, larvae but H. bacteriophora was ineffective (Polavarapu et al., 2007).
Maize, Vegetable and Tuber Crops
Research on the use of EPNs against insect pests of corn, vegetable and tuber crops has been extensively summarized in Cabanillas et al. (2005), Gassman and Clifton (2017), Bélair et al. (2005) and Georgis et al. (2006) (see also references therein). The root-feeding larvae of corn rootworms (CRW), Diabrotica spp., are among the most destructive pests of corn. Recent studies in Europe after the introduction of the western CRW have shown the potential of EPNs for the management of the pest (Toepfer et al., 2014 and references therein). In plant scale field experiments, EPNs (2.8–3.5 × 109 IJs/ha) were applied during sowing (April) in a solid stream spray into the soil or as a narrow stream spray onto the soil along rows of young corn plants (June) (Toepfer et al., 2008). Mean reduction in root damage after April and June applications, respectively, were 75% and 72% for H. bacteriophora, 69% and 67% for H. megidis, and 32% and 91% for S. feltiae. In multi-year and -site field scale trials using conventional farm equipment, H. bacteriophora provided control similar to that of soil insecticides (30–80%) (Toepfer et al., 2010). Application as a stream into the soil when sowing may be the most promising approach (Toepfer et al., 2014).
Mature larvae of the corn earworm, Helicoverpa zea, drop to the ground and pupate in the soil at 5–10 cm depth making prepupae and pupae a good target for EPNs. Steinernema riobrave (2 × 109 IJs/ha) provided better control when applied at the time when 50% of the larvae were late instars (still on plant) (100% control) or when 10% of the larvae had dropped to the ground (95% control) than when 40% of the larvae were medium size (40% control). Application via in-furrow irrigation was more effective (95%) than application before (56%) or after (84%) irrigation (Cabanillas and Raulston, 1995). In another study, S. carpocapsae did not control prepupae and pupae while S. riobrave provided 95% control, likely due to its greater tolerance of high soil temperatures (>38°C) in the system (Cabanillas and Raulston, 1996). Larvae of the black cutworm, Agrotis ipsilon, damage corn seedlings by feeding at the base of the plant, often cutting the plant off at the base. Application of S. carpocapsae has reduced seedling damage between 50% at 5.4 × 109 IJs/ha (Capineira et al., 1988) and 76–83% at 1–10 days after treatment with 1.25 × 109 IJs/ha (Levine and Oloumi-Sadeghi, 1992).
Larvae of various cutworm species in different vegetables are amenable to control by EPNs. For example, S. feltiae was as effective as standard insecticides in controlling turnip cutworm, Agrotis segetum, larvae in lettuce fields (2.5 × 109 IJs/ha in sandy soil and at 1010 IJs/ha in loamy soil) (Lössbroek and Theunissen, 1985). Cucumber beetles/rootworms (Diabrotica spp., Acalymma vittatum) damage a variety of cucurbit crops and are susceptible to various EPN species. Thus, S. riobrave decreased survival of A. vittatum larvae in both organic and conventional soil management systems. All larval stages, pupae, and newly emerged adults of the sugar-beet weevil, Temnorhinus mendicus, were susceptible to EPNs in field trials, but the greatest efficacy (90–95% control) was achieved with direct sprays at 2.5 × 109 IJs/ha on the crop at first larval hatch followed by irrigation or rainfall; S. carpocapsae tended to be more effective than H. bacteriophora and both were more effective than standard insecticides (Boselli et al., 1997). EPNs did not provide adequate control or were not competitive with cheaper or more effective insecticides in field tests against several other pests of vegetables and tuber crops including Colorado potato beetle (Leptinotarsa decemlineata), sweet potato weevil (Cylas formicarius), carrot weevil (Listronotus oregonensis), cabbage maggot (Delia radicum), wire worms (Agriotes spp.), and some lepidopteran leaf feeders and dipteran leaf miners.
Greenhouse Production
Research on the use of EPNs against insect pests of greenhouses has been extensively summarized in Tomalak et al. (2005) and Wraight et al. (2017) (see also references therein). The larvae of fungus gnats, Bradysia spp., are among the most important pests of many crops grown in soils with high organic matter content in greenhouses where they have continuous and overlapping generations. While mostly feeding on organic matter and fungi, the larvae can cause serious damage to roots and interfere with callus development and root formation of fresh cuttings for propagation. They also provide routes of entry for soil-borne pathogens. S. feltiae is the only EPN species that is as effective as chemical insecticides giving 80–100% control at 2.5 × 106 IJs/m2 (e.g., Harris et al., 1995; Jagdale et al., 2004). However, at temperature above 26°C, H. indica and H. bacteriophora may be a better choice. The soil-dwelling stages (prepupae, pupae) of another major pest, thrips, in particular western flower thrips, Frankliniella occidentalis, can be controlled with various Heterorhabditis species that cause higher mortality (24–60%) than various Steinernema species (3–54%). The rates required for effective control (around 400 IJs/cm2) were uneconomical (e.g., Ebssa et al., 2004). Nonetheless, high volume foliar applications of S. feltiae combined with adjuvants has been successful enough that the species is now commercially used in Europe and Canada for thrips control (Wraight et al., 2017).
Mushroom Production
Research on the use of EPNs for the control insect pests in mushroom production has been extensively summarized by Jess et al. (2005). Larvae of sciarid flies (Lycoriella and Bradysia spp.) cause damage by feeding on mycelia and the developing sporophors and because their excretions render the substrate unsuitable for mycelial development. Direct application of S. feltiae into or on to casing at casing time has provided control comparable to but more economical than with chemical insecticides (e.g., Scheepmaker et al., 1998). Conversely, larvae of phorid flies which vector fungal pathogens have not been controlled effectively with EPNs.
Cost: Benefit Analysis and Factors Affecting Commercial Success
A detailed evaluation of factors affecting the commercial success of EPNs was conducted by Shapiro et al. (2002) using cotton, turfgrass and citrus as model commodities. The general conclusions drawn from these systems are generally still valid, but may vary slightly among different commodities and pests. Among the factors affecting the success of EPN commercialization the most important are (1) a suitable nematode for the target pest and (2) favorable economics. Other potential factors include EPN ease of use (shelf life, compatibility with typical application technology), relative efficacy of the EPNs compared to other available control tactics, and the reliable provision of a nematode product of high quality.
The suitability of an EPN strain is affected by a number of ecological and biological factors. The IJs have to be able to tolerate environmental extremes that commonly occur in the target system and during the usual application procedures with the typically used equipment (e.g., moisture, temperature, UV exposure). They have to possess the adequate foraging behaviors to allow for effective host location. Finally, the strain needs to have a high level of virulence to the target pest. High persistence in the system may partially compensate for lower virulence. In the end, host suitability has to be proven by high and consistent field performance of the strain.
The economic viability of EPNs in a given commodity is affected by the cost of the EPNs relative to other control options, the value of the commodity that affects the potential profit margin, and the growers' perceived need to control the pest. The relative size of the commodity within the agricultural market affects EPN viability because small niche markets tend to have fewer control options due to registration costs for chemicals (i.e., less competition). One of the advantages of EPNs are generally lower, or lack of, registration costs relative to chemical insecticides due to the recognized safety level of EPNs. For example, in the USA registrations are not required at all.
An excellent example for successful EPN use has been the management of D. abbreviatus in Florida (Shapiro et al., 2002; Dolinski et al., 2012). Over the last decades, several EPN species with high host suitability have been available, the citrus system, particularly in the sandier central ridge region, is highly conducive for EPN activity, application can be concentrated under the trees where the IJs are protected from UV light and which effectively reduces the necessary IJ rate per total orchard hectarage by 3–10×, and chemical insecticides have not been available for soil applications. EPN use in turfgrass in the USA, on the other hand, has been very limited despite some examples of good host suitability and favorable conditions at least in irrigated turfgrass areas (Koppenhöfer et al., 2015; Koppenhöfer and Wu, 2017). Competition from a plethora of available synthetic insecticides has been the major limitation, as these insecticides tend to be cheaper (by a factor of around 10 × if off patent), have greater ease-of-use (long shelf life, less limited by environmental extremes), and are often even more effective.
New Developments to Improve Efficacy
EPNs have to date been utilized only in a relatively small proportion of IPM programs. Wider usage could be achieved through a number of approaches to enhance EPN efficacy including development of improved strains, mass production, formulation and application technology; combination with other management tools and environmental manipulation (Shapiro-Ilan et al., 2017).
Strain Improvement and Stabilization
The most basic method to improve EPNs is discovery of new strains or species that are superior to those already in culture. Old or new strains can be improved through several genetic mechanisms (Gaugler, 1987; Shapiro-Ilan et al., 2017). Traits important to biocontrol such as environmental tolerance, dispersal, and host-finding have been improved through directed selection (Shapiro-Ilan et al., 2017). Attention has to be given to avoid inadvertent selection for one trait (reducing biocontrol potential) while directing selection toward another (trade-offs). Another concern is that relaxation of selection pressure can also lead to reversion. Controlled crosses (hybridization) can be used to improve strains as in a kind of breeding program for superior nematodes. Hybridization and selection methods can be combined (Mukuka et al., 2010). To avoid deterioration of biocontrol traits (e.g., environmental tolerance, virulence, reproductive capacity) during repeated culturing in laboratory or commercial settings (Shapiro-Ilan et al., 2018), strains can be stabilized through cryopreservation or creation of single or multiple purebred homozygous lines that are impervious to trait changes (Bai et al., 2005; Anbesse et al., 2013). The use of selected homozygous lines can lead to improved stabilization as well as enhanced pest control efficacy (Sharifi-Far et al., 2018).
Mass Production
There are three basic approaches for commercial production of EPNs: in vivo production, in vitro solid fermentation and in vitro liquid fermentation (Shapiro-Ilan et al., 2012). Liquid fermentation is considered to have the most efficient economy of scale (and in vivo is considered to have the least); thus most commercial EPN production occurs using liquid fermentation (Shapiro-Ilan et al., 2012). But liquid culture requires the highest level of start-up costs and expertise. The economy of scale and cost projections for solid fermentation lie between in vivo and liquid culture. However, in certain countries lower labor and raw materials expenses allow solid culture to be highly profitable and it is therefore the chosen method. Several approaches can be used to improve in vitro culture including optimization of media and fermentation characteristics (Shapiro-Ilan et al., 2012; Leite et al., 2017).
Commercial level in vivo production uses large quantities of insects. The insects are inoculated with EPNs and bulk harvest is obtained from the infected hosts. G. mellonella or Tenebrio molitor larvae are the most common hosts for in vivo mass production, but other insects can be used. Most in vivo production systems are based on the White trap system, which is the standard for laboratory scale culturing. Thus, in vivo production is generally a two-dimensional system relying on a series of shelves and trays. The size of White traps as well as the quantity can be expanded based on need (Shapiro-Ilan et al., 2012). In vivo production is deemed to be of a low economy of scale due to the expenses related to labor and insect hosts. However, in vivo production can be improved using various approaches (Shapiro-Ilan et al., 2012, 2016b). Host diets can be improved (e.g., content of lipids, proteins or other nutrients) to increase EPN fitness (the nematodes are improved based on superior quality insects). Moreover, in vivo production can be greatly improved through mechanization of the process. Everything from insect host production (Morales-Ramos et al., 2011) to EPN inoculation of hosts, harvesting and packaging IJs can also be mechanized to reduce labor expenses (Shapiro-Ilan et al., 2016b).
Formulation and Application Technology
EPNs application is facilitated by a variety of formulations that provide stability and ease-of-use (Shapiro-Ilan et al., 2017). EPN formulations have included alginate and polyacrylamide gels, activated charcoal, diatomaceous earth, clay, paste, peat, vermiculite, polyurethane sponge, and water dispersible granules (Shapiro-Ilan et al., 2012). Formulations that are user-friendly and can simply be added to the tank and sprayed are most desirable. Vermiculite and diatomaceous earth are currently popular formulations. EPN efficacy can be enhanced through the improvement of formulations. For example, formulations that allow aboveground applications by providing protection against desiccation and UV can expand EPN usage. As mentioned previously, a sprayable gel, Barricade®, can be used aboveground to kill target pests such as the wood boring pest S. pictipes. Other additives that are used to enhance aboveground EPN applications have been studied as well such as chitosan and wood flour foam (some of which may be amenable to organic systems (van Niekerk and Malan, 2015; Shapiro-Ilan et al., 2017).
EPNs can be applied using most standard agricultural equipment including various sprayers or irrigation systems (Shapiro-Ilan et al., 2017). Optimum application depends on a variety of factors including environmental conditions (moisture/humidity and avoiding UV radiation), appropriate application rates, nozzle and sprayer type, etc. EPN efficacy can be enhanced via novel application approaches. For example, relative to traditional applications in aqueous sprays, applying nematodes with phoretic hosts (Shapiro-Ilan and Brown, 2013), in their infected-hosts (Dolinski et al., 2015), or in pre-infected hosts that reach cryptic habitats (Gumus et al., 2015) has resulted in increased efficacy. Certain plant volatiles may be used to enhance EPN efficacy by directing nematode behavior and enhancing infectivity (Willett et al., 2018). Furthermore, other “boosters” that directly increase EPN activity will lead to improved efficacy. For example, infected host macerate or ascaroside-based nematode pheromones can boost EPN infectivity and dispersal and have been shown to improve efficacy against soil pests (Wu et al., 2018; Oliveira-Hofman et al., 2019; Shapiro-Ilan et al., 2019).
Combinations With Other Control Agents
The goal of combining other control agents with EPNs is effective pest control with reduced use of hazardous synthetic insecticides, increased consistency and control levels, and lower costs through reduced rates of EPNs and/or chemicals. Combinations have been particularly well studied in turfgrass (Koppenhöfer and Grewal, 2005; Koppenhöfer et al., 2015; and references therein), but the concept should also work in food commodities. Combination of two EPN species against larvae of A. ipsilon and the annual bluegrass weevil, Listronotus maculicollis or third instars of several white grub species (Koppenhöfer et al., 2015) generally have resulted in additive effects on mortality. Bacillus thuringiensis subsp. japonensis (Btj) combined with EPNs overall resulted in weak synergistic effects against third instars of different white grub species (e.g., Koppenhöfer et al., 1999). H. megidis and S. glaseri showed a strong synergistic interaction with the fungus Metarhizium anisopliae in second- and third-instar Hoplia philanthus when the fungus was applied 3–4 weeks before the EPNs (e.g., Ansari et al., 2006).
In combinations of EPNs with synthetic insecticide, the most consistent synergistic interaction was observed between the neonicotinoid imidacloprid and several EPN species (S. glaseri, H. bacteriophora, H. marelata, H. megidis) in third instars of several white grub species (e.g., Koppenhöfer et al., 2002). However, two rather scarab-specific species, S. kushidai and S. scarabaei, generally did not interact with imidacloprid. Imidacloprid–H. bacteriophora combinations provide more consistent synergism when applied against third instar white grubs but control rates tend to be higher against second instars and early third instars using rates as low as 25 and 50% of the full rates for H. bacteriophora and imidacloprid, respectively (Koppenhöfer and Fuzy, 2008). The anthranilic diamide chlorantraniliprole and H. bacteriophora showed a weak synergistic interaction in the control of third-instar of several white grub species.
Recommendations for the use of EPN combinations can be made only for the better studied combinations, i.e., with Btj, imidacloprid and chlorantraniliprole against white grubs. These combinations should be more effective or equally effective at lower rates when applied against grubs, i.e., young third instars or second instars, and species that are more susceptible to at least one of the control agents.
Combinations of EPNs (H. bacteriophora, S. feltiae, H. megidis) with plant beneficial Pseudomonas spp. (P. chlororaphis, P. protegens) and multiple species of arbuscular mycorrhizal fungi applied to wheat (Imperiali et al., 2017) and corn (Jaffuel et al., 2019) were successfully introduced into the native populations but provided no significant additive or synergistic benefits compared to the individual organisms or organism groups combined.
Environmental Manipulation
EPN efficacy may also be improved through practices that enhance biotic or abiotic factors to make the environment more conducive for their persistence, dispersal or infectivity. IJs could be protected from UV radiation or desiccation by adding ground covers, mulches or crop residues to the cropping system (Campos-Herrera et al., 2015a; Shapiro-Ilan et al., 2015a, 2017). Basic soil parameters such as texture or pH can be optimized. Changes in soil properties due to daily fertigation in an advanced production system approach in citrus orchards in Florida, however, reduced densities of a native (S. diaprepesi) and an exotic applied (S. riobrave) EPN species. The reduction was due to increases in densities a phoretic bacterium, Paenibacillus sp., that limits activity of EPNs and of some nematode-parasitic fungi or nematode trapping fungi (Campos–Herrera et al., 2013).
Conservation and manipulation of natural EPN populations has been well studied in citrus orchards in Florida (Campos-Herrera et al., 2015b) which contain an unusually rich and abundant EPN fauna composed of nine different species. In areas with drier soils, S. diaprepesi, H. indica, and H. zealandica were the dominant species and played an important role in significantly suppressing weevil populations. In areas with poorly drained soils (higher clay and organic matter content), H. indica and S. khuongi dominated but weevils were more abundant. Addition of coarse sand to orchards with the poorly draining soils to imitate the more weevil-suppressive soil and augmentation with the four EPN species resulted in the same weevil-suppressive conditions as observed in orchards that naturally had those conditions (Duncan et al., 2013).
Biotic agents that interact positively with EPNs could also be encouraged within the cropping system. Organisms that synergize EPN efficacy (see above section) might be encouraged within cropping systems through environmental manipulations. Entomopathogenic fungi might be enhanced by improving ground cover or increasing planting density (Jaffuel et al., 2017). Manure or other organic materials could be added to increase earthworm populations and thereby improve EPN dispersal via phoresy (Shapiro-Ilan et al., 2017).
Conclusions and Outlook
Over the last three decades, extensive research efforts have aided the commercialization of EPNs in many commodities with varying degrees of success (3.6). Generally, the most significant factors in this success have been availability of EPN strains suitable for the target pests and systems as well as favorable economics. Compared to other available control tactics, the relative efficacy of the EPNs and their ease of use have also improved. Nonetheless, lower cost and greater ease of use of synthetic insecticides are still limiting EPN use. While many insecticides have lost registration due to pesticide legislation in many countries, new active ingredients from several new insecticide classes that are considered low–risk insecticides (e. g., neonicotinoids, anthranilic diamides) have usually filled the gaps. Nonetheless, public concerns about health risks and environmental hazards of insecticide use persist.
A growing interest in alternatives to synthetic insecticides and in organic agriculture opens opportunities for EPNs, but they will need to be further improved with respect to efficacy (improved application technologies, more virulent strains from field populations or through biotechnology), reduced costs (better production technologies) and greater ease of use (formulations with extended shelf life and tolerance to temperature extremes). Any improvements in those areas will likely be only incremental, yet, could suffice to increase EPN use, at least where pesticide regulations, local ordinances, and public opinion already impinge on synthetic insecticides use.
Although EPNs are considered an excellent tool for pest management in organically produced crops, according to Scopus only seven (of the more than 3,000) papers published on EPNs feature organic crops or systems in the title. Possibly, this is due to the concept that EPNs can be used in organic as well as conventional systems and thus it is not necessary to separate the two. Nonetheless, more research is required to investigate interactions with EPNs that may be specific to organic systems.
Much of previous efforts have concentrated on using EPNs for fast and short-term control of pests following the paradigm of synthetic insecticides. However, the ability of EPNs to persist and recycle in host populations, beckons to be exploited for long term pest suppression. In a few systems this has already been studied through the use of species highly adapted to a narrow range of hosts like S. scapterisci or S. scarabaei (Frank and Walker, 2006; Koppenhöfer and Fuzy, 2009) or through the use of persistent native and but more generalist strains (Shields, 2015; Shields and Testa, 2017). Either approach could be achieved with inoculative releases of the EPNs or periodic augmentative releases, depending on the characteristics of the system into which they would be applied and the biology and ecology of the target pest(s). To maintain the critical qualities of any of the strains used in these approaches, advanced methods are needed such as through creation of homozygous purebred lines or other techniques.
However, short of major breakthroughs in formulation technology that would increase the ease of use of EPNs to a level more comparable with that of synthetic insecticides, significant increases in the use of EPNs will need to be furthered through education and legislations. To open more opportunities for the use of EPNs, major changes in insecticides use pattern will have to be encouraged through legislative incentives, regulations, and restrictions (Bélair et al., 2010).
Author Contributions
AK was lead author and primarily responsible for sections Introduction, EPN use in Different Food Production Systems, and Conclusions and outlook. DS-I was primarily responsible for section New Developments to Improve Efficacy. IH was primarily responsible for section EPN Biology and Ecology. All authors contributed to the article and approved the submitted version.
Funding
Rutgers University paid lead authors salary.
Conflict of Interest
The authors declare that the research was conducted in the absence of any commercial or financial relationships that could be construed as a potential conflict of interest.
References
Adeolu, M., Alnajar, S., Naushad, S., and Gupta, R. S. (2016). Genome-based phylogeny and taxonomy of the 'Enterobacteriales': proposal for Enterobacterales ord. nov. divided into the families Enterobacteriaceae, Erwiniaceae fam. nov., Pectobacteriaceae fam. nov., Yersiniaceae fam. nov., Hafniaceae fam. nov., Morganellaceae fam. nov., and Budviciaceae fam. nov. Int. J. Syst. Evol. Microbiol. 66, 5575–5599. doi: 10.1099/ijsem.0.001485
Ali, J. G., Alborn, H. T., and Stelinski, L. L. (2010). Subterranean herbivore-induced volatiles released by citrus roots upon feeding by Diaprepes abbreviatus recruit entomopathogenic nematodes. J. Chem. Ecol. 36, 361–368. doi: 10.1007/s10886-010-9773-7
Anbesse, S., Sumaya, H. N., Dörfler, V. A., Strauch, O., and Ehlers, R. U. (2013). Stabilization of heat tolerance traits in Heterorhabditis bacteriophora through selective breeding and creation of inbred lines in liquid culture. BioControl 58, 85–93. doi: 10.1007/s10526-012-9467-x
Ansari, M. A., Shah, F. A., Tirry, L., and Moens, M. (2006). Field trials against Hoplia philanthus (Coleoptera: Scarabaeidae) with a combination of an entomopathogenic nematode and the fungus Metarhizium anisopliae CLO 53. Biol. Control 39, 453–459. doi: 10.1016/j.biocontrol.2006.07.004
Bai, C., Shapiro-Ilan, D. I., Gaugler, R., and Hopper, K. R. (2005). Stabilization of beneficial traits in Heterorhabditis bacteriophora through creation of inbred lines. Biol. Control 32, 220–227. doi: 10.1016/j.biocontrol.2004.09.011
Baiocchi, T., Lee, G., Cho, D.-H., and Dillman, A. R. (2017). Host seeking parasitic nematodes use specific odors to assess host resources. Sci. Rep. 7:6270. doi: 10.1038/s41598-017-06620-2
Bal, H. K., Taylor, R. A. J., and Grewal, P. S. (2014). Ambush foraging entomopathogenic nematodes employ sprinting emigrants for long distance dispersal in the absence of hosts. Parasitol. 100, 422–432. doi: 10.1645/12-165.1
Bedding, R., Akhurst, R., and Kaya, H. (1993). Nematodes as the Biological Control of Insect Pests. East Melbourne: CSIRO Publishing. doi: 10.1071/9780643105218
Bélair, G., Koppenhöfer, A. M., Dionne, J., and Simard, L. (2010). Current and potential use of pathogens in the management of turfgrass insects as affected by new pesticide regulations in North America. Intern. J. Pest Manag. 56, 51–60. doi: 10.1080/09670870903076012
Bélair, G., Wright, D. J., and Curto, G. (2005). “Vegetable and tuber crop applications”, in Nematodes as Biocontrol Agents, eds P. S. Grewal, R. -U. Ehlers, and D. I. Shapiro-Ilan (Wallingford: CABI Publishing), 231–254.
Bender, G. S., Bates, L. M., Bethke, J. A., Lewis, E., Tanizaki, G., Morse, J. G., et al. (2014). Evaluation of insecticides, entomopathogenic nematodes, and physical barriers for control of Diaprepes abbreviatus (Coleoptera: Curculionidae) in citrus. J. Econ. Entomol. 107, 2137–2146. doi: 10.1603/EC14150
Booth, S. R., Tanigoshi, L. K., and Shanks, C. H. Jr. (2002). Evaluation of entomopathogenic nematodes to manage root weevil larvae in Washington State cranberry, strawberry, and red raspberry. Environ. Entomol. 31, 895–902. doi: 10.1603/0046-225X-31.5.895
Boselli, M., Curto, G. M., and Tacconi, R. (1997). Field efficacy of entomopathogenic nematodes against the sugar-beet weevil Temnorhinus (=Conorrhynchus) mendicus Gyll. (Coleoptera: Curculionidae). Biocontrol Sci. Technol. 7, 231–238. doi: 10.1080/09583159730929
Burnell, A. M., and Stock, S. P. (2000). Heterorhabditis, Steinernema and their bacterial symbionts - lethal pathogens of insects. Nematology 2, 31–42. doi: 10.1163/156854100508872
Cabanillas, H. E., and Raulston, J. R. (1995). Impact of Steinernema riobravis (Rhabditida: Steinernematidae) on the control of Helicoverpa zea (Lepidoptera: Noctuidae) in corn. J. Econ. Entomol. 88, 58–64. doi: 10.1093/jee/88.1.58
Cabanillas, H. E., and Raulston, J. R. (1996). Evaluation of Steinernema riobravis, S. carpocapsae, and irrigation timing for the control of corn earworm, Helicoverpa zea. J. Nematol. 28, 75–82.
Cabanillas, H. E., Wright, R. J., and Vyas, R. V. (2005). “Cereal, fibre, oilseed and medicinal crop applications”, in Nematodes as Biocontrol Agents, eds P. S. Grewal, R. -U. Ehlers, and D. I. Shapiro-Ilan (Wallingford: CABI Publishing), 265–279. doi: 10.1079/9780851990170.0265
Campos-Herrera, R. (2015). Nematode Pathogenesis of Insect and Other Pests. Cham: Springer International Publishing. doi: 10.1007/978-3-319-18266-7
Campos-Herrera, R., El-Borai, F. E., and Duncan, L. W. (2015a). Modifying soil to enhance biological control of belowground dwelling insects in citrus groves under organic agriculture in Florida. Biol. Control 84, 53–63. doi: 10.1016/j.biocontrol.2015.02.002
Campos-Herrera, R., El-Borai, F. E., and Duncan, L. W. (2015b). “It takes a village: entomopathogenic nematode community structure and conservation biological control in Florida (U.S.) orchards,” in Nematode Pathogenesis of Insect and Other Pests, ed R. Campos-Herrera (Cham: Springer International Publishing), 329–351. doi: 10.1007/978-3-319-18266-7_13
Campos–Herrera, R., Pathak, E., El–Borai, F. E., Schumann, A., Abd–Elgawad, M. M. M., and Duncan, L. W. (2013). New citriculture system suppresses native and augmented entomopathogenic nematodes. Biol. Control 66, 183–194. doi: 10.1016/j.biocontrol.2013.05.009
Capineira, J. L., Pelissier, L. D., Menout, G. S., and Epsky, N. D. (1988). Control of black cutworm, Agrotis ipsilon, with entomogenous nematodes (Nematoda: Steinernematidae, Heterorhabditidae). J. Invertebr. Pathol. 52, 427–435. doi: 10.1016/0022-2011(88)90055-9
Chiriboga, X., Campos-Herrera, R., Jaffuel, G., Röder, G., and Turlings, T. C. J. (2017). Diffusion of the maize root signal (E)-β-caryophyllene in soils of different textures and the effects on the migration of the entomopathogenic nematode Heterorhabditis megidis. Rhizosphere 3, 53–59. doi: 10.1016/j.rhisph.2016.12.006
Cowles, R. S., Polavarapu, S., Williams, R. N., Thies, A., and Ehlers, R.-U. (2005). “Soft fruit applications,” in Nematodes as Biocontrol Agents, eds P. S. Grewal, R.-U. Ehlers, and D. I. Shapiro-Ilan (Wallingford: CABI Publishing), 191–213.
Dara, S. K. (2017), “Microbial control of arthropod pests in small fruit and vegetables in temperate climate,” in Microbial Control of Insect and Mite Pests, ed L. A. Lacey (Amsterdam: Elsevier), 209–221. doi: 10.1016/B978-0-12-803527-6.00014-7.
De Waal, J. Y., Malan, A. P., and Addison, M. F. (2011). Efficacy of entomopathogenic nematodes (Rhabditida: Heterorhabditidae and Steinernematidae) against codling moth, Cydia pomonella (Lepidoptera: Tortricidae) in temperate regions. Biocontrol Sci. Technol. 21, 489–502. doi: 10.1080/09583157.2011.607922
De Waal, J. Y., Malan, A. P., Levings, J., and Addison, M. F. (2010). Key elements in the successful control of diapausing codling moth, Cydia pomonella (Lepidoptera: Tortricidae) in wooden fruit bins with as South African isolate of Heterorhabditis zealandica (Rhabditida: Heterorhabditidae). Biocontrol Sci. Technol. 20, 1161–1176. doi: 10.1080/09583151003599708
Dembilio, ó., Llácer, E., del Mar Martínez de Altube, M., and Jacas, J. A. (2015). Field efficacy of imidacloprid and Steinernema carpocapsae in a chitosan formulation against the red palm weevil Rhynchophorus ferrugineus (Coleoptera: Curculionidae) in Phoenix canariensis. Pest Manag. Sci. 66, 365–370. doi: 10.1002/ps.1882
Dillman, A. R., Chaston, J. M., Adams, B. J., Ciche, T. A., Goodrich-Blair, H., Stock, S. P., et al. (2012). An entomopathogenic nematode by any other name. PLoS Pathogens 8:e1002527. doi: 10.1371/journal.ppat.1002527
Dolinski, C., Choo, H. Y., and Duncan, L. W. (2012). Grower acceptance of entomopathogenic nematodes: Case studies on three continents. J. Nematol. 44, 226–235.
Dolinski, C., Shapiro-Ilan, D. I., and Lewis, E. E. (2015). “Insect cadaver applications: pros and cons,” in Nematode Pathogenesis of Insect and Other Pests, ed R. Campos-Herrera (Cham: Springer International Publishing), 207–230. doi: 10.1007/978-3-319-18266-7_8
Duncan, L. W., Graham, J. H., Zellers, J., Bright, D., Dunn, D. C., and El-Borai, F. E. (2007). Food web responses to augmentation biological control using entomopathogenic nematodes in bare and composted-manure amended soil. J. Nematol. 39, 176–189.
Duncan, L. W., Stuart, R. J., El-Borai, F. E., Campos-Herrera, R., Pathak, E., Giurcanu, M., et al. (2013). Modifying orchard planting sites conserves entomopathogenic nematodes, reduces weevil herbivory and increases citrus tree growth, survival and fruit yield. Biol. Control 64, 26–36. doi: 10.1016/j.biocontrol.2012.09.006
Ebssa, L., Borgemeister, C., and Poehling, H. M. (2004). Effectiveness of different species/strains of entomopathogenic nematodes for control of western flower thrips (Frankliniella occidentalis) at various concentrations, host densities and temperatures. Biol. Control 29, 145–154. doi: 10.1016/S1049-9644(03)00132-4
Ehlers, R.-U. (2005). “Forum on safety and regulation,” in Nematodes as Biocontrol Agents, eds P. S. Grewal, R. -U. Ehlers, and D. I. Shapiro-Ilan (Wallingford: CABI Publishing), 107–114. doi: 10.1079/9780851990170.0107
Frank, J. H., and Walker, T. J. (2006). Permanent control of pest mole crickets (Orthoptera: Gryllotalpidae) in Florida. Am. Entomol. 52, 139–144. doi: 10.1093/ae/52.3.138
Gassman, A. J., and Clifton, E. H. (2017). “Current and potential applications of biopesticides to manage insect pests of maize,” in Microbial Control of Insect and Mite Pests, ed L. A. Lacey (Amsterdam: Elsevier), 173–184. doi: 10.1016/B978-0-12-803527-6.00011-1
Gaugler, R. (1987). “Entomogenous nematodes and their prospects for genetic improvement,” in Biotechnology in Invertebrate Pathology and Cell Culture ed K. Maramorosch (San Diego, CA: Academic Press), 457–484. doi: 10.1016/B978-0-12-470255-4.50033-X
Gaugler, R. (2002). Entomopathogenic Nematology. Wallingford, UK: CABI Publishing. doi: 10.1079/9780851995670.0000
Gaugler, R., Bednarek, A., and Campbell, J. F. (1992). Ultraviolet inactivation of Heterorhabditid and Steinernematid nematodes. J. Invertebr. Pathol. 59, 155–160. doi: 10.1016/0022-2011(92)90026-Z
Gaugler, R., and Kaya, H. K. (1990). Entomopathogenic Nematodes in Biological Control. Boca Raton, FL, USA: CRC Press.
Georgis, R., Koppenhöfer, A. M., Lacey, L. A., Belair, G., Duncan, L. W., Grewal, P. S., et al. (2006). Successes and failures of entomopathogenic nematodes. Biol. Control 38, 103–123. doi: 10.1016/j.biocontrol.2005.11.005
Grewal, P. S., Ehlers, R.-U., and Shapiro-Ilan, D. I. (2005). Nematodes as Biocontrol Agents. Wallingford: CABI Publishing. doi: 10.1079/9780851990170.0000
Grewal, P. S., Lewis, E. E., and Gaugler, R. (1996). Response of infective juvenile stage parasites (Rhabditida: Steinernematidae) to volatile cues from infected hosts. J. Chem. Ecol. 23, 503–515. doi: 10.1023/B:JOEC.0000006374.95624.7e
Grewal, P. S., Selvan, S., and Gaugler, R. (1994). Thermal adaptation of entomopathogenic nematodes-niche breadth for infection, establishment and reproduction. J. Therm. Biol. 19, 45–253. doi: 10.1016/0306-4565(94)90047-7
Griffin, C. T. (2015). “Behaviour and population dynamics of entomopathogenic nematodes following application,” in Nematode Pathogenesis of Insect and Other Pests, ed R. Campos-Herrera (Cham: Springer International Publishing), 57–95. doi: 10.1007/978-3-319-18266-7_3
Griffin, C. T., Boemare, N. E., and Lewis, E. E. (2005). “Biology and behavior,” in Nematodes as Biocontrol Agents, eds P. S. Grewal, R. -U. Ehlers and D. I. Shapiro-Ilan (Wallingford: CABI Publishing), 47–64. doi: 10.1079/9780851990170.0047
Gumus, A., Karagoz, M., Shapiro-Ilan, D. I., and Hazir, S. (2015). A novel approach to biocontrol: release of live insect hosts pre-infected with entomopathogenic nematodes. J. Invertebr. Pathol. 130, 56–60. doi: 10.1016/j.jip.2015.07.002
Harris, M. A., Oetting, R. D., and Gardner, W. A. (1995). Use of entomopathogenic nematodes and a new monitoring technique for control of fungus gnats, Bradysia coprophila (Diptera: Sciaridae), in floriculture. Biol. Control 5, 412–418. doi: 10.1006/bcon.1995.1049
Helms, A. M., Ray, S., Matulis, N. L., Kuzemchak, M. C., Grisales, W., Tooker, J. F., et al. (2019). Chemical cues linked to risk: Cues from below-ground natural enemies enhance plant defences and influence herbivore behaviour and performance. Funct. Ecol. doi: 10.1111/1365-2435.13297
Hiltpold, I., and Hibbard, B. E. (2018). Indirect root defenses cause induced fitness costs in Bt-resistant western corn rootworm. J. Econ. Entomol. 111, 2349–2358. doi: 10.1093/jee/toy220
Hiltpold, I., Toepfer, S., Kuhlmann, U., and Turlings, T. C. J. (2010). How maize root volatiles affect the efficacy of entomopathogenic nematodes in controlling the western corn rootworm? Chemoecology 20, 155–162. doi: 10.1007/s00049-009-0034-6
Imperiali, N., Chiriboga, M. X., Schlaeppi, K., Fesselet, M., Villacrés, D., Jaffuel, G., et al. (2017). Combined field inoculations of Pseudomonas bacteria, arbuscular mycorrhizal fungi and entomopathogenic nematodes and their effects on wheat performance. Front. Plant Sci. 8:1809. doi: 10.3389/fpls.2017.01809
Jaffuel, G., Blanco-Pérez, R., Büchi, L., Mäder, P., Fliessbach, A., Charles, R., et al. (2017). Effects of cover crops on the overwintering success of entomopathogenic nematodes and their antagonists. Appl. Soil Ecol. 114, 62–73. doi: 10.1016/j.apsoil.2017.02.006
Jaffuel, G., Imperiali, N., Shelby, K., Campos-Herrera, R., Geisert, R., Maurhofer, M., et al. (2019). Protecting maize from rootworm damage with the combined application of arbuscular mycorrhizal fungi, Pseudomonas bacteria and entomopathogenic nematodes. Sci. Reports 9, 1–12. doi: 10.1038/s41598-019-39753-7
Jagdale, G. B., Casey, M. L., Grewal, P. S., and Lindquist, R. K. (2004). Effect of application rate and timing, potting medium, and host plant on efficacy of Steinernema feltiae against fungus gnat, Bradysia coprophila, in floriculture. Biol. Control 29, 296–305. doi: 10.1016/S1049-9644(03)00164-6
Jess, S., Schweizer, H., and Kilpatrick, M. (2005). “Mushroom applications,” in Nematodes as Biocontrol Agents, eds P. S. Grewal, R. -U. Ehlers and D. I. Shapiro-Ilan (Wallingford: CABI Publishing), 191–213. doi: 10.1079/9780851990170.0191
Kaya, H. K. (1990). “Soil ecology,” in Entomopathogenic Nematodes in Biological Control, eds R. Gaugler and H. K. Kaya (Boca Raton, FL: CRC Press), 93–115.
Koppenhöfer, A. M., Choo, H. Y., Kaya, H. K., Lee, D. W., and Gelernter, W. D. (1999). Increased field and greenhouse efficacy with a combination of entomopathogenic nematode and Bacillus thuringiensis against scarab grubs. Biol. Control 14, 37–44. doi: 10.1006/bcon.1998.0663
Koppenhöfer, A. M., Cowles, R. S., Cowles, E. A., Fuzy, E. M., and Baumgartner, L. (2002). Comparison of neonicotinoid insecticides as synergists for entomopathogenic nematodes. Biol. Control 24, 90–97. doi: 10.1016/S1049-9644(02)00008-7
Koppenhöfer, A. M., and Fuzy, E. M. (2008). Early timing and new combinations to increase the efficacy of neonicotinoid–entomopathogenic nematodes (Rhabditida: Heterorhabditidae) combinations against white grubs (Coleoptera: Scarabaeidae). Pest Manag. Sci. 64, 725–735. doi: 10.1002/ps.1550
Koppenhöfer, A. M., and Fuzy, E. M. (2009). Long-term effects and persistence of Steinernema scarabaei applied for suppression of Anomala orientalis (Coleoptera: Scarabaeidae). Biol. Control 48, 63–72. doi: 10.1016/j.biocontrol.2008.09.005
Koppenhöfer, A. M., and Grewal, P. S. (2005). “Compatibility and interactions with agrochemicals and other biocontrol agents,” in Nematodes as Biocontrol Agents, eds P. S. Grewal, R. -U. Ehlers and D. I. Shapiro-Ilan (Wallingford: CABI Publishing), 363–381. doi: 10.1079/9780851990170.0363
Koppenhöfer, A. M., Kostromytska, O. S., McGraw, B. A., and Ebssa, L. (2015). “Entomopathogenic nematodes in turfgrass: ecology and management of important insect pests in North America,” in Nematode Pathogenesis of Insect and Other Pests, ed R. Campos-Herrera (Cham: Springer International Publishing), 309–327. doi: 10.1007/978-3-319-18266-7_12
Koppenhöfer, A. M., Shapiro-Ilan, D. I., and Hiltpold, I. (2020). “Advances in the use of entomopathogenic nematode biopesticides in suppressing crop insect pests,” in Biopesticides for Sustainable Agriculture, eds N. Birch and T. Glare (Cambridge: Burleigh Dodds Science Publishing), 1–38. doi: 10.19103/AS.2020.0073.10
Koppenhöfer, A. M., and Wu, S. (2017). “Microbial control of insect pests of turfgrass,” in Microbial Control of Insect and Mite Pest: From Theory to Practice, ed L. A. Lacey (Amsterdam: Elsevier), 331–341. doi: 10.1016/B978-0-12-803527-6.00022-6
Lacey, L. A. (2017). Microbial Control of Insect and Mite Pests: From Theory to Practice. Amsterdam: Elsevier.
Lacey, L. A., Arthurs, S. P., Unruh, T. R., Headrick, H. L., and Fritts, R. Jr. (2006). Entomopathogenic nematodes for the control of codling moth (Lepidoptera: Tortricidae) in apple and pear orchards: effect of nematode species and seasonal temperatures, adjuvants, application equipment and post-application irrigation. Biol. Control 37, 214–223. doi: 10.1016/j.biocontrol.2005.09.015
Lacey, L. A., Neven, L. G., Headrick, H. L., and Fritts, R. Jr. (2005). Factors affecting entomopathogenic nematodes (Steinernematidae) for the control of overwintering codling moth (Lepidoptera: Tortricidae) in fruit bins. J. Econ. Entomol. 98, 1863–1869. doi: 10.1093/jee/98.6.1863
Leite, L. G., Shapiro-Ilan, D. I., Hazir, S., and Jackson, M. A. (2017). Effect of inoculum age and physical parameters on in vitro culture of the entomopathogenic nematode Steinernema feltiae. J. Helminthol. 91, 686–695. doi: 10.1017/S0022149X16000821
Levine, E., and Oloumi-Sadeghi, H. (1992). Field evaluation of Steinernema carpocapsae (Rhabditida: Steinernematidae) against the black cutworm in field corn. J. Entomol. Sci. 27, 427–435. doi: 10.18474/0749-8004-27.4.427
Lewis, E. E., and Clarke, D. J. (2012). “Nematode parasites and entomopathogens,” in Insect Pathology, eds E. F. Vega and H. K. Kaya (Amsterdam: Elsevier), 395–424. doi: 10.1016/B978-0-12-384984-7.00011-7
Lössbroek, T. G., and Theunissen, J. (1985). The entomogenous nematode Neoaplectana bibionis as a biological control agent of Agrotis segetum in lettuce. Entomol. Exp. Appl. 39, 261–264. doi: 10.1111/j.1570-7458.1985.tb00468.x
Lu, D., Macchietto, M., Chang, D., Barros, M. M., Baldwin, J., Mortazavi, A., et al. (2017). Activated entomopathogenic nematode infective juveniles release lethal venom proteins. PLoS Pathogen 13:e1006302. doi: 10.1371/journal.ppat.1006302
Maniania, N. K., Ekesi, S., and Dolinski, C. (2017). “Entomopathogens routinely used in pest control strategies: orchards in tropical climate,” in Microbial Control of Insect and Mite Pests, ed L. A. Lacey (Amsterdam: Elsevier), 269–282. doi: 10.1016/B978-0-12-803527-6.00018-4
Moore, S. D., and Duncan, L. W. (2017). “Microbial control of insect and mite pests of citrus,” in Microbial Control of Insect and Mite Pests, ed L. A. Lacey (Amsterdam: Elsevier), 283–298. doi: 10.1016/B978-0-12-803527-6.00019-6
Morales-Ramos, J. A., Rojas, M. G., Shapiro-Ilan, D. I., and Tedders, W. L. (2011). Automated Insect Separation System, US Patent 8,025,027 B1.
Mukuka, J., Strauch, O., and Ehlers, R.-U. (2010). Improvement of heat and desiccation tolerance in Heterorhabditis bacteriophora through cross–breeding of tolerant strains and successive genetic selection. BioControl 55, 511–521. doi: 10.1007/s10526-010-9271-4
Oliveira-Hofman, C., Kaplan, F., Stevens, G., Lewis, E., Wu, S., Alborn, H. T., et al. (2019). Pheromone extracts act as boosters for entomopathogenic nematodes efficacy. J. Invertebr. Pathol. 164, 38–42. doi: 10.1016/j.jip.2019.04.008
Peters, A. (1996). The natural host range of Steinernema and Heterorhabditis spp. and their impact on insect populations. Biocontrol Sci. Technol. 6, 389–402. doi: 10.1080/09583159631361
Piedra Buena, A., López–Cepero, J., and Campos–Herrera, R. (2015). “Entomopathogenic nematode production and application: regulation, ecological impact and non–target effects,” in Nematode Pathogenesis of Insects and Other Pests - Ecology and Applied Technologies for Sustainable Plant and Crop Protection. Series: Sustainability in Plant and Crop Protection., A. Ciancio (series Ed.). Vol. 1, ed R. Campos-Herrera (Switzerland: Springer International Publishing), 253–280. doi: 10.1007/978-3-319-18266-7_10
Polavarapu, S., Koppenhöfer, A. M., Barry, J. D., Holdcraft, R. J., and Fuzy, E. M. (2007). Entomopathogenic nematodes and neonicotinoids for remedial control of oriental beetle, Anomala orientalis (Coleoptera: Scarabaeidae), in highbush blueberry. Crop Protection 26, 1266–1271. doi: 10.1016/j.cropro.2006.10.026
Portillo-Aguilar, C., Villani, M. G., Tauber, M. J., Tauber, C. A., and Nyrop, J. P. (1999). Entomopathogenic nematode (Rhabditida: Heterorhabditidae and Steinernematidae) response to soil texture and bulk density. Environ. Entomol. 28, 1021–1035. doi: 10.1093/ee/28.6.1021
Rasmann, S., Köllner, T. G., Degenhardt, J., Hiltpold, I., Toepfer, S., Kuhlmann, U., et al. (2005). Recruitment of entomopathogenic nematodes by insect-damaged maize roots. Nature 434, 732–737. doi: 10.1038/nature03451
Scheepmaker, J. W. A., Geels, F. P., Smits, P. H., and van Griensven, L. G. L. D. (1998). Influence of Steinernema feltiae and diflubenzuron on yield and economics of the cultivated mushroom Agaricus bisporus in Dutch mushroom culture. Biocontrol Sci. Technol. 8, 269–275. doi: 10.1080/09583159830333
Schroeder, W. J., and Beavers, J. B. (1987). Movement of the entomogenous nematodes of the families Heterorhabditidae and Steinernematidae in soil. J. Nematol. 19, 257–259.
Shanks, C. H. Jr., and Agudelo-Silva, F. (1990). Field pathogenicity and persistence of heterorhabditid and steinernematid nematodes (Nematoda) infecting black vine weevil larvae (Coleoptera: Curculionidae) in cranberry bogs. J. Econ. Entomol. 83, 107–110. doi: 10.1093/jee/83.1.107
Shapiro, D. I., Gouge, D., and Koppenhöfer, A. M. (2002). “Factors affecting field efficacy: analysis of case studies in cotton, turf, and citrus,” in Entomopathogenic Nematology, ed R. Gaugler (Wallingford: CABI Publishing), 333–356.
Shapiro-Ilan, D., Arthurs, S. P., and Lacey, L. A. (2017). “Microbial control of arthropod pests of orchards in temperate climates,” in Microbial Control of Insect and Mite Pests, ed L. A. Lacey (Amsterdam: Elsevier), 253–267. doi: 10.1016/B978-0-12-803527-6.00017-2
Shapiro-Ilan, D. I., and Brown, I. (2013). Earthworms as phoretic hosts for Steinernema carpocapsae and Beauveria bassiana: implications for enhanced biological control. Biol. Control 66, 41–48. doi: 10.1016/j.biocontrol.2013.03.005
Shapiro-Ilan, D. I., Cottrell, T. E., Mizell, R. F., Horton, D. L., Behle, R. W., and Dunlap, C. A. (2010). Efficacy of Steinernema carpocapsae for control of the lesser peachtree borer, Synanthedon pictipes: improved aboveground suppression with a novel gel application. Biol. Control 54, 23–28. doi: 10.1016/j.biocontrol.2009.11.009
Shapiro-Ilan, D. I., Cottrell, T. E., Mizell, R. F. III., and Horton, D. L. (2015b). Field suppression of the peachtree borer, Synanthedon exitiosa, using Steinernema carpocapsae: effects of irrigation, a sprayable gel and application method. Biol. Control. 82, 7–12. doi: 10.1016/j.biocontrol.2014.12.003
Shapiro-Ilan, D. I., Cottrell, T. E., Mizell, R. F. III., and Horton, D. L. (2016a). Curative control of the peachtree borer using entomopathogenic nematodes. J. Nematol. 48, 170–176. doi: 10.21307/jofnem-2017-024
Shapiro-Ilan, D. I., Duncan, L. W., Lacey, L. A., and Han, R. (2005). “Orchard applications,” in Nematodes as Biocontrol Agents, ed P. S. Grewal, R. -U. Ehlers and D. I. Shapiro-Ilan (Wallingford: CABI Publishing), 215–229. doi: 10.1079/9780851990170.0215
Shapiro-Ilan, D. I., and Gardner, W. A. (2012). Improved control of Curuclio caryae (Coleoptera: Curculionidae) through multi-stage pre-emergence applications of Steinernema carpocapsae. J. Entomol. Sci. 47, 27–34. doi: 10.18474/0749-8004-47.1.27
Shapiro-Ilan, D. I., Han, R., and Dolinski, C. (2012). Entomopathogenic nematode production and application technology. J. Nematol. 44, 206–217.
Shapiro-Ilan, D. I., Hazir, S., and Leite, L. (2015a). Viability and virulence of entomopathogenic nematodes exposed to ultraviolet radiation. J. Nematol. 47, 184–189.
Shapiro-Ilan, D. I., Hiltpold, I., and Lewis, E. E. (2018). “Ecology of invertebrate pathogens: nematodes,” in Ecology of Invertebrate Diseases, ed A. E. Hajek and D. I. Shapiro-Ilan (Hoboken, NJ: John Wiley & Sons, Ltd.), 415–440. doi: 10.1002/9781119256106.ch11
Shapiro-Ilan, D. I., Kaplan, F., Oliveira-Hofman, C., Schliekelman, P., Alborn, H. T., and Lewis, E. E. (2019). Conspecific pheromone extracts enhance entomopathogenic infectivity. J. Nematol. 51, e2019–e2082. doi: 10.21307/jofnem-2019-082
Shapiro-Ilan, D. I., Lewis, E. E., and Schliekelman, P. (2014). Aggregative group behavior in insect parasitic nematode dispersal. Int. J. Parasitol. 44, 49–54. doi: 10.1016/j.ijpara.2013.10.002
Shapiro-Ilan, D. I., Morales-Ramos, J. A., and Rojas, M. G. (2016b). “In vivo production of entomopathogenic nematodes,” in Microbial-Based Biopesticides – Methods and Protocols, eds T. Glare and M. Moran-Diez (New York, NY: Human Press), 137–158. doi: 10.1007/978-1-4939-6367-6_11
Shapiro-Ilan, D. I., Wright, S. E., Tuttle, A. F., Cooley, D. R., and Leskey, T. C. (2013). Using entomopathogenic nematodes for biological control of plum curculio, Conotrachelus nenuphar: effects of irrigation and species in apple orchards. Biol. Control 67, 123–129. doi: 10.1016/j.biocontrol.2013.07.020
Sharifi-Far, S., Shapiro-Ilan, D. I., Brownbridge, M., and Hallett, R. H. (2018). The combined approach of strain discovery and the inbred line technique for improving control of Delia radicum with Heterorhabditis bacteriophora. Biol. Control 118, 37–43. doi: 10.1016/j.biocontrol.2017.12.004
Shields, E. J. (2015). “Utilizing persistent entomopathogenic nematodes in a more classical biological control approach,” in Nematode Pathogenesis of Insect and Other Pests, R. Campos-Herrera (Cham: Springer International Publishing), 165–184. doi: 10.1007/978-3-319-18266-7_6
Shields, E. J., and Testa, A. M. (2017). Biological control of alfalfa snout beetle with persistent entomopathogenic nematodes: Expanding a single farm's success to an area-wide biological control program. Am. Entomol. 63, 216–223. doi: 10.1093/ae/tmx046
Siegel, J., Lacey, L. A., Higbee, B. S., Noble, P., and Fritts, R. Jr. (2006). Effect of application rates and abiotic factors on Steinernema carpocapsae for control of overwintering navel orangeworm (Lepidoptera: Pyralidae, Amyelois transitella) in fallen pistachios. Biol. Control 36, 324–330. doi: 10.1016/j.biocontrol.2005.09.012
Stelinski, L. L., Willett, D., Rivera, M. J., and Ali, J. G. (2019). ‘Tuning' communication among four trophic levels of the root biome to facilitate biological control. Biol. Control 131, 49–53. doi: 10.1016/j.biocontrol.2019.01.006
Stock, S. P. (2015). “Diversity, biology and evolutionary relationships,” in Nematode Pathogenesis of Insect and Other Pests, ed R. Campos-Herrera (Switzerland: Springer International: Publishing), 3–27. doi: 10.1007/978-3-319-18266-7_1
Stuart, R. J., Barbercheck, M. E., and Grewal, P. S. (2015). “Entomopathogenic nematode in the soil environment: distributions, interactions and the influence of biotic and abiotic factors,” in Nematode Pathogenesis of Insect and Other Pests, ed R. Campos-Herrera (Cham: Springer International Publishing), 97–137. doi: 10.1007/978-3-319-18266-7_4
Toepfer, S., Hatala-Zseller, I., Ehlers, R. U., Peters, A., and Kuhlmann, U. (2010). The effect of application techniques on field-scale efficacy: can the use of entomopathogenic nematodes reduce damage by western corn rootworm larvae? Agric. Forest Entom. 12, 389–402. doi: 10.1111/j.1461-9563.2010.00487.x
Toepfer, S., Peters, A., Ehlers, R. U., and Kuhlmann, U. (2008). Comparative assessment of the efficacy of entomopathogenic nematode species at reducing western corn rootworm larvae and root damage in maize. J. Appl. Entom. 132, 337–348. doi: 10.1111/j.1439-0418.2008.01274.x
Toepfer, S., Knuth, P., Glas, M., and Kuhlmann, U. (2014). Successful application of entomopathogenic nematodes for the biological control of western corn rootworm larvae in Europe – a min review. Julius-Kühn-Archiv. 444, 59–66. doi: 10.5073/jka.2014.444.019
Tomalak, M., Piggott, S., and Jagdale, G. B. (2005). “Glasshouse applications,” in Nematodes as Biocontrol Agents, ed P. S. Grewal, R. -U. Ehlers and D. I. Shapiro-Ilan (Wallingford: CABI Publishing), 147–166. doi: 10.1079/9780851990170.0147
Torres-Barragan, A., Suazo, A., Buhler, W. G., and Cardoza, Y. J. (2011). Studies on the entomopathogenicity and bacterial associates of the nematode Oscheius carolinensis. Biol. Control 59, 123–129. doi: 10.1016/j.biocontrol.2011.05.020
Treverrow, N., and Bedding, R. A. (1993). “Development of a system for the control of the banana weevil borer, Cosmopolites sordidus, with entomopathogenic nematodes”, in Nematodes and the Biological Control of Insect Pests, eds R. A. Bedding, R. Akhurst and H. K. Kaya (Melbourne: CSIRO Publishing), 41–47.
Turlings, T. C. J., Hiltpold, I., and Rasmann, S. (2012). The importance of root-produced volatiles as foraging cues for entomopathogenic nematodes. Plant Soil 359, 51–60. doi: 10.1007/s11104-012-1295-3
van Niekerk, S., and Malan, A. P. (2015). Adjuvants to improve aerial control of the citrus mealybug Planococcus citri (Hemiptera: Pseudococcidae) using entomopathogenic nematodes. J. Helminthol. 89, 189–195. doi: 10.1017/S0022149X13000771
Willett, D. S., Alborn, H. T., Stelinski, L. L., and Shapiro-Ilan, D. I. (2018). Risk taking of educated nematodes. PLoS ONE 13:e0205804. doi: 10.1371/journal.pone.0205804
Wilson, M. J., Ehlers, R.-U., and Glazer, I. (2012). Entomopathogenic nematode foraging strategies – is Steinernema carpocapsae really an ambush forager? Nematol. 14, 389–394. doi: 10.1163/156854111X617428
Wraight, S. P., Lopes, R. B., and Faria, M. (2017). “Microbial control of mite and insect pests of greenhouse crops”, in Microbial Control of Insect and Mite Pests, ed L. A. Lacey (Amsterdam: Elsevier), 237–252. doi: 10.1016/B978-0-12-803527-6.00016-0
Wu, S., Kaplan, F., Lewis, E., Alborn, H. T., and Shapiro-Ilan, D. I. (2018). Infected host macerate enhances entomopathogenic nematode movement towards hosts and infectivity in a soil profile. J. Invertebr. Pathol. 159, 141–144. doi: 10.1016/j.jip.2018.10.007
Zhang, C. X., Yang, S. Y., Xu, M. X., Sun, J., Liu, H., Liu, J. R., et al. (2009). A novel species of Serratia, family Enterobacteriaceae: Serratia nematodiphila sp.nov., symbiotically associated with entomopathogenic nematode Heterorhabditidoides chongmingensis (Rhabditida: Rhabditidae). Int. J. Syst. Evol. Microbiol. 59, 1603–1608. doi: 10.1099/00207713-59-10-2646-a
Keywords: entomopathogenic nematodes, biology, ecology, pest management, commercialization
Citation: Koppenhöfer AM, Shapiro-Ilan DI and Hiltpold I (2020) Entomopathogenic Nematodes in Sustainable Food Production. Front. Sustain. Food Syst. 4:125. doi: 10.3389/fsufs.2020.00125
Received: 20 April 2020; Accepted: 14 July 2020;
Published: 20 August 2020.
Edited by:
Surendra Dara, University of California System, United StatesReviewed by:
Raquel Campos-Herrera, Institute of Vine and Wine Sciences (ICVV), SpainSenthilkumar Murugaiyan, Tamil Nadu Agricultural University, India
Copyright © 2020 Koppenhöfer, Shapiro-Ilan and Hiltpold. This is an open-access article distributed under the terms of the Creative Commons Attribution License (CC BY). The use, distribution or reproduction in other forums is permitted, provided the original author(s) and the copyright owner(s) are credited and that the original publication in this journal is cited, in accordance with accepted academic practice. No use, distribution or reproduction is permitted which does not comply with these terms.
*Correspondence: Albrecht M. Koppenhöfer, YS5rb3BwZW5ob2ZlckBydXRnZXJzLmVkdQ==; David I. Shapiro-Ilan, ZGF2aWQuc2hhcGlyb0B1c2RhLmdvdg==; Ivan Hiltpold, aXZhbi5oaWx0cG9sZEBhZ3Jvc2NvcGUuYWRtaW4uY2g=