- 1Soil and Crop Sciences, Colorado State University, Fort Collins, CO, United States
- 2Natural Resource Ecology Laboratory Colorado State University, Fort Collins, CO, United States
- 3Natural Resources Sciences, McGill University, Montreal, QC, Canada
- 4United States Department of Agriculture, Central Great Plains Research Station, Akron, CO, United States
To meet the nutritional demands of a rapidly growing population in the face of increasing climate variability, innovative tools are needed to rapidly regenerate soil health in agricultural systems. Using food wastes to improve soil health presents a viable opportunity to improve soils and efficiently manage waste. In a previous laboratory study, we found that potassium lactobionate, a byproduct of cheese production, greatly enhanced soil water holding capacity and nutrient availability. To further explore its potential as a soil amendment, we conducted agronomic trials in winter wheat and corn at the USDA-ARS Central Great Plains Research Station in Akron, Colorado. We evaluated lactobionate for potential improvements in key soil health indices, focusing on soil moisture, carbon, and nitrate. Lactobionate was applied at 5 rates and either as broadcast or surface banding depending on the crop, and soil samples were collected from 0 to 5 cm and 5 to 15 cm depths. Four weeks after broadcast application in the wheat trial, we observed a significant increase in soil moisture and microbial biomass in the 5–15 cm-depth and a decrease in soil nitrate at both soil depths and across rates, relative to unamended plots (p < 0.1). We also saw a non-significant 14% increase in corn yield with subsurface banding of lactobionate but no observed changes in other soil properties measured in the corn trial. We found no significant changes in soil pH, total soil carbon and nitrogen, and soil ammonium concentration with lactobionate for both trials. Our observations suggest the potential for lactobionate to modify soil water content, microbial biomass, nitrate, and yield but outcomes varied by crop trial and amendment rates. This implies that while recycling food waste for use as a soil amendment may have benefits for key soil health parameters, the timing, mode and application amount need to be optimized for maximal effects of lactobionate.
Introduction
Productive soils are the foundation of a sustainable and secure global food supply. Human activity has degraded nearly 40% of the world's soils (Oldeman, 1994) through intensive tilling, erosion, mining and industrial activities, and excessive chemical inputs. This has led to a decline in many indicators of soil health, including nitrogen (N) retention and use efficiency, carbon (C) sequestration, and water infiltration and retention (Gugino et al., 2009). Water limitations and nutrient supply remain the major limiting factors to crop productivity globally (Tilman et al., 2002). As a result, modern day agriculture often depends significantly on the continuous use of freshwater irrigation and synthetic agrochemicals for optimal crop production. Such reliance on high water and chemical inputs contributes to the depletion of limited water resources—particularly in arid and semi-arid locations (Morison et al., 2007), eutrophication, and ground water pollution (Cassman, 1999).
To feed a growing global human population and achieve optimal crop productivity in the face of increasing climate variability, we need to rapidly regenerate soil health in a sustainable manner by targeting the key indicators of soil functional capacity relevant to the challenges of a particular cropping system. While there is no universal definition for soil health, it broadly acknowledges the functional capacity of soils to sustain plant productivity, maintain water and air quality, and support human well-being and other essential ecosystem services (Doran and Parkin, 1994; Doran, 2002; Kibblewhite et al., 2008). Healthy soils have also been described as active living entities (Kibblewhite et al., 2008; Lal, 2016) and thus imply that biological presence and activity are key to soil functions. Management approaches that can support soil water retention, increase soil C stocks, sustain microbial activity, and improve the timing of nutrient supply are especially needed in regions such as the U.S. Great Plains where water limitation, and subsequently soil C and N, are often the pivotal attributes impacting soil functioning and crop productivity (Ko et al., 2012; Robertson et al., 2018). These soil properties are related to each other and connected to the overall functional capacity of soils. For instance, soil water retention has been linked with soil C (Lal, 2014) while recent reports have shown that soil microbial biomass and their byproducts form a significant source of stable soil C (Kallenbach et al., 2016). One common approach for both increasing soil water retention and soil C and N is through the application of organic amendments to soils. For example, in a global meta-analysis carried out by Eden et al. (2017), the addition of organic wastes to soils improved plant available water on a long term basis and also conferred benefits on other soil properties.
Most studies on the recycling of organic wastes in agriculture have focused on composts and of manure from livestock production (Petersen et al., 2007; Hargreaves et al., 2008; Vasilica et al., 2009; Annabi et al., 2011). The application of these organic wastes to soils have been shown to improve crop yield (Luo et al., 2018), enhance microbial biomass and activity (Kallenbach and Grandy, 2011), support soil fertility (Chaparro et al., 2012), and sustain long term soil health long term (Xie et al., 2014). While it is well-documented that compost and other organic inputs confer positive effects on soils, there are many barriers to widespread implementation. Variation in composition and physicochemical properties of different soil amendments has been shown to modulate their effects on soils, thereby generating uncertainty in their efficacy and sustainability (Fereidooni et al., 2013; Malik et al., 2013; Ninh et al., 2015). The economic, labor and public health costs (including pathogen transmission and unpleasant odors) associated with compost and manure-based organic amendments also limits their accessibility to growers. Thus, there is an urgent need to develop alternative, sustainable approaches that can rapidly regenerate soil functional capacity, including water storage and nutrient retention, by considering other sources of single stream wastes such as food processing products.
A promising approach to enhance soil services and crop productivity is by the conversion of food byproducts and waste into soil amendments. In developed nations such as the United States, roughly 40% (52 million tons) of the food produced annually is not eaten, with most of the waste disposed in landfills (ReFED, 2016; Gunders and Bloom, 2017). As a result, $218 billion worth of labor and resources invested in agricultural production is wasted annually (ReFED, 2016). For developing countries, post-harvest food losses total $4 billion per year, contributing to chronic poverty and hunger (Food and Agriculture Organization, 2013). With 868 million malnourished people facing starvation daily, this wasted food is a missed opportunity (Bond, 2013). Food wastes occur at every level of the food supply chain, from field to fork. Food waste is not only an economic, but an environmental and moral issue as well. Foods diverted to landfills contribute directly to climate change via the emission of methane (CH4), a significant greenhouse gas 25 times more potent than carbon dioxide (CO2) (United States Environmental Protection Agency, 2019). Significant attention has been focused on food waste management at the retail and post-consumer level, reflecting the USEPA's food recovery hierarchy strategy (United States Environmental Protection Agency, 2016). However, industrial food processing and manufacturing byproducts represent 14% of total food waste generated (Commission for Environmental Cooperation, 2017). Food waste and food production byproducts diverted from landfills and incinerators to agricultural fields can also provide a rich source of organic nutrients and C that may facilitate microbial-mediated nutrient and C cycling, a foundation to biological agroecosystem management (Drinkwater et al., 2017). These food processing/manufacturing byproducts present a meaningful opportunity to put waste to work if they can be converted to useful organic soil amendments.
The production of mozzarella cheese produces large amounts of whey byproduct. For every kilogram of cheese produced, 9 kg of whey is generated (Robbins et al., 1996; Prazeres et al., 2012). In 2013, global whey production was estimated at 180 million tons (Dairy Processing Handbook, 2014). Whey primarily consists of water (93–94%), lactose (4.5–6%), proteins (0.6–1.1%), minerals (0.8–1.1%), and fats (0.06%) (Guimarães et al., 2010; Prazeres et al., 2012; Carvalho et al., 2013). Lactose constitutes up to 90% of whey organic load content and contributes to whey's high biodegradability index, biological oxygen demand, and chemical oxygen demand that burdens municipal sewage treatment systems (Berruga et al., 1997; Janczukowicz et al., 2008; Yadav et al., 2015). As a result, direct disposal of this waste without pre-treatment has been widely banned (Yadav et al., 2015). Whey and its lactose derivatives are highly underutilized (Affertsholt-Allen, 2007). Thus, there is a need to find alternative applications for lactose (Gänzle et al., 2008; Alonso et al., 2013).
A derivative of lactose called lactobionate contains unique properties that we previously showed improved soil water retention, soil microbial biomass, and soil C content (Kallenbach et al., 2019). Lactobionate is produced from the enzymatic oxidation of lactose and consists of gluconic acid, galactose, and metal ions (Potassium, Calcium). Antioxidant, chelating and emulsifying properties of lactobionate have made it useful in the pharmaceutical, cosmetic, and infant formula industries (Green et al., 2009; Wu et al., 2009; Gutiérrez et al., 2012). In a prior laboratory trial, we applied different forms of lactobionate (potassium lactobionate, calcium lactobionate, ammonium lactobionate) to soils of contrasting C contents from semi-arid locations in the USA (Colorado and California) (Kallenbach et al., 2019). All forms of lactobionate increased soil water retention as well as soil C and microbial biomass compared to the control. Potassium lactobionate showed the greatest and most dramatic effects on all of the soil properties measured. Soils amended with potassium lactobionate improved their water holding capacity by 100–600% compared to unamended soils. Lactobionate amended soils also exhibited a persistent increase (87%) in soil organic C 2 months after the amendment was applied (Kallenbach et al., 2019).
While lactobionate showed great potential as a soil amendment in our laboratory experiment, it might not exhibit the same effect under field conditions. High variability in the field with respect to climate and other associated parameters, coupled with the unknown potential interactions with plants and other uncontrollable factors are reasons why lactobionate and other soil amendments may not show the same success in the field as compared to controlled laboratory observations. Hence, we conducted agronomic trials on winter wheat and corn to assess the impact of potassium lactobionate on soil properties relevant to agronomic challenges in dryland agriculture. We focused on soil water retention since in dryland agriculture, increasing soil moisture is likely to have one of the most significant impacts on crop yields (Ko et al., 2012; Kallenbach et al., 2019). We also examined changes in soil C, mineral nitrogen, and microbial biomass, typically at low concentrations in this region and thus further limiting both water retention and nutrient supply. The objectives of the agronomic trials were (1) to validate laboratory observations at the field-level in two different cropping system and (2) to assess lactobionate impacts on soil water retention, crop nutrient availability, and soil C across different application levels to achieve the optimal impacts of lactobionate.
Materials and Methods
Field Layout and Soil Sample Collection
A field experiment was conducted at the USDA-ARS Central Great Plains Research Station located in Akron, CO (40.15 °N, 103.15 °W, 4540 feet elevation). The climate is semiarid, with an annual rainfall of 420.624 mm (average normal from 1981 to 2010) (usclimatedata.com, accessed 2018). The soil type is classified as a silty loam Weld series (Calderón et al., 2015) with a total C of 1.0%, total nitrogen (N) of 0.1% and pH of 5.7. Soils used for this trial were under high crop residue retention with no tillage management for both wheat and corn trials, respectively.
Potassium lactobionate (referred to as lactobionate for the remainder of this paper) was used in this study and supplied in liquid formulation by Leprino Foods Company (Denver, CO). This formulation had a 65% moisture and 35% solids (lactobionate) content (Figure 1). Lactobionate consists of 36.6% C, 4.8% potassium (K), pH of 6.7, and has no other nutrients. A complete randomized block design was used in this study, consisting of 5 levels of lactobionate treatments replicated 5 times for the wheat trial (0, 159, 318, 655, and 1,871 liters/hectare) and corn trial (0, 187, 374, 561, and 748 liters/hectare). Plots were 15.34 m long by 4.57 m wide for winter wheat and 24.38 m by 6.09 m wide for corn. The application rates were selected based on the economic feasibility of lactobionate supply from the manufacturer. The lactobionate treatments were applied using two approaches: for the wheat trial, it was directly applied to the soil surface as liquid formulation via spraying with the aid of a tractor mounted sprayer; and for the corn trial, it was banded into the soil near the zone of planting to a depth of 10 cm. The different rates and routes of application were selected to examine their differential influence on soil properties and crop productivity with no intentions on comparing routes of application but to examine the most economically feasible and efficient application rates. In September of 2017, winter wheat (Triticum aestivum) variety Snowmass 2.0 was planted across the plots 3 days after lactobionate applications. In May, 2018, a separate field trial was set up by banding lactobionate together with corn seeds (Zea mays) variety DeKalb 45-65 RIB. Since 1994, all plots have been managed using no-till dryland practices, common to the region for enhancing soil moisture retention. Soils were fertilized at typical rates for this region with urea ammonium nitrate (UAN) at a rate of 67.25 kg N/hectare and with diammonium phosphate at 22.41 kg P/hectare.
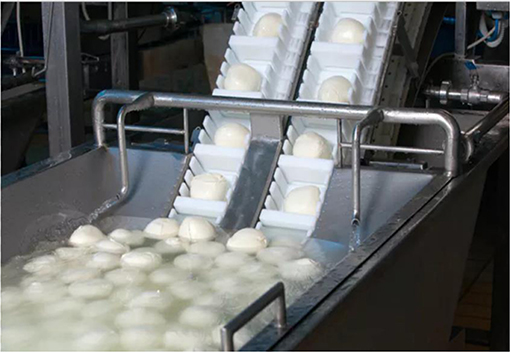
Figure 1. Production of lactobionate in a cheese factory (Shutterstock.com/ Giuseppe Parisi).
Prior to lactobionate application, soils were collected from each plot at two depths (0–5 cm, 5–15 cm) with a 2.5 cm-diameter hand-held soil corer. For the winter wheat trial, 5 soil cores were collected randomly in each plot and composited. For the corn trial, 5 soil cores were collected randomly between lactobionate-banded corn rows and composited. Soils were placed in ziplock bags on ice and transported at field-moisture level. The same procedure was completed 4 weeks after lactobionate application for the corn trial and at 5 weeks for the wheat trial due to unusually intense rainfall episodes immediately after lactobionate application. Soil samples were stored in a 4°C refrigerator upon arrival. The samples were then sieved to 2 mm and all analyses were conducted within a week of sample collection.
Soil Analyses
Soil Moisture and pH
Gravimetric soil moisture was estimated on a 10 g subsample by drying in a 105°C oven for 24 h, and the difference between the soil weight pre-drying and post-drying was used for soil dry weight correction. We also collected wheat volumetric moisture data directly from each plot using a soil moisture sensor probe (integrated across 12-cm depth) (Stevens Hydraprobe, Portland OR). Five volumetric moisture data points were collected per plot on the 21st of October and 22nd of November 2017 for the winter wheat trial. For the corn trial, volumetric moisture data was collected on the 11th of May and 8th of June, 2019. Soil pH was determined in 1:5 soil:water mixture using an Orion EA 9110 m (Thermo Scientific, Beverly, MA, USA). There was no treatment effect on soil pH across soil depths for the two field trial conducted (Tables 1, 2).
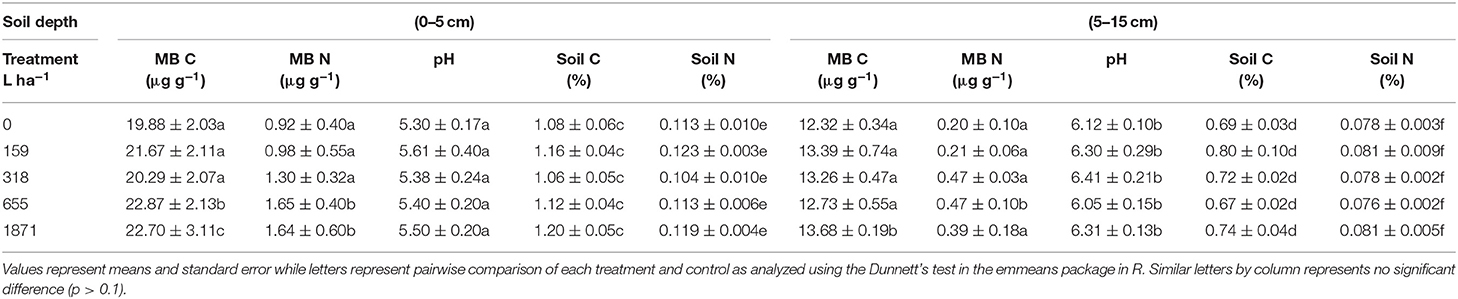
Table 1. Effect of lactobionate application rates on microbial biomass C and N, soil pH, soil C, and N across soil depths (0–5, 5–15 cm) for the wheat field trial.
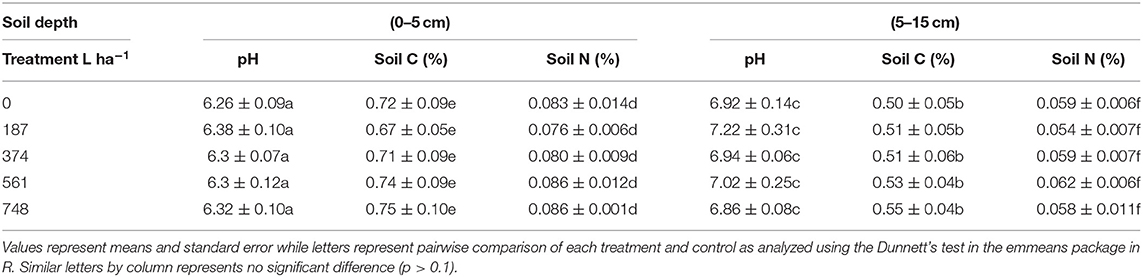
Table 2. Effect of lactobionate application rates on soil pH, soil C, and N across soil depths (0–5, 5–15 cm) for the corn field trial.
Soil C and N
We determined total soil C and N on oven-dry, pulverized soil samples analyzed on a LECO True-Spec CN analyzer (Leco Corp., St. Joseph, MI, USA). The output was estimated as a percentage. Soil nitrate ()-N and ammonium ()-N was determined by extraction using 25 ml of 2 M KCl, filtered using a Whatman Filter paper no. 42 and estimated on the Alpkem Flow Solution IV Automated wet chemistry system (O.I. Analytical, College Station TX, USA).
Microbial Biomass C and N
To determine microbial biomass C and N, the chloroform fumigation extraction method as described by Vance et al. (1987) was conducted on 10 g of soils stored in the 4°C refrigerator. Two ml of alcohol-free chloroform was added to a subset of soils and then extracted using 40 ml of 0.5 M K2SO4. Total organic C and total N was then measured with a TOC-V-TN analyzer (Shimadzu Corp., Kyoto, Japan). We calculated microbial biomass C and N by subtracting the unfumigated extracts from the fumigated extracts, using a conversion efficiency factor (k) of 0.45.
Wheat and Corn Yield and Protein Content
Wheat and corn yields were measured in July and November 2018, respectively. Both wheat and corn were harvested with a field-plot combine harvester with one pass through the center of each plot and yield quantified by plot. To determine grain protein content, N was first estimated using the same method as in soil total C and N on wheat and corn grain sub samples collected in each plot at harvest. Protein content was calculated from total grain N using a factor of 5.68 for corn (Sriperm et al., 2011) and 5.7 for wheat (Association of Official Analytical Chemists, 1984).
Statistical Analyses
To examine the effects of lactobionate application on soil moisture, soil mineral N, soil microbial biomass, wheat and corn yield, we fitted a general linear model with treatment as the fixed effect, and ran a one-way analysis of variance (ANOVA), followed by pairwise comparisons of control to each treatment using the Dunnett's test in the estimated marginal means (emmeans) package in R. The level of significance (p) was set at 0.1 due to the minimal number of replicates and also the variability of field trials. The datasets were evaluated for outliers, normality, and equal variance assumptions using the diagnostics function in R. Identified outliers were removed from the dataset and not used in the final analyses. All figures were created using the ggplot2 package in R (Wickham, 2009). All analyses were conducted in R version 3.5 (R Development Core Team, 2017).
Results
Soil Inorganic N
Four weeks after lactobionate was broadcasted for the winter wheat trial, we observed no significant difference in soil nitrate between the control treatment (0 L ha−1) and the intermediate treatment (159 L ha−1) at both soil depths (0–5 cm, 5–15 cm). However, we did observe a decreasing trend and significant reduction in soil nitrate concentration at higher lactobionate application rates at both soil depths examined (p = 0.03; Figures 2A,B). Soil ammonium did not differ significantly between the treatments in the surface soil (0–5 cm), but in the deeper soil (5–15 cm), a significant increase (p = 0.0371) was observed in the 318 L ha−1 treatment as compared with the control (Supplementary Information). Surface soil had greater soil nitrate and ammonium concentration across all treatment as compared to the deeper soil.
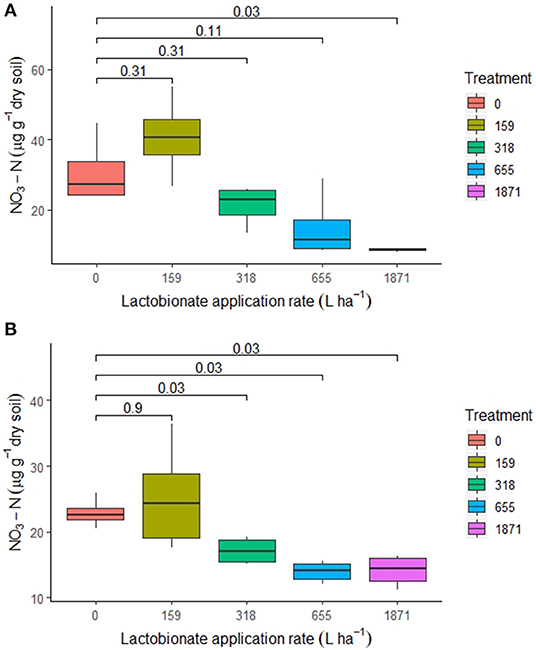
Figure 2. (A) Effect of lactobionate application on soil nitrate-N for wheat trial (0–5 cm). (B) Effect of lactobionate application on soil nitrate-N for wheat trial (5–15 cm). Horizontal lines and p-values above each boxplot is the pairwise comparison of each treatment and control.
Soil C and N
Total soil C content in the surface soil ranged from 1.06 to 1.2% for the surface soil and from 0.67 to 0.8% for the lower depth across all treatments (Table 1). There were no significant differences between control and treatment plots in total C content (p = 0.22) across soil depths. Total soil N in the surface soil ranged from 0.10 to 0.12% and 0.07 to 0.08% in the deeper soil across treatments. Similar to C, no significant differences in total N were observed between control and treatments across soil depth (p = 0.15). However, total soil C and N were higher in the surface soil as compared to deeper soil.
Soil Moisture
Mean gravimetric moisture content ranged from 0.12 to 0.15 g g−1 across treatments and depths while volumetric moisture content ranged from 10 to 27% (Figures 3A,B, Supplementary Information). The only significant treatment effect in the surface soil was between control and the 655 L ha−1 treatment (p = 0.027). We also observed higher gravimetric moisture content for treatments 159, 318, and 655 L ha−1 compared to control at the deeper soil depth (p = 0.036). Volumetric moisture content was measured at 4 and 8 weeks after lactobionate application with the aid of a moisture sensor probe. Higher volumetric moisture content was found in the higher application treatments (318, 655, and 1,871 L ha−1) as compared with the control when measured in October 2017, but by November 2017, a drastic fall in moisture content occurred across all treatments and there were no clear differences at that point (Supplementary Information).
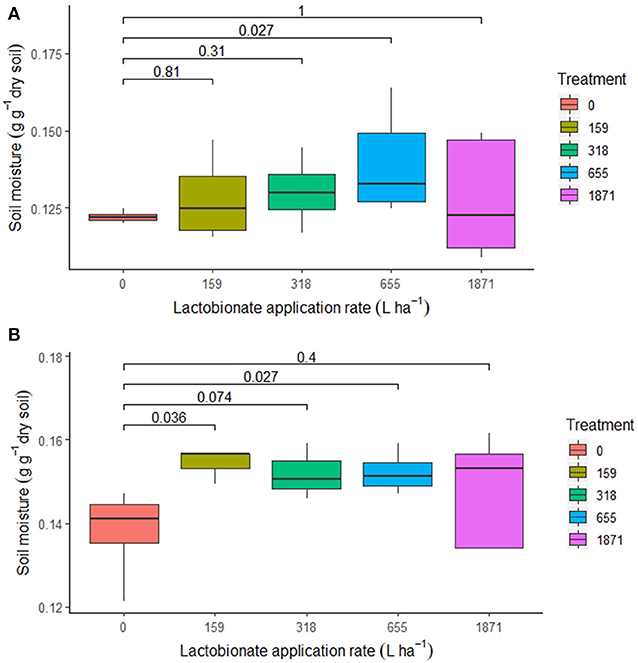
Figure 3. (A) Effect of lactobionate application on gravimetric soil moisture for wheat trial (0–5 cm). (B) Effect of lactobionate application on gravimetric soil moisture for wheat trial (5–15 cm). Horizontal lines and p-values above each boxplot is the pairwise comparison of each treatment and control.
Soil Microbial Biomass C and N
No differences were observed in microbial biomass C and N among treatments in the surface soil (p = 0.21; Table 1). The mean microbial biomass C and N in the surface soil ranged from 19 to 23 μg g−1 dry soil and 0.9 to 1.65 μg g−1 dry soil, respectively, and across treatments. In the deeper soil depth examined, we found higher microbial biomass C for the 318 and 1,871 L ha−1 treatments (p = 0.095, p = 0.06) as compared with the control (Table 1), while there was also no observable difference in microbial biomass N. However, the surface soil had higher microbial biomass C and N as compared with the deeper soil.
Wheat Yield and Grain C and N Content
Wheat yield ranged between 5,224 and 5,497 kg ha−1 across treatments (Figure 4). There was no significant difference in wheat yield between control plots and lactobionate-amended plots. There was also no observable difference in grain protein content between control and treatment plots (p > 0.1; Supplementary Information).
Corn Trial
Soil C and N
Lactobionate application did not influence soil C and N at either soil depth (p > 0.1). Top soil C (0.67–0.75%) was however higher than subsoil C (0.5–0.55%) across all treatments and a similar trend observed for soil N (Table 2).
Soil Inorganic N
Lactobionate application did not alter soil nitrate relative to unamended soils for both surface and deeper soil (p > 0.1; Table 3). Surface soil nitrate concentration across all treatments was higher than deeper soil. Average soil nitrate concentration ranged from 31 to 39 μg g−1 dry soil for surface soil and from 11 to 16 μg g−1 dry soil for deeper soil across all treatments. Average soil ammonium concentration in the surface soil ranged from 11 to 47 μg g−1 drysoil and from 3 to 9 μg g−1 dry soil for the lower depths across all treatments (Supplementary Information). Lactobionate applied at 374 L ha−1 significantly decreased surface soil ammonium (p = 0.052). There was no effect of lactobionate application on the deeper soil ammonium concentration.
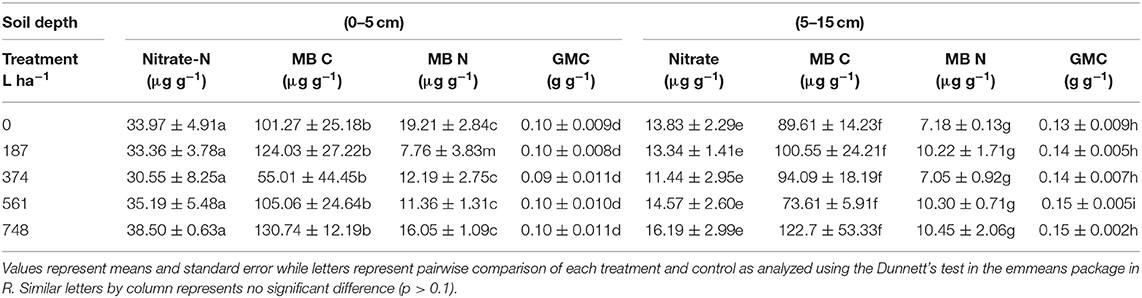
Table 3. Effect of lactobionate application rates on soil nitrate-N, microbial biomass C and N and gravimetric moisture content (GMC) across soil depths (0–5 cm, 5–15 cm) for the corn field trial.
Soil Moisture
Average gravimetric soil moisture ranged from 0.09 to 0.10 g g−1 in the surface soil and 0.13 to 0.15 g g−1 in the deeper soil across treatments (Table 3). No significant differences were observed in the gravimetric moisture content of lactobionate-amended soils and control soils at both soil depths sampled (p > 0.1). However, soil moisture tended to be slightly higher in the deeper soil with the 561 and 748 L ha−1 treatments than the control treatment. Likewise, no significant differences were observed in the volumetric moisture content of the treatment and control plots (data not shown).
Soil Microbial Biomass C and N
Average microbial biomass C at both soil depths sampled ranged from 73 to 125 μg g−1 dry soil, and there was no observable significant difference (p > 0.1) between treatments at both soil depths (Table 3). Microbial biomass N decreased in the 187 L ha−1 treatment (p = 0.061) as compared to control and also showed a decreasing trend, although this was not statistically significant in the surface soil. However, in the deeper soil, an increase in microbial biomass N was observed in the 561 L ha−1 treatment as compared to the control (p = 0.081).
Corn Yield and Grain C and N Content
Corn yield ranged from 3,565 to 4,080 kg ha−1 across treatments (Figure 5). Lactobionate application did not have a significant effect on corn yield (p > 0.1), however, a consistent increase in yield with increasing application rate was observed. Corn grain protein content did not increase or decrease with lactobionate application (p > 0.1; Supplementary Information).
Discussion
Agronomic Practices
While it is common practice to apply fertilizers and soil amendments directly to soil by spraying or broadcasting, recent studies have shown that a more efficient way of amendment application is subsurface banding. Subsurface banding of fertilizers has been shown to reduce ammonia volatilization of fertilizers (Bouwmeester et al., 1985), increase crop yield (Stevens et al., 2007), increase plant nitrogen use efficiency (Malhi et al., 2001; Sommer et al., 2004), and reduce nutrient leaching and runoff (Watts et al., 2011; Lamba et al., 2013). In our field experiments, the application of lactobionate through two different modes (broadcasting and banding) on wheat and corn, respectively, was not done to compare and contrast the more efficient method but to assess the impact of lactobionate under different systems to better elucidate if these impacts can be broadly applied across systems or are unique to a particular system. The key effects seen with broadcast application in wheat were an increase in soil moisture at the deeper soil depth, an increase in microbial biomass and a decrease in soil nitrate after 4 weeks of application. However, substantial rainfall events immediately after lactobionate broadcast application may have resulted in much of the lactobionate to leach through the soil profile or lost through overland water flow. This, along with evidence for the benefits of banding amendments described above, led us to apply lactobionate through banding in the corn trial. We believed that concentrating the byproduct at the zone of planting will prevent it from being easily leached or washed off while also maximizing its effect on the key indicators of interest.
Overall, with banding in the corn trial, no major effects were seen on the soil properties we measured. This may be a result of the fact that soils were not collected at the exact zone of banding (since this was where the corn was seeded) but between corn rows. Moreover, the differences in the lactobionate effects between the two trials may have been co-founded by season, as the corn lactobionate application was conducted in the summer as compared to the fall for the winter wheat trial. However, a key observation in the corn trial was the increasing trend in corn yield with lactobionate application rate. Other studies have also shown that subsurface banding can improve crop yield as shown in a study involving subsurface banding of poultry litter that increased the yield of cotton by 22% (Tewolde et al., 2015) and the banding of nitrogen fertilizer that increased sugarbeet yield (Stevens et al., 2007). While this is not an attempt to compare the most efficient mode of application considering the differences in crop, planting season and year, we suspect that the different application modes contribute to the varying degree of influence of lactobionate we observed on soil and crop properties between corn and wheat.
Soil Moisture
Given the chemical properties of lactobionate, we anticipated that it could be effective at increasing water retention in dryland cropping systems and thus be a potential amendment for mitigating plant drought stress. The potential for lactobionate to increase soil moisture could be controlled by four key mechanisms: cations (potassium, K+) that can improve soil aggregation by binding to negatively charged soil particles; hydroxyl groups (OH) present in lactobionate which directly improve water absorption; lactobionate-mediated increases in soil organic C (SOC) leading to increased soil aggregation and water sorption; and lactobionate-stimulated increase in microbial biomass resulting in higher SOC retention and biogenic aggregation via microbial polymeric exudation (Kallenbach et al., 2019).
We examined the effect of lactobionate application on soil moisture using two metrics (gravimetric and volumetric) at two soil depths and observed different effects in the dryland wheat and corn trials. For the wheat field soils, lactobionate applied at even the lowest rate (159 L ha−1) had a positive effect on gravimetric soil moisture in the subsoil while a high application rate (655 L ha−1) led to increased soil moisture in the surface soils. No effects were observed at 1,871 L ha−1 and this may be a result of overloading the soils with organic material to the point where it was clogging soil pores, limiting infiltration and hydraulic conductivity (Robbins and Lehrsch, 1998). The positive effect of lactobionate on wheat soil moisture in the subsoil might be the result of multiple rainfall episodes that occurred after its application in the wheat field trial, potentially transporting lactobionate deeper into the soil profile.
Adding materials rich in organic C such as sucrose and sawdust have also been found to increase soil moisture levels (Blumenthal et al., 2003; Averett et al., 2004). In a previous study, cheese whey applied directly to soils via furrow irrigation increased soil aggregate stability and infiltration, thus reducing erosion and enhancing water retention (Lehrsch et al., 2008). Furthermore, the volumetric moisture content of the wheat field soils was greatest under the highest lactobionate application rate as compared to the control for October 2017. However, by November, no differences were observed for volumetric moisture content of the treatments. For corn field soils, no clear differences in either gravimetric or volumetric soil moisture were seen at either soil depth. These differences in effects between the wheat field and corn field soils may be explained by the timing and mode of lactobionate application in each field, along with inherent differences in crop physiology and water uptake. Lactobionate was sprayed across the wheat field soils during the fall season while lactobionate was banded into the soil at the exact zone of planting for the corn field soils in the summer. As the soil samples were not collected from the exact banding zone but adjacent to it (in between corn rows), this may be a contributing factor to the lack of differences between control and treatment rates in the corn trial. Also, the differences seen by month (October vs. November) may imply that a one-time application may not be enough to see desired changes in soil moisture retention.
Soil Nitrogen
In dryland systems, following water, the next most often limiting factor for crop productivity is plant available nutrients, especially N. The global use of chemical fertilizers addresses N deficiencies, but has also contributed to greenhouse gas emissions and eutrophication. The addition of high C organic materials including lactobionate to soils could potentially alter microbial-mediated N cycling, primarily by stimulating microbial immobilization and subsequent turnover. We measured the impact of lactobionate application on soil nitrate and ammonium, to understand potential effects on N mineralization and crop available N. While we observed a significant decline in soil nitrate at intermediate to high levels of lactobionate applied at both soil depths for wheat field soils, we did not see this trend in the corn field soils.
The decline in soil nitrate may be a result of the high level of bioavailable C present in lactobionate which might have stimulated a temporal microbial immobilization of N. A similar trend has also been observed in field and laboratory studies of relatively high C organic materials (sucrose, wheat straw, vinasses, and sawdust) on soil N dynamics (Blumenthal et al., 2003; Averett et al., 2004; Ghani et al., 2005; Baruah et al., 2016; Moran-Salazar et al., 2016). This effect could be either beneficial or deleterious to soils and crop productivity depending on timing and frequency of application. If microbes feeding on C-rich lactobionate assimilate soil N into their biomass, when they eventually turnover, N is released for plant uptake. Also, the slight increase in soil nitrate seen in the 159 L ha−1 treatment may indicate that this rate was stimulating microbial activity just enough to increase N mineralization without inducing N limitations. However, at higher rates, microbial biomass may cross a threshold, moving from C to N limitations. Plant nitrate uptake could also be another reason for the decline in soil nitrate as plants are often better competitors for nitrate compared to microbes. Thus, the combination of plant mineral N uptake coupled with increased microbial biomass due to lactobionate application could have led to this temporal decline in soil nitrate. Lactobionate application did not significantly impact the level of soil ammonium in either the wheat or corn trials at both soil depths examined. This may be due to the higher concentration of nitrate supplied via fertilizer application. The application of C-rich organic materials including lactobionate to soils has previously been suggested to help reduce nitrate leaching and ammonia volatilization by sequestering soil mineral N in microbial biomass (Manevski et al., 2016; Cao et al., 2018).
Soil Microbial Biomass
Biological management of agricultural nutrient cycling can reduce nutrient losses and support microbial mineralization of organic N (Drinkwater et al., 2017). Soil organic amendments are a key tool to enhance soil nutrient cycling since they provide a readily available source of energy for the microbial community. Supporting high levels of microbial activity may also lead to SOM formation and soil aggregate stability which in turn can increase soil water retention (Murphy, 2015; Kallenbach et al., 2016). The challenge however in using soil amendments is balancing soil organic inputs with the C and N requirements of both the microbial community and crop needs.
In this field experiment, we determined how soil microbes respond to lactobionate application by measuring their biomass C and N. The increase we observed in microbial biomass C at the lower soil depth in wheat parallels what we observed for soil moisture and is not unexpected, as lactobionate contains monosaccharides fueling microbial metabolism. Our results are consistent with other studies that have observed an increase in microbial biomass C and N in soils treated with dairy effluents (Degens et al., 2000; Sparling et al., 2001; Sarathchandra et al., 2006). This increase in microbial biomass could decrease soil organic C mineralization by selecting for microbes with greater ability to degrade lactose and its derivatives rather than SOM (Degens et al., 2000).
The observable changes in microbial biomass C within 4 weeks of amendment application is not surprising as this pool is known to have a short turnover time and with high sensitivity to environmental changes and management relative to other soil C pools (Joergensen and Emmerling, 2006; Kallenbach and Grandy, 2011). Additions of labile C materials including sucrose and lactobionate to soils have temporarily increased microbial biomass and activity (Török et al., 2000; Eschen et al., 2006; Kallenbach et al., 2019). In contrast, the addition of C sources such as wheat straw and sawdust containing structurally complex molecules requiring enzymatic degradation are likely to have less of an impact on elevating microbial biomass (Dalenberg and Jager, 1981; Magill and Aber, 2000). Thus, the impact of a soil amendment on the soil microbial biomass will depend primarily on how labile or recalcitrant its C source is. While a clear change in biomass C was observed in the winter wheat field experiment, there was no observable effect on the total soil C. This could be as a result of the lower biomass input rates relative to the total soil C pool and changes may have occurred in only certain SOC fractions. For instance, increases in pools with relatively faster turnover times, such as the particulate organic C and the mineral associated organic C pools would not be detectable from our total SOC measurements.
Lactobionate Impact on Wheat and Corn
Ultimately, for a soil amendment to be adopted, its effects on soil health must translate into increased crop yield and/or quality, or reduced input costs. Thus, we assessed the effect of lactobionate on yield and protein content. For the wheat trial, lactobionate had no significant effect on yield. Wheat and corn grain protein content was also not significantly affected by lactobionate application. While corn yield was not significantly affected by lactobionate, an increase in yield with increasing lactobionate application rate was observed (up to 14% at the highest application rate), suggesting a dose-dependent benefit on corn yield. The differences between treatment effects on corn and wheat grain yields could be a result of multiple environmental and physiological factors associated with the crops, along with the different application modes. The length (8 months) and growing season (winter, spring, summer) of winter wheat as compared to that of corn (5 months, summer, fall) could partly account for the difference in lactobionate effects on yields of the two crops. Winter wheat is likely to experience greater nutrient and water limitation as compared to corn, with just a single application of the amendment (Chen et al., 2016; Manevski et al., 2016). The timing of lactobionate application could have also played a role, as lactobionate applied during the summer (for the corn trial) could have stimulated greater microbial activity which in turn could affect N cycling dynamics as compared to fall application at lower temperatures.
The similarity in wheat and corn grain protein between lactobionate-amended plots and control could have been as a result of the one-time application of lactobionate at the early stage of crop growth, when effects on soil mineral N might have been short-lived. Despite the reduction in soil nitrate after 4 weeks of lactobionate application for the winter wheat trial, this did not result in any deleterious effect on the wheat grain yield and protein content. Furthermore, the application of soil amendments such as compost, manure, straw, biochar, and other materials rich in organic C has shown multiple inconsistencies on crop yields especially in the short term due to differences in crop, climate and location (Christian et al., 1999; Malhi and Lemke, 2007; Coulter and Nafziger, 2008).
Conclusion
We explored the potential benefits of lactobionate, a byproduct of cheese manufacturing, on soil properties and crop productivity in wheat and corn dryland systems. Our findings suggest that lactobionate has the potential to be an effective soil amendment based on the benefits we observed in our study, but it depended on the agronomic system under evaluation. Lactobionate caused a temporary increase in gravimetric soil water content and decrease in soil nitrate in the wheat trial but showed no effect in the corn trial. This suggests that timing, mode, and frequency of application needs to be further optimized for maximal soil benefits of lactobionate. The limited statistical power relative to plot variability in this trial constrained our ability to conclusively determine effects of lactobionate on crop yields. Like many soil amendments and management approaches, effects on soil properties may accumulate with repeated treatments and effects on crop yield or quality can take several years to manifest. This is a grand challenge for the adoption of soil amendments, as farmers often make purchasing decisions based on short-term returns on investment and discount long-term benefits. On the other hand, technologies like lactobionate also benefit society by diverting food waste from landfills to the farm, where they can potentially decrease the environmental impact of agriculture and move toward a regenerative, circular economy. Given the potential benefits to farmers and global sustainability, more long term-field trials encompassing different crops and field sites are required to conclusively determine the potential benefits of lactobionate as a soil amendment.
Data Availability Statement
All datasets generated for this study are included in the article/Supplementary Material.
Author Contributions
OO was primarily responsible for experimental design, data collection, analysis, and all manuscript drafts. CK and MW were responsible for experimental design, contributed to data collection, and manuscript revisions. JS, FC, and MV were responsible for field operations and contributed to data collections. All authors contributed to manuscript draft revisions and contributed to conceptualization and design of experiment.
Funding
This study was supported by a donation to the Colorado State University Foundation made by Leprino Foods Company, Denver CO.
Disclaimer
The use of trade, firm, or corporation names is for the information and convenience of the reader. Such use does not constitute an official endorsement or approval by the United States Department of Agriculture or the Agricultural Research Service of any product or service to the exclusion of others that may be suitable. The USDA prohibits discrimination in all its programs and activities on the basis of race, color, national origin, age, disability, and where applicable, sex, marital status, familial status, parental status, religion, sexual orientation, genetic information, political beliefs, reprisal, or because all or part of an individual's income is derived from any public assistance program.
Conflict of Interest
The authors declare that the research was conducted in the absence of any commercial or financial relationships that could be construed as a potential conflict of interest.
Acknowledgments
Leprino Foods Company provided the lactobionate used in this study and supported this work through a donation to the Colorado State University Foundation. We are grateful for technical support provided by the EcoCore Analytical Laboratory, Colorado State University.
Supplementary Material
The Supplementary Material for this article can be found online at: https://www.frontiersin.org/articles/10.3389/fsufs.2019.00127/full#supplementary-material
References
Affertsholt-Allen, T. (2007). “Market developments and industry challenges for lactose and lactose derivatives,” in Proceedings of the International Dairy Federation Symposium “Lactose and Its Derivatives” (Moscow). Available online at: https://authorzilla.com/xGQd7/market-developments-and-industry-challenges-for-lactose-and.html (accessed February 12, 2019)
Alonso, S., Rendueles, M., and Díaz, M. (2013). Bio-production of lactobionic acid: current status, applications and future prospects. Biotechn. Adv. 31, 1275–1291. doi: 10.1016/j.biotechadv.2013.04.010
Annabi, M., Le Bissonnais, Y., Le Villio-Poitrenaud, M., and Houot, S. (2011). Improvement of soil aggregate stability by repeated applications of organic amendments to a cultivated silty loam soil. Agric. Ecosyst. Environ. 144, 382–389. doi: 10.1016/j.agee.2011.07.005
Association of Official Analytical Chemists (1984). Official Methods of Analysis of Association of Official Analytical Chemists, 14th Ed. Arlington, VA: Association of Official Analytical Chemists, 259.
Averett, J. M., Klips, R. A., Nave, L. E., Frey, S. D., and Curtis, P. S. (2004). Effects of soil carbon amendment on nitrogen availability and plant growth in an experimental tallgrass prairie restoration. Restor. Ecol. 12, 568–574. doi: 10.1111/j.1061-2971.2004.00284.x
Baruah, A., Bordoloi, N., and Baruah, K. K. (2016). Effect of organic amendments with varied CN ratios on grain productivity and nitrous oxide (N2O) emission from wheat grown in alluvial soil. Aust. J. Crop Sci. 10, 460–469. doi: 10.21475/ajcs.2016.10.04.p6970x
Berruga, M. I., Jaspe, A., and San-Jose, C. (1997). Selection of yeast strains for lactose hydrolysis in dairy effluents. Int. Biodeter. Biodeg. 40, 119–123. doi: 10.1016/S0964-8305(97)00053-X
Blumenthal, D. M., Jordan, N. R., and Russelle, M. P. (2003). Soil carbon addition controls weeds and facilitates prairie restoration. Ecol. Appl. 13, 605–615. doi: 10.1890/1051-0761(2003)013[0605:SCACWA]2.0.CO;2
Bond, B. (2013). Old Confusions. Sewanee Review 121, 43–45. Johns Hopkins University Press. Retrieved January 21, 2019, from Project MUSE database. doi: 10.1353/sew.2013.0011
Bouwmeester, R. J. B., Vlek, P. L. G., and Stumpe, J. M. (1985). Effect of environmental factors on ammonia volatilization from a urea-fertilized soil 1. Soil Sci. Soc. Am. J. 49, 376–381. doi: 10.2136/sssaj1985.03615995004900020021x
Calderón, F. J., Benjamin, J., and Vigil, M. F. (2015). A comparison of corn (Zea mays L.) residue and its biochar on soil C and plant growth. PLoS ONE 10:e0121006. doi: 10.1371/journal.pone.0121006
Cao, Y., Sun, H., Zhang, J., Chen, G., Zhu, H., Zhou, S., et al. (2018). Effects of wheat straw addition on dynamics and fate of nitrogen applied to paddy soils. Soil Tillage Res. 178, 92–98. doi: 10.1016/j.still.2017.12.023
Carvalho, F., Prazeres, A. R., and Rivas, J. (2013). Cheese whey wastewater: characterization and treatment. Sci. Total Environ. 445, 385–396. doi: 10.1016/j.scitotenv.2012.12.038
Cassman, K. G. (1999). Ecological intensification of cereal production systems: yield potential, soil quality, and precision agriculture. Proc. Natl. Acad. Sci. U.S.A. 96, 5952–5959. doi: 10.1073/pnas.96.11.5952
Chaparro, J. M., Sheflin, A. M., Manter, D. K., and Vivanco, J. M. (2012). Manipulating the soil microbiome to increase soil health and plant fertility. Biol. Fertil. Soils 48, 489–499. doi: 10.1007/s00374-012-0691-4
Chen, Y., Zhang, Z., Wang, P., Song, X., Wei, X., and Tao, F. (2016). Identifying the impact of multi-hazards on crop yield—a case for heat stress and dry stress on winter wheat yield in northern China. Eur. J. Agron. 73, 55–63. doi: 10.1016/j.eja.2015.10.009
Christian, D. G., Bacon, E. T. G., Brockie, D., Glen, D., Gutteridge, R. J., and Jenkyn, J. F. (1999). Interactions of straw disposal methods and direct drilling or cultivations on winter wheat (Triticum aestivum) grown on a clay soil. J. Agric. Eng. Res. 73, 297–309. doi: 10.1006/jaer.1999.0423
Commission for Environmental Cooperation (2017). Characterization and Management of Food Loss and Waste in North America. Foundational Report. Prepared for CEC by Tetra Tech Canada. Montreal, QC: Commission for Environmental Cooperation.
Coulter, J. A., and Nafziger, E. D. (2008). Continuous corn response to residue management and nitrogen fertilization. Agron. J. 100, 1774–1780. doi: 10.2134/agronj2008.0170
Dairy Processing Handbook (2014). Available online: https://ia902305.us.archive.org/24/items/DairyProcessingHandbookTetrapak/Dairy-Processing-Handbook-Tetrapak.pdf (accessed January 10, 2019).
Dalenberg, J. W., and Jager, G. (1981). Priming effect of small glucose addition to 14C-labelled soil. Soil Biol. Biochem. 13, 219–223. doi: 10.1016/0038-0717(81)90024-9
Degens, B. P., Schipper, L. A., Claydon, J. J., Russell, J. M., and Yeates, G. W. (2000). Irrigation of an allophanic soil with dairy factory effluent for 22 years: responses of nutrient storage and soil biota. Soil Res. 38, 25–36. doi: 10.1071/SR99063
Doran, J. W. (2002). Soil health and global sustainability: translating science into practice. Agric. Ecosyst. Environ. 88, 119–127. doi: 10.1016/S0167-8809(01)00246-8
Doran, J. W., and Parkin, T. B. (1994). “Defining and assessing soil quality,” in ‘Defining soil quality for a sustainable environment'. SSSA Special Publication No. 35, eds J. W. Doran, D. C. Coleman, D. F. Bezdicek, and B. A. Stewart (Madison, WI: Soil Science Society of America Inc.), 3–21.
Drinkwater, L. E., Schipanski, M., Snapp, S., and Jackson, L. E. (2017). “Ecologically based nutrient management,” in Agricultural Systems: Agroecology and Rural Innovation for Development, eds S. S. Snapp and B. Pound (Burlington, MA: Academic Press, 159–208.
Eden, M., Gerke, H. H., and Houot, S. (2017). Organic waste recycling in agriculture and related effects on soil water retention and plant available water: a review. Agron. Sustain. Dev. 37, 11. doi: 10.1007/s13593-017-0419-9
Eschen, R., Müller-Schärer, H., and Schaffner, U. (2006). Soil carbon addition affects plant growth in a species-specific way. J. Appl. Ecol. 43, 35–42. doi: 10.1111/j.1365-2664.2005.01110.x
Fereidooni, M., Raiesi, F., and Fallah, S. (2013). Ecological restoration of soil respiration, microbial biomass and enzyme activities through broiler litter application in a calcareous soil cropped with silage maize. Ecol. Eng. 58, 266–277. doi: 10.1016/j.ecoleng.2013.06.032
Food and Agriculture Organization (2013). The State of Food Insecurity in the World 2013. The Multiple Dimensions of Food Security. Rome: Food and Agriculture Organization.
Gänzle, M. G., Haase, G., and Jelen, P. (2008). Lactose: crystallization, hydrolysis and value-added derivatives. Int. Dairy J. 18, 685–694. doi: 10.1016/j.idairyj.2008.03.003
Ghani, A., Dexter, M., Sarathchandra, U., and Waller, J. (2005). Effects of dairy factory effluent application on nutrient transformation in soil. N. Zeal. J. Agric. Res. 48, 241–253. doi: 10.1080/00288233.2005.9513653
Green, B. A., Yu, R. J., and Van Scott, E. J. (2009). Clinical and cosmeceutical uses of hydroxyacids. Clin. Dermatol. 27,495–501. doi: 10.1016/j.clindermatol.2009.06.023
Gugino, B. K., Idowu, O. J., Schindelbeck, R. R., van Es, H. M., Wolfe, D. W., Moebius, B. N., et al. (2009). Cornell Soil Health Assessment Training Manual, 2nd Edn. Geneva: Cornell University.
Guimarães, P. M., Teixeira, J. A., and Domingues, L. (2010). Fermentation of lactose to bio-ethanol by yeasts as part of integrated solutions for the valorisation of cheese whey. Biotechnol. Adv. 28, 375–384. doi: 10.1016/j.biotechadv.2010.02.002
Gunders, D., and Bloom, J. (2017). Wasted: How America is Losing Up To 40 Percent of Its Food From Farm to Fork to Landfill. New York, NY: Natural Resources Defense Council. Available online at: https://www.nrdc.org/sites/default/files/wasted-food-IP.pdf (accessed March 1, 2019).
Gutiérrez, L. F., Hamoudi, S., and Belkacemi, K. (2012). Lactobionic acid: a high value-added ‘lactose derivative for food and pharmaceutical applications. Int. Dairy J. 26, 103–111. doi: 10.1016/j.idairyj.2012.05.003
Hargreaves, J. C., Adl, M. S., and Warman, P. R. (2008). A review of the use of composted municipal solid waste in agriculture. Agric. Ecosyst. Environ. 123, 1–14. doi: 10.1016/j.agee.2007.07.004
Janczukowicz, W., Zielinski, M., and Debowski, M. (2008). Biodegradability evaluation of dairy effluents originated in selected sections of dairy production. Bioresour. Technol. 99, 4199–4205. doi: 10.1016/j.biortech.2007.08.077
Joergensen, R. G., and Emmerling, C. (2006). Methods for evaluating human impact on soil microorganisms based on their activity, biomass, and diversity in agricultural soils. J. Plant Nutr. Soil Sci. 169, 295–309. doi: 10.1002/jpln.200521941
Kallenbach, C., and Grandy, A. S. (2011). Controls over soil microbial biomass responses to carbon amendments in agricultural systems: a meta-analysis. Agric. Ecosyst. Environ. 144, 241–252. doi: 10.1016/j.agee.2011.08.020
Kallenbach, C. M., Conant, R. T., Calderón, F., and Wallenstein, M. D. (2019). A novel soil amendment for enhancing soil moisture retention and soil carbon in drought-prone soils. Geoderma 337, 256–265. doi: 10.1016/j.geoderma.2018.09.027
Kallenbach, C. M., Frey, S. D., and Grandy, A. S. (2016). Direct evidence for microbial-derived soil organic matter formation and its ecophysiological controls. Nat. Commun. 7:13630. doi: 10.1038/ncomms13630
Kibblewhite, M. G., Ritz, K., and Swift, M. J. (2008). Soil health in agricultural systems. Philos. Trans. R Soc. A 363, 685–701. doi: 10.1098/rstb.2007.2178
Ko, J., Ahuja, L. R., Saseendran, S. A., Green, T. R., Ma, L., Nielsen, D. C., et al. (2012). Climate change impacts on dryland cropping systems in the Central Great Plains, USA. Clim. Change 111, 445–472. doi: 10.1007/s10584-011-0175-9
Lal, R. (2014). Societal value of soil carbon. J. Soil Water Conserv. 69, 186A–192A. doi: 10.2489/jswc.69.6.186A
Lal, R. (2016). Soil health and carbon management. Food Energy Security 5, 212–222. doi: 10.1002/fes3.96
Lamba, J., Srivastava, P., Way, T. R., Sen, S., Wood, C., and Yoo, K. H. (2013). Nutrient loss in leachate and surface runoff from surface-broadcast and subsurface-banded broiler litter. J. Environ. Qual. 42, 1574–1582. doi: 10.2134/jeq2013.02.0064
Lehrsch, G. A., Robbins, C. W., and Brown, M. J. (2008). Whey utilization in furrow irrigation: Effects on aggregate stability and erosion. Bioresour. Technol. 99, 8458–8463. doi: 10.1016/j.biortech.2008.02.050
Luo, G., Li, L., Friman, V. P., Guo, J., Guo, S., Shen, Q., et al. (2018). Organic amendments increase crop yields by improving microbe-mediated soil functioning of agroecosystems: a meta-analysis. Soil Biol. Biochem. 124, 105–115. doi: 10.1016/j.soilbio.2018.06.002
Magill, A. H., and Aber, J. D. (2000). Variation in soil net mineralization rates with dissolved organic carbon additions. Soil Biol. Biochem. 32, 597–601. doi: 10.1016/S0038-0717(99)00186-8
Malhi, S. S., Grant, C. A., Johnston, A. M., and Gill, K. S. (2001). Nitrogen fertilization management for no-till cereal production in the Canadian Great Plains: a review. Soil Tillage Res. 60, 101–122. doi: 10.1016/S0167-1987(01)00176-3
Malhi, S. S., and Lemke, R. (2007). Tillage, crop residue and N fertilizer effects on crop yield, nutrient uptake, soil quality and nitrous oxide gas emissions in a second 4-yr rotation cycle. Soil Tillage Res. 96, 269–283. doi: 10.1016/j.still.2007.06.011
Malik, M. A., Khan, K. S., Marschner, P., and Ali, S. (2013). Organic amendments differ in their effect on microbial biomass and activity and on P pools in alkaline soils. Biol. Fertil. Soils 49, 415–425. doi: 10.1007/s00374-012-0738-6
Manevski, K., Børgesen, C. D., Li, X., Andersen, M. N., Zhang, X., Abrahamsen, P., et al. (2016). Optimising crop production and nitrate leaching in China: measured and simulated effects of straw incorporation and nitrogen fertilisation. Eur. J. Agron. 80, 32–44. doi: 10.1016/j.eja.2016.06.009
Moran-Salazar, R. G., Sanchez-Lizarraga, A. L., Rodriguez-Campos, J., et al. (2016). Utilization of vinasses as soil amendment: consequences and perspectives. SpringerPlus 5:1007. doi: 10.1186/s40064-016-2410-3
Morison, J. I. L., Baker, N. R., Mullineaux, P. M., and Davies, W. J. (2007). Improving water use in crop production. Philos. Trans. R. Soc. B 363, 639–658. doi: 10.1098/rstb.2007.2175
Murphy, B. W. (2015). Impact of soil organic matter on soil properties—a review with emphasis on Australian soils. Soil Res. 53, 605–635. doi: 10.1071/SR14246
Ninh, H. T., Grandy, A. S., Wickings, K., Snapp, S. S., Kirk, W., and Hao, J. (2015). Organic amendment effects on potato productivity and quality are related to soil microbial activity. Plant Soil 386, 223–236.
Oldeman, L. R. (1994). “The global extent of soil degradation,” in Soil Resilience and Sustainable Landuse, eds D. J. Greenland, and I. Szabolcs (Wallingford: CAB International, 99–119.
Petersen, S. O., Sommer, S. G., Béline, F., Burton, C., Dach, J., et al. (2007). Recycling of livestock manure in a whole-farm perspective. Livest. Sci. 112, 180–191. doi: 10.1016/j.livsci.2007.09.001
Prazeres, A. R., Carvalho, F., and Rivas, J. (2012). Cheese whey management: a review. J. Environ. Manage. 110, 48–68. doi: 10.1016/j.jenvman.2012.05.018
R Development Core Team (2017). R: A Language and Environment for Statistical Computing. Vienna: R Foundation for Statistical Computing.
ReFED (2016). A Roadmap to Reduce U.S. Food Waste by 20 Percent. Available online at: https://www.refed.com/downloads/ReFED_Report_2016.pdf (accessed November 2016).
Robbins, C. W., Hansen, C. L., Roginske, M. F., and Sorensen, D. L. (1996). Extractable potassium and soluble calcium, magnesium, sodium, and potassium in two whey-treated calcareous soils. J. Environ. Qual. 25, 791–795. doi: 10.2134/jeq1996.00472425002500040020x
Robbins, C. W., and Lehrsch, G. A. (1998). “Cheese whey as a soil conditioner”, in Handbook of Soil Conditioners: Substances that Enhance the Physical Properties of Soil, eds A. Wallace and R. E. Terry (New York, NY: Marcel Dekker Inc.), 167–186.
Robertson, A. D., Zhang, Y., Sherrod, L. A., Rosenzweig, S. T., Ma, L., Ahuja, L., et al. (2018). Climate change impacts on yields and soil carbon in row crop dryland agriculture. J. Environ. Qual. 47, 684–694. doi: 10.2134/jeq2017.08.0309
Sarathchandra, U., Ghani, A., Waller, J., Burch, G., Sayer, S., Waipara, N., et al. (2006). Impact of carbon-rich dairy factory effluent on growth of perennial ryegrass (Lolium perenne) and soil microorganisms. Eur. J. Soil Biol. 42, 13–22. doi: 10.1016/j.ejsobi.2005.08.002
Sommer, S. G., Schjoerring, J. K., and Denmead, O. T. (2004). Ammonia emission from mineral fertilizers and fertilized crops. Adv. Agron. 82, 82008–82004. doi: 10.1016/S0065-2113(03)82008-4
Sparling, G. P., Schipper, L. A., and Russell, J. M. (2001). Changes in soil properties after application of dairy factory effluent to New Zealand volcanic ash and pumice soils. Soil Res. 39, 505–518. doi: 10.1071/SR00043
Sriperm, N., Pesti, G. M., and Tillman, P. B. (2011). Evaluation of the fixed nitrogen-to-protein (N:P) conversion factor (6.25) versus ingredient specific N:P conversion factors in feedstuffs. J. Sci. Food Agric. 91, 1182–1186. doi: 10.1002/jsfa.4292
Stevens, W. B., Blaylock, A. D., Krall, J. M., Hopkins, B. G., and Ellsworth, J. W. (2007). Sugarbeet yield and nitrogen use efficiency with preplant broadcast, banded, or point-injected nitrogen application. Agron. J. 99, 1252–1259. doi: 10.2134/agronj2006.0357
Tewolde, H., Shankle, M. W., Way, T. R., Adeli, A., Brooks, J. P., and He, Z. (2015). Enhancing management of fall-applied poultry litter with cover crop and subsurface band placement in no-till cotton. Agron. J. 107, 449–458. doi: 10.2134/agronj14.0266
Tilman, D., Cassman, K. G., Matson, P. A., Naylor, R., and Polasky, S. (2002). Agricultural sustainability and intensive production practices. Nature 418, 671–677. doi: 10.1038/nature01014
Török, K., Szili-Koväcs, T., Halassy, M., Tóth, T., Hayek, Z., Paschke, M. W., et al. (2000). Immobilization of soil nitrogen as a possible method for the restoration of sandy grassland. Appl. Vegetation Sci. 3, 7–14. doi: 10.2307/1478913
United States Environmental Protection Agency (2016). Food Recovery Hierarchy. Available online at: https://www.epa.gov/sustainable-management-food/food-recovery-hierarchy (accessed January 20 2019).
United States Environmental Protection Agency (2019). Methane Emissions from Landfills. Available online at: https://www.epa.gov/lmop/basic-information-about-landfill-gas (accessed January 20, 2019).
Vance, E. D., Brookes, P. C., and Jenkinson, D. S. (1987). An extraction method for measuring soil microbial biomass C. Soil Biol. Biochem. 19, 703–707.
Vasilica, S. T. A. N., Virsta, A., Dusa, E. M., and Glavan, A. M. (2009). Waste recycling and compost benefits. Notulae Botanicae Horti Agrobotanici Cluj-Napoca 37, 9–13. doi: 10.15835/nbha3723461
Watts, D. B., Way, T. R., and Torbert, H. A. (2011). Subsurface application of poultry litter and its influence on nutrient losses in runoff water from permanent pastures. J. Environ. Qual. 40, 421–430. doi: 10.2134/jeq2010.0089
Wickham, H. (2009). ggplot2: Elegant Graphics for Data Analysis. New York, NY: Springer-Verlag. doi: 10.1007/978-0-387-98141-3
Wu, D. Q., Lu, B., Chang, C., Chen, C. S., Wang, T., Zhang, Y. Y., et al. (2009). Galactosylated fluorescent labeled micelles as a liver targeting drug carrier. Biomaterials 30, 1363–1371. doi: 10.1016/j.biomaterials.2008.11.027
Xie, H., Li, J., Zhu, P., Peng, C., Wang, J., He, H., et al. (2014). Long-term manure amendments enhance neutral sugar accumulation in bulk soil and particulate organic matter in a Mollisol. Soil Biol. Biochem. 78, 45–53. doi: 10.1016/j.soilbio.2014.07.009
Keywords: lactobionate, soil health, soil amendment, soil water retention, soil microbial biomass, soil nitrogen
Citation: Olayemi OP, Kallenbach CM, Schneekloth JP, Calderón FJ, Vigil MF and Wallenstein MD (2020) From Factory to Field: Effects of a Novel Soil Amendment Derived From Cheese Production on Wheat and Corn Production. Front. Sustain. Food Syst. 3:127. doi: 10.3389/fsufs.2019.00127
Received: 04 May 2019; Accepted: 23 December 2019;
Published: 28 January 2020.
Edited by:
Maria Pilar Bernal, Spanish National Research Council, SpainReviewed by:
Maria Luz Cayuela, Spanish National Research Council, SpainEngracia Madejon, Institute of Natural Resources and Agrobiology of Seville (CSIC), Spain
Copyright © 2020 Olayemi, Kallenbach, Schneekloth, Calderón, Vigil and Wallenstein. This is an open-access article distributed under the terms of the Creative Commons Attribution License (CC BY). The use, distribution or reproduction in other forums is permitted, provided the original author(s) and the copyright owner(s) are credited and that the original publication in this journal is cited, in accordance with accepted academic practice. No use, distribution or reproduction is permitted which does not comply with these terms.
*Correspondence: Oladapo P. Olayemi, cGV0ZXIub2xheWVtaSYjeDAwMDQwO2NvbG9zdGF0ZS5lZHU=