- Carbon Management and Sequestration Center, The Ohio State University, Columbus, OH, United States
Per capita intake of animal protein is expected to increase globally through 2050, and the rate of increase will be more in developing or emerging economies than in developed countries. Global meat consumption between 1980 and 2050 is projected to increase from 133 million to 452 million tons, and 86% (279 million tons) of the increase will occur in developing countries. Animal-based agricultural systems occupy 45% of the global land area and contribute a large proportion of agricultural emissions. In addition to being a major source of nitrous oxide (N2O), methane (CH4), and other greenhouse gases (GHGs), livestock also use 8% of the global water withdrawal. The animal sector is dominated by resource-poor and small landholders of developing countries. Adverse effects of livestock on the environment are caused by the way animal husbandry is practiced, in no small part because animals are not integrated with other agricultural and forestry-based practices. Thus, improving and sustaining the livestock sector is critical to advancing the Sustainable Development Goals (SDGs) of the United Nations, especially SDG #1 (No Poverty), SDG #2 (Zero Hunger), SDG #6 (Clean Water and Sanitation), and SDG #13 (Climate Action). Separating raising of livestock from cultivating seasonal crops and perennial trees has decoupled the biogeochemical/biogeophysical cycling of carbon (C), water (H2O), nitrogen (N), phosphorus (P), and sulfur (S). This decoupling is a causative factor of the increase in emissions of N2O and CH4, eutrophication and contamination of water resources, degradation of rangelands, and decline in its biodiversity. Therefore, identifying and adopting systems that integrate livestock with crops and trees are critical for reducing the environmental footprint of animal-based dietary products. Incorporating pastures/forages in the rotation cycle along with controlled grazing, called ley farming, and agroforestry, such as alley cropping, are examples of integrated farming systems. Other strategies of reducing the environmental footprint comprise the following: reducing enteric fermentation by precision feeding and matching dietary protein to animal need, processing CH4 and N2O emissions for other uses, and managing manure and other animal waste prudently. Other important considerations are adopting multiple GHG perspectives and minimizing gas swapping, reducing wastage of animal products, decreasing the use of antibiotics, and restoring rangeland for sequestration of atmospheric CO2 as soil organic matter.
Introduction
The domestication of animals, which started as early as the 12th millennium circa BP (Zeder, 2008), began with dogs and was followed by that of ruminants (i.e., goats, sheep, cattle). Chickens were domesticated about 10,000 years ago, followed by oxen and horses as beasts of burden for plowing and transportation (Rutledge and McDaniel, 2011). Over millennia, the cultivation of crops was closely integrated with that of raising livestock. Since the mid-twentieth century, however, the separation of raising livestock from the growing of crops has caused environmental issues such as the degradation of soil health, eutrophication of water, emission of greenhouse gases (GHGs) into the atmosphere, and loss of biodiversity (Peyraud et al., 2014).
Raising livestock separately may not be a sustainable option (Broom et al., 2013) economically, pedologically or ecologically. In view of the numerous demands of the growing and increasingly affluent human population, achieving food and nutritional security is seemingly at odds with the necessity of reducing the negative environmental footprint of agriculture. An important cause of this dilemma may be the simplification of agro-ecosystems, and the attendant decline in diversity of farming systems at the soil scape, landscape, and the farm scale (Lemaire et al., 2014). The adverse effects of livestock on the environment are attributed to the way in which the animals are raised, and such issues can be addressed (Dalibard, 1995). In some climates and landscapes, separating livestock from crops and trees is an important cause of the decline in diversity at the farm scale, with the attendant adverse impacts on the environment. Such a simplification and loss of biodiversity also leads to decoupling of the cycling of carbon (C) from those of water (H2O), nitrogen (N), phosphorus (P), and sulfur (S) (Lal, 2010). Cycles of N and C, closely connected to livestock's role in land use and land use change (Steinfeld et al., 2006), may be decoupled by this simplification of the farming system. Emission of GHGs (i.e., CH4) is exacerbated when ruminants are concentrated, which tends to uncouple the C and N cycle by releasing the digestible C as CO2 and CH4 and digestible N in waste as N2O (Soussana and Lemaire, 2014). The risks of uncoupling, which has severe implications to climate change because CH4 and N2O have a high global warming potential (GWP), can be minimized by integrating livestock with crops and trees. Practices such as establishing vegetation buffers on agricultural fields to enhance biodiversity and conserve soil and water (i.e., agroforestry or alley cropping), can also reduce the environmental footprint of livestock raised on the same land unit (Goldstein et al., 2012).
The objectives of this article are to discuss: (1) the potential and challenges of increasing food and nutrition for the growing human population by raising livestock, (2) the livestock sector and the Sustainable Development Goals (SDGs) of the United Nations, (3) the conceptual basis of integrating livestock with crops and trees to increase the biodiversity of farming systems, (4) the options for sustainable management of grasslands for food and climate security, (5) the potential of integration of livestock with crops and trees to sequester carbon and reduce gaseous emissions, and (6) improved management of livestock in the tropics.
The Potential and Challenges of Increasing Food and Nutrition for the Growing Human Population by Raising Livestock
Fears of widespread famine were aggravated by the rapid population growth during the 1950s and 1960s (Ehrlich, 1968). The human population of 2.56 billion (B) in 1950 increased to 3.04 B in 1960, 3.71 B in 1970, and 4.34 B in 1980 at the 10-year growth rate of 18.9, 22.0, and 20.2%, respectively. The fears of widespread famine were averted by the spectacular increase in yields of cereal crops, achieved through the Green Revolution during the 1960s (Pingali, 2012). However, the world population has increased to 7.8 B in 2020 and is projected to be 9.8 B by 2050 and 11.2 B by 2100 (UN, 2019b). Whereas 820 million people are prone to undernourishment (FAO, 2017), about 2 B are suffering from malnourishment because of deficiencies in protein, micronutrients, and vitamins (Ritchie and Roser, 2019). However, the livestock sector can play an important role in eliminating hunger and malnourishment.
Since the 1960s, large parts of natural lands have been converted into agro-ecosystems to feed the growing world population. In addition to reducing biodiversity, conversion of natural ecosystems at a larger scale has also depleted and contaminated water resources, polluted air, and exacerbated the emission of GHGs into the atmosphere. There has also been a growing interest in increasing animal products to address malnourishment. The global population of livestock (i.e., cattle, sheep, goats, pigs, poultry) has increased drastically since the 1950s. This increase in both populations (i.e., human and animals) has also led to a growing concern whether the biosphere has the capacity to support such large populations of domesticated livestock and people.
The human population has increased from about 10–20 million at the dawn of settled agriculture to about 7.8 B (~10,000 times) in 2020 (UN, 2019a), and there is an equally alarming growth of the population of domesticated livestock. While the cattle population has declined from a of high of 1.4 B in 2011, it still remains at ~1 B in 2019 (The Economist, 2011; Shahbandeh, 2019). The global average stock of chicken is estimated at 19 B, and that of sheep and pigs at about 1 B. Global demand for animal-based produce is projected to double by 2050 (Herrero et al., 2009) because of the increasing affluence and the change in dietary preferences (Rojas-Downing et al., 2017). The global population of bovines is projected to increase from 1.9 B in 2010 to 2.4 B in 2030, 2.6 B in 2040, and 2.64 B in 2050 (Rosegrant et al., 2009; Thornton, 2010). The human population is increasing at an average global annual rate of 1.2%, but the population of domesticated livestock is increasing at an annual rate of 2.4%. The geographical distributions of livestock population also vary widely depending on biophysical, socio-economic, and cultural factors (Gilbert et al., 2018).
Along with the livestock population, the amount of livestock produce is also growing rapidly. Between 2000 and 2050, global production is projected to increase from 229 to 465 million tons of meat and 580 to 1043 million tons of milk (FAO, 2006; Steinfeld et al., 2006). More than 60 B land animals are used worldwide for meat, egg, and dairy production, and the global population of livestock may exceed 100 B by 2050 (Yitbarek, 2019), when the world's meat production is projected to double (FAO, 2019). All trends from 1980 to 2002 indicate that meat consumption increased from 47 million to 132 million tons in developing countries (NAS, 2015). All trends from 1980 to 2050 indicate that meat consumption is projected to increase from 86 million to 120 million tons in developed countries and 47 million to 326 million tons in developing countries (NAS, 2015). By 2050, the increase in meat production may be 290% for pig meat, 200% for sheep and goats, 180% for beef and buffalo meat, 180% for milk, 700% for poultry meat, and 90% for egg (Yitbarek, 2019). Similar to meat products, production of milk is also increasing globally. With a current average milk consumption of 100 kg per person per year (Reay and Reay, 2019), the projected increase in population will increase milk production as well. Each liter of fresh milk is equivalent to 3 kg of GHG emissions (Reay and Reay, 2019).
The strong nexus between livestock and anthropogenic climate change can neither be denied nor ignored. Indeed, livestock impact climate change, and the rapidly changing climate is also impacting livestock. It is precisely in this context that integrating livestock with crops and trees can play an important role in re-greening of the planet (Janzen, 2011). Harnessing the positive effects of livestock-based farming systems (e.g., nutritious food, eliminating hunger and hidden hunger) can lead to sustainable management of crops and trees and reduce the environmental footprint of farming (Herrero et al., 2009). In addition, sustainable management of rangelands by adopting ecologically based principles of animal husbandry can strengthen the provisioning of ecosystem services (ESs) from these fragile and ecologically-sensitive but economically important ecoregions (Havstad et al., 2007).
Livestock Sector and Sustainable Development Goals of the United Nations
The highly dynamic livestock sector is rapidly changing in response to the ever-increasing demands of the growing population, especially in developing countries. Thus, judicious management and eco-intensification of livestock-based systems can also address the daunting challenge of advancing the SDGs of the United Nations (Figure 1) because site-specific integration of crops with livestock is critical to advancing several SDGs. Specifically, prudent management of livestock can advance SDG #1 (No Poverty) by improving income of small landholders as well as that of commercial farmers. For small landholders in developing countries, livestock are not only a source of nourishment, they are also a source of renewable energy through draft animals, use of dung as household fuel, and also a source of manure as an amendment for crops. In addition to addressing the vulnerability of 820 million under-nourished people, most of them concentrated in South Asia and Sub-Saharan Africa (FAO, 2017), judicious production and use of animal-based diet can also alleviate malnutrition (hidden hunger) affecting 2 B people globally. Thus, livestock are critical to advancing SDG #2 (Zero Hunger).
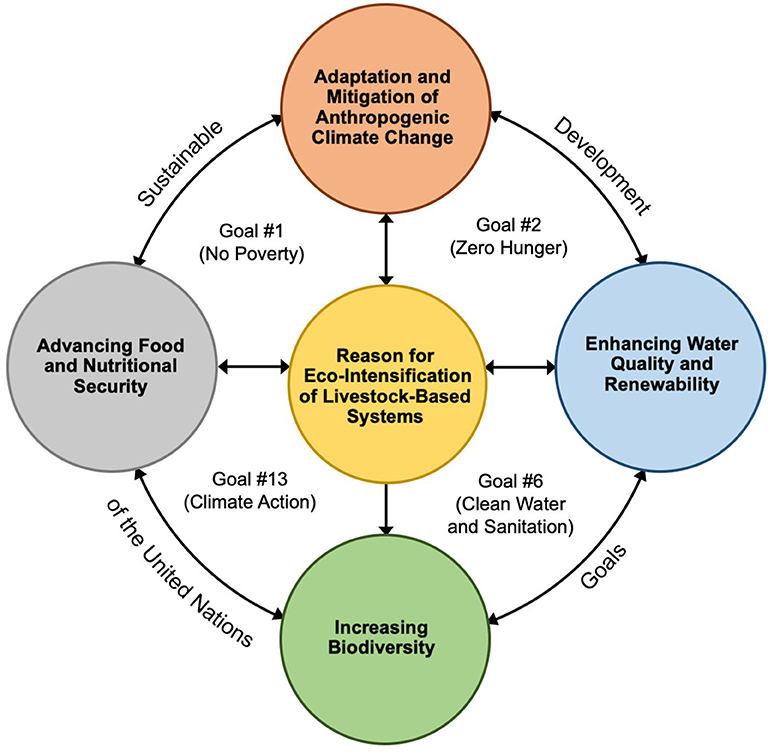
Figure 1. Eco-intensification of livestock-based systems to advance the Sustainable Development Goals of the United Nations.
The livestock industry, which consumes 8% of the global water supply (Schlink et al., 2010), has a strong impact on SDG #6 (Clean Water and Sanitation). Livestock production involves the use of both blue and green water (Falkenmark, 2003). Nearly one-third of the total water footprint of agriculture in the world is related to animal products (Mekonnen and Hoekstra, 2012), and beef has a larger water footprint than poultry and pork (Gerbens-Leenes et al., 2013). Therefore, reducing the water footprint of livestock, an important consideration of eco-intensification of livestock-based systems (Doreau et al., 2012), can advance SDG #6. Judicious management of livestock and rangelands is critical to improving the quality and renewability of water through buildup of soil organic matter content that can enhance soil water storage and denature and filter pollutants.
In addition to water, reducing emissions of GHGs from the livestock sector is pertinent to advancing SDG #13 (Climate Action). Because of its importance, the interaction between climate change and the livestock sector is now widely recognized (Thornton et al., 2009). Livestock are responsible for a large part of agricultural emissions (Gill et al., 2010; Havlík et al., 2014). Agriculture contributes about 10–12% of the current anthropogenic emissions. Some estimate that direct livestock non-carbon dioxide emissions caused about 19% of the total modeled warming of 0.81OC from all anthropogenic emissions in 2010 (Reisinger and Clark, 2018). GHG emission per unit of livestock product is more in ruminants than that in monogastric animals (Gill et al., 2010). Because of the high global warming potential (GWP) of CH4 and N2O, it is appropriate to combine the cumulative effect of all GHGs into CO2-equivalent (Pitesky et al., 2009).
Conceptual Basis of Integrating Livestock with Crops and Trees
Livestock use 30% of the Earth's entire land surface as permanent pastures; 33% of arable land is used to produce feed for the livestock (FAO, 2006), and thus livestock have a large environmental footprint (Smith et al., 2013). Pelletier and Tyedmers (2010) projected that the livestock sector will even more strongly impact the environment by 2050 with regards to three issues: (i) climate change, (ii) reactive nitrogen mobilization, and (iii) appropriation of plant biomass at a global scale. Pelletier and Tyedmers also predicted that the livestock sector alone may overshoot humanity's “safe operating space” by 2050 in each of these three domains. While (FAO, 2006) estimates in the report “Livestock's Long Shadow” have been strongly debated (Maday, 2019), emissions of GHGs from the livestock sector, especially that of CH4 and N2O, can be reduced and managed by adapting the integrated systems presented herein. It is also pertinent to carefully choose site-specific sustainable livestock production to reduce or mitigate emissions, and to develop policies that promote climate change adaptation and mitigation options (Rojas-Downing et al., 2017). Some concerns about the impacts of animal-based diet (Pitesky et al., 2009; Gerber et al., 2013b; Eshel et al., 2014; Hedenus et al., 2014) can be addressed through a judicious integration of crops with livestock. The latter can lead to an increase in the quantity and quality of food production and economic returns while also reducing pressure on land and water resources (Franzluebbers, 2007; Provenza et al., 2019).
Most emissions from the livestock sector occur in commodity (meat, milk) production or the supply-side. However, gaseous emissions are also affected by the demand-side, or the consumer population, which is not only growing in numbers but is also undergoing a nutrition transition in favor of the animal-based diet. Therefore, several studies have suggested that merely addressing the supply-side emissions from the livestock sector may be insufficient to limit the temperature rise to <2°C, and addressing the demand-side is also necessary (Kiff et al., 2016; Scherer and Verburg, 2017). Indeed, demand-side mitigation measures—including preferences for a plant-based diet, along with eating more poultry and fish than red meat, or grass-fed rather than grain-fed meat – have a greater potential to reduce emissions (1.5–15.6 Gt CO2-eq /yr) (1 Gt = gigaton = billion ton) than do supply-side measures (1.5–4.3 Gt CO2-eq/yr) (Smith et al., 2013). An integrated and judicious management of crops and livestock may mitigate some of the negative environmental impacts on the supply-side when crops are grown separately from that of raising the livestock (Herrero and Thornton, 2013).
Ruminant production systems are under pressure for several reasons: (i) methane emission, (ii) inefficient use of land, (iii) feed-food competition, and (iv) weakening of key ecosystems services through large-scale conversion of grasslands to crop production for livestock. However, livestock can produce human food of high nutritional quality from marginal lands that are mostly unsuitable for crop production. Thus, a viable strategy may involve the following: (i) raising animals from feed that is non-edible for humans, (ii) grazing livestock on land not suitable for crop production, and (iii) reducing emissions of GHGs (CH4, N2O). Some site-specific grassland-based ruminant production systems are much more efficient than concentrate-based systems for producing protein (Peyraud and Peeters, 2016). The challenge lies in developing sustainable systems of forage production that also lead to positive responses to societal demands for consuming more natural products (Peyraud and Peeters, 2016).
Site-specific options for integrated crop-livestock systems can also achieve synergies between agricultural production and environmental quality (Lemaire et al., 2014). Table 1 outlines examples of sustainable intensification of livestock-based systems, involving judicious combinations of sod/forages with crops and trees, which address some concerns of ruminant production systems. The term “sod” refers to the soil surface when covered with grass, sward, or turf. By using grassland-based ruminant-livestock systems (GRLS) models of African Guinea Savanna, Bateki et al. (2019) observed that sustainable intensification of livestock, integrated with crops and trees, could increase food security of the growing African population.
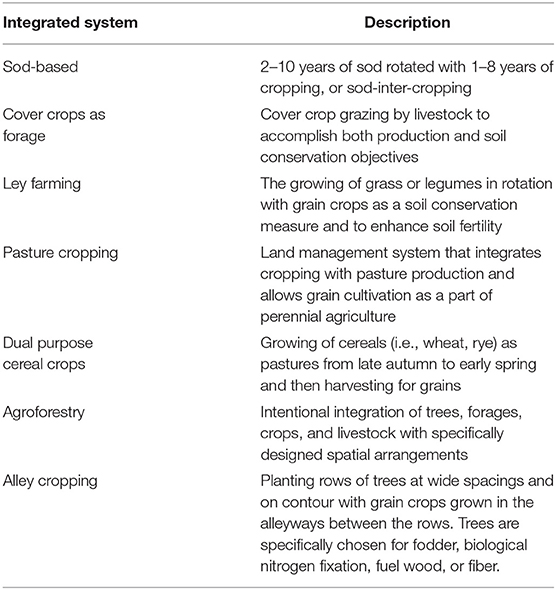
Table 1. Examples of integrated livestock systems with crops and trees (Compiled from Kang et al., 1990; Leakey, 1996; McCown, 1996; Bajracharya et al., 1998; Garrett et al., 2004; Fike et al., 2016; Jose and Dollinger, 2019; Munsell and Chamberlain, 2019; USDA-NRCS, 2020).
Agroforestry is a set of technologies in which trees are sequentially or simultaneously integrated with crops and/or livestock in a wide range of integrated systems (Leakey, 1996). Alley cropping is a system of planting trees on the contour at a wide spacing (4–10 m apart) with a food crop grown in the alley ways between the rows of trees. Planting several rows of trees and shrubs, which can also be used as forage, is a system that integrates livestock with both crops and trees. Trees can also be harvested as a source of fuel wood. Such a complex system is an example of an agro-silvopastorial system (Okali and Sumberg, 1985; Kang et al., 1990). In temperate alley cropping systems, tree species may include hard wood veneer or lumber species; softwood species for fiber production, or fruits and nuts for food (USDA, 2020). Trees grown on the contour can also be used as filter strip and for contour farming in strip cropping (USDA-NRCS, 2020). Grain crops (i.e., corn, soybean, cowpeas) are grown when the trees are young. When the ground is shaded, forages can be harvested and cattle grazed, and the prunings can also be used as green manure for cereals (i.e., corn). Leguminous trees serve as a source of nitrogen to enhance soil fertility.
Models are needed for simultaneous quantification of C and N flows and how they are affected by different livestock-crop-tree management systems. Several whole-farm based models have tried to estimate gaseous emissions (Snow et al., 2014; Bateki et al., 2019), but there is a need for more data on nutrient and C flows at the field level (Snow et al., 2014).
Options for Sustainable Management of Grasslands for Food and Climate Security
Site-specific options are needed for sustainable intensification of livestock systems in diverse socio-economic and biophysical regions prone to climate change. For example, livestock-based systems occupy 45% of the global land area; grasslands/savannas suitable for grazing cover 37% of Earth's surface area (NAS, 2015). These ecosystems are highly diverse and occur within the seasonally dry tropical to sub-tropical equatorial regions (Whitley et al., 2017). Savanna ecoregions, open-canopy and fire-dependent biomes, are also prone to climate change that may alter phenology, root-water access and fire dynamics (Whitley et al., 2017). Principal environmental drivers affecting biomass/feedstock productivity in savanna regions are water and nutrient availability, vapor pressure deficit, solar radiation and fire (Devi Kanniah et al., 2010). Therefore, understanding these controls and their management through eco- intensification is critical for enhancing net primary productivity (NPP) under the changing global environment (Kanniah et al., 2013). Important controls include restoring soil functions, conserving water to minimize the risks of drought, and adopting improved species of forages and meat of better nutritional quality (Herrero and Thornton, 2013; Provenza et al., 2019).
Climate change is already adversely impacting agro-pastoral production in Africa (Stige et al., 2006; O'Mara, 2012). Under these conditions, Teague et al. (2011) observed that multi-paddock (MP) grazing may be an option for sustainable intensification. Teague and colleagues reported that MP grazing at a high stocking rate increased SOC content and cation exchange capacity of soil compared with light continuous and heavy continuous grazing. Similarly, Kleppel (2019) reported that microbial biomass in MP grazed soils was higher, more diverse, and contained relatively more fungal than bacterial biomass than did conventional management and hay field. A 2-year study in South Africa by Chaplot et al. (2016) showed that topsoil SOC stocks were significantly increased in soil with either livestock exclosure and NPK fertilization or high density and short duration grazing compared with annual burning, livestock exclosure and livestock exclosure with topsoil tillage. This was accomplished by high intensity, short duration grazing (HDSD, 1200 cows per ha for only 3 days per year) followed by complete exclosure for the remainng 362 days each year (Chaplot et al., 2016). On the basis of a global assessment of holistic planned grazing, however, Hawkins (2017) concluded that only rangelands with higher precipitation have the resources to support MP grazing at a high stocking rate.
The Potential for Integrating Livestock with Crops and Trees to Sequester Carbon and Reduce Gaseous Emissions
Restoration and sustainable management of grasslands can play an important role in adaptation and mitigation of climate change (Lal, 2008). Technical potential of C sequestration in global savannas, through land restoration and integrated management of livestock with crops and trees, can be as much as 2.55 Gt C/y (Table 2). Pertinent animal feeding strategies (e.g, use of flax seeds, protein-intensive forages) can reduce enteric CH4 and NH3 emissions (Yáñez-Ruiz et al., 2018). Above all, carbon sequestration in grass—by planting species with high biomass production and biological nitrogen fixation, such as trees like Acacia albida and Leucaena leucocephala in west Africa (Kang et al., 1990; Pieri and Gething, 1992; Soussana et al., 2010)—is an important option to reduce net emissions from the livestock sector. In addition, recycling of livestock manure in a whole-farm perspective (Petersen et al., 2007) can reduce the input of fertilizers in croplands.
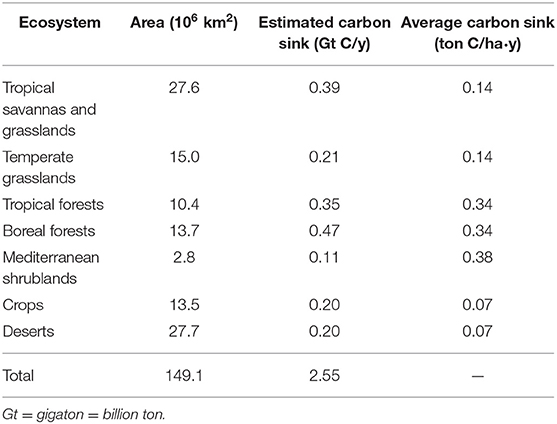
Table 2. Global land area under grasslands and the estimates of C sequestration (Adapted from Grace et al., 2006; Lal, 2008).
Adaptation and mitigation of climate change in the livestock sector requires translating of science into action by policy interventions that remove barriers to implementing proven technologies (Smith et al., 2007). Appropriate policy interventions are especially important in developing countries for achieving sustainable management of rangeland because of ecologically fragile and climatologically harsh environments. In India, for example, total annual CH4 emissions, estimated at 9–10 Tg (Tg = teragram = 1 million ton) from enteric fermentation and animal waste (Sirohi and Michaelowa, 2007), can be reduced by appropriate policy interventions such as payments for provisioning of ecosystem services.
The goal of enhancing and sustaining agricultural production for meeting the needs of the growing population while reducing the environmental footprint of agriculture necessitates local and site-specific integration of cropping with livestock systems. Soil C sequestration and decrease in gaseous emissions are in accord with SDG #13 of the U.N. Therefore, site-specific technologies for integrating livestock with crops and trees (Table 1) are needed to: (i) better moderate coupled biogeochemical cycles and reduce fluxes of pollutants into the atmosphere and the hydrosphere, (ii) create a more diversified and structured landscape mosaic that supports diverse habitats, and (iii) enhance capacity of the system to adapt to extreme events associated with climate change and alterations in the socio-economic and human dimensions (Lemaire et al., 2014). It is precisely in this context that management of grasslands can strengthen the coupled cycling of carbon (C) with those of H2O, N, P, and S within vegetation, soil organic matter (SOM) stock and soil biota in general, but the soil microbial biomass in particular (Lemaire et al., 2014).
The schematic in Figure 2 depicts the pathways of decreasing the environmental footprint of livestock products. Conceptually, choosing a livestock product with a lower emission footprint for a diet would reduce the overall negative impact on climate and the environment. The environmental footprint of a dietary product can be expressed in three ways (de Vries and de Boer, 2010): (i) per kg of product, (ii) per kg of protein, and (iii) per kg of average daily intake of each livestock product. Based on the lifecycle analysis (LCA) of 16 studies conducted in OECD (Organization for Economic Cooperation and Development) countries, de Vries and de Boer (2010) determined that the land and energy use and the GWP for 1 kg of product followed the order of beef > pork > poultry. This order was based on differences in feed efficiency, enteric CH4 emission, and reproduction rates. Similar trends were reported by (Eshel et al., 2014).
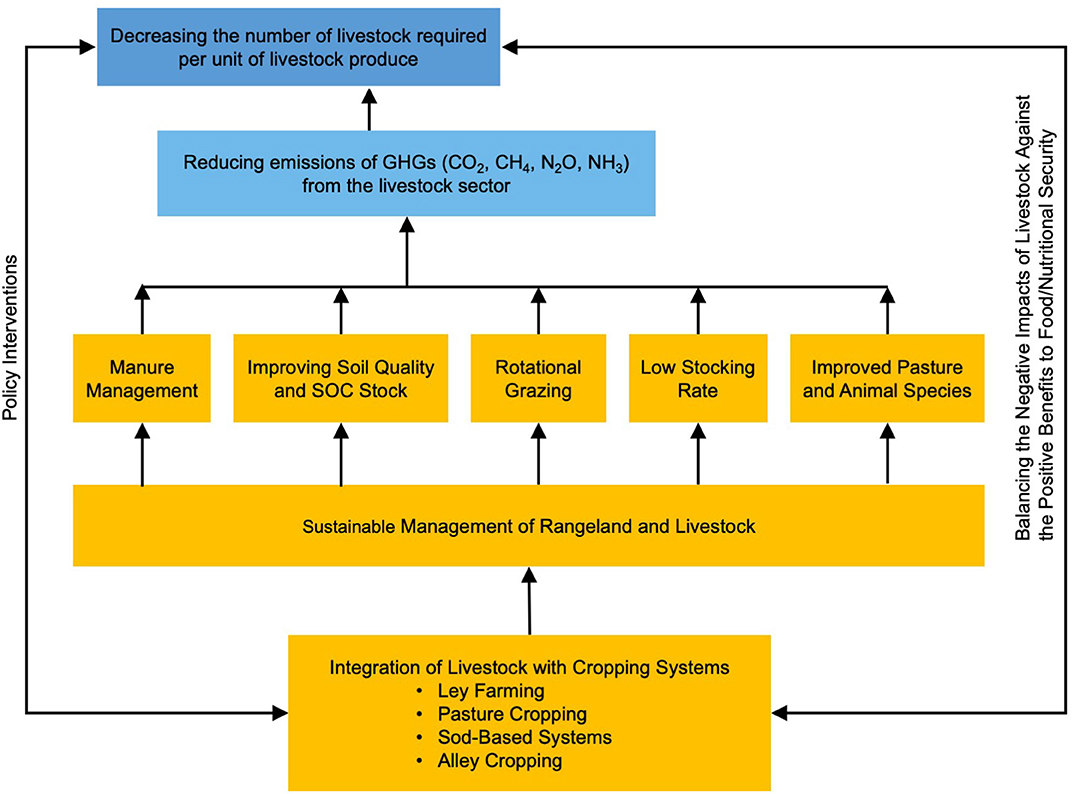
Figure 2. A flow chart depicting the integration of livestock with arable land use for decreasing the number of livestock required (SOC, soil organic carbon; GHGs, greenhouse gases).
Emissions of all gases (CO2, CH4, N2O) are used to compute CO2 equivalents (Lal, 2004). Direct emissions of CH4 and N2O in the livestock sector must be reduced. In this context, a multiple GHG perspective must be adopted (Figure 3) because CH4 has a GWP of 21 and N2O of 310. Because of the high GWP of CH4 in both confined and grazing systems, steps must be taken to develop credible methods of measuring CH4 emission by ruminants (Hill et al., 2016), and to reduce enteric fermentation by ruminants (Grossi et al., 2018). Precision feeding, matching feed intake with the need of the animal (Gerber et al., 2013a), and the choice of forages can also reduce the gaseous footprint. For example, the combination of highly digestible forages (Haque, 2018; van Gastelen et al., 2019) that contain secondary compounds such as tannins (Roca-Fernández et al., 2020) can also reuce methane emissions. The multiple GHG perspective is an important strategy that can address the potential pollution swapping—a reduction in one gas can lead to emission of another (Gerber et al., 2013a). Thus, a full accounting of all GHGs is required (Soussana et al., 2007).
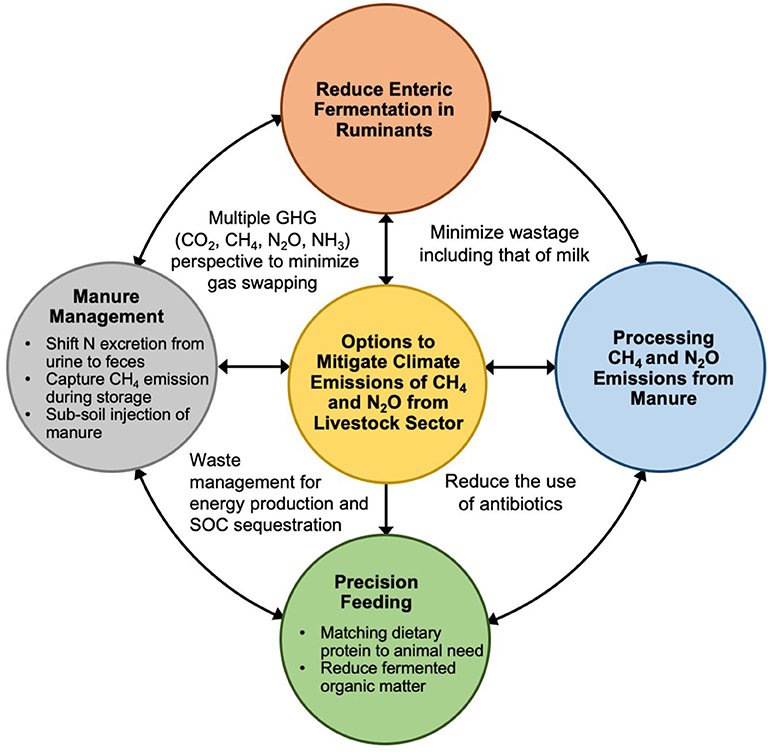
Figure 3. Measures to reduce emissions of greenhouse gases (GHGs) from the livestock sector (SOC, soil organic carbon).
Improved Management of Livestock in the Tropics
Livestock are an important component of agroecosystems in the tropics and adopting innovative livestock/farming approaches can enhance production and reduce environmental footprints. Judiciously combining crops with livestock within the same landscape has numerous co-benefits (Gil et al., 2015). For example, ley farming (Carberry et al., 1996; McCown, 1996), involving light grazing of legumes grown in rotation with crops, is a pertinent strategy for integrating crops and livestock. Built on the concept of ley farming, pasture cropping is a farmer-initiated concept of sowing a winter-active cereal into a summer-active native perennial pasture (Millar and Badgery, 2009). Self-regenerating annual legume pastures (Puckridge and French, 1983) can enhance soil fertility and increase cereal yield, along with more forage for sheep and cattle production. Ley farming, developed in Southern Australia since the 1930s, is also relevant to similar regions in Sub-Saharan Africa, South/Central Asia, and the Caribbean. However, soil/site specific choices of legumes and grazing patterns/intensity must be identified.
The numerous benefits of ley farming include (Bell et al., 2010): (i) enhancing soil N for the next crop, (ii) sequestering SOC and off-setting emissions, (iii) controlling weeds and other pests, (iv) minimizing risks of runoff, soil erosion, and deep drainage, (v) increasing livestock production, and (vi) sustaining crop yield. However, several challenges exist. Successfully implementing ley farming includes a critical appraisal of the following (Bell et al., 2010): (i) addressing difficulties with pasture establishment, (ii) suppressing/removing pasture plants before seeding crops, and (iii) reducing competition for water and some plant nutrients. Site-specific choice of pasture species is critical.
Integrating livestock with cropland and forestland can also be a prudent complimentary strategy. For example, growing Acacia albida (Faidherbia albida) as a permanent tree crop on farmlands (cereals, vegetables, and livestock) is a traditional agroforestry system in Sub-Saharan Africa (Poschen, 1986; Weil and Mughogho, 1993; Wanyancha et al., 1994). Faidherbia sp. has been widely used for enhancing soil fertility and as a source of shade and shelter for livestock in Sub-Saharan Africa (Pieri and Gething, 1992).
Widespread adoption of integrated systems can reduce the risks of rangeland degradation, as seen in China (Hou et al., 2008). India provides an example of how integrated systems can reduce land area under pasture. With 2.3% of the global land area, India supports 18% of the human and 11% of the world's livestock population: the latter consists of 536 million animals and 740 million poultry in 2019, which are raised on only 12.3 M ha of land under permanent pastures and grazing land (TAAS, 2019).
Successfully integrating crops with livestock has numerous economic, ecological, and other benefits (Figure 4), especially in developing countries of the tropics (Herrero et al., 2013). Important among these are: (i) creating another income stream for farmers and alleviating rural poverty (De Haan et al., 2001), (ii) developing a safety net for the poor and especially women farmers, (iii) enhancing assets for farmers, and (iv) alleviating malnourishment (Figure 4). However, livestock need additional land, water, nutrients, and forage resources. Therefore, judicious management of the growth of this sector is critical, especially for reducing environmental footprints. These technical dimensions must be objectively considered within the context of institutional support (market) and the human dimensions (Tarawali et al., 2011).
Conclusions
Intensive farming, which is designed to produce large amounts of economic food to meet the demands of the growing and increasingly affluent human population by using high inputs on small areas, has its merits and demerits. Intensification of crops and livestock systems have drastically increased per capita food production since the 1960s. However, the environmental footprint of livestock sector must be reduced by decreasing soil degradation, increasing water and nutrient use efficiency, reducing eutrophication of water, decreasing pollution of air, and minimizing the risks to global warming. Despite the successes in food production, there are 820 M people vulnerable to undernourishment and more than 2 B to malnourishment caused by the deficiency of protein, micro-nutrients and vitamins. The proportion of vulnerable population may increase as a result of the COVID-19 pandemic. Thus, the objective of sustainable agriculture is to adopt technologies that increase production, reduce the environmental footprint of food production systems (IPBES, 2019; IPCC, 2019; UNEP, 2019), and also minimize any risks of diseases and infections through intensive livestock farming (Sigsgaard and Balmes, 2017; Smit and Heederik, 2017).
A feasible option to produce the required amount of nutritious food while restoring and sustaining the environment is through site-specific integration of livestock with crops and trees. Such an approach of eco-intensification would simultaneously achieve several overlapping and interconnected SDGs including #2 (Zero Hunger), #3 (Good Health and Wellbeing), #6 (Clean Water and Sanitation), #13 (Climate Action) and #15 (Life on Land). Ignoring such an option would aggravate risks of environmental pollution, exacerbate perpetuation of natural ecosystems, increase harmful interactions between humans and the wildlife, and even aggravate the frequency and intensity of tragedies such as the COVID-19 pandemic (Lal, 2020b). Some recommendations of the Conference of Parties (COP) of the United Nations Framework Convention to Combat Climate Change (UNFCCC) are also in accord with the strategies of integrating livestock with crops and trees. Examples of these are the “4 Per 1,000” initiative launched at COP21 in Paris in 2015 and “Adapting African Agriculture“of COP 22 in Marrakech (Lal, 2019, 2020a). The scientific community and land managers should seize the opportunity to adopt innovative options such as those outlined in this article and promote sustainable agricultural practices which reconcile the need for producing more and nutritious food with the absolute necessity of improving the environment. Integrating livestock with crops and trees can reduce direct non-CO2 emissions and achieve the COP21 mitigation goal of limiting global warming to 2°C.
These efforts can be enhanced through research priorities identified by The Committee on Consideration for the Future of Animal Science Research (NAS, 2015). They include: (1) identifying appropriate mixes of intensification and extensification required to simultaneously increase production and reduce environmental footprints in different regions throughout the world, (2) enhancing sustainability of medium- and smaller-scale producers, (3) developing policy interventions to optimize demand for animal products, and (4) evaluating environmental impacts of diverse livestock-based production systems.
Author Contributions
The author confirms being the sole contributor of this work and has approved it for publication.
Conflict of Interest
The author declares that the research was conducted in the absence of any commercial or financial relationships that could be construed as a potential conflict of interest.
References
Bajracharya, R. M., Lal, R., and Kimble, J. M. (1998). “Long-term tillage effects on soil organic carbon distribution in aggregates and primary particle fractions of two Ohio soils,” in Management of Carbon Sequestration in Soil Advances in Soil Science, eds B. Lal, R. Kimble, J. M. Follett, and R. F. Stewart (Boca Raton, FL: CRC Press), 113–123.
Bateki, C. A., Cadisch, G., and Dickhoefer, U. (2019). Modelling sustainable intensification of grassland-based ruminant production systems: a review. Glob. Food Sec. 23, 85–92. doi: 10.1016/j.gfs.2019.04.004
Bell, L., Lawrence, J., Sustainable, C., Aprsu, E., Mara, B. O., and Qdpi, D. K. (2010). Ley Pastures–Their Fit in Cropping Systems. CSIRO Sustain. Ecosyst. Available online at: https://grdc.com.au/resources-and-publications/grdc-update-papers/tab-content/grdc-update-papers/2010/09/ley-pastures-their-fit-in-cropping-systems (accessed March 10 2020).
Broom, D. M., Galindo, F. A., and Murgueitio, E. (2013). Sustainable, efficient livestock production with high biodiversity and good welfare for animals. Proc. R. Soc. B Biol. Sci. 280:2025. doi: 10.1098/rspb.2013.2025
Carberry, P. S., McCown, R. L., Muchow, R. C., Dimes, J. P., Probert, M. E., Poulton, P. L., and Dalgliesh, N. P. (1996). Simulation of a legume ley farming system in Northern Australia using the agricultural production systems simulator. Austr. J. Exp. Agr. 36, 1037–1048. doi: 10.1071/EA9961037
Chaplot, V., Dlamini, P., and Chivenge, P. (2016). Potential of grassland rehabilitation through high density-short duration grazing to sequester atmospheric carbon. Geoderma 271, 10–17. doi: 10.1016/j.geoderma.2016.02.010
Dalibard, C. (1995). “Livestock's contribution to the protection of the environment,” in World Animal Review, ed J. Diouf (Food and Agriculture Organization of the United Nations), 104–112. Available online at: http://www.fao.org/3/v8180t/v8180T14.htm
De Haan, C., Van Veen, T. S., Brandenburg, B., Gauthier, J., Le Gall, F., Mearns, R., et al. (2001). Livestock Development : Implications for Rural Poverty, the Environment, and Global Food Security (English). Directions in Development. Washington, DC: The World Bank. doi: 10.1596/0-8213-4988-0
de Vries, M., and de Boer, I. J. M. (2010). Comparing environmental impacts for livestock products: a review of life cycle assessments. Livest. Sci. 128, 1–11. doi: 10.1016/j.livsci.2009.11.007
Devi Kanniah, K., Beringer, J., and Hutley, L. B. (2010). The comparative role of key environmental factors in determining savanna productivity and carbon fluxes: a review, with special reference to northern Australia. Prog. Phys. Geogr. Earth Environ. 34, 459–490. doi: 10.1177/0309133310364933
Doreau, M., Corson, M. S., and Wiedemann, S. G. (2012). Water use by livestock: a global perspective for a regional issue? Anim. Front. 2, 9–16. doi: 10.2527/af.2012-0036
Eshel, G., Shepon, A., Makov, T., and Milo, R. (2014). Land, irrigation water, greenhouse gas, and reactive nitrogen burdens of meat, eggs, and dairy production in the United States. Proc. Natl. Acad. Sci. U.S.A. 111, 11996–12001. doi: 10.1073/pnas.1402183111
Falkenmark, M. (2003). “Water cycle and people: water for feeding humanity,” in Land Use and Water Resources Research, Vol. 3 (University of Newcastle upon Tyne; Centre for Land Use and Water Resources Research), 1–4. doi: 10.22004/ag.econ.47862
FAO (2006). Livestock's Long Shadow: U.N. Report. Rome: Food and Agriculture Organization of the United Nations (FAO). Available online at: https://awellfedworld.org/long-shadow/
FAO (2019). FAO's Animal Production and Health Division: Meat & Meat Products. Rome, Italy: Food and Agriculture Organization of the United Nations (FAO). Available online at: http://www.fao.org/ag/againfo/themes/en/meat/home.html (accessed April 27, 2020).
FAO IFAD UNICEF, WFP, WHO. (2017). The State of Food Security and Nutrition in the World 2017: Building Resilience for Peace and Food Security. Rome Available at: http://www.fao.org/3/a-i7695e.pdf
Fike, J., Downing, A. K., and Munsell, J. (2016). Defining silvopastures : integrating tree production with forage-livestock systems for economic, environmental, and aesthetic outcomes. Virginia Coop. Ext. Publication CSES-146P. Available Online at: www.ext.vt.edu
Franzluebbers, A. J. (2007). Integrated Crop-livestock systems in the southeastern USA. Agron. J. 99, 361–372. doi: 10.2134/agronj2006.0076
Garrett, H., Kerley, M., Ladyman, K., et al. (2004). Hardwood silvopasture management in North America. Agrofor. Syst. 61, 21–33. doi: 10.1023/B:AGFO.0000028987.09206.6b
Gerbens-Leenes, P. W., Mekonnen, M. M., and Hoekstra, A. Y. (2013). The water footprint of poultry, pork and beef: A comparative study in different countries and production systems. Water Resour. Ind. 1–2, 25–36. doi: 10.1016/j.wri.2013.03.001
Gerber, P. J., Hristov, A. N., Henderson, B., Makkar, H., Oh, J., Lee, C., et al. (2013a). Technical options for the mitigation of direct methane and nitrous oxide emissions from livestock: a review. Animal 7, 220–234. doi: 10.1017/S1751731113000876
Gerber, P. J., Steinfeld, H., Henderson, B., Mottet, A., Opio, C., Dijkman, J., et al. (2013b). Tackling Climate Change Through Livestock–A Global Assessment of Emissions and Mitigation Opportunities. Rome: Food and Agricultural Organization of the United Nations (FAO). Available online at: http://www.fao.org/3/a-i3437e.pdf
Gil, J., Siebold, M., and Berger, T. (2015). Adoption and development of integrated crop-livestock-forestry systems in Mato Grosso, Brazil. Agric. Ecosyst. Environ. 199, 394–406. doi: 10.1016/j.agee.2014.10.008
Gilbert, M., Nicolas, G., Cinardi, G., Van Boeckel, T. P., Vanwambeke, S. O., Wint, G. R. W., et al. (2018). Global distribution data for cattle, buffaloes, horses, sheep, goats, pigs, chickens and ducks in 2010. Sci. Data 5:180227. doi: 10.1038/sdata.2018.227
Gill, M., Smith, P., and Wilkinson, J. M. (2010). Mitigating climate change: the role of domestic livestock. Animal 4, 323–333. doi: 10.1017/S1751731109004662
Goldstein, J. H., Caldarone, G., Duarte, T. K., Ennaanay, D., Hannahs, N., Mendoza, G., et al. (2012). Integrating ecosystem-service tradeoffs into land-use decisions. Proc. Natl. Acad. Sci. U.S.A. 109, 7565–7570. doi: 10.1073/pnas.1201040109
Grace, J., Jose, J. S., Meir, P., Miranda, H. S., and Montes, R. A. (2006). Productivity and carbon fluxes of tropical savannas. J. Biogeogr. 33, 387–400. doi: 10.1111/j.1365-2699.2005.01448.x
Grossi, G., Goglio, P., Vitali, A., and Williams, A. G. (2018). Livestock and climate change: impact of livestock on climate and mitigation strategies. Anim. Front. 9, 69–76. doi: 10.1093/af/vfy034
Haque, M. N. (2018). Dietary manipulation: a sustainable way to mitigate methane emissions from ruminants. J. Anim. Sci. Technol. 60:15. doi: 10.1186/s40781-018-0175-7
Havlík, P., Valin, H., Herrero, M., Obersteiner, M., Schmid, E., Rufino, M. C., et al. (2014). Climate change mitigation through livestock system transitions. Proc. Natl. Acad. Sci. U.S.A. 111, 3709–3714. doi: 10.1073/pnas.1308044111
Havstad, K. M., Peters, D. P. C., Skaggs, R., Brown, J., Bestelmeyer, B., Fredrickson, E., et al. (2007). Ecological services to and from rangelands of the United States. Ecol. Econ. 64, 261–268. doi: 10.1016/j.ecolecon.2007.08.005
Hawkins, H.-J. (2017). A global assessment of Holistic Planned GrazingTM compared with season-long, continuous grazing: meta-analysis findings. Afr. J. Range Forage Sci. 34, 65–75. doi: 10.2989/10220119.2017.1358213
Hedenus, F., Wirsenius, S., and Johansson, D. J. A. (2014). The importance of reduced meat and dairy consumption for meeting stringent climate change targets. Clim. Change 124, 79–91. doi: 10.1007/s10584-014-1104-5
Herrero, M., Grace, D., Njuki, J., Johnson, N., Enahoro, D., Silvestri, S., et al. (2013). The roles of livestock in developing countries. Animal 7(SUPPL1), 3–18. doi: 10.1017/S1751731112001954
Herrero, M., and Thornton, P. K. (2013). Livestock and global change: Emerging issues for sustainable food systems. Proc. Natl. Acad. Sci. U.S.A. 110, 20878–20881. doi: 10.1073/pnas.1321844111
Herrero, M., Thornton, P. K., Gerber, P., and Reid, R. S. (2009). Livestock, livelihoods and the environment: understanding the trade-offs. Curr. Opin. Environ. Sustain. 1, 111–120. doi: 10.1016/j.cosust.2009.10.003
Hill, J., McSweeney, C., Wright, A. D. G., Bishop-Hurley, G., and Kalantar-zadeh, K. (2016). Measuring methane production from ruminants. Trends Biotechnol. 34, 26–35. doi: 10.1016/j.tibtech.2015.10.004
Hou, F. J., Nan, Z. B., Xie, Y. Z., Li, X. L., Lin, H. L., and Ren, J. H. (2008). Integrated crop-livestock production systems in China. Rangel. J. 30, 221–231. doi: 10.1071/RJ08018
IPBES (2019). Global Assessment Report on Biodiversity and Ecosystem Services of the Intergovernmental Science-Policy Platform on Biodiversity and Ecosystem Services, eds E. S. Brondizio, J. Settele, S. Díaz, and H. T. Ngo Bonn (Germany: IPBES secretariat). Available online at: https://ipbes.net/global-assessment
IPCC (2019). Special Report on Climate Change and Land., eds P. R. Shukla, J. Skea, E. C. Buendia, V. Masson-Delmotte, H.-O. Pörtner, D. C. Roberts, et al. (Geneva: Intergovernmental Panel on Climate Change).
Janzen, H. H. (2011). What place for livestock on a re-greening earth? Anim. Feed Sci. Technol. 166–167, 783–796. doi: 10.1016/j.anifeedsci.2011.04.055
Jose, S., and Dollinger, J. (2019). Silvopasture: a sustainable livestock production system. Agrofor. Syst. 93, 1–9. doi: 10.1007/s10457-019-00366-8
Kang, B. T., Reynolds, L., and Atta-Krah, A. N. (1990). Alley Farming. Adv. Agron. 43, 315–359. doi: 10.1016/S0065-2113(08)60481-2
Kanniah, K. D., Beringer, J., and Hutley, L. B. (2013). Response of savanna gross primary productivity to interannual variability in rainfall. Prog. Phys. Geogr. Earth Environ. 37, 642–663. doi: 10.1177/0309133313490006
Kiff, L., Wilkes, A., and Tennigkeit, T. (2016). The Technical Mitigation Potential of Demand-Side Measures in the Agri-Food Sector: A Preliminary Assessment of Available Measures. CCAFS Report No. 15. Copenhagen: CGIAR Research Program on Climate Change; Agriculture and Food Security (CCAFS). Available online at: www.ccafs.cgiar.org
Kleppel, G. S. (2019). Microbial community structure in pasture and hayfield soils of the Helderberg region of New York State: a comparison of management strategies. Agroecol. Sustain. Food Syst. 43, 1031–1053. doi: 10.1080/21683565.2019.1591564
Lal, R. (2004). Carbon emission from farm operations. Environ. Int. 30, 981–990. doi: 10.1016/j.envint.2004.03.005
Lal, R. (2008). “Savannas and global climate change: source or sink of atmospheric CO2?” in Savannas, eds F. G. Faleiro and A. L. Farias Neto (Cerrados, Planaltina, DF: EMBRAPA), 81–102.
Lal, R. (2010). Managing soils and ecosystems for mitigating anthropogenic carbon emissions and advancing global food security. Bioscience 60, 708–721. doi: 10.1525/bio.2010.60.9.8
Lal, R. (2019). Promoting “4 Per Thousand” and “Adapting African Agriculture” by south-south cooperation: conservation agriculture and sustainable intensification. Soil Tillage Res. 188, 27–34. doi: 10.1016/j.still.2017.12.015
Lal, R. (2020a). Food security impacts of the “4 per Thousand” initiative. Geoderma 374:114427. doi: 10.1016/j.geoderma.2020.114427
Lal, R. (2020b). Soil science beyond COVID-19. J. Soil Water Conserv. 75, 1–3. doi: 10.2489/jswc.2020.0408A
Lemaire, G., Franzluebbers, A., Carvalho, P. C., de, F., and Dedieu, B. (2014). Integrated crop-livestock systems: strategies to achieve synergy between agricultural production and environmental quality. Agric. Ecosyst. Environ. 190, 4–8. doi: 10.1016/j.agee.2013.08.009
Maday, J. (2019). The Scientist who Debunked Livestock's Long Shadow. Drovers. Available online at: https://www.drovers.com/article/scientist-who-debunked-livestocks-long-shadow (accessed March 9 2020).
McCown, R. L. (1996). Being realistic about no-tillage, legume ley farming for the Australian semi-arid tropics. Aust. J. Exp. Agric. 36, 1069–1080. doi: 10.1071/EA9961069
Mekonnen, M. M., and Hoekstra, A. Y. (2012). A global assessment of the water footprint of farm animal products. Ecosystems 15, 401–415. doi: 10.1007/s10021-011-9517-8
Millar, G. D., and Badgery, W. B. (2009). Pasture cropping: a new approach to integrate crop and livestock farming systems. Anim. Prod. Sci. 49, 777–787. doi: 10.1071/AN09017
Munsell, J. F., and Chamberlain, J. L. (2019). Agroforestry for a vibrant future: connecting people, creating livelihoods, and sustaining places. Agrofor. Syst. 93, 1605–1608. doi: 10.1007/s10457-019-00433-0
NAS (2015). Global Considerations for Animal Agriculture Research. in Critical Role of Animal Science Research in Food Security and Sustainability, National Academy of Sciences and National Research Council. (Washington, DC: The National Academies Press), 215–309. Available online at: https://www.ncbi.nlm.nih.gov/books/NBK285723/ (accessed March 10 2020).
Okali, C., and Sumberg, J. E. (1985). Sheep and goats, men and women: household relations and small ruminant development in southwest Nigeria. Agric. Syst. 18, 39–59. doi: 10.1016/0308-521X(85)90056-3
O'Mara, F. P. (2012). The role of grasslands in food security and climate change. Ann. Bot. 110, 1263–1270. doi: 10.1093/aob/mcs209
Pelletier, N., and Tyedmers, P. (2010). Forecasting potential global environmental costs of livestock production 2000-2050. Proc. Natl. Acad. Sci. U.S.A. 107, 18371–18374. doi: 10.1073/pnas.1004659107
Petersen, S. O., Sommer, S. G., Béline, F., Burton, C., Dach, J., Dourmad, J. Y., et al. (2007). Recycling of livestock manure in a whole-farm perspective. Livest. Sci. 112, 180–191. doi: 10.1016/j.livsci.2007.09.001
Peyraud, J. L., and Peeters, A. (2016). “The role of grassland based production system in the protein,” in The Multiple Roles of Grassland in the European Bioeconomy: Proceedings of the 26th General Meeting of the European Grassland Federation, Vol. 21. (Trondheim: Grassland Science in Europe), 29–43. Available online at: https://www.europeangrassland.org/fileadmin/documents/Infos/Printed_Matter/Proceedings/EGF2016.pdf
Peyraud, J. L., Taboada, M., and Delaby, L. (2014). Integrated crop and livestock systems in Western Europe and South America: a review. Eur. J. Agron. 57, 31–42. doi: 10.1016/j.eja.2014.02.005
Pieri, C. J. M. G., and Gething, P. (1992). Fertility of soils: a future for farming in the West African Savannah. Berlin: Springer-Verlag, Heidelberg. doi: 10.1007/978-3-642-84320-4
Pingali, P. L. (2012). Green revolution: Impacts, limits, andthe path ahead. Proc. Natl. Acad. Sci. U.S.A. 109, 12302–12308. doi: 10.1073/pnas.0912953109
Pitesky, M. E., Stackhouse, K. R., and Mitloehner, F. M. (2009). “Chapter 1 clearing the air. livestock's contribution to climate change,” in Advances in Agronomy, ed D. L. Sparks (Oxford: Academic Press Inc), 1–40. doi: 10.1016/S0065-2113(09)03001-6
Poschen, P. (1986). An evaluation of the Acacia albida-based agroforestry practices in the Hararghe highlands of Eastern Ethiopia. Agrofor. Syst. 4, 129–143. doi: 10.1007/BF00141545
Provenza, F. D., Kronberg, S. L., and Gregorini, P. (2019). Is grassfed meat and dairy better for human and environmental health? Front. Nutr. 6:26. doi: 10.3389/fnut.2019.00026
Puckridge, D. W., and French, R. J. (1983). The annual legume pasture in cereal-Ley farming systems of southern Australia: a review. Agric. Ecosyst. Environ. 9, 229–267. doi: 10.1016/0167-8809(83)90100-7
Reay, D., and Reay, D. (2019). “Climate-smart milk,” in Climate-Smart Food (Edinburgh: Palgrave Pivot, Cham), 49–66. doi: 10.1007/978-3-030-18206-9_5
Reisinger, A., and Clark, H. (2018). How much do direct livestock emissions actually contribute to global warming? Glob. Chang. Biol. 24, 1749–1761. doi: 10.1111/gcb.13975
Ritchie, H., and Roser, M. (2019). Micronutrient Deficiency. Our World Data. Available online at: https://ourworldindata.org/micronutrient-deficiency?utm_medium=syndication&utm_source=scribd (accessed April 27 2020).
Roca-Fernández, A. I., Dillard, S. L., and Soder, K. J. (2020). Ruminal fermentation and enteric methane production of legumes containing condensed tannins fed in continuous culture. J. Dairy Sci. 103, 7028–7038. doi: 10.3168/jds.2019-17627
Rojas-Downing, M. M., Nejadhashemi, A. P., Harrigan, T., and Woznicki, S. A. (2017). Climate change and livestock: Impacts, adaptation, and mitigation. Clim. Risk Manag. 16, 145–163. doi: 10.1016/j.crm.2017.02.001
Rosegrant, M. W., Fernandez, M., Sinha, A., Alder, J., Ahammad, H., Fraiture, C., et al. (2009). “Looking into the future for agriculture and AKST(Agricultural Knowledge Science and Technology),” in Agriculture at a Crossroads: The Global Report, International Assessment of Agricultural Knowledge Science and Technology for Development (IAASTD) (Washington, DC: Island Press), 307–37. Available online at: https://cgspace.cgiar.org/handle/10568/37336 (accessed March 11 2020).
Rutledge, K., and McDaniel, M. (2011). Domestication. Natl. Geogr. Mag. Available online at: https://www.nationalgeographic.org/encyclopedia/domestication/ (accessed March 9 2020).
Scherer, L., and Verburg, P. H. (2017). Mapping and linking supply- and demand-side measures in climate-smart agriculture. A review. Agron. Sustain. Dev. 37:66. doi: 10.1007/s13593-017-0475-1
Schlink, A. C., Nguyen, M. L., and Viljoen, G. J. (2010). Water requirements for livestock production: a global perspective. Rev. Sci. Tech. 29, 603–619. doi: 10.20506/rst.29.3.1999
Shahbandeh, M. (2019). Cattle Population Worldwide 2012-2019. Statista. Available online at: https://www.statista.com/statistics/263979/global-cattle-population-since-1990/ (accessed March 9 2020).
Sigsgaard, T., and Balmes, J. (2017). Environmental effects of intensive livestock farming. Am. J. Respir. Crit. Care Med. 196, 1092–1093. doi: 10.1164/rccm.201706-1075ED
Sirohi, S., and Michaelowa, A. (2007). Sufferer and cause: indian livestock and climate change. Clim. Change 85, 285–298. doi: 10.1007/s10584-007-9241-8
Smit, L. A. M., and Heederik, D. (2017). Impacts of intensive livestock production on human health in densely populated regions. GeoHealth 1, 272–277. doi: 10.1002/2017GH000103
Smith, P., Haberl, H., Popp, A., Erb, K. H., Lauk, C., Harper, R., et al. (2013). How much land-based greenhouse gas mitigation can be achieved without compromising food security and environmental goals? Glob. Chang. Biol. 19, 2285–2302. doi: 10.1111/gcb.12160
Smith, P., Martino, D., Cai, Z., Gwary, D., Janzen, H., Kumar, P., et al. (2007). Policy and technological constraints to implementation of greenhouse gas mitigation options in agriculture. Agric. Ecosyst. Environ. 118, 6–28. doi: 10.1016/j.agee.2006.06.006
Snow, V. O., Rotz, C. A., Moore, A. D., Martin-Clouaire, R., Johnson, I. R., Hutchings, N. J., et al. (2014). The challenges - and some solutions - to process-based modelling of grazed agricultural systems. Environ. Model. Softw. 62, 420–436. doi: 10.1016/j.envsoft.2014.03.009
Soussana, J. F., Allard, V., Pilegaard, K., Ambus, P., Amman, C., Campbell, C., et al. (2007). Full accounting of the greenhouse gas (CO2, N2O, CH4) budget of nine European grassland sites. Agric. Ecosyst. Environ. 121, 121–134. doi: 10.1016/j.agee.2006.12.022
Soussana, J. F., and Lemaire, G. (2014). Coupling carbon and nitrogen cycles for environmentally sustainable intensification of grasslands and crop-livestock systems. Agric. Ecosyst. Environ. 190, 9–17. doi: 10.1016/j.agee.2013.10.012
Soussana, J. F., Tallec, T., and Blanfort, V. (2010). Mitigating the greenhouse gas balance of ruminant production systems through carbon sequestration in grasslands. Animal 4, 334–350. doi: 10.1017/S1751731109990784
Steinfeld, H., Gerber, P., Wassenaar, T. D., F. A., O, Castel, V., Rosales, M., et al. (2006). Livestock's Long Shadow: Environmental Issues and Options. Rome: Food and Agriculture Organization of the United Nations.
Stige, L. C., Stave, J., Chan, K. S., Ciannelli, L., Pettorelli, N., Glantz, M., et al. (2006). The effect of climate variation on agro-pastoral production in Africa. Proc. Natl. Acad. Sci. U.S.A. 103, 3049–3053. doi: 10.1073/pnas.0600057103
TAAS ICAR ILRI, CGIAR. (2019). Efficient Land Use and Integrated Livestock Development - A Road Map. New Dehli, India: Trust for Advancement of Agricultural Sciences (TAAS). Available online at: https://www.taas.in/documents/pub-activity-39.pdf
Tarawali, S., Herrero, M., Descheemaeker, K., Grings, E., and Blümmel, M. (2011). Pathways for sustainable development of mixed crop livestock systems: taking a livestock and pro-poor approach. Livest. Sci. 139, 11–21. doi: 10.1016/j.livsci.2011.03.003
Teague, W. R., Dowhower, S. L., Baker, S. A., Haile, N., DeLaune, P. B., and Conover, D. M. (2011). Grazing management impacts on vegetation, soil biota and soil chemical, physical and hydrological properties in tall grass prairie. Agric. Ecosyst. Environ. 141, 310–322. doi: 10.1016/j.agee.2011.03.009
The Economist (2011). Global Livestock Counts: Counting Chickens. Econ. – Graph. Detail. Available online at: https://www.economist.com/graphic-detail/2011/07/27/counting-chickens
Thornton, P. K. (2010). Livestock production: recent trends, future prospects. Philos. Trans. R. Soc. B Biol. Sci. 365, 2853–2867. doi: 10.1098/rstb.2010.0134
Thornton, P. K., van de Steeg, J., Notenbaert, A., and Herrero, M. (2009). The impacts of climate change on livestock and livestock systems in developing countries: a review of what we know and what we need to know. Agric. Syst. 101, 113–127. doi: 10.1016/j.agsy.2009.05.002
UN (2019a). World Population Prospects 2019: Data Booklet (ST/ESA/SER.A/424). Rome: United Nations, Department of Economic and Social Affairs, Population Division. Available Online at: https://population.un.org/wpp/Publications/Files/WPP2019_DataBooklet.pdf
UN (2019b). World Population Prospects 2019: Highlights (ST/ESA/SER.A/423). Rome: United Nations, Department of Economic and Social Affairs, Population Division. Available online at: https://population.un.org/wpp/Publications/Files/WPP2019_Highlights.pdf
UNEP (2019). Emission Gap Report 2019. Nairobi: United Nations Environment Programme. Available Online at: https://wedocs.unep.org/bitstream/handle/20.500.11822/30797/EGR2019.pdf?sequence=1&isAllowed=y
USDA (2020). National Agroforestry Center. Available Online at: https://www.fs.usda.gov/nac/
USDA-NRCS (2020). Alley Cropping. Available Online at: https://www.nrcs.usda.gov/wps/portal/nrcs/detailfull/national/landuse/forestry/sustain/guidance/?cid=nrcsdev11_009300 (accessed April 27, 2020).
van Gastelen, S., Dijkstra, J., and Bannink, A. (2019). Are dietary strategies to mitigate enteric methane emission equally effective across dairy cattle, beef cattle, and sheep? J. Dairy Sci. 102, 6109–6130. doi: 10.3168/jds.2018-15785
Wanyancha, J. M., Mills, W. R., and Gwaze, D. P. (1994). Genetic variation in Acacia albida (Faidherbia albida) and its agroforestry potential in Zimbabwe. For. Ecol. Manage. 64, 127–134. doi: 10.1016/0378-1127(94)90286-0
Weil, R. R., and Mughogho, S. K. (1993). Nutrient cycling by acacia albida (syn. Faidherbia albida) in agroforestry systems. Technol. Sustain. Agric. Trop. 56, 97–108. doi: 10.2134/asaspecpub56.c8
Whitley, R., Beringer, J., Hutley, L. B., Abramowitz, G., De Kauwe, M. G., Evans, B., et al. (2017). Challenges and opportunities in land surface modelling of savanna ecosystems. Biogeosciences 14, 4711–4732. doi: 10.5194/bg-14-4711-2017
Yáñez-Ruiz, D. R., Morgavi, D., Misselbrook, T., Melle, M., Dreijere, S., Aes, O., et al. (2018). Mini-paper: Feeding Strategies to Reduce Methane and Ammonia Emissions. EIP-AGRI Focus Group: Reducing Livestock Emissions From Cattle Farming. Available online at: https://ec.europa.eu/eip/agriculture/sites/agri-eip/files/fg18_mp_feeding_strategies_2017_en.pdf
Yitbarek, M. B. (2019). Livestock and livestock product trends by 2050: review. Int. J. Anim. Res. 4:20. doi: 10.28933/IJAR-2019-07-2305
Keywords: gaseous emissions, food security, ecological footprint, sustainable development goals, waste management, farming systems
Citation: Lal R (2020) Integrating Animal Husbandry With Crops and Trees. Front. Sustain. Food Syst. 4:113. doi: 10.3389/fsufs.2020.00113
Received: 17 March 2020; Accepted: 23 June 2020;
Published: 29 July 2020.
Edited by:
Fred Provenza, Utah State University, United StatesReviewed by:
Scott Lloyd Kronberg, Northern Great Plains Research Laboratory (USDA-ARS), United StatesGary S. Kleppel, University at Albany, United States
Copyright © 2020 Lal. This is an open-access article distributed under the terms of the Creative Commons Attribution License (CC BY). The use, distribution or reproduction in other forums is permitted, provided the original author(s) and the copyright owner(s) are credited and that the original publication in this journal is cited, in accordance with accepted academic practice. No use, distribution or reproduction is permitted which does not comply with these terms.
*Correspondence: Rattan Lal, bGFsLjEmI3gwMDA0MDtvc3UuZWR1