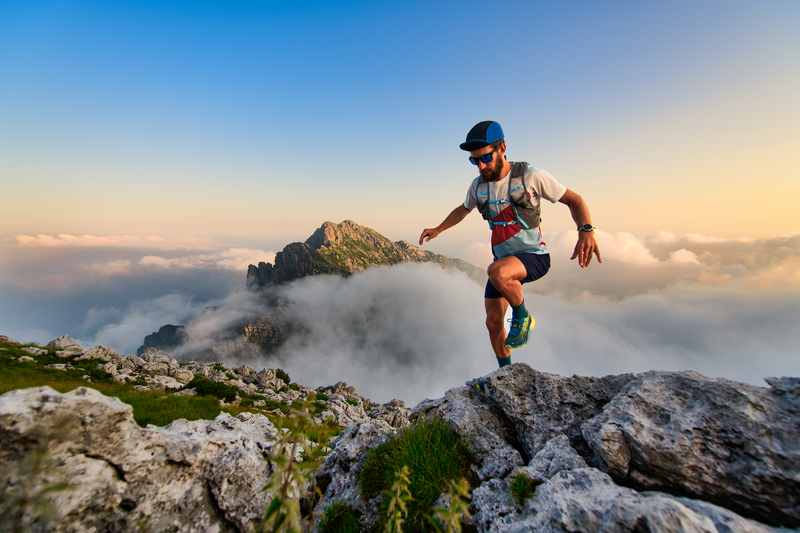
94% of researchers rate our articles as excellent or good
Learn more about the work of our research integrity team to safeguard the quality of each article we publish.
Find out more
ORIGINAL RESEARCH article
Front. Sustain. Food Syst. , 24 July 2020
Sec. Sustainable Food Processing
Volume 4 - 2020 | https://doi.org/10.3389/fsufs.2020.00111
This article is part of the Research Topic Structured Edible Oil: Towards a New Generation of Fat Mimetics View all 8 articles
Numerous studies conducted have shown a direct relationship between the high consumption of saturated and trans-fats and the risk of suffering from cardiovascular diseases, diabetes, and different cancers. Oleogels, with a suitable lipid profile of mono-, poly-unsaturated fatty acids, and similar functionality to traditional solid fat, can be a healthy alternative in food formulation. The aim of this study is to develop edible oleogels with a healthy and stable lipid profile, using the emulsion-template approach and hydrocolloids as oleogelators. Oleogels were developed from sunflower oil and sunflower oil with a high content of monounsaturated acids, using hydroxypropylmethylcellulose (HPMC) and xanthan gum (XG) as oleogelators. The influence of two drying conditions (60°C for 24 h and 80°C for 10 h 30 min) along with the composition of the oil on the structural, physical, and oxidative stability of oleogels were studied. All oleogels presented a stable network and high physical stability with oil losses <14% after 35 days of storage. Rheological properties showed that oleogels displayed a low frequency dependent and G′ > 105 Pa related to solid gel-like behavior. Oleogels made with sunflower oil rich in monounsaturated fatty acids resulted in higher oxidative stability, with those developed at drying temperatures of 80°C for 10 h 30 min having a greater structural and physical stability.
Food products such as chocolate, ice cream, meat, butters, margarine, and bakery products, are formulated with considerable amounts of solid fats, rich in saturated and/or trans-fatty acids. Solid fats have a key role in improving quality attributes such as mouthfeel and texture. Several studies have reported the relationship between the negative cardiovascular effects and the increased consumption of saturated and trans-fatty acids (Mozaffarian and Clarke, 2009; Morenga and Montez, 2017). Therefore, authorities have regulated or provided some suggestions to limit consumption of many food products formulated with a large amount of saturated and/or trans-fats (Health Canada, 2012; Food and Drug Administration (FDA), 2015; European Union (EU), 2019). Thus, the food industry and food scientists show great interest to find new strategies and product formulations with a better nutritional profile, trans-fat free, low content in saturated fatty acids, and a high content in unsaturated fats (Moghtadaei et al., 2018; Pehlivanoglu et al., 2018; Luo et al., 2019).
Oleogels have gained popularity for their potential application in cosmetic and pharmaceutical industries (Vintiloiu and Leroux, 2008; Bastiat and Leroux, 2009) and with food processing (Singh et al., 2017). Oleogelation allows structuring high concentration liquid oil (>90%) into a “gel-like” system with viscoelastic properties (Rogers et al., 2009).
In many of these oleogels, gelation is achieved by using low molecular weight organogelators (LWOG) such as hydroxylated fatty acids (Rogers et al., 2008), waxes (Lim et al., 2017; Martins et al., 2017), and lecithin (Bodennec et al., 2016). Besides LWOG, there are structured systems where liquid oil is organized into a polymer network. Within polymer gelation, cellulose derivative, ethylcellulose (EC) is a non-aqueous gelator with the ability to produce oleogels using a direct approach (Laredo et al., 2011; Zetzl et al., 2012; Giacintucci et al., 2018). The most common limitations of the EC oleogels are the poor oxidative stability because of the high temperatures (>135–140°C) required to induce the polymer EC gelation (Gravelle et al., 2012). Therefore, using hydrocolloid-based oleogelators including different sources of proteins (Patel et al., 2015; de Vries et al., 2017) and polysaccharides like celluloses ethers, methylcellulose (MC) (Patel et al., 2014a; Tanti et al., 2016a,b; Meng et al., 2018a), and hydroxypropylmethylcellulose (HPMC) (Patel et al., 2013; Oh and Lee, 2018; Oh et al., 2019; Bascuas et al., 2020), have attracted noticeable research attention. Hydrocolloids are widely used in food because of their commercial availability, large production, and low cost (Scholten, 2019; Abdolmaleki et al., 2020).
HPMC is a surface-active amphiphilic biopolymer and can be adsorbed to the oil droplet, protecting the oil droplets, thus, decreasing the amount of oil available for separation (Wollenweber et al., 2000; Li et al., 2013). Moreover, the addition of thickening agents, like XG, has shown an increase of the emulsion stability through bulk phase viscosity enhancement and interaction between the polysaccharides (Meng et al., 2018b; Encina-Zelada et al., 2019). Since HPMC and XG have a predominantly hydrophilic characteristic, their dispersibilities are limited in non-polar solvents. To overcome this problem, HPMC and XG must first be hydrated in an aqueous solution. Foam-template and emulsion-template are the most indirect methods used in structuring edible oils with hydrocolloids. In the foam-template approach, a water soluble cellulose derivative is foamed and freeze-dried to create a porous structure and has been shown to absorb a large amount of oil (Patel et al., 2013). However, freeze-drying is an expensive and time-consuming technique (do Vale Morais et al., 2016). The emulsion-template approach, first prepared by Romoscanu and Mezzenga (2006) using proteins, comprises an indirect multi-step process. In this method, first, an oil-in-water emulsion is produced as a template stabilized by a combination of water soluble biopolymers. Second, the water phase is removed to drive the structure formation; finally, the dried product is homogenized to obtain an oleogel. In both methods, as the oil binding is purely physical, it is necessary to shear the oil-sorbed polymer obtain a strong gel (Patel et al., 2013; Oh et al., 2019).
Sunflower oil is one of the most attractive vegetable oils used by food industry and is one of the most ingested worldwide, with a domestic consumption of 18.07 million metric tons during 2018–2019 (United States Department of Agriculture (USDA), 2020). Because of its low cost and high overall acceptability, sunflower oil has been used to produce oleogels (Yang et al., 2017; Jiang et al., 2018; Okuro et al., 2018; Tavernier et al., 2018). However, the predominant unsaturated fatty acids present in sunflower oils are susceptible to oxidation (Kozłowska and Gruczynska, 2018). Patel et al. (2014b) structured sunflower oil using MC and XG into solid-like oleogels using the emulsion-template approach; the drying of the emulsion in the oven (80°C for 32 h) gave oleogels with a poor oxidative stability. Developing strategies to improve the oil oxidative stability without influencing its nutritional and sensory properties, while maintaining the feasibility of use by food industry, represents an important advance in the quality of the oleogels made with hydrocolloids. Therefore, to improve the oxidative stability of oleogels, different strategies could optimize the processing conditions and the oil composition of the oleogel, favoring monounsaturated fatty acids (MUFA) with a longer oxidation induction period (Lee et al., 2007). It would be interesting to investigate the impact of using high MUFA oils and different processing conditions not only on the chemical stability, but also on the structural properties of oleogels based on water-soluble food polymers.
The objective of this work is to structure sunflower oil and sunflower oil with a high monounsaturated fatty acid content using the emulsion-template method, with HPMC and XG as oleogelators, to achieve oleogels with high structural, physical, and oxidative stability. For this, the influence of processing conditions and oil composition on the structure, physical, and chemical properties of oleogel will be compared and analyzed.
Hydroxypropylmethylcellulose (HPMC “K4M”; 4000 cP) was provided by Dow Chemical Company (Midland, MI, United States) and xanthan gum (XG; Satiaxine CX 931) by Cargill R & D (Vilvoorde, Belgium). Water (Bezoya, Segovia, Spain, with a calcium content 6.32 mg L−1), refined sunflower oil (fatty acids composition: SFA: 13, MUFA: 23, PUFA: 64, Consum, España), and high oleic sunflower oil (fatty acids composition: SFA: 10, MUFA: 65, PUFA: 25, Carrefour, España) were purchased in local supermarkets.
Based on the procedures described by Patel et al. (2014a) with some modifications, we prepared oleogels using emulsion-template method, HPMC (1 g) was dispersed in 38.4 g cold water and mixed using a stirrer (Heildolph RZR 1, Schwabach, Germany) at 1,010 rpm for 30 min, the resulting aqueous solution was stored at 8°C overnight. Subsequently, 0.6 g of XG was added to the HPMC solution and stirred (Heildolph RZR 1, Schwabach, Germany) for 5 min at 1,010 rpm, 60 g of oil was then added and homogenized (Ultraturrax T18, IKA, Germany) at 13,000 rpm for 6 min. The emulsions were spread on aluminum foil and dried in an oven (KB115, BINDER, Germany) using two different drying conditions: 80°C for 10 h 30 min, and 60°C for 24 h. These were the minimum times needed to reach constant dry weight (moisture: 2.31 ± 0.532% at 60°C; 1.59 ± 0.112% at 80°C) at the indicated conditions. The dried products were ground in a grinder (Moulinex A320R1, Paris, France) for 4 s to produce the oleogels. Four oleogels S60 (sunflower oil and drying at 60°C), SH60 (high oleic sunflower oil and drying at 60°C), S80 (sunflower oil and drying at 80°C), and SH80 (high oleic sunflower oil and drying at 80°C) were prepared in triplicate.
The microstructure of oleogels was studied by optical microscopy with a Nikon Eclipse 80i optical microscope (Nikon Co., Ltd., Tokyo, Japan) and incorporated camera (ExwaveHAD, model No. DXC-190, Sony Electronics Inc., Park Ridge, New Jersey, USA. UU.). The oleogels were cut with a cryostat (CM 1950, Leica) to obtain 20 μm thick sections that were placed on a glass slide. These sections were visualized by polarized light and by clear field microscopy using 2% Sudan as a staining agent to study the lipid fraction. The images were captured and stored at 1,280 × 1,024 pixels using the microscope software (NIS-Elements M, Version 4.0, Nikon, Tokyo, Japan).
Determination was made by measuring the percentage of oil migration over 35 days at 20°C, using the method of Doan et al. (2016) with modifications. The weight of released oil was measured at intervals of 1, 7, 14, 21, 28, and 35 days. A funnel and filter paper was positioned above an Erlenmeyer flask collecting the dripping liquid oil from the oleogels. The weight of the funnel, the filter paper, and the Erlenmeyer flask were measured (M1). Then 10 g of oleogel was weighed (M3) and set into the funnel. Samples were removed at each time interval with a flat, small spatula. The weight of the funnel, the filter paper, and the flask with the liquid oil released was measured again (M2). The results were expressed as g oil loss per 100 g oleogel, calculated using Equation 1 and were measured in triplicate for each sample.
The experimental data were fitted to a first-order equation using Solver software (Microsoft Excel):
where OLmax is the value of OL (oil loss) at sufficiently long (infinite) time, k is the kinetic constant, and t is the chosen time.
Peroxide values (PV) and specific absorption in the visible ultraviolet (k232 and k270) were used to study the oxidative stability of the oleogels during storage. The PV was analyzed using the acetic acid/chloroform solution method, according to Cho and Lee (2015), and k232 and k270 were determined according to ISO 3656:2011 (ISO, 2011), using a UV-VIS spectrophotometer (UV-VIS spectrophotometer, 1000, CECIL, UK). All the samples were stored at 20°C for 35 days and were evaluated every 7 days.
The rheological behavior of oleogels was evaluated using small amplitude oscillatory shear in a controlled stress rheometer [AR-G2, TA Instruments (Crawley, England)] with a Peltier heating system. A 20 mm diameter plate-plate sensor geometry with a serrated surface and a 1.5 mm gap was used. The oleogels rested for a 10 min equilibration time after reaching the measurement position.
Stress sweeps were conducted at a frequency of 1 Hz to measure the extent of the linear viscoelastic response. Frequency sweeps from 0.1 to 10 Hz at a stress wave amplitude (100 Pa) inside the linear region were performed. Storage modulus (G′), loss modulus (G″), and tan δ (G″/G′) values were recorded. The testing temperature was always 20°C.
Results were statistically analyzed using analysis of variance (ANOVA) with the least significant differences (LSD) calculated at a level of significance p < 0.05. Statistical analyses were conducted using XLStat 2019 (Addinsoft, Barcelona, España).
Figure 1 shows that the oleogel S60 constitutes a polymeric network that extends, forming branches that compartmentalize and trap fat globules (Figure 1A). However, accumulations of free and unstructured fat can also be seen (Figure 1E). This may be because of a coalescence phenomenon between fat globules, likely because the network formed by the structuring agents has not resisted the drying process and has not physically trapped all the fat globules (Figure 1E). Camino et al. (2009) and Wollenweber et al. (2000) studied the role of HPMC as a fat structuring agent; they found that this hydrocolloid could adsorb at the surface of fat globules, forming a viscoelastic multilayer structure because of a train loop tail conformation. In contrast, Patel et al. (2014a) studied the role of XG on the structure of oleogels, finding that XG can increase the viscosity of the emulsion by improving its stability in combination with MC during the drying process. In fact, they observed oil leakage on emulsions stabilized by using only cellulose derivates. Here, in the S80 oleogel, the hydrocolloids form a homogeneous network where most of the fat globules remain trapped (Figure 1F). In this oleogel structuring agents have a more homogeneous distribution and more structured fat (Figure 1B) than in the oil of S60 (Figure 1E) is observed. In oleogel SH60 the polymeric network formed by hydrocolloids is observed distributed throughout the oleogel (Figures 1C,G), surrounding the fat globules (Figure 1G). However, unstructured fat and coalescence phenomena can also be observed. In oleogel SH80 hydrocolloids show a uniform and homogeneous network (Figure 1D) that surrounds and traps fat globules (Figure 1H). In SH80 the fat appears more structured than in the other oleogels, probably because the hydrocolloid network has a greater stability. Comparing the same type of oil oleogels undergone drying at 80°C, more stable polymeric networks exist, capable of retaining fat globules than those dried at 60°C. Probably, drying the emulsion at 60°C, which is slower than at 80°C, favors the attractive interactions between the xanthan gum helix (XG-XG), and weakens HPMC-XG interactions. This would lead to the formation of a weaker network when drying is carried out at 60°C if compared to 80°C (Carnali, 1992; Lapasin and Pricl, 1995). Monounsaturated sunflower oil seems to be retained more in the polymeric network than sunflower oil.
Figure 1. Optical microscopy micrographs of oleogels prepared with sunflower (S) and high oleic sunflower oil (SH), and developed by conventional drying at 60°C (S60, SH60) and 80°C (S80, SH80), under (A–D) polarized light, (E–H) bright field with Sudan 2%.
The physical stability of oleogels is related to their ability to retain oil during storage. Figure 2 shows the proportion of oil loss at 1, 7, 14, 21, 28, and 35 days of storage at 20°C of all oleogels developed in this study. The experimental data were fitted to a first-order equation. Exponential decay kinetic model presented a R2 more than 0.99 for all cases (Table 1), evidencing the excellent fit between the formula and the experimental data.
Figure 2. Oil loss curves for oleogels prepared with sunflower (S) and high oleic sunflower oil (SH), and developed by conventional drying at 60°C (S60, SH60) and 80°C (S80, SH80).
Freshly made oleogels showed no oil losses, however the greatest loss of oil took place in all the oleogels studied during the first 24 h of storage. k values were significantly lower (p < 0.05) for S60 and SH60, indicating a slower loss of oil for these samples within the first 24 h. However, OLmax values indicated that oleogels S80 and SH80 presented significantly lower (p < 0.05) amount of oil exuded over the whole storage, while S60 and SH60 oleogels had the highest values, without significant differences (p < 0.05) between them. Other authors (Meng et al., 2018c) also developed stable oleogels with soybean oil using the emulsion-template method, varying HPMC concentration (0.2–1%) and constant XG concentration (0.3%); however, the stability during storage was not studied. They suggested that the formation of semi-crystalline structure due to the hydrogen bonding within the chains of the polysaccharides, resulted in oleogels with high physical stability, especially from oleogel made with highest HPMC concentration. As explained before, a stronger network would be obtained when drying at higher temperature; the strength of the network would be helping to prevent the oil release from the oleogel.
Table 2 shows the peroxide values (PV) of the oleogels stored for 35 days at 20°C. The upper limit for the PV of fresh oils is <15–20 meq kg−1 (Codex Alimentarius, 2001; Gómez-Alonso et al., 2004). PV > 20 correspond to very poor-quality fats and oils, which normally would have significant off flavors (O'Keefe and Pike, 2010). The PV of sunflower and high oleic sunflower oils used in this study were 3.24 ± 0.07 and 6.27 ± 0.29 meq kg−1, respectively. These values indicate that both oils are suitable.
The oleogels S60, S80, SH60, and SH80 had initial PV of 12.86 ± 0.51, 16.22 ± 0.68, 7.78 ± 0.76, and 7.69 ± 0.07, respectively (Table 2). The process of making oleogels causes oxidative degradation of the oil, mainly in those formulated with sunflower oil. However, all the oleogels studied showed adequate initial PV, while oleogels made with high oleic sunflower oil (SH60 and SH80) presented significantly lower PV (p < 0.05) throughout storage, but with no significant differences (p < 0.05) between them. The oleogels made with sunflower oil (S60 and S80) had the highest PV, with S60 showing the highest PV throughout storage.
Regarding the behavior of each oleogel, those made with sunflower oil (S60 and S80) showed a significant increase (p < 0.05) in PV throughout the entire storage, reaching values >40 meq kg−1 at the end of the storage period. The oleogel made with high oleic sunflower oil (SH60), showed significant increases (p < 0.05) of PV on storage days 14 and 28 and SH80 on days 14, 21, and 35, with both oleogels reaching PV around 15 meq kg−1 at the end of the storage period.
The specific UV extinction coefficient (k) at 232 and 270 nm is an estimator of fat deterioration. The k232 is normally considered an indicator of primary oxidation products, such as hydroperoxides and conjugated dienes. While k270 measures conjugated trienes (as secondary oxidation products), ketones, aldehydes, and primary oxidation products of linolenic acid (Maskan and Bagci, 2003; Tavakoli et al., 2017). The specific absorption values in the visible ultraviolet (k232 and k270) of the oils used in the production of oleogels were 3.40 ± 0.28 and 3.51 ± 0.14 for sunflower oil, and 2.15 ± 0.02 and 0.78 ± 0.04 for high oleic sunflower. These values agree with Albi et al. (1997), who reported a k232 and k270 value for fresh sunflower oil of 4.70 and 3.15, and 2.32 and 0.83 for high oleic sunflower.
The oleogels S60, S80, SH60, and SH80 had initial values of k232 and k270 of 3.92 ± 0.50, 2.86 ± 0.01; 4.50 ± 0.23, 5.33 ± 0.15; 2.51 ± 0.05, 0.80 ± 0.08; and 2.20 ± 0.09, 0.86 ± 0.06; respectively (Table 3). The SH60 and SH80 oleogels showed significantly lower values of k232 and k270 (p < 0.05) throughout the storage period, without significant differences (p < 0.05) between them. While the S60 and S80 oleogels presented the highest values, with S60 showing the highest k232 values, and the lowest k270 values. In oleogels made with sunflower oil (S60 and S80) a significant increase (p < 0.05) of k232 values was observed throughout storage, while the k270 values remained stable while increasing at the end of storage, specifically on day 28.
In oleogels made with oleic-rich sunflower oil (SH60 and SH80), a significant increase in k232 values was observed every 14 days of storage, but from day 28 these values remained stable. The values of k270 remained stable in oleogel SH60 until day 7 of storage and after increased, mainly on day 14. In oleogel SH80 the values of k270 remained stable until day 21; on day 28 there was a significant increase (p < 0.05), but remained stable until day 35. The oleogels made with oleic-rich sunflower oil were more stable to oxidation than the oleogels made with sunflower oil. The high content in MUFA in SH60 and SH80 oleogels would be helping to delay oxidation, as MUFA have longer induction periods than PUFA, which are major components in S60 and S80 oleogels.
To better understand the structural changes, the dynamic mechanical spectra were studied. The viscoelastic properties of the samples are shown in Figure 3. Over the entire frequency range studied (0.1–10 Hz) (Figure 3A), a low G′ and G″ dependence with frequency was observed, suggesting and all oleogels presented an elastic modulus (G′) higher than viscous modulus (G″), indicating a typical behavior of solid gels (Guenet, 2016). Luo et al. (2019) obtained comparable low frequency dependent results from camellia-oil based oleogels structured with tea polyphenol-palmitate and varying citrus pectin concentration. Meng et al. (2018c) dried soybean emulsions in a vacuum drying oven at 90°C and analyzed the frequency sweep of oleogels formulated with 0.2–1% of HPMC (400 and 1500 cP) and 0.3% XG, showing similar dependency on frequency, but a lower value of G′, which may be for the viscosity of HPMC (4000 cP) and the higher XG concentration (0.6%) used in our study. The loss tangent (tan δ = G″/G′) of oleogels, showed a similar trend, with a tan δ ≈ 0.1, confirming the existence of a strong internal network (Figure 3B). These results were corroborated analyzing statistical differences at 1 Hz (Table 4) with no significant differences between oleogels for the dynamic modulus (G′ and G″) and tan δ values higher for the S80 and SH80 oleogels, indicating that these samples had lower viscoelastic behavior.
Figure 3. Frequency sweep (A), and tan δ as a function of frequency (B) for oleogels prepared with sunflower (S) and high oleic sunflower oil (SH), and developed by conventional drying at 60°C (S60, SH60) and 80°C (S80, SH80).
It is possible to develop physically, chemically, and structurally stable oleogels using sunflower oil and sunflower oil with a high content monounsaturated fatty acids, using HPMC and XG as structuring agents with the emulsion-template method and the drying conditions 60°C for 24 h and 80°C for 10 h 30 min). The stability of oleogels during storage is influenced by the composition of the oil and the drying conditions of the oleogel process. However, oleogels made with sunflower oil high in monounsaturated fatty acids show better oxidative stability during storage than those made with sunflower oil, regardless of the drying conditions used. Furthermore, drying at 80°C for 10 h 30 min generates oleogels with greater structural and physical stability than drying at 60°C for 24 h, regardless of the oil composition of the oleogel. Therefore, the oxidative stability of the oleogel is greatly influenced by the type of oil, improving when the oil has a high content of monounsaturated fatty acids, still, the drying conditions (time and temperature) have a marked influence on the structural and physical stability.
The raw data supporting the conclusions of this article will be made available by the authors, without undue reservation.
SB: experimental work and writing the draft manuscript. AS: investigation and methodology. IH: writing–review and editing and funding acquisition. AQ: supervision and funding acquisition. All authors contributed to the article and have approved the final manuscript.
The authors would like to thank Universitat Politècnica de València by FPI-UPV 2017 grant and the project RTI2018-099738-B-C22 from the Ministerio de Ciencia, Innovacion y Universidades.
The authors declare that the research was conducted in the absence of any commercial or financial relationships that could be construed as a potential conflict of interest.
Special thanks goes to Phillip John Bentley for assistance in correcting the English manuscript.
Abdolmaleki, K., Alizadeh, L., Nayebzadeh, K., Hosseini, S. M., and Shahin, R. (2020). Oleogel production based on binary and ternary mixtures of sodium caseinate, xanthan gum, and guar gum: optimization of hydrocolloids concentration and drying method. J. Texture Stud. 51, 290–299. doi: 10.1111/jtxs.12469
Albi, T., Lanzón, A., Guinda, A., Pérez-Camino, M. C., and León, M. (1997). Microwave and conventional heating effects on some chemical parameters of edible fats. Agric. Food Chem. 45, 3000–3003. doi: 10.1021/jf970168c
Bascuas, S., Hernando, I., Moraga, G., and Quiles, A. (2020). Structure and stability of edible oleogels prepared with different unsaturated oils and hydrocolloids. Int. J. Food Sci. Technol. 55, 1458–1467. doi: 10.1111/ijfs.14469
Bastiat, G., and Leroux, J. C. (2009). Pharmaceutical organogels prepared from aromatic amino acid derivatives. J. Mater. Chem. 19, 3867–3877. doi: 10.1039/b822657a
Bodennec, M., Guo, Q., and Rousseau, D. (2016). Molecular and microstructural characterization of lecithin-based oleogels made with vegetable oil. RSC Adv. 6, 47373–47381. doi: 10.1039/C6RA04324K
Camino, N. A., Pérez, O. E., Sanchez, C. C., Rodriguez Patino, J. M., and Pilosof, A. M. R. (2009). Hydroxypropylmethylcellulose surface activity at equilibrium and adsorption dynamics at the air-water and oil-water interfaces. Food Hydrocoll. 23, 2359–2368. doi: 10.1016/j.foodhyd.2009.06.013
Carnali, J. O. (1992). Gelation in physically associating biopolymer systems. Rheol. Acta 31, 399–412. doi: 10.1007/BF00701120
Cho, Y. J., and Lee, S. (2015). Extraction of rutin from Tartary buckwheat milling fractions and evaluation of its thermal stability in an instant fried noodle system. Food Chem. 176, 40–44. doi: 10.1016/j.foodchem.2014.12.020
Codex Alimentarius (2001). Codex Standard for Named Vegetable Oils. Codex Alimentarius CX-STAN 210-1999, Vol. 8. Rome: Secretariat of the Joint FAO/WHO Food Standards Programme; FAO, 11–25.
de Vries, A., Gomez, Y. L., Van der Linden, E., and Scholten, E. (2017). The effect of oil type on network formation by protein aggregates into oleogels. RSC Adv. 7, 11803–11812. doi: 10.1039/C7RA00396J
do Vale Morais, A. R., Alencar, É. D. N., Júnior, F. H. V., de Oliveira, C. M., Marcelino, H. R., Barratt, G., et al. (2016). Freeze-drying of emulsified systems: a review. Int. J. Pharm. 503, 102–114. doi: 10.1016/j.ijpharm.2016.02.047
Doan, C. D., Patel, A. R., Tavernier, I., De Clercq, N., Van Raemdonck, K., de Walle, D. V., et al. (2016). The feasibility of wax-based oleogel as a potential co-structurant with palm oil in low-saturated fat confectionery fillings. Eur. J. Lipid Sci. Technol. 118, 1903–1914. doi: 10.1002/ejlt.201500172
Encina-Zelada, C. R., Cadavez, V., and Teixeira, J. A. (2019). Optimization of quality properties of gluten-free bread by a mixture design of Xanthan, Guar, and Hydroxypropyl Methyl cellulose gums. Foods 8:156. doi: 10.3390/foods8050156
European Union (EU) (2019). Commission Regulation (EU) 2019/649 of 24 April 2019 amending Annex III to Regulation (EC) No 1925/2006 of the European Parliament and of the Council as regards trans-fat, other than trans-fat naturally occurring in fat of animal origin. J. Euro. Union. 2019, 17–20.
Food and Drug Administration (FDA) (2015). Final Determination Regarding Partially Hydrogenated Oils (Removing Trans-Fat). Available online at: (March 9, 2020).
Giacintucci, V., Di Mattia, C. D., Sacchetti, G., Flamminii, F., Gravelle, A. J., Baylis, B., et al. (2018). Ethylcellulose oleogels with extra virgin olive oil: the role of oil minor components on microstructure and mechanical strength. Food Hydrocoll. 84, 508–514. doi: 10.1016/j.foodhyd.2018.05.030
Gómez-Alonso, S., Mancebo-Campos, V., Salvador, M. D., and Fregapane, G. (2004). Oxidation kinetics in olive oil triacylglycerols under accelerated shelf-life testing (25–75°C). Eur. J. Lipid Sci. Technol. 106, 369–375. doi: 10.1002/ejlt.200300921
Gravelle, A. J., Barbut, S., and Marangoni, A. G. (2012). Ethylcellulose oleogels: manufacturing considerations and effects of oil oxidation. Food Res. Int. 48, 578–583. doi: 10.1016/j.foodres.2012.05.020
Guenet, J. M. (2016). Organogels: Thermodynamics, Structure, Solvent Role, and Properties. Cham: Springer International Publishing.
Health Canada (2012). It's Your Health-Fats: The Good The Bad and The Ugly. Available online at: https://www.hc-sc.gc.ca/hl-vs/alt_formats/pdf/iyh-vsv/med/fats-gras-eng.pdf (accessed March 9, 2020).
ISO (2011). Animal and Vegetable Fats and Oils. Determination of Ultraviolet Absorbance Expressed as Specific UV Extinction. Geneva: International Organization for Standardization.
Jiang, Y., Liu, L., Wang, B., Sui, X., Zhong, Y., Zhang, L., et al. (2018). Cellulose-rich oleogels prepared with an emulsion-templated approach. Food Hydrocoll. 77, 460–464. doi: 10.1016/j.foodhyd.2017.10.023
Kozłowska, M., and Gruczynska, E. (2018). Comparison of the oxidative stability of soybean and sunflower oils enriched with herbal plant extracts. Chem. Pap. 72, 2607–2615. doi: 10.1007/s11696-018-0516-5
Lapasin, R., and Pricl, S., (eds). (1995). “Rheology of polysaccharide systems,” in Rheology of Industrial Polysaccharides: Theory and Applications (Boston, MA: Springer) 250–494. doi: 10.1007/978-1-4615-2185-3
Laredo, T., Barbut, S., and Marangoni, A. G. (2011). Molecular interactions of polymer oleogelation. Soft Matter 7, 2734–2743. doi: 10.1039/c0sm00885k
Lee, J., Lee, Y., and Choe, E. (2007). Temperature dependence of the autoxidation and antioxidants of soybean, sunflower, and olive oil. Eur. Food Res. Technol. 226, 239–246. doi: 10.1007/s00217-006-0532-5
Li, X., Al-Assaf, S., Fang, Y., and Phillips, G. O. (2013). Competitive adsorption between sugar beet pectin (SBP) and hydroxypropyl methylcellulose (HPMC) at the oil/water interface. Carbohydr. Polym. 91, 573–580. doi: 10.1016/j.carbpol.2012.08.075
Lim, J., Jeong, S., Oh, I. K., and Lee, S. (2017). Evaluation of soybean oil-carnauba wax oleogels as an alternative to high saturated fat frying media for instant fried noodles. LWT Food Sci. Technol. 84, 788–794. doi: 10.1016/j.lwt.2017.06.054
Luo, S. Z., Hu, X. F., Jia, Y. J., Pan, L. H., Zheng, Z., Zhao, Y. Y., et al. (2019). Camellia oil-based oleogels structuring with tea polyphenol-palmitate particles and citrus pectin by emulsion-templated method: preparation, characterization, and potential application. Food Hydrocoll. 95, 76–87. doi: 10.1016/j.foodhyd.2019.04.016
Martins, A. J., Cerqueira, M. A., Cunha, R. L., and Vicente, A. A. (2017). Fortified beeswax oleogels: effect of β-carotene on the gel structure and oxidative stability. Food Funct. 8, 4241–4250. doi: 10.1039/C7FO00953D
Maskan, M., and Bagci, H. I. (2003). The recovery of used sunflower seed oil utilized in repeated deep-fat frying process. Eur. Food Res. Technol. 218, 26–31. doi: 10.1007/s00217-003-0794-0
Meng, Z., Qi, K., Guo, Y., Wang, Y., and Liu, Y. (2018a). Physical properties, microstructure, intermolecular forces, and oxidation stability of soybean oil oleogels structured by different cellulose ethers. Eur. Food Res. Technol. 120:1700287. doi: 10.1002/ejlt.201700287
Meng, Z., Qi, K., Guo, Y., Wang, Y., and Liu, Y. (2018b). Effects of thickening agents on the formation and properties of edible oleogels based on hydroxypropyl methyl cellulose. Food Chem. 246, 137–149. doi: 10.1016/j.foodchem.2017.10.154
Meng, Z., Qi, K., Guo, Y., Wang, Y., and Liu, Y. (2018c). Macro-microstructure characterization and molecular properties of emulsion-templated polysaccharide oleogels. Food Hydrocoll. 77, 17–29. doi: 10.1016/j.foodhyd.2017.09.006
Moghtadaei, M., Soltanizadeh, N., and Goli, S. A. H. (2018). Production of sesame oil oleogels based on beeswax and application as partial substitutes of animal fat in beef burger. Food Res. Int. 108, 368–377. doi: 10.1016/j.foodres.2018.03.051
Morenga, L. T., and Montez, J. M. (2017). Health effects of saturated and trans-fatty acid intake in children and adolescents: systematic review and meta-analysis. PLoS ONE 12:e0186672. doi: 10.1371/journal.pone.0186672
Mozaffarian, D., and Clarke, R. (2009). Quantitative effects on cardiovascular risk factors and coronary heart disease risk of replacing partially hydrogenated vegetable oils with other fats and oils. Eur. J. Clin. Nutr. 63, S22–S33. doi: 10.1038/sj.ejcn.1602976
Oh, I., Lee, J. H., Lee, H. G., and Lee, S. (2019). Feasibility of hydroxypropyl methylcellulose oleogel as an animal fat replacer for meat patties. Food Res. Int. 122, 566–572. doi: 10.1016/j.foodres.2019.01.012
Oh, I. K., and Lee, S. (2018). Utilization of foam structured hydroxypropyl methylcellulose for oleogels and their application as a solid fat replacer in muffins. Food Hydrocoll. 77, 796–802. doi: 10.1016/j.foodhyd.2017.11.022
O'Keefe, S. F., and Pike, O. A. (2010). “Fat characterization,” in Food Analysis, ed S. S. Nielsen (New York, NY: Springer), 239–260. doi: 10.1007/978-1-4419-1478-1_14
Okuro, P. K., Tavernier, I., Bin Sintang, M. D., Skirtach, A. G., Vicente, A. A., Dewettinck, K., et al. (2018). Synergistic interactions between lecithin and fruit wax in oleogel formation. Food Funct. 9, 1755–1767. doi: 10.1039/C7FO01775H
Patel, A. R., Cludts, N., Bin Sintang, M. D., Lesaffer, A., and Dewettinck, K. (2014b). Edible oleogels based on water soluble food polymers: preparation, characterization, and potential application. Food Funct. 5, 2833–2841. doi: 10.1039/C4FO00624K
Patel, A. R., Cludts, N., Bin Sintang, M. D., Lewille, B., Lesaffer, A., and Dewettinck, K. (2014a). Polysaccharide-based oleogels prepared with an emulsion-templated approach. ChemPhysChem 15, 3435–3439. doi: 10.1002/cphc.201402473
Patel, A. R., Rajarethinem, P. S., Cludts, N., Lewille, B., De Vos, W. H., Lesaffer, A., et al. (2015). Biopolymer-based structuring of liquid oil into soft solids and oleogels using water-continuous emulsions as templates. Langmuir 31, 2065–2073. doi: 10.1021/la502829u
Patel, A. R., Schatteman, D., Lesaffer, A., and Dewettinck, K. (2013). A foam-templated approach for fabricating organogels using a water-soluble polymer. RSC Adv. 3, 22900–22903. doi: 10.1039/C3RA44763D
Pehlivanoglu, H., Demirci, M., Toker, O. S., Konar, N., Karasu, S., and Sagdic, O. (2018). Oleogels, a promising structured oil for decreasing saturated fatty acid concentrations: production and food-based applications. Crit. Rev. Food Sci. Nutr. 58, 1330–1341. doi: 10.1080/10408398.2016.1256866
Rogers, M. A., Wrigh, A. J., and Marangoni, A. G. (2008). Engineering the oil binding capacity and crystallinity of self-assembled fibrillar networks of 12-hydroxystearic acid in edible oils. Soft Matter 4, 1483–1490. doi: 10.1039/b803299h
Rogers, M. A., Wright, A. J., and Marangoni, A. G. (2009). Oil organogels: the fat of the future? Soft Matter 5, 1594–1596. doi: 10.1039/b822008p
Romoscanu, A. I., and Mezzenga, R. (2006). Emulsion-templated fully reversible protein-in-oil gels. Langmuir 22, 7812–7818. doi: 10.1021/la060878p
Scholten, E. (2019). Edible oleogels: how suitable are proteins as a structurant? Curr. Opin. Food Sci. 27, 36–42. doi: 10.1016/j.cofs.2019.05.001
Singh, A., Auzanneau, F. I., and Rogers, M. A. (2017). Advances in edible oleogel technologies - a decade in review. Food Res. Int. 97, 307–317. doi: 10.1016/j.foodres.2017.04.022
Tanti, R., Barbut, S., and Marangoni, A. G. (2016a). Hydroxypropyl methylcellulose and methylcellulose structured oil as a replacement for shortening in sandwich cookie creams. Food Hydrocoll. 61, 329–337. doi: 10.1016/j.foodhyd.2016.05.032
Tanti, R., Barbut, S., and Marangoni, A. G. (2016b). Oil stabilization of natural peanut butter using food grade polymers. Food Hydrocoll. 61, 399–408. doi: 10.1016/j.foodhyd.2016.05.034
Tavakoli, A., Sahari, M. A., and Barzegar, M. (2017). Antioxidant activity of Berberis integerrima seed oil as a natural antioxidant on the oxidative stability of soybean oil. Int. J. Food Prop. 20, S2914–S2925. doi: 10.1080/10942912.2017.1382509
Tavernier, I., Doan, C. D., Van der Meeren, P., Heyman, B., and Dewettinck, K. (2018). The potential of waxes to alter the microstructural properties of emulsion-templated oleogels. Eur. J. Lipid Sci. Technol. 120:1700393. doi: 10.1002/ejlt.201700393
United States Department of Agriculture (USDA) (2020). Consumption of Vegetable Oils Worldwide from 20135/2014 to 2019/2020, by Oil Type (in Million Metric Tons). Available online at: https://www.statista.com/statistics/263937/vegetable-oils-global-consumption/ (accessed March 28, 2020).
Vintiloiu, A., and Leroux, J. C. (2008). Organogels and their use in drug delivery - a review. J. Control. Release 125, 179–192. doi: 10.1016/j.jconrel.2007.09.014
Wollenweber, C., Makievski, A. V., Miller, R., and Daniels, R. (2000). Adsorption of hydroxypropyl methylcellulose at the liquid/liquid interface and the effect on emulsion stability. Colloids Surf. A: Physicochem. Eng. Asp. 172, 91–101. doi: 10.1016/S0927-7757(00)00569-0
Yang, S., Li, G., Saleh, A. S. M., Yang, H., Wang, N., Wang, P., et al. (2017). Functional characteristics of oleogel prepared from sunflower oil with β-sitosterol and stearic acid. J. Am. Oil Chem. Soc. 94, 1153–1164. doi: 10.1007/s11746-017-3026-7
Keywords: oleogelation, HPMC, xanthan gum, sunflower oil, rheological properties, peroxide value, light microscopy
Citation: Bascuas S, Salvador A, Hernando I and Quiles A (2020) Designing Hydrocolloid-Based Oleogels With High Physical, Chemical, and Structural Stability. Front. Sustain. Food Syst. 4:111. doi: 10.3389/fsufs.2020.00111
Received: 15 April 2020; Accepted: 17 June 2020;
Published: 24 July 2020.
Edited by:
Luiz Henrique Fasolin, Campinas State University, BrazilReviewed by:
Yong Wang, Jinan University, ChinaCopyright © 2020 Bascuas, Salvador, Hernando and Quiles. This is an open-access article distributed under the terms of the Creative Commons Attribution License (CC BY). The use, distribution or reproduction in other forums is permitted, provided the original author(s) and the copyright owner(s) are credited and that the original publication in this journal is cited, in accordance with accepted academic practice. No use, distribution or reproduction is permitted which does not comply with these terms.
*Correspondence: Isabel Hernando, bWloZXJuYW5AdGFsLnVwdi5lcw==
Disclaimer: All claims expressed in this article are solely those of the authors and do not necessarily represent those of their affiliated organizations, or those of the publisher, the editors and the reviewers. Any product that may be evaluated in this article or claim that may be made by its manufacturer is not guaranteed or endorsed by the publisher.
Research integrity at Frontiers
Learn more about the work of our research integrity team to safeguard the quality of each article we publish.