- 1Horticulture Section, School of Integrative Plant Science, College of Agriculture and Life Sciences, Urban Horticulture Institute, Cornell University, Ithaca, NY, United States
- 2Soil and Crop Sciences Section, School of Integrative Plant Science, College of Agriculture and Life Sciences, Cornell University, Ithaca, NY, United States
Rooftop farming intends to diversify options for enhancing sustainability of cities. From a policy perspective, vegetable production and stormwater management are among important goals of rooftop farming for bolstering public funding and policy support. However, crops with high value and market demand like salad greens often have high irrigation requirements, which risks increasing drainage output of water and nutrients. To date, no studies have compared various soil mixes intended for rooftop farms in terms of stormwater retention and yield of drought-sensitive crops constrained by regional precipitation patterns. Here, we report the results of a 5-week greenhouse experiment with leaf lettuce comparing five soil mixes made of coconut coir, biochar, and animal manure compost, plus a commercial rooftop farm soil using expanded shale, using an irrigation rate mimicking average growing season precipitation for New York City, USA. Three soil mixes had good yield, with water retention rates ranging up to 100%, while levels of drainage nitrogen output were <13% of current levels at the Brooklyn Grange, an operational rooftop farm in NYC. This finding suggests that improved soil design could enhance sustainability of rooftop farming in terms of water and nutrient management. Further research is needed for adjustment of nitrogen mineralization rates, long-term amendment plan, locally available waste inventory for substituting coconut coir, and leachate and rainwater harvesting systems.
Introduction
Agriculture is an emerging component of twenty first century urban planning to achieve diverse goals of sustainability. Efficient food supply chain and urban food security are among the goals specific to urban agriculture, while urban green space, including urban agriculture, are integral components of “green infrastructure” projects in a wide range of practices including stormwater management, energy conservation, biodiversity restoration, and waste management by using soils made of recycled materials (Mougeot, 2006; Lovell, 2010; Ackerman et al., 2013; Specht et al., 2014; Thomaier et al., 2015; Ahern, 2016; Grard et al., 2017; Harada et al., 2017). Horticultural technologies can expand options for adapting agriculture to urban planning. For example, green roof technologies for growing ornamentals on urban rooftops have been applied to intensive vegetable production systems, known as rooftop farms, which are retrofitted to underutilized roofs, incentivized by funding subsidies and policy supports for enhancing the sustainability of built environments (Ackerman et al., 2013; Harada et al., 2017). As a part of the New York City's 20-year stormwater management plan, for example, the Community-Based Green Infrastructure Program provided $592,730 for the construction of the Brooklyn Grange, a 0.61-ha rooftop farm atop an 11-story building of the former Brooklyn Navy Yard (NYC DEP, 2011). Furthermore, NYC's new zoning code known as “Zone Green” allows modification of rooftops for enhancing urban sustainability such as photovoltaic power generation and vegetated roofs including rooftop farms. These sustainable building solutions will be mandated by the Climate Mobilization Act of NYC in 2024, which could offer further opportunities for rooftop farming (NYC DCP, 2012; New York City Council, 2019).
Leafy vegetables, such as mustard greens (Brassica juncea) and leafy lettuce (Lactuca sativa), are in demand in cities, making them important crops for urban agriculture (Buehler and Junge, 2016; Baudoin et al., 2017). However, these leafy vegetables are drought-sensitive, and require that minimum soil water potential be maintained above 6–10 kPa to maintain growth and quality (Shock and Wang, 2011). This management regime can increase drainage loss of water and nutrients, posing negative impact on water quality, while increasing the costs of irrigation and nutrient subsidies (Shock and Wang, 2011). Rooftop farming may have advantages for achieving precise water and nutrient management similar to greenhouse production systems. For example, both systems use shallow (<500 mm) synthetic soils made of organic materials, such as peat moss, coconut coir, biochar, and compost, blended with mineral materials, such as vermiculite, sand, and expanded shale (Bunt, 2012; Harada et al., 2017). Furthermore, measurements of readily available water (RAW, the amount of water plants can extract from soil without drought stress) can be specific to each crop. In agriculture using field soils, RAW is estimated by the moisture release between 10 and 100 kPa of soil water tension (Gradwell and Birrell, 1979). Greenhouse agriculture focuses on RAW between 1 and 5 kPa or between 1 and 10 kPa, while moisture release up to 30 kPa is sometimes considered as available water (de Boodt and Verdonck, 1971; Fonteno, 1988; Milks et al., 1989; Argo, 1998; Bunt, 2012). Methods and concepts of greenhouse agriculture for growing drought-sensitive crops using shallow synthetic soils could be useful for improving hydrologic performance of rooftop farm soils.
Despite these readily available technologies, current rooftop farming practices have not focused on sustainable water and nutrient management. For example, studies of the Brooklyn Grange report that drainage output of water and nitrogen (N) was 197 and 83% of irrigation and fertilizer input during the growing season respectively, because preferential flow and hydrophobicity of the soil components reduced the water holding capacity of the soil (Harada et al., 2018a,b). These hydrologic properties of soil can be specific to levels of moisture fluctuations in outdoor environments, which are not traditionally studied for synthetic soils in greenhouse agriculture. Conversely, synthetic soils are not the traditional subjects of agriculture using field soils. Another factor reducing water holding capacity of the rooftop farm soils is blending expanded shale with animal manure and spent soils from mushroom production for manufacturing Rooflite Intensive Ag (Rooflite hereafter), the commercial mix used at the Brooklyn Grange (Kong et al., 2015; Skyland USA LLC, 2015; Harada et al., 2018b). In vegetated rooftops, including ornamental green roofs and rooftop farms, mineral aggregates, such as expanded shale, crushed bricks, pumice, are often used to promote rapid drainage in order to avoid exceeding the bearing capacity of the roof structures (Ampim et al., 2010). It must be recognized that these soils were originally developed for growing drought-tolerant ornamentals like sedum species, and may not contribute to the water holding capacity necessary for growing drought-sensitive crops (Oberndorfer et al., 2007; Eksi et al., 2015; Harada et al., 2017). Synthetic soils made of organic materials, known as potting soils or container mixes, can have higher water holding capacity and less bulk density than those using mineral aggregates. Studies of rooftop farms using potting soils report satisfactory yield and quality (Cho, 2008; Cho et al., 2010; Orsini et al., 2014; Grard et al., 2015, 2017; Sanyé-Mengual et al., 2015b), but none have specifically studied the effects soil composition and depth have on water and nutrient budgets.
Environmental planning addressing rooftop agriculture at the intersection of science and planning can provide specific benchmarks for evaluating the ecological performance of rooftop farms. In NYC's green infrastructure master plan, for example, the stormwater retention target is 25 mm day−1 for a variety of sites, including rooftop farms (NYC DEP, 2010). Given that NYC's monthly normal rainfall is 102 ± 9 mm during the growing season (Table 1), the stormwater management scenario means that project sites could retain rainfall events of 25 mm day−1 occurring four times a month. Furthermore, the observed precipitation exceeded evapotranspiration (ET) during the growing season at the Grange, suggesting that the farm could be self-sufficient in terms of water use (Harada et al., 2018b). This is important from an urban planning perspective because supplemental irrigation uses potable water, posing possible conflicts with human demands for water consumption. There are studies of rooftop farming aiming to inform urban planning by estimating possible contributions to city-wide fresh vegetable demands, competitive production costs in regional economy, and life-cycle costs including water, fertilizer, electricity, and building material consumption (Orsini et al., 2014; Grard et al., 2015, 2017; Sanyé-Mengual et al., 2015a,b), but none of these studies have focused on the role soil plays in this system. The objective of this study is to compare various soil mixes intended for rooftop farms in terms of stormwater retention and yield of drought-sensitive leafy greens, using an irrigation rate mimicking average growing season precipitation for New York City, USA.
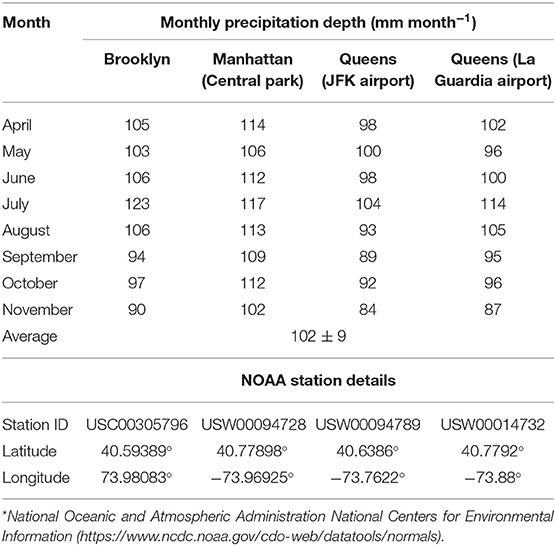
Table 1. Monthly normal rainfall during the growing season 1981–2010 in New York City, USA, reported by NOAA*.
Methods and Materials
During a 5-week greenhouse experiment, 6 mixes × 2 soil depths × 5 replicates = 60 experimental units (pots) were compared in terms of the water retention, drainage N output, and yield of leaf lettuce. All treatment units were received 25 mm of irrigation once a week, equivalent to the stormwater retention capacity of the NYC's stormwater management scenario within the context of monthly normal rainfall.
Laboratory Measurements of Moisture Release
Soil composition was based on the moisture released of the components at 0.93, 10, and 30 kPa tension (Table 2) using the method of Harada et al. (2018b). Briefly, volumetric water contents (VWC) at 0.93 kPa were measured by stacking, saturating, and draining soil rings filled with samples, while VWC at 10 and 30 kPa were measured by using a sand table. Coconut coir released the most water of 42% between 0.93 and 10 kPa (Table 2), and was selected as base material for all experimental mixes. Biochar was included in the mixes because it released the most water between 10 and 30 kPa (19%) and between 0.93 and 30 kPa (48%) (Table 2), suggesting that biochar could maintain yield within a broader range of moisture levels in comparison to coconut coir. Another material included in soil composition treatments was food waste and animal manure compost (FA compost) because, when mixed with coconut coir, moisture release levels of FA compost exceeded those of food and paper waste compost (FP compost) between 0.93 and 10 kPa and between 0.93 and 30 kPa (Table 2).
Soil Composition Treatments
Four experimental mixes, one commercial potting mix, and one commercial rooftop farm mix using expanded shale comprised a total of six soil composition treatments summarized in Table 3, while Table S1 provides soil property details. Coconut coir, biochar, and FA compost were used for experimental mixes (C100, CCp50, CB50, CBCp33). Each experimental mix received 1 g L−1 of commercial organic fertilizer (Pro-Start 2-3-3, North Country Organics, Bradford, VT) followed by 8-weeks of storage in open plastic buckets which were turned manually to promote aeration, while pH was adjusted by adding elemental sulfur at various rates summarized in Table S2. Of two commercial mixes, GreenTree Mix with biochar (GB) is a commercial mix using the same coconut coir and biochar in experimental mixes, while Rooflite (RL) is a commercial blend developed specifically for rooftop farming and used at the Brooklyn Grange.
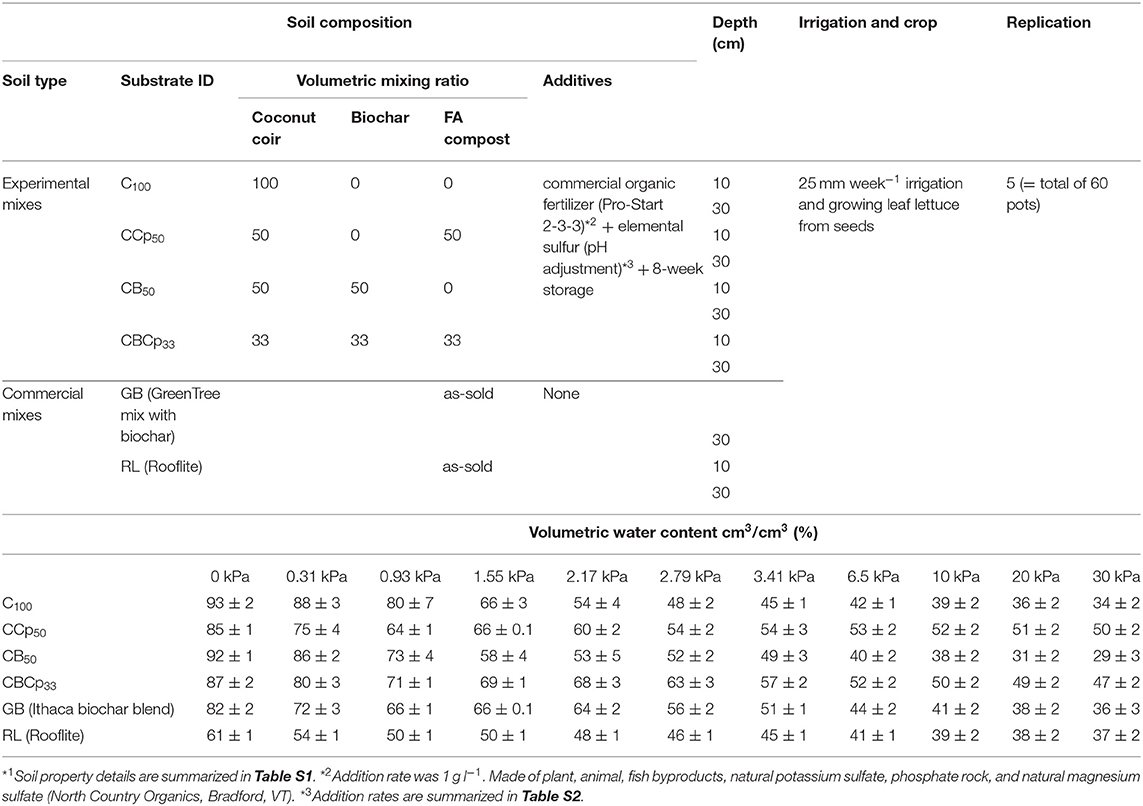
Table 3. Treatments of the greenhouse experiment*1 (bottom table shows detailed moisture release levels for each soil composition treatment).
Greenhouse Experiment
Experimental treatments and schedule are summarized in Table 3 and Figure 1, respectively. Six soil composition treatments × two soil depth treatments (10 vs. 30 cm) × five replicates consisted a total of 60 experimental units (pots) for 5-week greenhouse experiment at Guterman Bioclimatic Laboratory, Cornell University (Ithaca, NY USA). Cylindrical pots made of high-density polyethylene (product ID: TP1020R, Stuewe and Sons, Inc., Tangent, OR USA) were cut to the soil depth, randomly arranged on a greenhouse bench. A class-A evaporation pan was used to measuring evaporation. At time 0, each pot was irrigated to container capacity, or the maximum level of soil water required to produce a trace leachate sample. Each pot received 40 seeds of the leaf lettuce variety used at the Grange (Product ID: 2301.25, Johnny's Selected Seeds Inc., Winslow, ME USA), and germination rate was determined and reduced to 8 seedlings per pot after 1 week. Pots were weighed each week before and after 25 mm irrigation was applied and leachate samples were collected. At week 5, pots were weighed and all leaves were collected. Unvegetated pots were irrigated to container capacity to produce leachate samples, weighed and used as soil samples. The number of pots in which roots had reached the bottom of the soil column was determined.
Samples and Analyses
Soil leachate, soil, and leaf samples were analyzed by three different methods (Table 4). The Saturated Media Extract method (SME) (Lang, 1996; Dole and Wilkins, 1999) was used for producing water extracts from soil pastes. NO3-N and NH4-N concentrations of soil leachate samples and SME water extracts were analyzed by colorimetric method (SEAL AutoAnalyzer 2; SEAL Analytical GmbH, Norderstedt, Germany), while phosphorus (P), calcium (Ca), magnesium (Mg), and potassium (K) concentrations were analyzed only for SME water extracts by Inductively Coupled Plasma Atomic Emission Spectroscopy (ICP-AES). Total N and carbon (C) concentrations of soil and leaf samples oven-dried at 60°C were analyzed by combustion (Vario El Cube CHNOS Elemental Analyzer; Elementar Analysensysteme GmbH, Langenselbold, Germany).
Soil Samples
Before the experiment, subsamples were collected from each soil composition treatment and ingredients of experimental mixes. Each subsample was halved for total N and C concentration analysis and SME. After the experiment, a soil sample from each pot was halved and either preserved at 4°C for later SME and total N and C concentration analysis, or oven dried to determine the dry soil weight of each pot. Details of soil analyses are summarized in Table S1.
Leaf Samples
At the end of the experiment, leaf samples from each pot were weighed, and oven-dried at 60°C to determine dry weight followed by total N and C concentration analysis.
Soil Leachate Samples
Leachate samples were collected from pots after each irrigation for measuring volume, pH, and EC, followed by 4°C storage for later analysis of NO3-N and NH4-N concentrations. At time 0 and at the end of the experiment, soil was irrigated up to container capacity, producing trace leachate for measurements of pH and EC. The number of pots producing leachate samples in each irrigation event is summarized in Table S5.
Unit Conversion and Estimation Methods
Where Soil Volume = 1,344 cm3 for 10 cm pot, and 4,032 cm3 for 30 cm pot; Pot Surface Area= 134.4 cm2. Crop Coefficient is estimated by using Kp of 0.6 and 0.8, respectively, for light wind speed (Allen et al., 1998).
Results
Results for the entire study period and weekly results are summarized in Figures 2A–H, and in Figures 3A–H, respectively, while details of the results are summarized in Tables S3–S5. Yield for greens mix (lettuce and mustard greens) reported by a field study of the Brooklyn Grange (3.5 kg m−2 226 days−1 = 0.54 kg m−2 5 weeks−1) (Harada et al., 2018a) is used as reference for determining satisfactory yield. Drainage output of N (336 kg N ha−1 226 days−1 = 5.2 g N m−2 5 weeks −1 = 1.0 g N m−2 week−1) (Harada et al., 2018a) and ET (3.47 mm day-1 = 121 mm 5 weeks−1 = 24.3 mm week−1) (Harada et al., 2018b) at the Brooklyn Grange are shown in Figures 2E,G, 3A,B,G,H, respectively, as reference levels for general comparison.
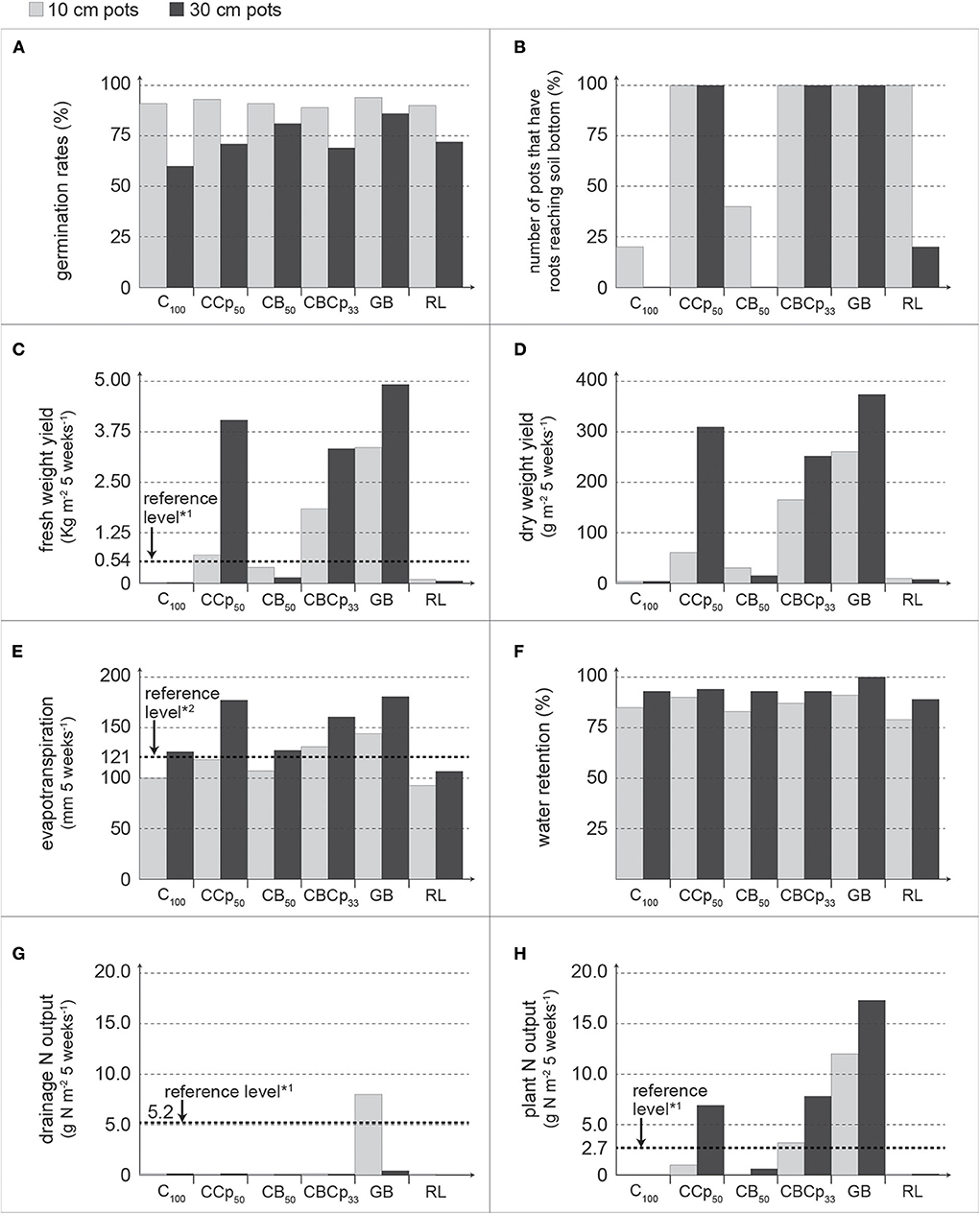
Figure 2. Cumulative results of the greenhouse experiment for the entire 5-week study period. (A) Germination rates, (B) Number of pots that have roots reaching soil bottom, (C) fresh weight yield, (D) dry weight yield, (E) evapotranspiration, (F) water retention, (G) drainage N output, (H) plant N output. *1Levels of the Brooklyn Grange, reported by Harada et al. (2018a), *2levels of the Brooklyn Grange, reported by Harada et al. (2018b).
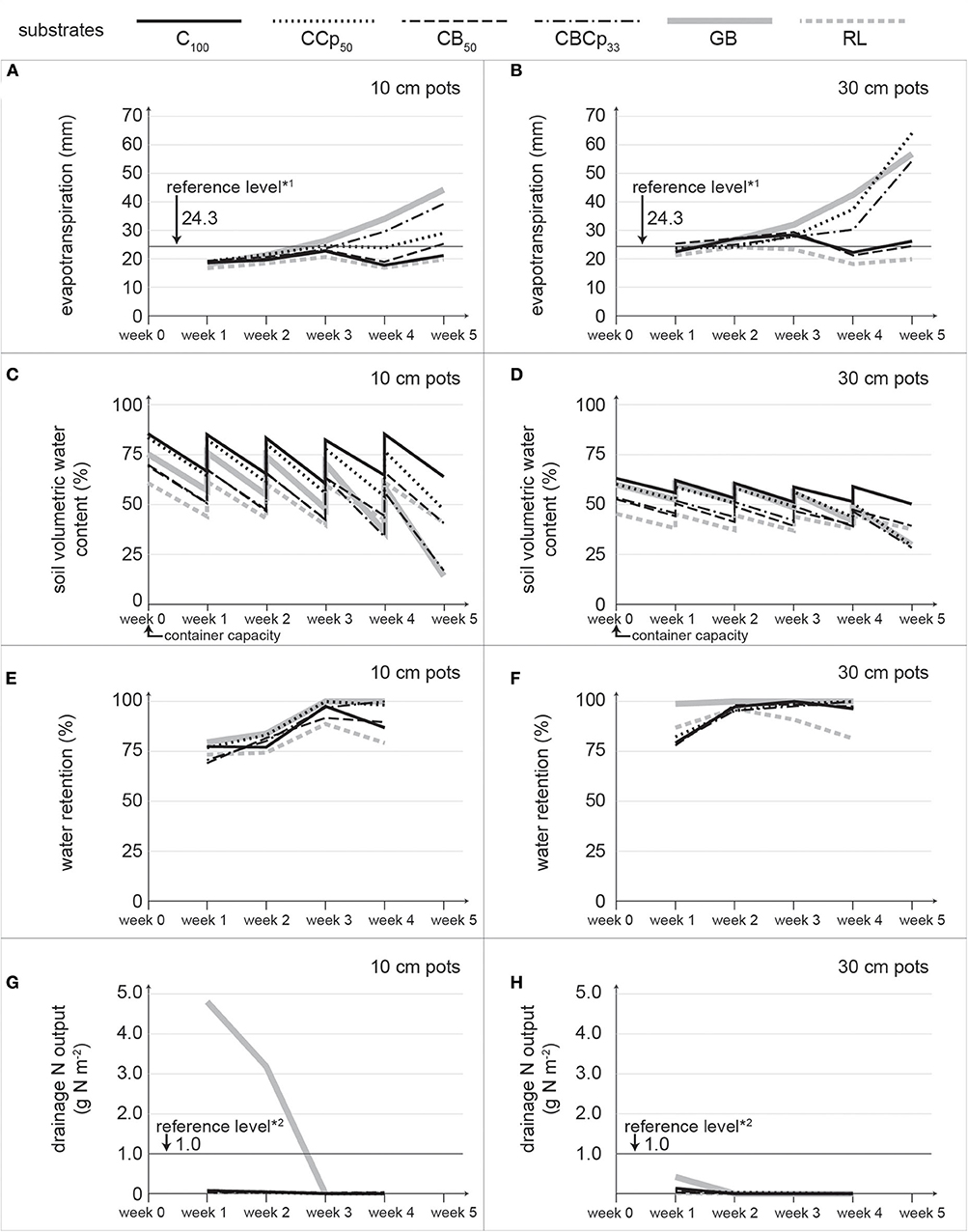
Figure 3. Weekly results of the greenhouse experiment. (A) Evapotranspiration for 10 cm pots, (B) evapotranspiration for 30 cm pots, (C) soil volumetric water content for 10 cm pots, (D) soil volumetric water content for 30 cm pots, (E) water retention for 10 cm pots, (F) water retention for 30 cm pots, (G) drainage N output for 10 cm pots, (H) drainage N output for 30 cm pots. *1Levels of the Brooklyn Grange, reported by Harada et al. (2018a), *2levels of the Brooklyn Grange, reported by Harada et al. (2018b).
Yield
Yield ranged from 0.02 to 4.92 kg m−2 5 weeks−1. For both depths, GB and C100 had the highest and the lowest levels of yield, respectively. Levels of yield for CCp50, CBCp33, and GB for both depths were satisfactory, ranging between 0.70 and 4.92 kg m−2 5 weeks−1, or 130 and 911% of the reference yield at the Brooklyn Grange (0.54 kg m−2 5 weeks−1) (Figure 2C). For those three mixes having satisfactory yield, soil depth had positive effects on yield, while yield's sensitivity to soil depth, or the ratio of yield for 30 cm soil to that of 10 cm soil, was highest for CCp50 (577%), followed by CBCp33 (181%) and GB (146%). Yield for all other mixes (C100, CB50, RL) for both soil depths were unsatisfactory, including RL, the actual mix used by the Brooklyn Grange. Yield of C100 and RL for both soil depths ranged only between 0.02 and 0.09 kg m−2 5 weeks−1, or between 4 and 17% of the reference yield at the Brooklyn Grange, due to the stunted growth upon germination. Yield of CB50 for both soil depths ranged only between 0.14 and 0.39 kg m−2 5 weeks−1, or between 26 and 72% of the reference yield at the Brooklyn Grange, due to the chlorotic leaf discoloration in week 2 onward.
Water Retention
Cumulative water retention rates over the entire study period ranged between 79 and 100%. In both soil depths, GB and RL had the highest and lowest rates of water retention, respectively. For all mixes, 30 cm deep soils retained more water than 10 cm deep soils, with water retention rates ranging 79–91% for 10 cm deep soils, and between 89 and 100% for 30 cm deep soils, respectively. For those three mixes having satisfactory yields, retention rates ranged between 87 and 100% including both soil depths. If soils retain water as intended in NYC's green infrastructure master plan (NYC DEP, 2010), then all irrigation must be retained (water retention rate = 100%). This standard was met only by the highest performing treatment, 30 cm deep GB if small amount (0.03 ± 0.07 mm) of leachate in week 1 is ignored (Figure 3F).
Drainage N Output
Cumulative drainage N output over the entire study period ranged between 0.03 and 7.99 g N m−2 5 weeks−1, or between 0.6 and 154% of the reference level of the Brooklyn Grange (5.2 g N m−2 5 weeks−1). In both soil depths, GB and RL had highest and lowest drainage N output, respectively. The ranking of drainage N output between 30 and 10 cm soil depths was inconsistent across mixes. Of all treatments, 10 cm deep GB had the highest drainage N output (7.99 g N m−2 5 weeks−1), and was the only treatment exceeding the reference level of the Brooklyn Grange due to the high N concentration of leachate in week 1 (NO3-N: 933.3 mg l−1, NH4-N: 7.5 mg l−1) and week 2 (NO3-N: 792.3 mg l−1, NH4-N: 2.7 mg l−1), respectively (Figure 3G), while drainage N output of all other treatments ranged only between 0.03 and 0.41 g N m−2 5 weeks−1, including RL, the same mix used in the Brooklyn Grange. Although, water retention rate of 30 cm deep GB was 100%, small amount of leachate produced in week 1 had high N concentration (NO3-N: 1207.7 mg l−1, NH4-N: 58.8 mg l−1), making drainage N output of GB highest in 30 cm deep soils.
ET
Over the study period, cumulative ET ranged between 92.7 and 180.8 mm 5 weeks−1, or between 77 and 149% of ET at the Brooklyn Grange (121 mm 5 weeks−1). In both soil depths, GB and RL had the highest and lowest ET, respectively. For all mixes, 30 cm deep soils had more ET than 10 cm deep soils, while ET's sensitivity to soil depths, or the ratio of ET for 30 cm soil to that of 10 cm soil, ranged from 115% (RL) to 150% (CCp50). In terms of weekly ET, both soil depths for three mixes having satisfactory yields (CCp50, CBCp33, and GB) had the highest ET in week 5 (29.1–64.1 mm week−1). All mixes had crop coefficients (Kc) between 0.2 and 0.3 during germination and from 0.1 to 0.6 for week 5 when leaf area was maximum.
Soil Water
Across treatments, VWC at container capacity ranged between 45 and 85%. Differences among the mixes remained nearly constant during the study. In greenhouse agriculture, VWC at water column pressure of half the soil depth is interpreted as container capacity, meaning that container capacity for 30 cm deep soil can be estimated by the soil VWC at 15 cm H2O, or 1.5 kPa. Difference between soil VWC at 1.55 kPa and container capacity for soil depth of 30 cm ranged from 3% for C100 to 17% for CBCp33. Lowest VWC for CCp50, CBCp33, and GB including both soil depths, were 29, 17, and 14% f, all of which exceeded soil water tension of 30 kPa, while having satisfactory yields.
Discussion
Sustainability of Rooftop Farming
The irrigation rate (25 mm week−1) in this study was only 69% of total water input at the Brooklyn Grange (irrigation + rainfall = 36.4 mm week−1). This irrigation rate was equal to the stormwater retention specification of the NYC's stormwater management scenario within the context of monthly normal rainfall of NYC. Three mixes (CCp50, CBCp33, GB) had yield exceeding the Grange by up to 911% while retaining up to 100% of irrigation. Except for the 10 cm deep GB treatment, drainage N output was <13% of that observed at the Grange, indicating a real possibility that these mixes could increase the sustainability of rooftop farming in terms of both water and nutrient management. At the Grange, the fact that precipitation alone exceeds ET demonstrates the potential for reducing supplemental irrigation. To achieve self-sufficient water use within rooftop farming systems, improved soil design could be complemented by recycling leachate from drainage outfalls. Also, rainwater can be harvested from uncropped roof surfaces (35–60% of total roof area) and stored for use during rainless periods (Orsini et al., 2014; Harada et al., 2018a). An example of this strategy is a rooftop greenhouse near Barcelona, Spain where the irrigation system from an adjacent rooftop satisfies 80–90% of the total water demand (Sanjuan-Delmás et al., 2018). Possible problems for recycling leachate from drainage outfalls include salt accumulation and increases in pathogens along with increased construction monetary costs of rooftop farming (Vallance et al., 2011; Sanyé-Mengual et al., 2015b).
Soil Depth
Water accumulates at the bottom of bounded soil columns (Bunt, 2012), which can make water more available to crops in 10 cm deep soils than in 30 cm deep soils. Cho (2008) report that the transpiration rates of lettuce in 5 cm pots exceeded rates in 10 and 20 cm. Three of our mixes (CCp50, CBCp33, GB) had satisfactory yields, however, the 30 cm deep pots had higher yield, ET, and water retention than 10 cm deep soils perhaps because total amount of available soil water (VWC × soil depth) is greater. For those three mixes, water depths of 30 cm deep soils at container capacity ranged from 217 to 240% of those in 10 cm deep soils, while weekly minimum water depths for 30 cm deep soils ranged between 186 and 630% of those in 10 cm deep soils. Roots reached the bottom of the soil column in both pot depths, so plants could extract water from the entire profile. Another possible factor for increased yield in 30 cm deep pots is higher air filled porosity (AFP) and oxygen availability to roots (Bunt, 2012). Greater VWC in 10 cm deep pots could reduce AFP, while top 20 of 30 cm deep soils could have much higher oxygen levels for root development. For example, the ratio of yield in 30 cm deep soil to that of 10 cm was highest for CCp50, which had highest proportion of compost, suggesting that small particles of compost reduced AFP and yield of 10 cm deep CCp50. In summary, 30 cm deep soil produced higher yield of drought-sensitive crops under restricted water supply.
N Management
Non-synthetic N was the only N source for all mixes in this study because organic cultural practices are an important marketing strategy for urban agriculture (Ackerman et al., 2013; Pölling et al., 2016, 2017; Harada et al., 2018a). Total inorganic N (TIN = NO3-N + NH4-N) is readily available to plants, while non-synthetic N contains organic N, which must be microbially converted to TIN for enhancing the levels of yield, making the control of N mineralization an integral part of soil management (Cameron et al., 2013). The only difference between the C100 and CCp50 mixes was the addition of compost to CCp50, while water extractable TIN decreased over the experiment only for C100, suggesting that the low rates of N mineralization was inadequate in C100. However, increasing TIN would increase N output in the leachate (Cameron et al., 2013) as evidenced by the fact that water extractable TIN for the unused GB mix (1041.1 TIN mg l−1) was much higher than all other mixes (1.5–16.9 TIN mg l−1). It is likely that animal manure and earthworm castings used in compost for GB increased TIN concentration, making 10 cm deep GB the only treatment exceeding the Brooklyn Grange in terms of drainage N output. Drainage N output of 30 cm deep GB did not exceed that of the Brooklyn Grange, because only a small amount of leachate was produced, while if drainage occurs under field conditions, then drainage N output of 30 cm deep GB could also exceed that of the Brooklyn Grange.
Water extractable TIN for used CCp50 ranged up to 1,207% of that for unused CCp50, suggesting the rates of N mineralization exceeding the net N output including drainage, plant uptake, and denitrification. High rates of N mineralization could require increased addition of organic N addition over time, while also increasing the risk of high levels of drainage N output after winter fallow periods. N mineralization rates could be adjusted by reducing the addition rates of compost and organic fertilizer. Drainage volume and N output can increase when plant N and water uptake is reduced or eliminated during the episodes of seeding and germination, crop failure, and winter fallow periods. Possible solutions include the use of cover crops during fallow periods, and desynchronized seeding and harvest by cropping mixed species (Malézieux et al., 2009; Cameron et al., 2013).
Based on the general nutrient management guideline of container mixes (Warncke and Krauskopf, 1983; Bunt, 2012), water extract NO3-N concentration of unused mixes exceeded the optimum range (100–199 NO3-N mg l−1) only for GB, while those for CCp50 and CBCp33 were below the acceptable range (40–99 NO3-N mg l−1). From the perspective of stormwater management, however, much lower range of water extractable N could be recommended. For example, Berghage et al. (2008) studied 30 ornamental green roofs for establishing reference soil specification of best stormwater management practices, and recommended water extractable TIN between 1.5 and 3.0 TIN-N mg l−1 for reducing drainage N output while maintaining crop performance. In summary, we recommend the CCp50 and CBCp33 mixes for rooftop farming to achieve satisfactory yield while reducing N output in the drainage water.
Soils Using Expanded Shale
At the Brooklyn Grange farm, satisfactory yields of leaf lettuce were maintained at VWCs of 20% in 25 cm deep Rooflite. In this study, leaf lettuce in Rooflite failed for both soil depths despite VWCs ranging from 37 to 61%. This suggests that low surface water content is a possible culprit. In a radically different cultural system using sedum, Rowe et al. (2014) compared growth using overhead sprinklers, drip, and subsurface irrigation in soils using coarse mineral aggregate like expanded shale. Yield was highest for the overhead sprinkler treatments because of increased moisture at the soil surface, while drip and subsurface irrigation treatments had lower surface moisture because capillary rise is limited by the large pore size in mixes using expanded shale. This means that soils using expanded shale can require frequent irrigation for growing drought-sensitive crops because ET rates exceeding water movement from deeper soil strata can easily reduce surface moisture. At the Brooklyn Grange overhead sprinklers are used up to 5 times a day for maintaining a moist soil surface, suggesting that the weekly irrigation used in this study did not maintain sufficient levels of surface moisture. In summary, soils using coarse mineral aggregates may not be suitable for rooftop farming aiming to maximize the use of soil water storage for stormwater management growing drought-sensitive crops.
Implications for Long-Term Management
In this short term study container capacity and soil depth remained constant, while these soil properties would change in rooftop farms where they are used indefinitely. In an experimental rooftop farming system, for example, Grard et al. (2015) grew lettuce and tomatoes in compost mixes made of prunings, crushed wood, and ground coffee wastes in Paris, France, and report that initial soil depth of 30 cm decreased to 20–15 cm over 2 years. This suggests that volumetric half-life of potting soils in the rooftop environments can be only 2 years. While coconut coir is used as base material for mixes is commonly used in horticultural as environmentally sound substitute for peat moss (Abad et al., 2002, 2005), further research is needed for substituting coconut coir with locally available wastes, such as saw dust, prune waste, and other lignocellulosic wastes (Barrett et al., 2016).
Unlike peat moss and coconut coir, biochar can be stable in soil (Lehmann et al., 2009), while maintaining moisture release between 10 and 30 kPa, making it potentially useful ingredient of rooftop soils. The biochar used in this study had high pH (10.0) and pH failed to stabilize in the CB50 mix which contained the highest proportion of biochar. Altland and Krause (2010) reported that pH adjustment for potting soils by using elemental sulfur can take up to 4 weeks to stabilize pH. In our study, the pH of CB50 continued to decrease from time 0 (6.0–6.3) to week 5 (4.1–5.1), following an 8-week pH stabilization period. In summary, pH adjustment of soils using biochar require further research, while long-term field research is necessary for establishing the management practices.
Conclusions
Under the irrigation rate equivalent to the stormwater retention capacity of the NYC's stormwater management scenario, three potting soils had satisfactory yield, with water retention rates ranging up to 100%, while levels of drainage N output were as much as 13% lower than that observed for the Brooklyn Grange, suggesting that mixes resembling potting soils could enhance the sustainability of rooftop farming in terms of water and nutrient management. Further research is required for (1) optimum addition rates of compost and organic fertilizer for adjusting N mineralization rates to plant N uptake; (2) optimum depths specific to soil composition; (3) vegetation strategies for reducing unvegetated periods; (4) long-term amendment plan and locally available waste inventory for substituting coconut coir; pH adjustment in mixes using biochar; and (5) leachate and rainwater recycling system design.
Data Availability Statement
All datasets generated for this study are included in the article/Supplementary Material.
Author Contributions
YH: experimental design, experiment, sample analysis, and manuscript preparation. TW: experimental design and manuscript preparation. NB and JR-A: experimental design. All authors contributed to the article and approved the submitted version.
Funding
This study was based on the United States Department of Agriculture (USDA) Hatch Grant (1001972), Sustainable Agriculture Research and Education (SARE) Partnership Grant Projects (ONE16-276), and the Toward Sustainability Foundation, and the Japan Society for the Promotion of Science (JSPS) KAKENHI Grant Number JP20K15549.
Conflict of Interest
The authors declare that the research was conducted in the absence of any commercial or financial relationships that could be construed as a potential conflict of interest.
Supplementary Material
The Supplementary Material for this article can be found online at: https://www.frontiersin.org/articles/10.3389/fsufs.2020.00123/full#supplementary-material
References
Abad, M., Fornes, F., Carrion, C., Noguera, V., Noguera, P., Maquieira, A., et al. (2005). Physical properties of various coconut coir dusts compared to peat. Hortscience 40, 2138–2144. doi: 10.21273/HORTSCI.40.7.2138
Abad, M., Noguera, P., Puchades, R., Maquieira, A., and Noguera, V. (2002). Physico-chemical and chemical properties of some coconut coir dusts for use as a peat substitute for containerised ornamental plants. Bioresour. Technol. 82, 241–245. doi: 10.1016/S0960-8524(01)00189-4
Ackerman, K., Dahlgren, E., and Xu, X. (2013). Sustainable Urban Agriculture: Confirming Viable Scenarios for Production. Final Report No.13-07.
Ahern, J. (2016). Novel urban ecosystems: concepts, definitions and a strategy to support urban sustainability and resilience. Landscape Archit. Front. 4, 10–22. Retrieved from https://scholarworks.umass.edu/larp_faculty_pubs/66 (Accessed 01 June 2019)
Allen, R., Pereira, L., Raes, D., and Smith, M. (1998). “Chapter 4: determination of ETo,” in Crop Evapotranspiration: Guidelines for Computing Crop Water Requirements. FAO Irrigation and drainage paper 56, eds Allen, R., Pereira, L., Raes, D., and Smith, M. (Rome: Food and Agriculture Organization of the United Nations).
Altland, J. E., and Krause, C. (2010). Modification of switchgrass substrate ph using compost, peatmoss, and elemental sulfur. HortTechnology 20, 950–956. doi: 10.21273/HORTSCI.20.6.950
Ampim, P. A., Sloan, J. J., Cabrera, R. I., Harp, D. A., and Jaber, F. H. (2010). Green roof growing substrates: types, ingredients, composition and properties. J. Environ. Hortic. 28:244. Retrieved from https://meridian.allenpress.com/jeh/article/28/4/244/79348/Green-Roof-Growing-Substrates-Types-Ingredients (Accesses 01 June 2019)
Argo, W. R. (1998). Root medium physical properties. HortTechnology 8, 481–485. doi: 10.21273/HORTTECH.8.4.481
Barrett, G., Alexander, P., Robinson, J., and Bragg, N. (2016). Achieving environmentally sustainable growing media for soilless plant cultivation systems–a review. Sci. Hortic. 212, 220–234. doi: 10.1016/j.scienta.2016.09.030
Baudoin, W., Desjardins, Y., Dorais, M., Charrondière, U., Herzigova, L., El-Behairy, U., et al. (2017). “Rooftop gardening for improved food and nutrition security in the urban environment,” in Rooftop Urban Agriculture (Springer), 219–233. doi: 10.1007/978-3-319-57720-3
Berghage, R., Wolf, A., Miller, C., Sanford, K., and Sanford, D. (2008). “Testing green roof media for nutrient content©,” in Combined Proceedings International Plant Propagators' Society, 386.
Buehler, D., and Junge, R. (2016). Global trends and current status of commercial urban rooftop farming. Sustainability 8:1108. doi: 10.3390/su8111108
Bunt, B. (2012). Media and Mixes for Container-Grown Plants: A Manual on the Preparation and Use of Growing Media for Pot Plants. London: Springer Science and Business Media.
Cameron, K., Di, H., and Moir, J. (2013). Nitrogen losses from the soil/plant system: a review. Ann. Appl. Biol. 162, 145–173. doi: 10.1111/aab.12014
Cho, Y. Y. (2008). Soil depth affects the growth of lettuce and chicory in extensive green roofs. Hortic. Environ. Biotechnol. 49, 434–438.
Cho, Y. Y., Choi, K. Y., and Lee, Y.-B. (2010). Effects of irrigation methods on the growth, water holding capacity of substrate and nutrient uptake of lettuce, chicory and endive grown in an extensive green roof system. Hortic. Environ. Biotechnol. 51, 348–354.
de Boodt, M., and Verdonck, O. (1971). The physical properties of the substrates in horticulture. III Symp. Peat Hortic. 26, 37–44. doi: 10.17660/ActaHortic.1972.26.5
Dole, J. M., and Wilkins, H. F. (eds.). (1999). “Nutrition,” in Floriculture: Principles and Species, (Upper Saddle River, NJ: Prentice Hall Inc.), 57–78.
Eksi, M., Rowe, D. B., Fernández-Cañero, R., and Cregg, B. M. (2015). Effect of substrate compost percentage on green roof vegetable production. Urban For. Urban Greening 14, 315–322. doi: 10.1016/j.ufug.2015.03.006
Fonteno, W. (1988). An approach to modeling air and water status of horticultural substrates. Symp. Substrates Hortic. Other Soils In situ 238, 67–74. doi: 10.17660/ActaHortic.1989.238.7
Gradwell, M., and Birrell, K. (1979). Methods for physical analysis of soils. New Zealand Soil Bureau scientific report; 10C. Department of Scientific and Industrial Research, New Zealand.
Grard, B. J.-P., Bel, N., Marchal, N., Madre, F., Castell, J.-F., Cambier, P., et al. (2015). Recycling urban waste as possible use for rooftop vegetable garden. Future Food J. Food Agric. Soc. 3, 21–34. Retrieved from https://hal.archives-ouvertes.fr/hal-01198279/document (Accessed 01 June 2019)
Grard, B. J.-P., Chenu, C., Manouchehri, N., Houot, S., Frascaria-Lacoste, N., and Aubry, C. (2017). Rooftop farming on urban waste provides many ecosystem services. Agron. Sustainable Dev. 38:2. doi: 10.1007/s13593-017-0474-2
Harada, Y., Whitlow, T. H., Bassuk, N. L., and Russell-Anelli, J. (2017). “Biogeochemistry of rooftop farm soils,” in Urban Soils, eds R. Lal and B. A. Stewart (Portland, OR: Taylor and Francis Group) 275–294. doi: 10.1201/9781315154251-13
Harada, Y., Whitlow, T. H., Templer, P. H., Howarth, R. W., Walter, M. T., Bassuk, N. L., et al. (2018a). Nitrogen biogeochemistry of an urban rooftop farm. Front. Ecol. Evol. 6:153. doi: 10.3389/fevo.2018.00153
Harada, Y., Whitlow, T. H., Todd Walter, M., Bassuk, N. L., Russell-Anelli, J., and Schindelbeck, R. R. (2018b). Hydrology of the brooklyn grange, an urban rooftop farm. Urban Ecosyst. 21, 673–689. doi: 10.1007/s11252-018-0749-7
Kong, A. Y., Rosenzweig, C., and Arky, J. (2015). Nitrogen dynamics associated with organic and inorganic inputs to substrate commonly used on rooftop farms. HortScience 50, 806–813. doi: 10.21273/HORTSCI.50.6.806
Lang, H. (1996). “Growing media testing and interpretation,” in Water, Media, and Nutrition for Greenhouse Crops, ed D. Reed (Batavia, IL: Ball Publishing), 123–139.
Lehmann, J., Czimczik, C., Laird, D., and Sohi, S. (2009). “Stability of biochar in soil,” in Biochar for Environmental Management: Science and Technology, eds J. Lehmann and S. Joseph (London: Earthscan), 183–206.
Lovell, S. T. (2010). Multifunctional urban agriculture for sustainable land use planning in the United States. Sustainability 2, 2499–2522. doi: 10.3390/su2082499
Malézieux, E., Crozat, Y., Dupraz, C., Laurans, M., Makowski, D., Ozier-Lafontaine, H., et al. (2009). “Mixing plant species in cropping systems: concepts, tools and models: a review,” in Sustainable Agriculture, eds E. Lichtfouse, M. Navarrete, P. Debaeke, S. Véronique, and C. Alberola (Dordrecht: Springer Netherlands), 329–353. doi: 10.1007/978-90-481-2666-8_22
Milks, R. R., Fonteno, W. C., and Larson, R. A. (1989). Hydrology of horticultural substrates. II. Predicting physical properties of media in containers. J. Am. Soc. Hortic. Sci. 114, 53–56.
Mougeot, L. J. (2006). Growing Better Cities: Urban Agriculture for Sustainable Development. Ottawa, ON: IDRC.
New York City Council (2019). Council to Vote on Climate Mobilization Act Ahead of Earth Day. Available online at: https://council.nyc.gov/press/2019/04/18/1730/ (accessed April 30, 2019).
NYC DCP (2012). Zone Green Text Amendment. Available online at: http://www1.nyc.gov/assets/planning/download/pdf/plans/zone-green/zone_green.pdf (accessed April 01, 2016).
NYC DEP (2010). NYC Green Infrastructure Plan: A Sustainable Strategy for Clean Waterways. Available online at: http://www.nyc.gov/html/dep/html/stormwater/nyc_green_infrastructure_plan.shtml (accessed April 01, 2016).
NYC DEP (2011). DEP Awards $3.8 Million in Grants for Community-Based Green Infrastructure Program Projects. Available online at: http://www.nyc.gov/html/dep/html/press_releases/11-46pr.shtml#.VvIn6fkrKUk (accessed April 01, 2016).
Oberndorfer, E., Lundholm, J., Bass, B., Coffman, R. R., Doshi, H., Dunnett, N., et al. (2007). Green roofs as urban ecosystems: ecological structures, functions, and services. BioScience 57, 823–833. doi: 10.1641/B571005
Orsini, F., Gasperi, D., Marchetti, L., Piovene, C., Draghetti, S., Ramazzotti, S., et al. (2014). Exploring the production capacity of rooftop gardens (RTGs) in urban agriculture: the potential impact on food and nutrition security, biodiversity and other ecosystem services in the city of Bologna. Food Secur. 6, 781–792. doi: 10.1007/s12571-014-0389-6
Pölling, B., Mergenthaler, M., and Lorleberg, W. (2016). Professional urban agriculture and its characteristic business models in Metropolis Ruhr, Germany. Land Use Policy 58, 366–379. doi: 10.1016/j.landusepol.2016.05.036
Pölling, B., Sroka, W., and Mergenthaler, M. (2017). Success of urban farming's city-adjustments and business models–findings from a survey among farmers in Ruhr Metropolis, Germany. Land Use Policy 69, 372–385. doi: 10.1016/j.landusepol.2017.09.034
Rowe, D. B., Kolp, M. R., Greer, S. E., and Getter, K. L. (2014). Comparison of irrigation efficiency and plant health of overhead, drip, and sub-irrigation for extensive green roofs. Ecol. Eng. 64, 306–313. doi: 10.1016/j.ecoleng.2013.12.052
Sanjuan-Delmás, D., Llorach-Massana, P., Nadal, A., Ercilla-Montserrat, M., Muñoz, P., Montero, J. I., et al. (2018). Environmental assessment of an integrated rooftop greenhouse for food production in cities. J. Cleaner Prod. 177, 326–337. doi: 10.1016/j.jclepro.2017.12.147
Sanyé-Mengual, E., Oliver-Solà, J., Montero, J. I., and Rieradevall, J. (2015a). An environmental and economic life cycle assessment of rooftop greenhouse (RTG) implementation in Barcelona, Spain. Assessing new forms of urban agriculture from the greenhouse structure to the final product level. Int. J. Life Cycle Assess. 20, 350–366. doi: 10.1007/s11367-014-0836-9
Sanyé-Mengual, E., Orsini, F., Oliver-Solà, J., Rieradevall, J., Montero, J. I., and Gianquinto, G. (2015b). Techniques and crops for efficient rooftop gardens in Bologna, Italy. Agron. Sustainable Dev. 35, 1477–1488. doi: 10.1007/s13593-015-0331-0
Shock, C. C., and Wang, F.-X. (2011). Soil water tension, a powerful measurement for productivity and stewardship. HortScience 46, 178–185. doi: 10.21273/HORTSCI.46.2.178
Skyland USA LLC (2015). Rooflite Intensive Ag Product Specification. Available online at: http://www.rooflitesoil.com/products/intensive-ag (accessed June 01, 2016).
Specht, K., Siebert, R., Hartmann, I., Freisinger, U. B., Sawicka, M., Werner, A., et al. (2014). Urban agriculture of the future: an overview of sustainability aspects of food production in and on buildings. Agric. Human Values 31, 33–51. doi: 10.1007/s10460-013-9448-4
Thomaier, S., Specht, K., Henckel, D., Dierich, A., Siebert, R., Freisinger, U. B., et al. (2015). Farming in and on urban buildings: present practice and specific novelties of zero-acreage farming (ZFarming). Renewable Agric. Food Syst. 30, 43–54. doi: 10.1017/S1742170514000143
Vallance, J., Déniel, F., Floch, G. L., Guérin-Dubrana, L., Blancard, D., and Rey, P. (2011). “Pathogenic and beneficial microorganisms in soilless cultures,” in Sustainable Agriculture, Vol. 2, eds E. Lichtfouse, M. Hamelin, M. Navarrete, and P. Debaeke (Dordrecht: Springer Netherlands), 711–726. doi: 10.1007/978-94-007-0394-0_31
Keywords: urban agriculture (UA), rooftop agriculture, soilless media, stormwater management (SWM), green roof, green infrastructure (GI), urban ecology, ecosystem services (ES)
Citation: Harada Y, Whitlow TH, Bassuk NL and Russell-Anelli J (2020) Rooftop Farm Soils for Sustainable Water and Nitrogen Management. Front. Sustain. Food Syst. 4:123. doi: 10.3389/fsufs.2020.00123
Received: 07 August 2019; Accepted: 10 July 2020;
Published: 20 August 2020.
Edited by:
Mariana Benítez, National Autonomous University of Mexico, MexicoReviewed by:
Ilias Travlos, Agricultural University of Athens, GreeceGiorgio Prosdocimi Gianquinto, University of Bologna, Italy
Copyright © 2020 Harada, Whitlow, Bassuk and Russell-Anelli. This is an open-access article distributed under the terms of the Creative Commons Attribution License (CC BY). The use, distribution or reproduction in other forums is permitted, provided the original author(s) and the copyright owner(s) are credited and that the original publication in this journal is cited, in accordance with accepted academic practice. No use, distribution or reproduction is permitted which does not comply with these terms.
*Correspondence: Yoshiki Harada, eWg1MzUmI3gwMDA0MDtjb3JuZWxsLmVkdQ==