- 1Institute of Environmental Physics, University of Heidelberg, Heidelberg, Germany
- 2Max Planck Institute for Chemistry, Mainz, Germany
How to feed a growing global population in a secure and sustainable way? The conventional, biogenic agriculture has yet failed to provide a reliable concept which circumvents its severe environmental externalities—such as the massive use of land area, water for irrigation, fertilizer, pesticides, herbicides, and fossil fuel. In contrast, the artificial synthesis of carbohydrates from atmospheric carbon dioxide, water, and renewable energy would allow not only for a highly reliable production without those externalities, but would also allow to increase the agricultural capacities of our planet by several orders of magnitude. All required technology is either commercially available or at least developed on a lab-scale (at least for sugar). No directed research has, however, yet been conducted towards an industry-scale carbohydrate synthesis because the biogenic carbohydrate production was economically more competitive. Taking the environmental and socio-economic externalities of the conventional sugar production into account, this economical narrative has to be questioned. We estimate the production costs of artificial sugar at ~1 €/kg. While the today's spot market price for conventional sugar is about 0.3 €/kg, we estimate its total costs (including external costs) at ≥0.9 €/kg in humid regions and ≥2 €/kg in semi-arid regions. Accordingly, artificial sugar appears already today to be the less expensive way of production. The artificial sugar production allows in principle also for a subsequent synthesis of other carbohydrates such as starch. These synthetic products could directly supply a large fraction of the human calorie requirements and could be in addition used as a feedstock to microorganisms, fungi, insects, or livestock in order to enhance the sustainability of the remaining biogenic production of, e.g., fats and proteins. This manuscript aims at rising research interest and awareness for a transition to a non-agricultural and more resource-conserving way to supply carbohydrates for food and feed.
1. Introduction
Since the inception of agriculture by mankind about ten millenia ago, the basis of the food supply for the human population has been the farming of field crops. Today, the conventional agriculture occupies more than a third of all land area but nevertheless requires the massive use of water for irrigation, fertilizers, pesticides, herbicides, and fossil fuel in order to meet our demand in food (Plourde et al., 2013; FAO, 2018). Just some of the negative environmental externalities of our agricultural system are groundwater depletion, soil erosion, environmental pollution, an alarming decrease in biodiversity, and a contribution of 10–12% to the anthropogenic greenhouse gas emission fluxes (Famiglietti, 2014; IPCC, 2014a; Lu et al., 2015; Borrelli et al., 2017; Dudley and Alexander, 2017; FAO, 2017, 2019). On the one hand, these externalities are severe threads to the ecologically basis of our society and the reduction of agricultural activities to a sustainable level thus appears to be mandatory (O'Neill et al., 2018). On the other hand, the expected growths in global population and per-capita meat consumption will demand an even higher agricultural output (OECD and FAO, 2018). This dilemma becomes even worse if the ongoing climate change indeed causes a steady decline in agricultural productivity as the increasing number of droughts throughout the globe may indicate (IPCC, 2014b). With the conventional agriculture failing to provide a convincing answer to the question: how to provide a secure and sustainable food supply to a growing global population?
The predominant bottlenecks in the biogenic food supply are the extremely poor energy conversion efficiency of the plant-driven photosynthesis with typical efficiencies of 1–3% for solar-to-biomass and below 0.5% for solar-to-food (in this paper, food refers to the energy intake supplied to humans by digesting the end-product) and the enormous demand for water, amounting to 400–2,000 kg of fresh water for 1 kg of food (Rockström et al., 2007; Zhu et al., 2008; Gerbens-Leenes and Hoekstra, 2009). In contrast, the abiotic synthesis of complex organic compounds from carbon dioxide (CO2), water (H2O), and (solar/renewable) energy—also called artificial photosynthesis or power-to-X—could not only provide solar-to-food conversion efficiencies beyond 10% but is in addition virtually free of environmental externalities. In particular, it would require less than 1 kg of water to synthesize 1 kg of food. This contrast in conversion efficiencies gave rise to research interest in replacing biofuels by entirely synthetic fuels as ecologically sustainable fuels for the transportation sector or as a storage technology for renewable energy. For instance, power-to-gas synthesis of hydrogen (H2) or methane (CH4) has been installed on an industrial scale with energy conversion efficiencies of 77% and 41%, respectively (Thema et al., 2019). For the synthesis of more complex carbon compounds such as liquid hydrocarbons from atmospheric CO2, energy conversion efficiencies of up to 70% have been predicted (Graves et al., 2011; Sunfire, 2019b).
It is surprising that the numerous publications on power-to-X never mention the artificial synthesis of food, although the transition towards an artificial synthesis of food appears to be a possible solution for the dilemma highlighted above. This can be illustrated impressively by the theoretical area required to feed an adult human: The global annual average solar irradiation reaching Earth's surface is about 185 W m−2 = 16.0 MJ m−2 d−1 (Wild et al., 2013). The recommended calorie intake for an adult human varies between 7−19 MJ d−1 subject to parameters such as age, weight, gender, and physical activity (FAO, 2001). Assuming a mean calorie demand of 12 MJ d−1 (and ignoring the minor differences between the physical and physiological energy contents of food), thus in theory a ground area of just 0.75 m2 could harvest enough solar energy to feed an adult human.
The World Health Organization recommends a human diet whose calorie intake is contributed by 55–75% via carbohydrates, 15–30% via fats, and 10–15% via proteins—indicating that carbohydrates are the most important component in our food supply (WHO and FAO, 2003). Carbohydrates are—when strictly defined—compounds with a chemical formula Cm(H2O)n. The most relevant carbohydrates are monosaccharides (CH2O)n, (e.g., glucose and fructose both with n = 6) and monosaccharide polymers Cn(H2O)n-1 which are called with respect to their degree of polymerization disaccharides (e.g., sucrose and lactose both with n = 12), oligosaccharides, or polysaccharides (e.g., starch and cellulose). The abiotic monosaccharide synthesis scheme
is understood in principle and practical experience for all intermediate steps is available (see next section). The artificial synthesis of monosaccharides allows in principle also for a subsequent artificial synthesis of any monosaccharide polymer, that is of any carbohydrate (Clancy and Whelan, 1967; Seeberger and Haase, 2000; Plante et al., 2001; Seeberger and Werz, 2005; Kadokawa and Kobayashi, 2010).
To date, however, a practical use of artificial carbohydrates has been investigated only for niche applications. For instance, the National Aeronautics and Space Administration (NASA) investigated in the 1960s the artificial production of carbohydrates as possible food supply for manned space missions (see the NASA history and related references at https://history.nasa.gov/SP-202/sess5.2.htm). While those early studies apparently ceased in the 1970s, NASA has recently reanimated the investigations aiming for an efficient artificial sugar supply on Mars (NASA, 2018). Unnatural monosaccharides such as L-glucose were investigated as a possible zero-calorie sweetener in the food industry or diabetes therapy but the synthesis costs have been estimated to be not competitive to other commercially available sweetener (Vogel and Robina, 2008).
In contrast, no effort has yet been made to investigate an industry-scale production of artificial carbohydrates as direct or intermediate parts of a food supply on Earth. This ignorance was comprehensible because an economical competitiveness of artificial carbohydrates with biogenic food appeared to be absurd in the past. As of today, the commercializations of power-to-food have therefore focussed on partially artificial approaches which aim on the production of higher-priced protein, e.g., by feeding bioreactors with CH4 (potentially generated by power-to-gas processes) (Cumberlege et al., 2016; Matassa et al., 2016).
This economic narrative has to be reassessed for a future food supply. First of all, because the future imperative of a sustainable food supply will presumably cause a strong increase in the production costs for conventional carbohydrates. In addition, the expected production costs for artificial sugar have been reduced by orders of magnitude in the last decades due to decreasing costs for renewable energy, enhanced electroliser efficiencies, and enhanced CO2 direct-air-capture (DAC) technology (e.g., Lackner, 2009; Keith et al., 2018).
This paper reports a first feasibility study on the transition towards an at least partially artificial production of carbohydrates. The study is oriented along already demonstrated technologies and its techno-economic characteristics, e.g., efficiencies and costs, are taken from today or the near-future estimates. For the sake of clearness, our analysis focuses on the synthesis of monosaccharides and in particular hexoses. More specific, all quantitative estimates are conducted for glucose, which is the most important hexose in the human metabolism. The last parts of this paper discusses our findings for a broader spectrum of possible synthesis products.
2. The Formose Reaction
Although there may be several possible routes to synthesize monosaccharides from CO2 and H2O, the original and present literature concentrates on the path via formaldeyhde (CH2O) which is known as the formose reaction (Butlerow, 1861; Fischer, 1913; Breslow, 1959; Vogel and Robina, 2008). The formose reaction has raised scientific interest as a possible key step in the origin of life on Earth and several naturally abundant catalysts for the abiotic synthesis of formose products have been investigated (e.g., Lambert et al., 2010; Iqbal and Novalin, 2012; Delidovich et al., 2014; Kitadai and Maruyama, 2018; Pallmann et al., 2018). Formaldehyde can be synthesized from syngas (a mixture of carbon monoxide (CO) and H2) and syngas can be obtained, for instance, from the co-electrolysis of CO2 and H2O.
The optimum synthesis path from CO2 and H2O to, e.g., glucose is subject to future research. For this first feasibility study, we exemplify the concept by highlighting that Deng et al. (2013) demonstrated for the formose reaction scheme
an almost perfect carbon yield—that is the fraction of initial carbon atoms which are part of the desired output species—in hexoses (96%) which could be in principle further enhanced to >99%. While their set-up and reaction scheme was designed to yield branch-chained ketohexoses (which they further processed to precursors for hydrocarbons), they unintendedly also synthesized straight-chained ketohexoses such as fructose with carbon yields of about 5% at 273 K and 11% at 303 K. Though not yet demonstrated, we assume in the following that an adjusted thermodynamic and catalytic set-up allows for an almost perfect carbon yield (96%) in straight-chained hexoses. For the rest of this paper, hexose refers to straight-chain hexoses only.
From thermodynamics, the conversion of CO2(g) and H2O(l) to hexose
requires at least the differences in the free enthalpies of formation of (under standard conditions and varying between the different hexoses by several parts per thousand, e.g., ).
The total theoretical energy demand is the sum of ΔHhexose plus the energy required to extract the input resources from the environment. The theoretical minimum energy demand to extract CO2 and H2O from the local atmosphere is and (see Appendix A and e.g. Atkins et al., 2018). The energy demand to extract H2O from the environment is nevertheless typically much lower, e.g., industry-scale sea water desalination requires just (Meindertsma et al., 2010). Thus the energy demand for the water extraction is, at least in humid or coastal regions, negligible compared to the energy demand for the CO2 extraction. The total energy demand to synthesize a hexose then reads
3. Targeted Hexose Stereoisomer Synthesis
One point to watch in the abiotic synthesis of sugar is the necessity to manufacture a particular stereoisomer, as will be detailed below. (Straight-chained) hexoses can exist in 16 possible aldohexose stereoisomers and 8 possible ketohexose stereoisomers and these 24 isomers can be furthermore grouped in 12 possible pairs of enantiomers distinguished by the prefixes D- and L-. Aldohexoses and ketohexoses differ in their functional group (i.e., containing an aldehyde group or a ketone group). Stereoisomers have the same atomic constitution but different orientations of their atoms in space. Enantiomers have molecular structures which are mirror images of each other and have thus identical chemical and physical properties except for their ability to rotate plane-polarized light in opposite directions, however, their physiological properties can differ. Most life on Earth synthesizes only 6 hexoses (D-glucose, D-fructose, D-mannose, D-galactose, D-tagatose, L-sorbose), another 4 hexoses (D-allose, L-altrose, D-gulose, D-psicose) have been found in some microorganisms, but the remaining 14 hexoses have not been observed in nature. Accordingly, the metabolism of life on Earth has specialized exclusively on the biogenic stereoisomers. In particular, the metabolism can usually—and if at all—digest only one of the two possible enantiomers. Abiotic enantiomers taste identical for humans but can apparently not be digested by humans. Moreover, they may have adverse effects on the human digestion system (e.g., relaxing properties, Raymer et al., 2003) and the long-term effects of a life-long nutrition with significant amounts of unnatural hexose enantiomers on humans have not yet been studied sufficiently (Wang et al., 2017). In fact, presumably only a small fraction of microorganisms can use L-glucose as an energy source at all (Bautista et al., 2000; Shimizu et al., 2012; Fukano et al., 2018).
A mixture of hexoses synthesized via the formose reaction could contain all possible stereoisomers and could be furthermore expected to be a racemic mixture—that is it contains equal amounts in all D- and L-enantiomers. In practice, a high or even exclusive yield in a particularly targeted hexose stereoismer (or a mixture of several particular stereoisomers) has to be aimed for because a mixture containing non-digestible hexoses may be rejected as a healthy human diet due to physiological concerns. In addition, it has to be assured that no potentially poisonous substances (e.g., catalysts) are in the final product. However, biogenic carbohydrates are also potentially contaminated with toxic substances (e.g., arsenic in rice). Physiological considerations on the human diet thus need to trade off between systematically avoided and potentially added contaminations.
There are three possible approaches to face this physiological constraint: (1) The most direct approach would be the application of catalysts which yield exclusively the targeted hexose stereoisomer(s). Such catalysts have been reported, e.g., for an enantioselective synthesis of D-glyceraldehyde in the presence of suitable L-amino acids (Breslow and Cheng, 2010), the enzymatic synthesis of three- to six-carbon monosaccharides via engineered D-fructose-6-phosphate aldolase from Escherichia coli (Szekrenyi et al., 2015, who prominently mentioned the synthesis of L-glucose), and a high yield of the formose reaction in six- and seven-carbon monosaccharides in the presence of a boronic acid (Michitaka et al., 2017). Although no catalysts have yet been proposed for a synthesis of, e.g., pure D-glucose—due to the lack in research interest?—it appears to be plausible that those can be developed as well.
(2) Alternatively (in absence of or in support for a non-exclusive catalyst), an adjustment of the thermodynamic conditions during the formose reaction as well as a subsequent separation and reprocessing of non-targeted stereoisomers may result in a high yield and high degree of purity in the targeted stereoisomer(s). Numerous methods are available for a subsequent separation of enantiomers, for instance, preferential crystallization (Levilain and Coquerel, 2010; Tassinari et al., 2019), diastereomer crystallization (Kozma et al., 1995), enantioselective liquid-liquid extraction (Schuur et al., 2011), or radiation with circular polarized light (Rukhlenko et al., 2016; Sugahara et al., 2018). The simplest approach of reprocessing non-targeted stereoisomers would be to burn them and thus to recover at the very least the energy demand to extract CO2 and H2O from the environment. But also more efficient approaches such as a “cracking” of the non-targeted stereoisomers back to formaldehyde appear to be possible.
(3) Less physiological concerns may be raised when the racemic diet is fed to livestock (for the production of meat, milk, and eggs) or insects and microorganisms (both for the production of biogenic proteins) as there is no plausible mechanism how the unnatural enantiomers could affect the quality of the final food product.
Which approach is ultimately applied is determined by techno-economic considerations.
4. Energy Demand of the Artificial Sugar Synthesis
In this section, we exemplify a possible sugar synthesis plant outline (Figure 1) and estimate the energy conversion efficiency of the artificial sugar synthesis. The estimated key figures of the different process steps are summarized in Table 1. With the CO2 direct-air-capture (DAC) technology and, e.g., sea water desalination at hand, the only actual input resource of the synthesis plant is electric energy. We exclude for the sake of universality the actual realization of the energy supply from our techno-economic investigation and consider that the energy is provided externally from a base-load source. Of course, an ecologically sustainable sugar synthesis would rely on renewable energy (e.g., a mixture of solar power, wind power, and hydroelectric power combined with an energy storage in order to provide a base-load supply). The actual technology enters our comparison via the electricity costs exclusively. For a subsequent comparison of the biogenic and artificial sugar production, we assume an energy supply by solar panels with a solar-to-power efficiency of ηStP = 20%.

Figure 1. Sketch of the integrated synthesis plant compartments. The only actual input is electric energy and the output are the targeted monosaccharides.
Today, the required CO2 can be extracted directly with < 50 kJ mol−1 from the exhaust flux of coal-fired power plants or other industrial CO2 sources (Lackner, 2009; He and Hägg, 2014). In order to reach the goal of a carbon neutral industry by the mid of this century, the required CO2 has then to be extracted instead from the ambient air by DAC. While the minimum thermodynamic energy demand is = 19.5 kJ mol−1 (see Appendix A), the minimum practically required energy demand for DAC has been estimated to be (Lackner, 2009). At present, the practically required energy demand for DAC, although it has been drastically reduced within the last decade, is still around 300 kJ mol−1, e.g., Keith et al. (2018) reported for a pilot plant , of which 231 kJ mol−1 are thermal energy input. Nevertheless, further drastic efficiency enhancements can be expected in the future of this just emerging technology.
The synthesis of formaldehyde (ΔHf,0 = 570.1 kJ mol−1)
has a theoretical thermal efficiency of 84%. The mature industry-scale formaldehyde synthesis starts with the preparation of syngas (m CO + n H2), e.g., via first water electrolysis (Equation 6, ΔHf,0 = 571.6 kJ mol−1) and a subsequent reduction of CO2 via the water-gas shift reaction (Equation 7, ΔHf,0 = 41.2 kJ mol−1)
where the second process becomes exothermal at temperatures larger than 1100 K. This two-step process is well-established when using low-temperature electrolysis what comes, however, with the drawback of an overall energy conversion efficiency of just 40–80% (Thema et al., 2019).
In contrast, high-temperature co-electrolysis allows for the simultaneous synthesis of syngas from CO2 and H2O with an efficiency of 89% (Graves et al., 2011; Omojola, 2015; Zheng et al., 2017). Although high-temperature electrolisers are considered to be not yet economically competitive due to short electroliser lifetimes, their lifetime and therefore costs are expected to enhance in the near future (Schmidt et al., 2017). For instance, the German company Sunfire demonstrated a co-electroliser with an 82%-efficiency and a lifetime of >500 h (Sunfire, 2019a,b). We assume in the following that the technologically proven 89%-efficiency and the cost estimates for 2030 reported by Schmidt et al. (2017) as plausible key figures for the long-term performance of industry-scale high-temperature co-electrolysis.
The subsequent formaldehyde synthesis is industrially realized via the intermediate step of the methanol synthesis
with an energy efficiency of 56% and carbon yield of 91% (Spath and Dayton, 2003; Bahmanpour et al., 2014). The total energy efficiency of this quasi-mature formaldehyde synthesis scheme is then 50% and its carbon yield is 91%.
A direct one-step implementation of Equation (5) would omit energy-consuming intermediate steps and thus yield high energy conversion efficiencies. Research interest in such direct approaches has been reanimated recently (Bahmanpour et al., 2015; Heim et al., 2017). In particular, Nakata et al. (2014) demonstrated on a lab-scale energy efficiencies of up to for the synthesis of formaldehyde from a mixture of CO2 and sea water.
Ultimately, we do not choose a particular reaction scheme for the synthesis of hexoses from formaldehyde but assume an energy conversion efficiency which equals the theoretical thermal efficiency of 83% and a carbon yield of 96% (see above).
The total energy demand for the hexose synthesis reads
with the total efficiency ηsynthesis = ∏i = 4−7γi · ηi of the chemical synthesis (see process steps i in Table 1). The total system efficiency (where the energy demand for the water supply is neglected in the second line)
is in first approximation linear in ηsynthesis and only weakly dependent on the energy demand for the CO2 extraction. Applying the parameters from Table 1, we obtain ηsynthesis = 36% and ηsystem = 31% for the quasi-mature technology. An upper limit for the system efficiency can be estimated by applying instead and the reaction scheme demonstrated by Nakata et al. (2014) with and assuming an addition perfect carbon yield of ; corresponding to ηsystem = 73% (Figure 2).
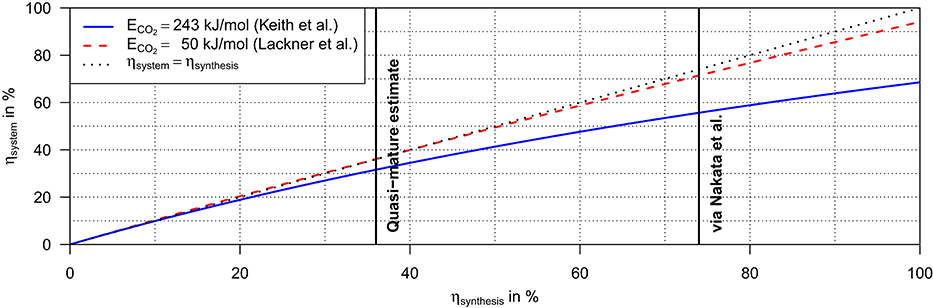
Figure 2. System efficiency of the hexose synthesis dependent on the efficiency of the synthesis 6 CO2(g) + 6 H2O(l) → (CH2O)6(s) + 6 O2(g) and the energy demand of direct air capture of 1 mole of CO2.
Accordingly, the power-to-food efficiency of the hexose synthesis range between ηsystem = 31–73%. Assuming an electricity supply by solar panels with a solar-to-power efficiency of ηStP = 20%, the total solar-to-food efficiency of the hexose synthesis would be ηStF = ηStP · ηsystem = 6−15%.
5. Production Costs of Artificial Hexoses
In this section, we estimate the production costs to produce artificial hexoses. The only actual input resource consumed by the hexose synthesis plant is electric energy (the cost of CO2 and H2O is also expressed in terms of energy consumption, as explained above). Accordingly, the production cost Chexose of artificial hexoses can be separated as
with the electricity price Cel and the overall “fixed” non-material costs C0, that is the operation and maintenance costs and the levelized capital costs. The energy content of hexose is 4.5 MWh/t so Equation (11) reads in more handy units
The fixed costs C0 predominantly sum up the non-material costs of the CO2–DAC device, the electroliser, the formaldehyde synthesis reactor, and the formose reactor and assuming a continuous full-load operation (Table 2). It is not the aim of this paper to give a detailed cost calculation but rather to find a rough estimate for the fixed costs. For the following estimates, the non-perfect carbon yields of the subsequent processes have been taken into account, that is the estimates contain already a multiplicative over-production correction factor of for the CO2–DAC device and the electroliser and of for the formaldehyde reactor. Furthermore, a conversion factor of 1 € = 1.12 US$ is applied.

Table 2. Fixed non-material costs of the required plant facilities and applying a conversion factor of 1 €= 1.12 US$.
(1) The non-material costs for the CO2–DAC device are €/thexose for the device realized by Keith et al. (2018).
(2) The costs for high-temperature co-electrolisers have been estimated for 2030 as (retrieved from Schmidt et al., 2017, and assuming ). For the following calculations we assume the lower estimate by Schmidt et al. (2017). For comparison, Graves et al. (2011) estimated costs for their integrated fuel synthesis facility corresponding to <1 US$/GJfuel corresponding to <17 €/thexose and corresponding to 17−67 €/thexose.
(3) The costs for the industrial facilities for the formaldehyde reactor and the formose reactor could be estimated from the reported non-material costs of the two-steps production scheme natural gas → syngas → methanol of about (Boulamanti and Moya, 2017). When we assume that is also a good estimate for the two-steps reaction scheme syngas → methanol → formaldehyde, the non-material costs of the formaldehyde reactor would be . As an intermediate results, our estimates indicate production costs of synthetic formaldehyde of when assuming electricity costs of Cel = 50 €/MWh. For comparison, the production costs of methanol from fossil methane are about CCH3OH = 350 €/tCH3OH (Bahmanpour et al., 2014) subject to a market price for fossil methane of roughly CCH4 = 16 €/MWh. Though the industrial conversion of methanol to formaldehyde adds further costs, it can be expected that the total production costs of “fossil” formaldehyde is significantly below our estimates for the costs of artificial formaldehyde. This comparison indicates that our estimates for the production costs of synthetic formaldehyde appears to be in a realistic range—and potentially the cheapest way to produce formaldehyde in a carbon-neutral future.
(4) The cost for the formose reactor can be separated into the facility costs for the synthesis of any hexose (or rather a mixture of hexoses) from formaldehyde and the additional costs for the targeted production of a particular hexose stereoisomer. Concerning the facility costs, the formose reactor design reported by Deng et al. (2013) appears to be at most as complicated as the formaldehyde synthesis reactor chain. Accordingly, we assume that the production of hexoses from formaldehyde may be (per carbon atom) at most as expensive as the production of formaldehyde from syngas. That is, we assume .
(5) Concerning the targeted production technology, a cost estimate appears to be speculative. For instance, the production costs of glucose (referring to D-glucose) would read
For the sake of the argument, we assume in the following that appropriate and sufficiently cheap catalysts will be available in the mid-term future. That is, we tacitly neglect in the following economic comparison but highlighted this major source of uncertainty in Table 2 and in the conclusions.
The artificial hexose synthesis does not cause significant externalities and thus its production costs represent also its total costs. For the range ηsystem = 31−73% in the system efficiency of the hexose synthesis plant estimated above, the total costs for artificial hexose (but exclusively the additive costs of the targeted stereoisomer production) would be about 0.5–1.0 €/kg or 0.8–1.6 €/kg for electricity costs of 50 or 100 €/MWh, respectively (Figure 3).
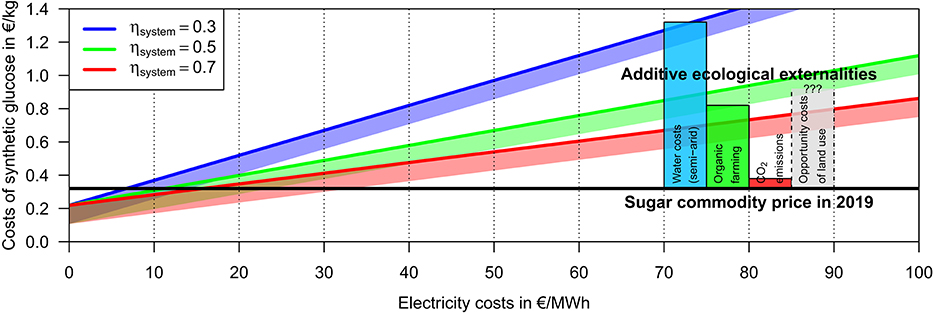
Figure 3. Estimated production costs of 1 kg synthetic glucose—exclusive the potentially non-negligible costs for the targeted hexose stereoisomer production—dependent on the electricity costs (x-axis), the non-material costs (intercept with the y-axis), and the system efficiency of the glucose synthesis plant (lines of different color). The thick colored lines indicate the expected costs for commercialized or soon commercialized technology summarized in the Tables 1, 2. The shaded polygons indicate the corresponding cost ranges when the non-material costs are further reduced down to the estimates by Graves et al. (2011). On the right side of the figure, the market price of conventional sugar and the lower estimates for costs of the ecological externalities of the conventional sugar production are indicated (see Table 3). The opportunity costs of the land use are added for qualitative completeness but its quantitative range has to been estimated.
Another approach to arrive at an estimate of the production cost for artificial sugar would be to judge from present market prices for artificial sugars which are, e.g., 31 US$/g for 25 g L-glucose and 350 US$/g for 2 g L-fructose (prices taken from www.carbosynth.com). These prices most likely primarily reflect the salary for hand-crafted goods. For instance, a discount rate of roughly 10% for any doubling in purchased mass is effectively offered—indicating the drastically increasing returns to scale of the artificial sugar production. An industry-scale automatization of the sugar synthesis thus may plausibly reduce the production costs by the four to five orders of magnitude which would be required for economical competitiveness. In fact, all processes of the proposed synthesis plant outline appear to be automatable and thus relatively inexpensive compared to the electricity costs.
6. Total Costs of the Conventional Sugar Production
The average spot market price for conventional white sugar was 0.32 €/kg in 2018 (OECD and FAO, 2018) subject to a volatility span since 2015 between 0.22–0.52 €/kg (data from NASDAQ). The spot market price is a distorted proxy for the actual production costs and consumer prices due to politically motivated subsidies and market restrictions. As of today, the minimum achieved production costs for sugar are 0.29 €/kg based on corn milling, 0.19 €/kg based on sugar beet, and 0.41 €/kg based on sugar cane (Cheng et al., 2019). The minimum consumer price for sugar lies in Germany (exclusively the value-added tax of 7%) at around 0.55 €/kg for non-organic sugar and 2.4 €/kg for organic sugar (organic refers in the following to “organic farming”). This figure includes additive costs for transportation, retail, and marketing of about Cretail = 0.23 €/kg which are plausibly independent from the actual way of production (non-organic, organic, artificial) and an additive premium of 1.85 €/kg for the production and marketing in the case of organic sugar.
An estimate of the total costs of the conventional sugar production needs to add all externalities. In the following, we estimate the additional contributions to the sugar price from a series of externalities (Table 3). The most obvious ecological externalities are the water demand, the consequences of a non-organic agriculture, the CO2 emissions, and the demand in land area.
1) The conventional production of 1 kg sugar requires kg water for sugar beets and kg water for sugar cane (weighted global averages and the span of national extremes, Gerbens-Leenes and Hoekstra, 2009). In semi-arid regions such as the Western United States, the Middle East, or Northern India, the demand in additional groundwater for irrigation is on the order of 1,000 kg water per 1 kg sugar and even the most efficient semi-arid production (Spain) requires still 500 kg groundwater. These extreme amounts of water are today usually provided by an unsustainable extraction of ancient groundwater (Aeschbach-Hertig and Gleeson, 2012; Wada, 2016; Grogan et al., 2017), thus future agriculture can there only be maintained either by long-distance transport of fresh water from humid regions or by sea water desalination. The costs of sea water desalination are today as low as 1 €/tfresh water when assuming electricity costs of 50 €/MWh from renewable energies (Advisian, 2019). Assuming that the water costs have not yet contributed significantly to the market price for sugar, the production costs of sugar would increase by 0.5−1 €/kgsugar in semi-arid regions when the water costs are internalized.
In humid regions, the larger part of the water demand is supplied for free by precipitation, however, even the most efficient regions require at least 200 kg additional groundwater (or surface water) per 1 kg sugar for irrigation and subsequent processing. These still immense amounts of water are extracted usually for free, following the economical assumption that precipitation replenishes the extracted groundwater in a sustainable way. For instance, the common groundwater extraction fees are in Germany 0.05−0.12 €/tgroundwater ~ 0.02 €/kgglucose (variation between German states excluding the city states, BUND, 2019). The German agriculture is even freed to pay these fees at all. The recent dry summers of 2018 and 2019 in Germany and Europe in general have, however, suggest that this practice may need to be changed. While today an internalization of the water costs would only negligibly increase the costs of sugar from humid regions, the climate change may impose that the water extraction costs will rise also there to significant numbers and first political initiatives aim to stop the above mentioned water subsidy for the German agriculture (Government of Mecklenburg-Vorpommern, 2018).
2) The low production costs of conventional sugar are achieved also by the increase in per-area yield due to the massive use of fertilizer, pesticides, and herbicides. The accompanying ecological externalities such as groundwater pollution and the loss in biodiversity are severe (see above) and thus a drastic reduction of the non-organic production appears to be mandatory in near future. In consequence, only organic sugar should be supplied. The production costs of non-organic sugar beets in Germany has been estimated as 25−28 €/tbeet corresponding to 0.16−0.18 €/kgsugar (Degner, 2016) and reported contract prices between beet farmers and the sugar industry have been in this range as well. In contrast to that, organic sugar beets have been contracted in Germany for the season 2019 for 105−115 €/tbeet (Südzucker, 2019). Assuming a mass yield of extracted sugar per beet of about 16% (Bundesinformationszentrum Landwirtschaft, 2018), this indicates additive farming costs of about 0.5 €/kgsugar for organic farming, at least in Germany. Remarkably, these additional farming costs are much lower than the additional consumer costs of 1.85 €/kg (see above) indicating that the marketing of organic sugar causes yet large costs due to, e.g., the smaller market volume.
3) The processing of sugar beets requires at the most efficient processing facilities about 1.3−1.5 kWh/kgsugar causing CO2 emission of 0.3−0.4 kgCO2/kgsugar (German electric energy mix) but higher numbers for older facilities with larger emissions exist (Nordzucker, 2018a,b). The energy demand of farming is in Europe on average 0.13 tons of oil equivalent per hectare utilized agricultural area (that is about 0.13 kWh/kgsugar, see next section) adding roughly another 0.05 kgCO2/kgsugar (Eurostat, 2019). The removal of CO2 from the atmosphere may currently cost at the very least 0.15 €/kgCO2 (combined CO2-DAC plus CO2 storage costs). On the one hand, such a fully-compensating CO2 tax add costs of 0.06 €/kgsugar to the conventional sugar costs. On the other hand, the above assumption of renewable electricity costs of 50 €/MWh would imply for consistence that the conventional sugar production would also operate then carbon-free and with similar electricity costs as today.
4) Sugar beet plantation occupy about 1% of the area of Germany and starch-supplying crop (e.g., cereals, potatoes, maize) plantation occupy about 18% (Bundesinformationszentrum Landwirtschaft, 2017). An artificial carbohydrate production would free (at least parts of) these areas for other uses such as enhanced local recreation areas and nature reservation, which would allow a recovery of the local biodiversity, or a more generous area supply for the agricultural production of vegetables, fruits, or livestock cultivation.
In summary, the lower limit of the total costs of conventional sugar can be estimated at about 1 €/kg for humid regions and about 2 €/kg for semi-arid regions when including just the external costs of the water demand and the environmental pollution. The (difficult to estimate) opportunity costs of its land demand further increase the total costs. In addition, the conventional sugar production causes several socio-economic externalities:
(1) Droughts and diseases can temporarily reduce the global carbohydrate supply possibly causing food commodity speculations. This temporary economical pressure can be severe to poor people in low-income countries. The yield of an artificial sugar production would be highly reliable and the installed capacities can be appropriately adopted to long-term changes in the food demand.
(2) A reliable food supply for its population is a primary geopolitical goal of any nation. Many nations therefore support their domestic agriculture with unconditional subsidies and additional drought compensations rather than relying on potentially cheaper imports. An artificial sugar production would largely avoid these dependencies and subsidies.
(3) Bad working conditions and poor pay for the employees probably also contribute to the low production costs of conventional sugar in developing countries. As quantitative illustration, Fairtrade International has established a fair trade premium of 0.06 US$/kgsugar for conventional farming and 0.08 US$/kgsugar for organic farming (Fairtrade International, 2019).
(4) A fully automatized sugar production would not further require the agricultural work force. From an economic point of view, this exclusion of the production factor work would be another positive effect of the transition towards an artificial carbohydrate supply, however, from a social point of view it could be seen as thread for enhanced unemployment rates.
(5) It has to be mentioned that the conventional sugar production leads also to additional, non-sugar biomass which has a real economical value. This positive (but relatively small) externality has to be subtracted from the total production costs.
7. Comparison of Biogenic and Artificial Sugar Production
After we listed a large set of the negative externalities of the conventional agriculture, we want to focus on the comparison of the conventional and artificial sugar production on the three central ecological differences and its contributions to their economic competitiveness: (1) the demand in water, (2) the environmental pollution caused by non-organic farming (that is by the massive use of fertilizers, pesticides, and herbicides), and (3) the demand in (fertile) land area.
We compare the above derived figures for synthetic hexose (e.g., glucose or fructose) on the one hand and biogenic sucrose (a disaccharide which is composed of glucose and fructose) on the other hand. A comparison of one particular sugar species implies additional transformation costs (from synthetic monosaccharides to disaccharides or from biogenic sucrose to monosaccharides). Whether these costs have to be added to the synthetic or to the biogenic production costs depends on the subsequent food processing steps. Furthermore, all those sugars have basically the same physiological properties and are therefore used (within given limitations) as interchangeable substitutes in processed food products. For these two reasons, we here neglect the transformation costs for simplicity and treat those monosaccharides and disaccharides as the same macronutrient in the following economical comparison.
The very first knock-out criterion whether biogenic agriculture is competitive lies in the energetic and financial costs for the water supply. This criterion impeded the establishment of a significant agriculture in arid regions but may also frustrate the competitiveness of agriculture in semi-arid regions once the unsustainable extraction of groundwater becomes physically or politically impossible. Depending on sea water desalination as a water supply would at least quadruple the sugar production costs to more than 1.4 €/kg. In consequence, sugar production in semi-arid regions would obviously not be competitive to the conventional production in humid regions or an artificial sugar production. In humid regions, the water externality is typically not yet a major cost contribution. Brazil as the world's largest sugar producer, however, faces water scarcity in some of the plantation regions (Bordonal et al., 2018) and increasing water costs there could have massive effects on the world sugar price. In summary, a future decline in the sugar production in semi-arid or otherwise water-scarce regions can be expected.
An undisputable advantage of an artificial sugar production is that it can be applied virtually anywhere. Particularly beneficial conditions appear to be—of all things—at the coastal regions of low-latitude deserts such as the Sahara. Effective energy costs are there expected to be extremely low because of (1) direct production costs of solar power by photovoltaic or solar thermal collector of less than 50 €/MWh, (2) basically no requirement of an energy storage besides the day–night contrast, (3) the coastal locations allow for a sustainable and relatively cheap water supply by desalination, (4) and the land required for the solar plants and synthesis facilities is typically cheap there because its ecological value and economical opportunity costs are typically low.
Second, while the artificial sugar production does not cause any environmental pollution, the imperative of a transition towards organic farming implies sugar production costs of above 0.8 €/kg (as illustrated at least in Germany). This is about the estimated costs for artificial sugar at electricity costs of 50 €/MWh, thus artificial sugar is already competitive to conventional sugar from humid regions even without considering the further externalities discussed above.
Third, these intermediate findings that conventional but organic sugar from humid regions may still be cheaper than artificial sugar does hold only for the today's balance in demand and supply. On the one hand, the above projections of a decline in the suitable land area for conventional agriculture as well as a potential reduction in the per-area yield due to a transition from non-organic to organic farming imply a reduction of the supply. On the other hand, the expected global population growth will cause an increase in the global sugar demand. Without an artificial sugar supply, this discrepancy would either result in an increase of the sugar price on the world market because the conventional sugar industry would nevertheless have to expand towards less fertile and therefore more expensive lands or in enhanced agricultural land use in humid regions.
The first scenario of an increasing world market price could imply that the production costs of artificial sugar may break-even with the rising world market price even if artificial production is less economical than the conventional sugar production in high-yield regions. The second scenario of an enhanced agricultural activity in humid regions is in concurrence with other land uses. Considering the severe loss in biodiversity in particular in humid regions, it appears possible that future environmental policy prohibits not only such an expansion but even demands a reduction of the agricultural land also in humid regions in favor for natural reservations. In fact, large land areas could be freed by the introduction of an artificial carbohydrate production as can be illustrated by the minimum area which is required to supply the average calorie intake for one adult human by biogenic or artificial calories, which we call in the following a human's “calorie–footprint” (CF). As above, we assume again an average calorie intake of 12 MJ d−1 = 4.4 GJ a−1 for an adult human.
We illustrate the CF for the conditions in Germany: The average solar irradiation which reaches the Earth surface is about 95 W m−2 = 3.0 GJ m−2 a−1 in Germany (Wild et al., 2013). Accordingly, a perfect solar-to-food conversion would imply a theoretical calorie–footprint of . We estimated above a solar-to-food efficiency of ηStG = 6−15% for the artificial sugar production which implies a calorie–footprint of . In contrast, crop plants are only able to convert several tenth of a percent of the incoming solar irradiation to carbohydrates. For instance, the maximum possible sugar yield from sugar beets in Germany is estimated as 2.4 kg m−2 a−1 when assuming optimum growth conditions (Hoffmann and Kenter, 2018); corresponding to a solar-to-sugar conversion efficiency of 1.4%. The practically achieved sucrose yield is lower, e.g., in Germany the average sucrose yield from sugar beets is 1.1−1.3 kg m−2 a−1 (Zuckerverbände Deutschland, 2019). Accordingly, sugar beets yield 18−21 MJ m−2 which corresponds to a solar-to-food conversion efficiency of about 0.7% and .
On a global average, the calorie–footprint is lower for artificial sugar but orders of magnitude higher for biogenic sugar because of the in average much less fertile land when compared to Germany. Accordingly, while the conventional agriculture may fail in the long-term to provide enough food for the current 8 billion humans, covering the one third of the Earth's land area which is currently use for agriculture with solar panels and assuming a solar-to-food efficiency of 15% for artificial sugar would provide (in form of pure sugar) the total calorie demand of 10 trillion humans or about 1,250 times the present world population.
8. Beyond Monosaccharides
The replacement of conventional sugar in our food supply by artificial monosaccharides would have already direct positive impacts on the sustainability of our food supply as shown above. The biogenic sugar production, however, contributes only several percent to the ecological externalities of our food supply while the production of polysaccharides such as starch (amylose, amylopectin) contributing the largest part (e.g., as mentioned above, 18% of the territory of Germany are occupied by plantations of starch-supplying crops while only 1% of its territory is occupied by sugar beet plantations). Accordingly, a much more important implication of an artificial monosaccharide production would be to provide a foundation for a more sustainable production of polysaccharides. Such a subsequent, synthetic production of polysaccharides could be achieved via a polymerization of synthetic monosaccharides. A crucial advance in this direction would focus on the synthesis of starch. Another advance could be the synthesis of cellulose, e.g., in order to supply sustainable feedstock for livestock breeding or the paper industry. We note that (essentially pure) starch (from agricultural production) in the form of flour has been the main ingredient of many types of food like bread, pasta, or beer. Many other products of the modern food industry also contain starch as basic ingredient. A techno-economic investigation of those possible subsequent food production steps is beyond the scope of this paper.
Another advance beyond the scope of this paper would be an at least partially artificial production of fats, proteins, and other nutrients. Digestible fats could be as well entirely synthetically produced from atmospheric CO2 and water via a combination of glycerol ((CH2O)3H2) synthesized, e.g., by the formose reaction and fatty acids synthesized, e.g., by the Fischer–Tropsch process. We note that fat synthesis may allow for a targeted production of healthy or else desired fats. Furthermore, synthesized products could be supplied as sustainable feedstock for biogenic agriculture of microorganisms, fungi, insects, and livestock in order to significantly reducing the environmental externalities of their associated biogenic products.
We here want to highlight again the underlying rational that artificial and biogenic macronutrients are chemically identical. Thus, processed food such as bread, pasta, mashed potatoes etc. can be in principle produced with the same properties such as appearance, taste, and smell when the biogenic macronutrients are substituted by their artificial complements. Because a good fraction of the human macronutrients uptake is nowadays already supplied by processed food, a large part of the ecological externalities currently caused by the biogenic production of, e.g., cereals, rice, potatoes, and fat- and protein-supplying plants could in fact be avoided by using synthetic ingredients.
An estimation of the production costs of synthetic starch appears to be premature, but there are good reasons to be convinced that also for starch a synthetic production could be economically competitive with biogenic production: On the one hand, the production costs of synthetic starch will be most likely above the costs for synthetic monosaccharides estimated in this manuscript due to the additional costs for the monosaccharide polymerization, but these additional costs may be of the order of the costs of the formose reactor (+0.04 €/kgstarch) and thus negligible in comparison to the other costs factors. On the other hand, the production costs of biogenic starch are usually lower than for biogenic sugar but not much. For example, the production costs of conventional wheat flour are in first approximation given by the spot market price for conventional wheat, which was on average 0.22 €/kgwheat in 2016–2018 (OECD and FAO, 2018), plus the grinding costs. Accordingly, the conventional production costs of conventional flour and conventional sugar should be roughly the same. Furthermore, also the ecological externalities of both biogenic products (use of water and pesticides etc.) are roughly the same. In consequence, it is plausible to assume that the total costs of biogenic starch does not deviate much from the totals costs for biogenic sugar.
9. Some Words on Anticipated Societal Changes
A large-scale supply of artificial carbohydrates and other products would nevertheless not result in a total displacement of biogenic agriculture but rather disburdens the current agricultural system from the need to allocate resources such as fertile area and groundwater to the production of macronutrients. In consequence, the biogenic agriculture could enhance its activities in those production sectors where a synthesis is not economically or not possible at all. This applies, first of all, to the production of micronutrients such as taste producing substances and vitamins. In addition, it can be expected that even in a world where a healthy human diet could be produced entirely synthetically, there would be still a demand in non-processed, biogenic food products such as a real turkey with real potatoes and real cranberries.
The transition towards an (entirely) artificial food supply would mark another milestone of human's long-lasting desire to become less dependent on nature. Like for the neolithic and industrial revolutions, the societal, political, and cultural system may change significantly and irreversibly.
Getting rid of the production factor soil in favor of the production factor capital paired with increasing returns to scale in the chemical industry could possibly result in food production becoming an industry as any other industry, with the danger of extreme concentration of market power in the hands of an international oligopoly. If unchecked, such a development could bring most direct and potentially most devastating impacts on society similar to those of the early industrial revolution. It would be a crucial challenge for policymakers to accompany this transition on the one hand by political-economic reforms, which ensure that the national sovereignty does not become subdued by corporate market power, and on the other hand by appropriate social-political reforms, which avoid a division of society in people which can still afford biogenic food and those which can no more satisfy such a demand.
Getting rid of agriculture as one of the oldest achievements of civilization may lead to mankind further growing away from its roots and traditions. As one aspect of many, mankind then needs to reinvestigate its relation to life. For example, if a “vegan+” food supply is possible, where neither animals nor plants need to be killed or else abused, would it be ethically tolerable to continue so?
Getting rid of an Earth-bound food supply would remove the most fundamental limitation of long-term manned space missions and may thus mark the starting point of mankind colonizing the galaxy.
10. Conclusions
We compiled a reaction scheme which allows the artificial synthesis of monosaccharides—exemplified for hexoses—from atmospheric carbon dioxide, water, and electric energy via the formose reaction. All process steps have been demonstrated and described in the literature. The subsequent step of a targeted production of, e.g., pure D-glucose, either via suitable catalysts or a subsequent separation from the mixture of hexoses has not yet been demonstrated in the literature but has been made plausible within this paper. While this paper focused on monosaccharide, it has been highlighted that the artificial monosaccharide production would in principle allow also for the artificial production of starch.
We estimate a solar-to-food energy conversion efficiency of at least 6–15% which implies an at least 10-times higher per-area yield of sugar compared to conventional agriculture. We estimate (total) production costs of 0.5–1.6 €/kg for artificial glucose when produced on an industrial scale and subject to renewable energy costs of 50–100 €/MWh (but exclusively the yet speculative costs for the stereoisomer separation via a catalyst or subsequent processing). We estimate that the production costs of conventional sugar increase at least to ≥0.9 €/kg for humid conditions and ≥2 €/kg for semi-arid conditions when including the costs of its most obvious ecological externalities such as an unsustainable water demand and environmental pollution. Accordingly, the artificial sugar production may already today have lower total production costs than the conventional sugar production.
In conclusion, the artificial carbohydrate production can provide an affordable and secure food supply for a multiple of the today's world population, while at the same time lowering the ecological and climatological externalities of the conventional agriculture. In addition, the artificial carbohydrate production would avoid socio-economic externalities such as an insecure food supply, national food dependencies, need of human work force, and occupation of valuable land. A transition towards such an at least partially artificial food supply appears not only to be smart but may be the only possibility to avoid future global conflicts on food resources. In addition, the freed land area could be used to extenuate other man-made degradations of the ecological system, for instance, by the establishment of nature reserves for endangered species, by afforestation programs to mitigate the climate change, or simply by enhancing sustainable organic farming.
Author Contributions
Both authors contributed equally to the conception and realization of the study. FD wrote the manuscript.
Conflict of Interest
The authors declare that the research was conducted in the absence of any commercial or financial relationships that could be construed as a potential conflict of interest.
Acknowledgments
This manuscript has been released on September 9, 2019 as a Pre-Print at ChemRxiv (see Dinger and Platt, 2019).
References
Aeschbach-Hertig, W., and Gleeson, T. (2012). Regional strategies for the accelerating global problem of groundwater depletion. Nat. Geosci. 5:853. doi: 10.1038/ngeo1617
Atkins, P. W., DePaula, J., and Keeler, J. (2018). Atkins' Physical Chemistry, 11th Edn. Oxford: Oxford University Press. doi: 10.3366/edinburgh/9781474400046.003.0035
Bahmanpour, A., Hoadley, A., and Tanksale, A. (2014). Critical review and exergy analysis of formaldehyde production processes. Rev. Chem. Eng. 30, 583–604. doi: 10.1515/revce-2014-0022
Bahmanpour, A. M., Hoadley, A., and Tanksale, A. (2015). Formaldehyde production via hydrogenation of carbon monoxide in the aqueous phase. Green Chem. 17, 3500–3507. doi: 10.1039/C5GC00599J
Bautista, D. A., Pegg, R. B., and Shand, P. J. (2000). Effect of l-glucose and d-tagatose on bacterial growth in media and a cooked cured ham product. J. Food Protect. 63, 71–77. doi: 10.4315/0362-028X-63.1.71
Bordonal, R. D. O., Carvalho, J. L. N., Lal, R., de Figueiredo, E. B., de Oliveira, B. G., and La Scala, N. (2018). Sustainability of sugarcane production in Brazil. A review. Agron. Sustain. Dev. 38:13. doi: 10.1007/s13593-018-0490-x
Borrelli, P., Robinson, D. A., Fleischer, L. R., Lugato, E., Ballabio, C., Alewell, C., et al. (2017). An assessment of the global impact of 21st century land use change on soil erosion. Nat. Commun. 8:2013. doi: 10.1038/s41467-017-02142-7
Boulamanti, A., and Moya, J. A. (2017). Production costs of the chemical industry in the EU and other countries: ammonia, methanol and light olefins. Renew. Sustain. Energy Rev. 68, 1205–1212. doi: 10.1016/j.rser.2016.02.021
Breslow, R. (1959). On the mechanism of the formose reaction. Tetrahedron Lett. 1, 22–26. doi: 10.1016/S0040-4039(01)99487-0
Breslow, R., and Cheng, Z.-L. (2010). L-amino acids catalyze the formation of an excess of d-glyceraldehyde, and thus of other d sugars, under credible prebiotic conditions. Proc. Natl. Acad. Sci. U.S.A. 107, 5723–5725. doi: 10.1073/pnas.1001639107
Bundesinformationszentrum Landwirtschaft (2017). Statistisches Jahrbuch Über ernährung, Landwirtschaft und Forsten.
Bundesinformationszentrum Landwirtschaft (2018). Bericht Zur Markt- und Versorgungslage Zucker 2018.
Butlerow, A. (1861). Bildung einer zuckerartigen substanz durch synthese. Justus Liebigs Annalen der Chemie 120, 295–298. doi: 10.1002/jlac.18611200308
Cheng, M.-H., Huang, H., Dien, B. S., and Singh, V. (2019). The costs of sugar production from different feedstocks and processing technologies. Biofuels Bioprod. Biorefin. 13, 723–739. doi: 10.1002/bbb.1976
Clancy, M., and Whelan, W. (1967). Enzymic polymerization of monosaccharides: I. the enzymic polymerization of d-galactose. Arch. Biochem. Biophys. 118, 724–729. doi: 10.1016/0003-9861(67)90410-9
Cumberlege, T., Blenkinsopp, T., and Clark, J. (2016). Assessment of Environmental Impact of Feedkind Protein. 26. Available online at: http://calysta.com/Carbon%20Trust%20Report%20on%20FeedKind%20Protein.pdf
Degner, J. (2016). Richtwerte für Leistungen und Kosten der Produktion von zuckerrüben. Thüringer Landesanstalt Für Landwirtschaft.
Delidovich, I. V., Simonov, A. N., Taran, O. P., and Parmon, V. N. (2014). Catalytic formation of monosaccharides: From the formose reaction towards selective synthesis. ChemSusChem 7, 1833–1846. doi: 10.1002/cssc.201400040
Deng, J., Pan, T., Xu, Q., Chen, M.-Y., Zhang, Y., Guo, Q.-X., and Fu, Y. (2013). Linked strategy for the production of fuels via formose reaction. Sci. Rep. 3:1244. doi: 10.1038/srep01244
Dinger, F., and Platt, U. (2019). Towards an artificial carbohydrates supply on Earth. ChemRxiv. Preprint. doi: 10.26434/chemrxiv.9783791.v1
Dudley, N., and Alexander, S. (2017). Agriculture and biodiversity: a review. Biodiversity 18, 45–49. doi: 10.1080/14888386.2017.1351892
Fairtrade International (2019). Available online at: https://info.fairtrade.net/product/sugar
Famiglietti, J. S. (2014). The global groundwater crisis. Nat. Clim. Change 4:945. doi: 10.1038/nclimate2425
Fischer, E. (1913). Synthesen in der zuckergruppe. Berichte der deutschen chemischen Gesellschaft 23, 2114–2141. doi: 10.1002/cber.18900230268
Fukano, K., Ozawa, K., Kokubu, M., Shimizu, T., Ito, S., Sasaki, Y., et al. (2018). Structural basis of l-glucose oxidation by scyllo-inositol dehydrogenase: implications for a novel enzyme subfamily classification. PLoS ONE 13:e0198010. doi: 10.1371/journal.pone.0198010
Gerbens-Leenes, W., and Hoekstra, A. (2009). The Water Footprint of Sweeteners and Bio-ethanol from Sugar Cane, Sugar Beet and Maize. Number 38 in Value of water research report. Unesco-IHE Institute for Water Education.
Government of Mecklenburg-Vorpommern (2018). Risikomanager Auf dem Feld: Dürrefolgen Fordern Bauern Auch in 2019.
Graves, C., Ebbesen, S. D., Mogensen, M., and Lackner, K. S. (2011). Sustainable hydrocarbon fuels by recycling CO2 and H2O with renewable or nuclear energy. Renew. Sustain. Energy Rev. 15, 1–23. doi: 10.1016/j.rser.2010.07.014
Grogan, D. S., Wisser, D., Prusevich, A., Lammers, R. B., and Frolking, S. (2017). The use and re-use of unsustainable groundwater for irrigation: a global budget. Environ. Res. Lett. 12:034017. doi: 10.1088/1748-9326/aa5fb2
He, X., and Hägg, M.-B. (2014). Energy efficient process for co2 capture from flue gas with novel fixed-site-carrier membranes. Ener. Proc. 63, 174–185. doi: 10.1016/j.egypro.2014.11.018
Heim, L. E., Konnerth, H., and Prechtl, M. H. G. (2017). Future perspectives for formaldehyde: pathways for reductive synthesis and energy storage. Green Chem. 19, 2347–2355. doi: 10.1039/C6GC03093A
Hoffmann, C. M., and Kenter, C. (2018). Yield potential of sugar beet-have we hit the ceiling? Front. Plant Sci. 9:289. doi: 10.3389/fpls.2018.00289
IPCC (2014a). “Agriculture, forestry and other land use (AFOLU),” in Climate Change 2014: Mitigation of Climate Change. Contribution of Working Group III to the Fifth Assessment Report of the Intergovernmental Panel on Climate Change. Cambridge: Cambridge University Press.
IPCC (2014b). “Food security and food production systems,” in Climate Change 2014: Impacts, Adaption, and Vulnerability. Part A: Global and Sectoral Aspects. Contribution of Working Group II to the Fifth Assessment Report of the Intergovernmental Panel on Climate Change. Cambridge: Cambridge University Press.
Iqbal, Z., and Novalin, S. (2012). The formose reaction: a tool to produce synthetic carbohydrates within a regenerative life 35 support system. Curr. Organ. Chem. 16, 769–788. doi: 10.2174/138527212799957968
Kadokawa, J., and Kobayashi, S. (2010). Polymer synthesis by enzymatic catalysis. Curr. Opin. Chem. Biol. 14, 145–153. doi: 10.1016/j.cbpa.2009.11.020
Keith, D. W., Holmes, G., Angelo, D. S., and Heidel, K. (2018). A process for capturing CO2 from the atmosphere. Joule 2, 1573–1594. doi: 10.1016/j.joule.2018.05.006
Kitadai, N., and Maruyama, S. (2018). Origins of building blocks of life: a review. Geosci. Front. 9, 1117–1153. doi: 10.1016/j.gsf.2017.07.007
Kozma, D., Madará sz, Z., Ács, M., and Fogassy, E. (1995). A new method for enantiomer enrichment: distillation to separate the free and complexed enantiomers after partial salt formation. Chirality 7, 381–382. doi: 10.1002/chir.530070512
Lackner, K. (2009). Capture of carbon dioxide from ambient air. Eur. Phys. J. Spcl. Top. 176, 93–106. doi: 10.1140/epjst/e2009-01150-3
Lambert, J. B., Gurusamy-Thangavelu, S. A., and Ma, K. (2010). The silicate-mediated formose reaction: bottom-up synthesis of sugar silicates. Science 327, 984–986. doi: 10.1126/science.1182669
Levilain, G., and Coquerel, G. (2010). Pitfalls and rewards of preferential crystallization. CrystEngComm 12, 1983–1992. doi: 10.1039/c001895c
Lu, Y., Song, S., Wang, R., Liu, Z., Meng, J., Sweetman, A. J., et al. (2015). Impacts of soil and water pollution on food safety and health risks in China. Environ. Int. 77, 5–15. doi: 10.1016/j.envint.2014.12.010
Matassa, S., Boon, N., Pikaar, I., and Verstraete, W. (2016). Microbial protein: future sustainable food supply route with low environmental footprint. Microb. Biotechnol. 9, 568–575. doi: 10.1111/1751-7915.12369
Meindertsma, W., van Sark, W., and Lipchin, C. (2010). Renewable energy fueled desalination in Israel. Desalin. Water Treat. 13, 450–463. doi: 10.5004/dwt.2010.1004
Michitaka, T., Imai, T., and Hashidzume, A. (2017). Formose reaction controlled by a copolymer of n,n-dimethylacrylamide and 4-vinylphenylboronic acid. Polymers 9:549. doi: 10.3390/polym9110549
Nakata, K., Ozaki, T., Terashima, C., Fujishima, A., and Einaga, Y. (2014). High-yield electrochemical production of formaldehyde from CO2 and seawater. Angew. Chem. Int. Ed. 53, 871–874. doi: 10.1002/anie.201308657
Omojola, K. (2015). High temperature co-electrolysis of carbon dioxide and steam in a solid oxide cell for synthesis gas production (Ph.D. thesis). Unniversity of Sheffield, Sheffield, United Kingdom.
O'Neill, D. W., Fanning, A. L., Lamb, W. F., and Steinberger, J. K. (2018). A good life for all within planetary boundaries. Nat. Sustain. 1, 88–95. doi: 10.1038/s41893-018-0021-4
Pallmann, S., Šteflová, J., Haas, M., Lamour, S., Henß, A., and Trapp, O. (2018). Schreibersite: an effective catalyst in the formose reaction network. N. J. Phys. 20:055003. doi: 10.1088/1367-2630/aabb99
Plante, O. J., Palmacci, E. R., and Seeberger, P. H. (2001). Automated solid-phase synthesis of oligosaccharides. Science 291, 1523–1527. doi: 10.1126/science.1057324
Plourde, J. D., Pijanowski, B. C., and Pekin, B. K. (2013). Evidence for increased monoculture cropping in the central United States. Agric. Ecosyst. Environ. 165, 50–59. doi: 10.1016/j.agee.2012.11.011
Raymer, G. S., Hartman, D. E., Rowe, W. A., Werkman, R. F., and Koch, K. L. (2003). An open-label trial of l-glucose as a colon-cleansing agent before colonoscopy. Gastrointestin. Endosc. 58, 30–35. doi: 10.1067/mge.2003.293
Rockström, J., Lannerstad, M., and Falkenmark, M. (2007). Assessing the water challenge of a new green revolution in developing countries. Proc. Natl. Acad. Sci. U.S.A. 104:6253. doi: 10.1073/pnas.0605739104
Rukhlenko, I. D., Tepliakov, N. V., Baimuratov, A. S., Andronaki, S. A., Gun'ko, Y. K., Baranov, A. V., et al. (2016). Completely chiral optical force for enantioseparation. Sci. Rep. 6:36884. doi: 10.1038/srep36884
Schmidt, O., Gambhir, A., Staffell, I., Hawkes, A., Nelson, J., and Few, S. (2017). Future cost and performance of water electrolysis: an expert elicitation study. Int. J. Hydrogen Energy 42, 30470–30492. doi: 10.1016/j.ijhydene.2017.10.045
Schuur, B., Verkuijl, B. J. V., Minnaard, A. J., de Vries, J. G., Heeres, H. J., and Feringa, B. L. (2011). Chiral separation by enantioselective liquid–liquid extraction. Org. Biomol. Chem. 9, 36–51. doi: 10.1039/C0OB00610F
Seeberger, P. H., and Haase, W.-C. (2000). Solid-phase oligosaccharide synthesis and combinatorial carbohydrate libraries. Chem. Rev. 100, 4349–4394. doi: 10.1021/cr9903104
Seeberger, P. H., and Werz, D. B. (2005). Automated synthesis of oligosaccharides as a basis for drug discovery. Nat. Rev. Drug Discov. 4, 751–763. doi: 10.1038/nrd1823
Shimizu, T., Takaya, N., and Nakamura, A. (2012). An L-glucose catabolic pathway in Paracoccus species 43P. J. Biol. Chem. 287, 40448–40456. doi: 10.1074/jbc.M112.403055
Spath, P., and Dayton, D. (2003). Preliminary Screening-Technical and Economic Assessment of Synthesis Gas to Fuels and Chemicals With Emphasis on the Potential for Biomass-Derived Syngas. National Renewable Energy Laboratory, U.S. Department of Energy. doi: 10.2172/1216404
Südzucker (2019). Biorüben-Preis 2019. Available online at: https://bisz.suedzucker.de/anbau/biozuckerrueben-in-der-suedzucker-ag/preise-biorueben-2018
Sugahara, H., Meinert, C., Nahon, L., Jones, N. C., Hoffmann, S. V., Hamase, K., et al. (2018). D-amino acids in molecular evolution in space-absolute asymmetric photolysis and synthesis of amino acids by circularly polarized light. Biochim. Biophys. Acta Proteins Proteomics 1866, 743–758. doi: 10.1016/j.bbapap.2018.01.004
Sunfire (2019a). Breakthrough for Power-to-X:Sunfire Puts First Co-electrolysis into Operation and Starts Scaling. Available online at: https://www.sunfire.de/en/company/news/detail/breakthrough-forpower-to-x-sunfire-puts-first-co-electrolysis-into-operationand-starts-scaling
Sunfire (2019b). Sunfire-Hylink–Technical Data. Available online at: https://www.sunfire.de/files/sunfire/images/content/Produkte_Technologie/factsheets/Sunfire-HyLink_FactSheet.pdf
Szekrenyi, A., Garrabou, X., Parella, T., Joglar, J., Bujons, J., and Clapés, P. (2015). Asymmetric assembly of aldose carbohydrates from formaldehyde and glycolaldehyde by tandem biocatalytic aldol reactions. Nat. Chem. 7:724. doi: 10.1038/nchem.2321
Tassinari, F., Steidel, J., Paltiel, S., Fontanesi, C., Lahav, M., Paltiel, Y., et al. (2019). Enantioseparation by crystallization using magnetic substrates. Chem. Sci. 10, 5246–5250. doi: 10.1039/C9SC00663J
Thema, M., Bauer, F., and Sterner, M. (2019). Power-to-gas: electrolysis and methanation status review. Renew. Sustain. Energy Rev. 112, 775–787. doi: 10.1016/j.rser.2019.06.030
Vogel, P., and Robina, I. (2008). “De novo synthesis of monosaccharides,” in Glycoscience, 2nd Edn., eds B. O. Fraser-Reid, K. Tatsuta, and J. Thiem (Berlin; Heidelberg; New York, NY: Springer-Verlag), 858–956. doi: 10.1007/978-3-540-30429-6_20
Wada, Y. (2016). Modeling groundwater depletion at regional and global scales: present state and future prospects. Surv. Geophys. 37, 419–451. doi: 10.1007/s10712-015-9347-x
Wang, Q.-P., Simpson, S. J., Herzog, H., and Neely, G. G. (2017). Chronic sucralose or l-glucose ingestion does not suppress food intake. Cell Metab. 26, 279–280. doi: 10.1016/j.cmet.2017.07.002
Wild, M., Folini, D., Schär, C., Loeb, N., Dutton, E. G., and König-Langlo, G. (2013). The global energy balance from a surface perspective. Clim. Dyn. 40, 3107–3134. doi: 10.1007/s00382-012-1569-8
Zheng, Y., Wang, J., Yu, B., Zhang, W., Chen, J., Qiao, J., and Zhang, J. (2017). A review of high temperature co-electrolysis of H2O and CO2 to produce sustainable fuels using solid oxide electrolysis cells (SOECS): advanced materials and technology. Chem. Soc. Rev. 46, 1427–1463. doi: 10.1039/C6CS00403B
Zhu, X.-G., Long, S. P., and Ort, D. R. (2008). What is the maximum efficiency with which photosynthesis can convert solar energy into biomass? Curr. Opin. Biotechnol. 19, 153–159. doi: 10.1016/j.copbio.2008.02.004
Zuckerverbände Deutschland (2019). Rübenverarbeitung und Zucker- 90 Erzeugung in Deutschland. Available online at: http://www.zuckerverbaende.de/zuckermarkt/zahlen-und-fakten/zuckermarkt-deutschland/ruebenanbau-zuckererzeugung.html
Appendix
A. Energy Demand for the Extraction of CO2 and H2O From the Local Atmosphere
Given a gas mixture which contains component A with a molar mixing ratio χ ≪ 1, the absolute temperature T, and the universal gas constant R = 8.314 J mol−1 K−1. The mixing entropy ΔSM relative to the component A for one mole of mixture is given by (see any textbook on thermodynamics, e.g., Atkins et al., 2018)
and the mixing entropy ΔSM relative to the component A for one mole of the component A is given by
The minimum required energy to separate 1 mole of the component A from the mixture is given by
Assuming T = 300 K, the energy demand for the extraction of 1 mole of CO2 or H2O from the local atmosphere reads
Keywords: artificial synthesis, formose reaction, monosaccharides, sustainable food supply, water-smart food supply
Citation: Dinger F and Platt U (2020) Towards an Artificial Carbohydrates Supply on Earth. Front. Sustain. Food Syst. 4:90. doi: 10.3389/fsufs.2020.00090
Received: 07 November 2019; Accepted: 18 May 2020;
Published: 07 July 2020.
Edited by:
Kathleen L. Hefferon, Cornell University, United StatesReviewed by:
Gilles François Billen, UMR7619 Milieux Environnementaux, Transferts et Interactions dans les hydrosystèmes et les Sols (METIS), FranceHaritha Bollinedi, Indian Agricultural Research Institute (ICAR), India
Copyright © 2020 Dinger and Platt. This is an open-access article distributed under the terms of the Creative Commons Attribution License (CC BY). The use, distribution or reproduction in other forums is permitted, provided the original author(s) and the copyright owner(s) are credited and that the original publication in this journal is cited, in accordance with accepted academic practice. No use, distribution or reproduction is permitted which does not comply with these terms.
*Correspondence: Florian Dinger, ZmRpbmdlckBpdXAudW5pLWhlaWRlbGJlcmcuZGU=