- 1Centre for Agroecology, Water and Resilence, Coventry University, Coventry, United Kingdom
- 2Game and Wildlife Conservation Trust, Fordingbridge, United Kingdom
- 3Institute of Biological, Environmental and Rural Science, University of Aberystwyth, Aberystwyth, United Kingdom
There is global concern that invertebrate populations are declining rapidly, particularly in agricultural habitats. Declines have been attributed to the intensification of farming systems, with many studies focussing on a lack of semi-natural habitat in the landscape and the use of insecticides. However, within-field arable weeds are also an important driver of invertebrate abundance and the ecosystem services to which they contribute. This study focuses on the role of arable weeds in supporting invertebrate populations and selected ecosystem services they deliver., using winter wheat as a case study. Weed-invertebrate relationships were investigated across seven studies of winter-sown wheat spanning 18 years. Both phytophagous and predatory invertebrates responded to weed cover but to different degrees. Phytophages showed a stronger positive relationship with weed cover than the predators, because they rely on the resources provided by the weeds whereas predatory species response is likely to be mediated by their prey. Farmland bird chick-food indices were positively related to both broadleaf and grass cover in cropped fields, indicating that increased weed cover can provide increased invertebrate food for birds in winter wheat. Despite this potential, there were insufficient invertebrate food resources for birds in the majority of wheat fields sampled. Weed diversity did not play a significant role in moderating the relationships between weeds and invertebrate abundance, however this may be a function of the low weed diversity in modern winter wheat fields. In this study the weed species most frequently shown to predict the invertebrate community were: Poa annua, Stellaria media, Fumaria officinalis, Sinapis arvensis, Senecio vulgaris, Persicaria lapathifolia, Sonchus spp., Matricaria discoidea, Persicaria maculosa, Agrostis spp., Lamium purpureum, Lamium album, Veronica spp., Atriplex spp., Myosotis spp. and Anagallis arvensis. We conclude that even in an intensively grown cereal, arable weeds can play an important role in maintaining and restoring invertebrate populations, that 10% weed cover is needed to fulfill the potential and that a successful outcome will be driven by the presence of weed species that support invertebrates that provide ecosystem services.
Introduction
The IPBES 2019 Global Assessment Report on Biodiversity and Ecosystem Services reports losses in diversity across taxa and explicitly warns of the associated loss of ecosystem services. Agriculture, which occupies 38% of the world's landmass, is cited as playing a major role in these declines (e.g., Lanz et al., 2018). The need to restore biodiversity on farmland has been highlighted in the scientific literature for many years (e.g., Herzog et al., 1970), but despite this, farmland biodiversity, across taxa, continues to decline. Increasingly there are reports suggesting that both arable weeds (Richner et al., 2015) and invertebrates (Hallmann et al., 2017; Sánchez-Bayo and Wyckhuys, 2019), are declining sharply across Europe, which could be expected to be reflected in the decline of taxa at higher trophic levels. A recent study that investigated bird population trends across Europe and Denmark found that insectivore and seed eating birds declined while omnivorous species remained relatively stable (Bowler et al., 2019). This trend was particularly strong among farmland specialists. The authors suggest that birds represent a good proxy for lower order taxa and surmised that their data indicated that insects and seed availability was also on the decline, however the links were not quantified. In this study we investigate the functional link between arable weeds and invertebrates, and the potential for these to provide ecosystem services such as pest control and to support farmland bird populations. We focus on wheat, a globally important crop, which is third in terms of global production, after rice and maize, but is the crop that is grown across the widest geographic range (Shewry and Hey, 2015). We suggest that small changes to the agronomic management of wheat could contribute substantially to farmland biodiversity gains.
In order to sustainably meet rising demands for agricultural products it is necessary to encourage regulating or supporting ecosystem services via agronomic practice (Bommarco et al., 2013). Understanding the extent to which these services are already supported in conventional systems provides a useful baseline for further research; it is essential to understand the community composition of ecosystem service-providing organisms, and their relationships, at a range of scales, if we are to deliver effective ecological intensification (Bommarco et al., 2013). While a wealth of work exploring how to increase biodiversity and ecosystem service providers in agricultural systems has largely paid attention to field margins and non-crop habitat (Griffiths et al., 2008; Vickery et al., 2009; Tschumi et al., 2016), some of the most serious declines in biodiversity have taken place within the cropped area of fields (Aebischer, 1991; Robinson and Sutherland, 2002; Holland et al., 2008; Storkey et al., 2013; Ewald et al., 2015). Authors have recently emphasized the untapped potential of cropped areas to support biodiversity (Potts et al., 2010; Seifert et al., 2015; Storkey and Neve, 2018); this approach has also long been championed by ornithologists who are concerned for birds that avoid field edges and forage almost exclusively in the open crop, such as skylarks Alauda arvensis (Morris et al., 2004). If policy instruments are to be developed to address cropped areas, it is essential to show the value of in-crop measures as they have so far proved unpopular to farmers. This is illustrated by the choices farmers make when selecting agri-environment scheme options: under the English Entry Level Stewardship (now replaced by Countryside Stewardship), take-up of in-field options to restore traditional cereal ecosystem floras accounted for only 0.35% of the points agreed with farmers (Hodge and Reader, 2010).
Arable weeds have the potential to support the delivery of regulating ecosystem services within cropped areas (pollination and pest control) via invertebrates and birds (Storkey and Westbury, 2007; Petit et al., 2011). Furthermore arable weeds support provisioning ecosystem services (production of game) and cultural services (the well-being associated with seeing and appreciating wildlife) by supporting invertebrates, which are essential in the diet of the majority of farmland bird chicks and for some adult birds (Holland et al., 2006), However, the general trend has been for arable weed abundance and diversity to decline over the last 60 years, attributed to increased intensification of farming (Sutcliffe and Kay, 2000; Fried et al., 2009; Potts et al., 2010; Storkey et al., 2011). Long-term studies have demonstrated species losses and gains; although some common species remained stable or increased, many decreased and rare species suffered the greatest losses (Potts et al., 2010; Storkey et al., 2011). In particular the introduction of herbicides and an increase in herbicide efficacy have particularly impacted on annual plants (Southwood and Cross, 1969; Ewald and Aebischer, 2000; Walker et al., 2006). Likewise, changes to rotations and cropping patterns have impacted perennials: for example, the switch to autumn sown cereal crops has encouraged autumn-germinating arable weeds over spring germinators, particularly pernicious species such as Alopecurus myosuroides (Black Grass), Anisantha sterilis (Sterile Brome) and Galium aparine (Cleavers) (Hald, 1999), which have flourished in nitrogen-rich conditions. The subsequent dominance of a few competitive species has contributed to a reduction in the diversity of arable weeds (Wilson and King, 2003). Modern farming practices may benefit a small number of species, and there are reports of new weed taxa colonizing but in general, the literature suggests that arable weed diversity is declining (Potts et al., 2010).
Parallel declines in both invertebrates and farmland birds have been linked with reduced numbers of arable plants (Newton, 2004, Aebischer, 1991; Robinson and Sutherland, 2002; Benton et al., 2003) and levels of chick-food on cropped land are considered inadequate to support farmland birds (Holland et al., 2012). Innovative studies to help clarify the complex web of interactions occurring between weeds and invertebrates in agro-ecosystems have been called for (Barberi et al., 2010).
In this paper we synthesized data from seven UK studies over a period of 18 years, providing information on both arable weeds and invertebrates in winter wheat. The aim was to identify the most commonly occurring weeds; to test the strength of the relationship between arable weeds and invertebrate ecosystem service providers; to establish whether the cropped area of wheat fields have the potential to provide ecosystem services and to establish whether there is any threshold of weed cover that can be used as a minimum target by policy makers to improve ecosystem service provision in cereal crops.
Methods
Data Sets
Data were collated from seven field-scale experiments which involved the collection of both arable weeds (% cover) and invertebrate (abundance) data from the cropped area of winter wheat fields. A total of 7146 pairs of data (weed and invertebrates) were collected.
None of the studies explicitly aimed to investigate weed-invertebrate relationships, but were either designed to examine the effect of experimental “treatments” on plants and invertebrates independently (six studies) or were a long-term monitoring exercise (one study) (summary in Table 2).
Study 1: Aim: To enhance farmland biodiversity by integrating novel habitat management approaches within the crop. Experimental design: 10 farms in England, 3 fields per farm (one treatment per field), mid-field sampling position, sampled three times (May, June and July) in each of 2 years (2002, 2003) (Smith et al., 2009). Treatments: skylark plots and wide-spaced rows, control (conventional winter wheat crop).
Study 2: Aim: To increase the abundance and availability of plant species, such as spring-germinating weeds, and associated invertebrates by optimizing herbicide inputs. Experimental design: Replicated small plot trials on three farms in England, manipulation of herbicide inputs over 3 years (2003–2005), sampled on one occasion in late June (Jones and Smith, 2007). The design differed between farms as shown in Table 1.
Study 3. Aim: To enhance farmland biodiversity by integrating novel habitat management approaches, in the crop (undrilled patches) and non-cropped margins (sown field margins. Experimental design: 26 farms in England and Scotland, four fields per farm, edge to mid-field sampling twice (June and July) in each of 3years (2003–2006); Treatments: Conventional field centers with no field margins; Field centers containing two undrilled patches per ha with field margins; Conventional field centers with field margins; Field centers containing two2 undrilled patches per ha with no field margins (Clarke et al., 2007).
Study 4: Aim: to research the long-term effects of farming on arable weeds and invertebrate chick food. Experimental design: one farm in Leicestershire, 34 fields sampled at the edge and in the middle of the crop on one occasion (June) in each year between the 1992 and 2010. Crops sampled include winter wheat, spring beans, winter beans, spring barley, winter barley, oilseed rape and set-aside, permanent pasture, hemp and linseed, only winter wheat fields are included in this dataset (Stoate, 2017).
Study 5: Aim: To examine the (then) current risk assessment based regulatory procedures in relation to indirect effects of pesticides. Experimental design: three farms in Hampshire, Lincolnshire and Yorkshire, approximately 40 fields per farm, edge and mid-field sampling positions, sampling three times (May, June and July) in each of 2 years but staggered over 4 years (1999–2002). There were four 100 ha blocks on each of three sites which had staggered entry in April 1999, 2000, 2001 respectively. Work ran for 2 years at each site. Year 1 was a base-line year whilst in the second year two blocks received elevated insecticide inputs and two acted as controls (Holland et al., 2012).
Study 6: Aim: To determine whether organic farming leads to elevated levels of invertebrate chick food. Experimental design: eight farms in southern England, organic, and conventional headlands, sampled over a three-year period (1990–1992) (Moreby and Sotherton, 1997).
Study 7: Aim: to evaluate the value of brood cover in increasing invertebrate food for gray partridge chicks. Experimental design: Study 1: 10 farms in England, 6 “environments” (conventional winter wheat, conservation headlands plus 4 seed mixes), two fields per “environment,” two samples per field, edge of crop sampling position. Study 2: three farms, 6 “environments” (conventional winter wheat and conservation headlands plus four seed mixes), three fields per “environment,” two samples per field, edge of crop sampling. Sampled twice (June and July 2010) unpublished data.
In all studies the weed and invertebrate data were collected at the same location (i.e., they were coincident) and, within each study, by the same team of people. Invertebrates were collected using a D-vac suction sampler (Dietrick, 1961) and all invertebrate identification was undertaken by the same laboratory. An effort was made to identify all plants to genus and in the majority of cases to species; unidentified individuals were split into dicotyledons and monocotyledons. For most analyses, species-level data was used. In some cases, where plants were unallocated to species, the genus aggregate was used. For example, the meadow grasses Poa spp. can be difficult to identify in the field and in many cases a proportion of the individuals were identified only to genus. Invertebrates were identified to family in all studies. Study sites were geographically wide-spread across the UK and so include a range of soil types.
Each dataset was broken down, as far as possible, into independent subsets i.e., either fields, treatments or blocks within year or site (Table 2). Where multiple months were sampled, to ensure consistency across studies, only data collected in June were included. Where multiple sub-samples were taken, they were averaged to ensure no pseudo replication.
For each dataset, each subset was taken in turn and invertebrates related to weeds using regression. The response variables were the four calculated invertebrate quantities in section Calculated Variables; the explanatory variables were, separately, overall weed cover, grass cover and broadleaved cover. Data were log transformed. Regression coefficients and standard errors were calculated (Genstat 12th edition, Lawes Agricultural Trust, Rothamsted).
Statistical Analysis
Calculated Variables
In addition to the species-, genus-, and family-specific taxa described above, we calculated the following aggregate groups.
Plants: As grasses (monocotyledon) and broadleaf (dicotyledon) plants are frequently differentiated by herbicide programmes, we aggregated arable weed cover (%) into these two groups.
Invertebrates: Several functional groups are defined below (except where stated otherwise, the taxa mentioned refer to adult individuals).
We categorized invertebrates as phytophagous and predatory at the family level as diet will affect invertebrate response to vegetation cover. The predatory group corresponded to invertebrates beneficial for pest control in crops; we included families in which species were mostly predatory (Boys, 2014). Invertebrates using floral resources (Syrphidae, for example) are also important for pest control, but numbers were too low in our datasets to be included. Indices are based upon sampling an area of 0.46 m2 using a Dvac suction sample (Dietrick, 1961) using the standard 0.092 m2 nozzle, with each sample comprised of five, 10 second sub-samples.
Phytophages = (counts of) Aphididae + Orthoptera + Gastropoda + Homoptera + Symphyta larvae + Lepidoptera larvae + Chrysomelidae + Curculionidae + Elateridae + Nitidulidae + Bibionidae + Tipulidae larvae + Oedemera.
Predators = (counts of) Araneae + Opiliones + Carabidae + Coccinellidae adults and larvae + Staphylinidae + Cantharidae + Nabidae + Odonata.
To consider the extent to which wheat fields provide invertebrate chick-food resources for birds, we calculated a Chick Food Index (CFI), calculated as a weighted index of five invertebrate groups that are linked to the survival of gray partridge chicks using Dvac samples (Potts and Aebischer, 1991).
CFI = 0.1411 (Carabidae + Elateridae) +0.1199 (Neuroptera + Lepidoptera + Symphyta, adults & larvae) + 0.0832 (Chrysomelidae + Curculionidae, adults & larvae)+0.00614 (Homoptera + Heteroptera – Aphididae, all stages) + 0.000368 (Aphididae, all stages).
We also calculated a Generalized Bird CFI (GBCFI) described fully in DEFRA (2010). The GWCT database on farmland bird diets (Holland et al., 2006) was interpreted to identify bird species that feed predominantly within the crops and for which there was information on the composition of invertebrates (identified to family level) in their diet. Invertebrate taxa were selected as important in the diet of three bird species (gray partridge, skylark and yellowhammer) if they represented 10% or more of the avian diet (using either numbers of invertebrates or biomass). The biomass of each selected taxa was determined using two approaches. The first was based upon the invertebrate length using the equation derived by Rogers et al., 1975); the second was actual biomass from the study conducted in Western Poland (Ryszkowski and Kark, 1977). Where there were no data for a taxon in these published resources, other literature sources were used: Syrphidae larvae (Grim, 2006), Carabidae (Cardenas and Hidalgo, 2007), Lycosidae (Greenberg and McGrane, 1996), Bibionidae and Lepidopteran adults (Pieris spp.) (Nentwig, 1982). Two types of indices were devised using 39 years of invertebrate data from the GWCT Sussex study, based on estimating the total biomass across either taxa or functional groups (taxa grouped according to size, morphology and availability to foraging birds) in each year. These indices were constructed using either abundance, calculated biomass or both. The best one was selected by testing for correlations with the indices for gray partridge, skylark and yellowhammer, calculated from the Sussex Study dataset.
GBCFI = 0.269 (Aphididae) + 13.692 (Carabidae) + 2.122 (Elateridae) + 3.019 (Chrysomelidae) + 2.208 (Curculionidae) + 1.678 (Staphylinidae adults) + 0.251 (Staphylinidae larvae) + 0.409 (Nitiulidae) + 4.532 (other Coleoptera) + 4.228 (Symphyta larvae) + 9.877 (Lepidoptera larvae) + 2.878 (Neuroptera larvae) + 1.479 (Formicidae) + 35.674 (Tipulidae) + 7.153 (Bibionidae) + 1.711 (other Diptera).
Synthesizing Data
In a typical meta-analysis, the derived statistics from multiple studies are combined and analyzed together. Study results are assigned weights according to the sample size, allowing the relative value of different studies to be compared objectively. This is especially useful because studies with small sample sizes can be included; a meta-analysis combines effect sizes across studies, resulting in greater statistical power (Borenstein et al., 2009). The aim of this study was to synthesize the seven available data sets to determine a representative estimate of the strength and direction of linear relationships between weeds and invertebrates in wheat fields.
Each dataset was taken in turn and analyzed separately using a meta-analysis approach, with the regression estimate from each subset as effect size. A summary effect size and standard error was obtained using a random effects model (Borenstein et al., 2009), Comprehensive Meta Analysis Version 2.2, 2010). This was repeated for each of the seven studies as follows:
The equations used to calculate the summary effect size and standard errors were those described by Borenstein et al. (2009). They were the same in the within-studies analyses and the between-studies analyses. The weight assigned to each group was calculated as the reciprocal of the within group variance and the between studies variance, or
• Where VYi was the within-group variance for group i and T2 was the between-groups variance.
• Next, the weighted mean was computed as
• The variance of the summary effect was estimated as the reciprocal of the sum of the weights
• And the estimated standard error of the summary effect was the square root of the variance,
• Finally, a Z-value to test the null hypothesis that the summary effect size was zero was calculated using
• And the p-value calculated using
• These seven summary effect sizes and their computed standard errors were then used to calculate an effect size across all studies.
In some cases, there were very few weeds or invertebrates recorded within individual subsets. It was not possible to generate a regression coefficient for this subset and the subset was excluded. This reduced the sample size, with the result that the sample size could be unequal between analyses – i.e., there may have been 20 subsets available to investigate grass cover but only 18 subsets with sufficient cover to consider broadleaves.
The equations used to calculate the summary effect size, their standard errors and the following statistics are described by Borenstein et al. (2009) and were calculated using Comprehensive Meta-analysis (2010). Q (standardized weighted sum of squares) was used to test the null hypothesis that all the studies (or subsets within a study) shared a common effect. A significant Q (P < 0.05) indicated that the relationship between weeds and invertebrates differed between studies (or subsets). The true effect size may vary from study to study and it is important to understand how much variation exists and whether it is due to “real” variation in effect size between studies or to sampling error. If Q is not statistically significant, no difference is detected, although such an outcome may arise through low statistical power as well as lack of difference. The ratio of excess dispersion to total dispersion was calculated as I2, which gives a measure of the percentage of the observed dispersion that is real. Effectively I2 is a “signal-to-noise ratio” (Borenstein et al., 2009). If I2 is high then a large proportion of the differences in effect size is due to real difference, if it is low, then most of the differences are associated with noise. As a rule of thumb I2 = 25% is considered low, 50% moderate and 75% high.
Meta-Regression
Meta-regression was employed to test whether heterogeneity in the relationship between invertebrate groups and weed cover variables was due to the diversity of the weeds present. This was restricted to three studies where there were sufficient data (studies, 1, 2, and 4). The principles of meta-regression between studies (or in this case, between subsets within studies) are essentially the same as those of regression between individual data points. The effect size in each functional group was used as the dependent variable, and the mean value of H (number of weed taxa) for each group was used as the covariate. A method of moments model was used, which does not assume that the true effect size is identical in all functional groups (Borenstein et al., 2009).
Relating Invertebrate Taxa to Weed Taxa
The RELATE routine in PRIMER-E (version 6) was used to determine if there was a relationship between invertebrate and weed resemblance matrices. RELATE aims to determine how related two groups of multivariate data are by calculating a rank correlation coefficient between their matrices. A simple permutation test was applied to the coefficient to test the null hypothesis that there was no relationship between the matrices. Where a significant relationship was identified, the BEST routine (Clarke and Gorley, 2006) was employed to select the weed taxa that best explained the community pattern of the invertebrate data. Only weed taxa which contributed more than 1% cover were included. Data were fourth-root-transformed prior to analysis. This was sufficient to normalize the data and to optimize relationships between plants and invertebrates. The aim of the BEST routine is to find the best match between multivariate among-sample patterns of two assemblages. The extent to which these two patterns match reflects the degree to which the chosen “predictor” data “explains” the biotic pattern. The routine then searches over subsets of the abiotic variables for a combination which optimizes that match i.e., subsets of biota which best match a different biotic matrix (Clarke and Gorley, 2015)
Establishing a Weed “Threshold”
In order to determine a threshold, or level, at which there is “sufficient” cover of weeds, it is necessary to decide on the target for invertebrate abundance, i.e., to decide what invertebrate abundance is sufficient. As raw abundance data is difficult to interpret, and no targets exist, we therefore used the Chick Food Index (CFI) described above. It has been determined that a CFI of 0.7 is sufficient to stabilize gray partridge numbers and this became our target threshold for invertebrates (Potts and Aebischer, 1991). Using the subsets containing CFI values that approached (data were included at 0.6) or exceeded 0.7, we took the significant regressions and calculated the values of weed cover needed to obtain a CFI of 0.7.
Table 9 shows that subsets varied in the amount of weed cover that would be necessary to achieve a CFI of 0.7. Using this data we aggregated the subsets according to the cover necessary into the following categories: Very low = weed cover < 0.1,; Low = weed cover 1–10%, Low medium = weed cover 10–20%, Medium = weed cover 20–60%, High = weed cover >60%. The composition of the weed assemblage in those subsets was compared between those groups using PRIMER V6, and the proportion of weeds in the very desirable, desirable, neutral and undesirable weed categories (Jones and Smith, 2007) for the invertebrates that make up CFI were calculated, to assess whether the sites that required “low” cover contained more desirable weeds than those that required “high” cover i.e., whether lower weed cover is necessary if the weeds present are “desirable.”
Throughout the results and discussion, we shall be using this notion of desirability as shown in Table S1, developed at FERA (Jones and Smith, 2007).
Results
Broad Dataset Characteristics
The average weed cover over all sites and years varied considerably between studies from as low as 2.7% in Study 3 up to 29% in Study 6 (Table 3). The ratio of grasses to broadleaves also differed considerably between studies (Table 3).
Poa annua annual meadow grass and Polygonum aviculare knotgrass were the most commonly occurring taxa (Table 4), followed by Stellaria media chickweed, Alopecurus myosuroides black grass and Viola arvensis field pansy.
For none of the datasets did the overall average CFI reach 0.7 but three of the datasets had subsets where CFI met or exceeded 0.7 (Table 5).
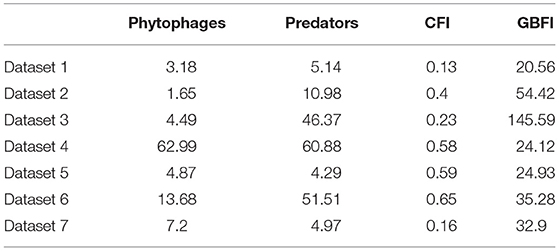
Table 5. Mean abundance of phytophagous and predatory invertebrates, the gray partridge chick-food index (CFI) and general farmland bird index (GBFI) in the seven studies.
Across all the studies, the most commonly occurring invertebrate groups were Arachnida, Coleoptera and Diptera, followed by Heteroptera and Parasitica (Table 6).
Relationship Between Invertebrates and Weeds
There were positive relationships between the invertebrate variables and the weed variables, resulting from the meta-analysis (Table 7). Both CFI and GBCFI were positively related to weed cover. Although predator abundance was related to total weed cover, when broadleaf cover and grass cover were analyzed separately, no significant relationship was detected. Phytophages were more strongly related to weed cover than predatory invertebrates; they were related to both grass cover and broadleaf cover separately.
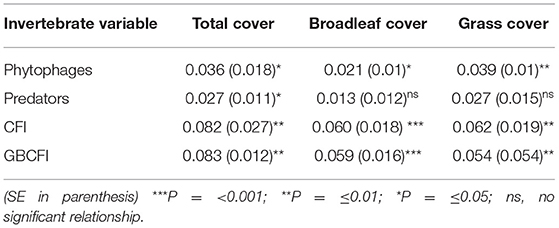
Table 7. Summary effect sizes (regression coefficients) derived from a random effects meta-analysis.
Between-Study Heterogeneity in Invertebrate-Weed Relationships
The effect size (strength of the relationship between phytophages and total weed cover) varied between studies (Q = 12.86*, see Table 8). The I2 value (ratio of signal-to-noise) was 50.1, indicating that 50% of variation was due to biological differences between the studies rather than spurious differences associated with random error. Q was significant only in two other cases, the relationships between predatory invertebrate abundance and total weed cover, and between predator abundance and grass cover (Table 8). The most heterogeneous relationship identified was between predator abundance and grass cover. Although Q was insignificant in all other cases, suggesting that the effect detected was common across studies, the I2 value was above 20% in 6 out of 9 cases, indicating that heterogeneity may still be present. It is possible that low power or high variance led to Q being insignificant.
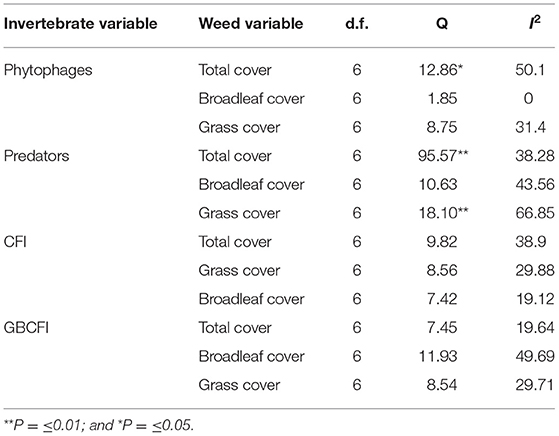
Table 8. Values for d.f., Q and if significant, and I2 between all seven studies included in analyses.
Weed Cover Target Level
The analysis was restricted to subsets where a significant relationship between CFI and weed cover was identified (Table 9). The amount of weed cover required to produce a CFI of 0.7 averaged 45% for overall cover, 23% for grass, and 1% for broadleaves but varied considerably between subsets.
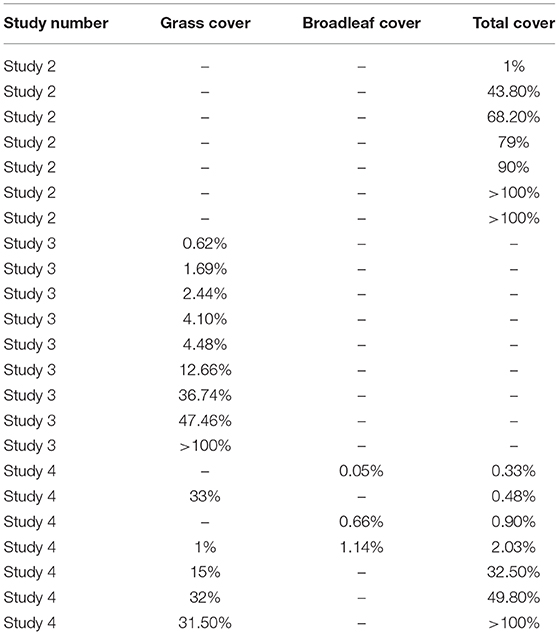
Table 9. Weed cover required to provide a CFI of 0.7 (the minimum needed to maintain gray partridge numbers), in winter wheat.
The proportion of very desirable, desirable, neutral and undesirable weed taxa varied between the different levels of weed cover. The percentage cover of the more desirable taxa, known to be associated with high insect abundance, was higher for where weed levels were lower (Figure 1). This indicates that if more desirable species are present then fewer weeds are needed to achieve the target chick-food index of 0.7.
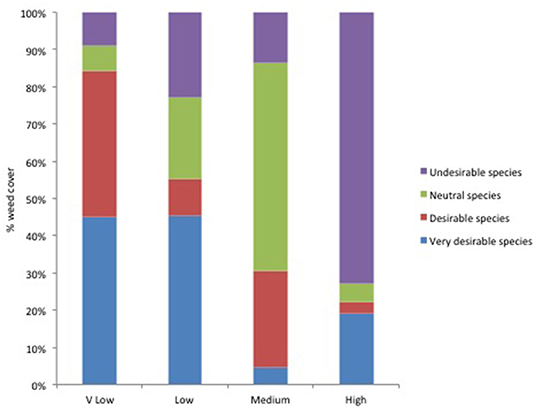
Figure 1. The weed cover necessary to reach the critical value of CFI 0.7 in three of the seven categories was categorized as: Very (V) low = <1%; Low = 1–10%; Medium = 20–60%; High = >60%. The proportion of weeds of four levels of desirability [Very desirable, Desirable, Neutral and Undesirable, according to Jones and Smith (2007) see Table S1], was calculated. These categories are based on known agronomic and biodiversity traits and were deigned as an accessible guide for farmers (Jones and Smith, 2007). The percentage cover of the more desirable taxa, known to be associated with high insect abundance, was higher for where weed levels were lower.
Best Predictors of Invertebrate Community Composition
The BEST routine identified the top five weed taxa in each study, in terms of their correlation with the invertebrate assemblage (Table 4). Of those taxa considered highly desirable, P. annua was found to be in the top five in six of the seven studies and P. aviculare and S. media were both found in the top five in two of the seven studies. Conversely of those taxa considered undesirable (because they are pernicious) Bromus sterilis, Gallium aparine and Cirsium arvense were found in the top five in four, three and two studies respectively.
Discussion
Summary
The power of this study lies in its extent; being based on seven studies spanning 18 years that give a comprehensive picture of weeds and invertebrates in UK wheat-based agro-ecosystems.
Overall, the weed flora was poor and the diversity was relatively low. Such an impoverished flora is most likely a consequence of extensive herbicide use since the 1950s and intensive soil cultivations. Analyses of weed seed banks have also found low seed densities (Robinson and Sutherland, 2002; Holland et al., 2008). The most frequently occurring flora in our studies were P. annua (Annual meadow grass), P. aviculare (Knotgrass), S. media (Chickweed), Alopecurus myosuroides (Black Grass), V. arvensis (Field Pansy), Aethusa cynapium (Fool's parsley), Chenopodium album (Fat Hen), G. aparine (Cleavers) and Veronica persica (Common Speedwell), all previously recognized as being common (Marshall et al., 2003). Of these, Cleavers and Black Grass are considered undesirable agronomically as they are pernicious weeds. Black Grass is a relatively poor resource for invertebrates, only being associated with eight Diptera species (Database of Insects and their Food Plants (DBIF; http://www.brc.ac.uk/dbif/hosts.aspx last accessed 15/05/2019) but though Cleavers is designated as undesirable it is associated with 50 species of invertebrate including moths and true bugs (DBIF). Some of the other species that frequently occurred in the seven studies have relatively high numbers of invertebrate species associated with them: S. media (84; moths, bugs, flies and beetles), P. aviculare (113; moths, beetles, flies, and bugs), P. annua (76; butterflies, moths, flies and aphids). In terms of host-specific species, the highest numbers recorded in the DBIF were for annual species e.g., P. annua (seven dependent invertebrate species, three of which were red listed), and P. aviculare (four dependent species, two red-listed).
Can Cropped Areas of Wheat Fields Support Ecological Services?
There is a strong case to be made that the cropped area of wheat fields can support ecological services if weed cover and diversity is sufficient. Weed seeds are an important food resource for some species of arthropods, birds and small mammals (Tooley and Brust, 2002; Westerman et al., 2003; Holmes and Froud-Williams, 2005; Holland et al., 2006). The most important seeds eaten by birds are produced by Stellaria (Chickweeds), especially S. media; Polygonum (Knotgrasses), especially P. aviculare; Chenopodium, especially C. album; and Poa (Meadow grasses), especially P. annua (Marshall et al., 2003; Holland et al., 2006). These are the four most commonly occurring arable weeds recorded in our studies, demonstrating the potential to provide resources for farmland birds. Seeds are also consumed by invertebrates, most commonly some carabid species (Tooley and Brust, 2002; Petit et al., 2014) and ants (Pearson et al., 2014). These two taxa are important dietary items for farmland bird chicks and adults (Holland et al., 2006).
For the predatory invertebrates there was a positive relationship with the total weed cover rather than for broadleaved or grasses specifically. There is some evidence that the shelter provided by weeds used by predators protects them from adverse weather conditions such as heavy rain or excessive heat, intraguild predation and pesticide sprays (Gontijo, 2018). The extent to which weeds provide food resources for natural enemies is less well understood. Besides harboring alternative prey, weed seeds, the vegetation and floral resources may also be consumed, with some evidence of seed predation by Carabidae, although this is has mostly been investigated in spermophagous species (Tooley and Brust, 2002) and their value for other natural enemies is unknown. Likewise, it is not known whether weeds supporting and enhancing the fecundity of parasitoids translates to improved biological control but field margins are considered to provide such a benefit (Heimpel and Jervis, 2005). Weeds can also harbor crop pests by providing resources for them or by acting as host plants (Norris and Kogan, 2005); how important this is also unquantified.
Does Weed Cover Predict Invertebrate Ecological Service Provision in the Cropped Area of Fields?
Positive relationships were identified between invertebrates and weeds. We measured phytophagous taxa, predatory taxa (as a surrogate for pest regulation) and bird food (using two indices developed at GWCT): the Gray Partridge Chick Food Index (CFI) and the Generalized Bird Chick Food Index (GBFI). All invertebrate groups were positively related to total weed cover, albeit weakly; both CFI and GBFI were more strongly related to weed cover than phytophagous and predatory taxa. Early work investigating the value of Conservation Headlands showed that areas unsprayed with herbicides supported both high weed abundance and a higher number of chick-food invertebrates, including Carabidae (Sotherton, 1991). The Carabidae themselves were better fed on unsprayed areas, having higher prey consumption and a more diverse diet than those on sprayed areas, consequently their productivity was higher (Chiverton and Sotherton, 1991). When the influence of various vegetation characteristics on a similar range of chick-food groups was examined for perennial and annual agri-environment scheme habitats, the level of broadleaf cover had a positive effect on chick-food invertebrates (Holland et al., 2014). It was expected that phytophagous taxa would be related to weed cover as in previous studies on selectively sprayed headlands in which restricted herbicide use led to increases in arable plants and associated invertebrates (Sotherton, 1991).
Although phytophagous species are dependent on plants for food, the relationship between these plant-feeding species and weed cover (both broadleaf and grass cover) was not as pronounced as that between weeds and CFI and GBCFI that comprise a mixture of phytophagous taxa and some predators. Predatory taxa showed the weakest response to weed cover, although where relationships were identified they were more likely to be with grasses. These results echo Hawes et al. (2009) and (Smith et al., 2009), who found a weak positive relationship between predators and grasses, although other authors found no relationship between levels of predators and grass cover in annual and perennial agri-environment scheme habitats (Holland et al., 2014). Regulation of phytophagous species by predators may buffer the effect of weed abundance; previous studies have shown that herbivores experience greater top-down than bottom-up population regulation (Siemann et al., 1998; Hawes et al., 2009). This effect may be obscured in the bird food indices as predatory species (e.g., carabids) are weighted heavily either because of their biomass or prevalence in the diet.
The relationship between CFI and weed abundance supports the now generally accepted thesis that weed cover and gray partridge survival are linked (Potts, 1991; Potts and Aebischer, 1991). When the vegetation was broken down into broadleaf and grass cover, there was little difference in the response of CFI between them in this study, indicating that both broadleaf and grass cover, at least to some extent, drove CFI in the field. A CFI of 0.7 or above was infrequently recorded, indicating that wheat fields currently support insufficient resources for birds and that is likely to be due to low levels of arable weed cover. Increased weed cover could potentially provide improved resources.
GBCFI is based on known invertebrate bird food taxa, weighted by an approximate body mass of the invertebrates therein. This also proves to be an index which is linked to overall weed cover, and separately to grass cover and broadleaf cover. This index is less bird-species-specific than the CFI, in that it incorporates invertebrates eaten by a wide range of bird species (DEFRA, 2010). As a general rule it does not differ from the CFI in a biologically meaningful way (although there are some local differences within studies) and its use as an index could be developed further. Conversely, the similarity shows the wide-applicability of the CFI, which can be used as an indicator for more than just gray partridge; it may be considered a useful guide to ecosystem service provision in arable habitats.
How Important Is Weed Species Diversity in These Relationships?
We investigated the moderating effect of weed species diversity on relationships between weeds and invertebrates but did not find a consistent pattern. This means that adding weed species did not strengthen or weaken the relationships, which might be what one would expect. It is possible that a lack of weed diversity within fields (which was typically <6 species) coupled with the level of taxonomic resolution of the invertebrate data meant that the effect of increasing weed diversity on invertebrate abundance was not detected.
How Heterogeneous Are the Weed Invertebrate Relationships?
It was clear that there was significant heterogeneity within studies and that the extent of variation and uncertainty varied between studies. This does not negate the overall positive result but it does counsel caution when extrapolating to individual sites. The within-study heterogeneity was unsurprising, we know that factors such as previous cropping and its management (Marshall and Arnold, 1994), field size and landscape context strongly effect weed composition/cover (Gaba et al., 2010) and thereby invertebrate composition/abundance (Marshall et al., 2003) and ecosystem services, such as pest management and pollination (Veres et al., 2013; Garibaldi et al., 2016). It is unsurprising that we find the strength of the invertebrate/weed relationship heterogeneous. Weed richness and weed diversity are considered to increase as field size decreases because of the associated increase in habitat heterogeneity within cultivated areas and the presence of more crop edges acting as refuges for weed species (Wilson and Aebischer, 1995; Gaba et al., 2010). Consequently, weed richness and diversity are higher in fine-grained landscapes (Baessler and Klotz, 2006). Heterogeneity between studies is typical in meta-analyses and despite the inherent variation between the seven studies considered here a positive signal emerges.
Can we Identify a Threshold of Weed Cover to Aim for in Cropped Fields?
CFI is the only quantitative index of bird diet for which a biologically meaningful threshold has been derived and therefore has the most utility. If we wish to provide a threshold of weed cover to guide land managers, we need first to establish an invertebrate target against which it can be measured. In this case, it has been established that CFI 0.7 is sufficient to support wild partridge broods and we used this as the minimum value to aim for. It is evident that a reasonable CFI can be obtained from areas with very low weed cover, and our results suggest that the most important factor is the quality, or desirability, of weeds in the field rather than absolute cover. The desirable species previously identified, and known to support insects, can be present in low abundance; 10% cover is sufficient (in some circumstances less) to attract insects. However, large cover of species categorized as undesirable, such as Black Grass, are unlikely to attract many invertebrates. There are two key caveats; (1) undesirable does not always mean “useless” for wildlife—if the undesirable component is made up largely of cleavers it will be attractive to invertebrates but unacceptable to farmers. (2) The species that have been categorized as neutral may in fact be desirable; the desirability of species we currently designate as “neutral” is a knowledge gap to fill.
Although field margins and beetle banks may be a source of beneficial and chick-food invertebrates (Thomas et al., 2001; Griffiths et al., 2008) the impact of these edge habitats does not extend very far into the crop, typically no more than 60 m (Holland et al., 1999, 2004, 2009). Other means of ensuring ecosystem service delivery across even the largest fields are needed, unless fields wider than 120 m are divided by beetle banks. Weeds may help in this process by providing an appropriate microclimate, structure or sources of food, both prey and plant material, that may increase survival and reproductive capacity of beneficial invertebrates. However, farmers are adverse to any practices that encourage weeds within crops. The poor uptake of Conservation headlands (unharvested headlands) or other practices that interfere with production in agri-environment schemes exemplify this (Boatman et al., 2007; Keenleyside et al., 2011). In the future a number of pressures may force farmers to consider exploiting biological control including governmental policy on herbicide use. The sustainable use of pesticides was adopted in 2009 and farmers were directed to demonstrate Integrated Pest Management by 2014. This may be encouraged as pest resistance to insecticides is increasing (Foster et al., 2014) whilst others are being withdrawn or restricted in their use (Anon, 2014).
Our understanding of the desirability of weeds can be refined by examining economic weed thresholds. These have been modeled (in winter wheat) for six of the most frequently recorded species in our studies: P. annua (Annual meadow grass), S. media (Chickweed), Alopecurus myosuroides (Blackgrass), V. arvensis (Field Pansy), G. aparine (Cleavers) and V. persica (Common Speedwell) (Storkey and Cussans, 2007). Of these only Blackgrass and Cleavers were predicted to result in a yield loss of 1%/plant/m2 or above. P. annua, Chickweed, Field pansy and Common speedwell were all recorded as causing a yield loss of <0.1%. However, weeds do not occur as single species stands and modeling the weed threshold of multiple species is problematic because weeds interact both with other weed species and the crop. There is a large literature base on how weed thresholds are calculated and implemented where the challenge of predicting crop losses through weed competition is addressed (see Swanton et al., 1999). Recent technology in remote sensing means that weed control can be increasingly targeted to balance biodiversity with crop protection (Huang et al., 2018) and could go some way to addressing the concerns of farmers.
The holygrail for policy makers and advisors is to be able to develop guidelines for the amount of uncropped land that should be allocated on farm for “biodiversity.” However, this is illusive. While researchers have suggested a minimum of 6% (Ewald and Aebischer, 2000) and 8% (Pywell et al., 2015), for birds even above 10% may be needed (Henderson et al., 2012). The current threshold for Ecological Focus Areas (EFA) in England is 5% and the quality of EFAs will determine how successful the outcome is for wild plants and animals and ES delivery. However, we suggest that value of EFAs and similar agri-environment measures could be greatly enhanced by managing desirable arable weeds in cropped area of fields at a threshold of 10%. The additional benefit is, as discussed, that weeds among the crop can support pest natural enemies where they are needed (close to their prey) and support farmland specialist birds who forage in crop fields. Although farmers may be resistant to weedy fields, there is a clear potential, if we could control species composition, to contribute to the restoration of farmland biodiversity.
Data Availability Statement
The datasets generated for this study will not be made publicly available some of the data was publicly funded and is available, however, some is not because the complete dataset includes unpublished GWCT information which GWCT is unwilling to release at present, however, we will provide summary information for each study.
Author Contributions
BS conceived the study, carried out analysis, and wrote the paper. NA advised on statistics and edited the paper. JE advised and supported analysis and edited the paper. SM advised on ecology and edited the paper. CP carried out analysis and edited the paper. JH co-wrote the paper.
Funding
This work was funded by the Esmee Fairbairn Foundation.
Conflict of Interest
The authors declare that the research was conducted in the absence of any commercial or financial relationships that could be construed as a potential conflict of interest.
Acknowledgments
The authors would like to thank the funders of the studies included in this project including Defra Sustainable Arable Link, the many field assistants who assisted with data collection and the landowners who gave access. We also thank Alfred Gathorne-Hardy for commenting on the text and the valuable comments of two anonymous referees.
Supplementary Material
The Supplementary Material for this article can be found online at: https://www.frontiersin.org/articles/10.3389/fsufs.2019.00118/full#supplementary-material
References
Aebischer, N. J. (1991). “Twenty years of monitoring invertebrates and weeds in cereal fields in sussex,” in The Ecology of Temperate Cereal Fields, eds L. G. Firbank, N. Carter, J. F. Darbyshire, and G. R. Potts (Oxford: Blackwell Scientific Publications), 305–331.
Anon (2014). The Future Availability and Efficacy of Plant Protection Products – Potential On-Farm Implications. Report for AHDB Cereals and Oilseeds. Available online at: https://theandersonscentre.co.uk/wp-content/uploads/2017/07/PPP-Report.pdf
Baessler, C., and Klotz, S. (2006). Effects of changes in agricultural land-use on landscape structure and arable weed vegetation over the last 50 years. Agric. Ecosyst. Environ. 115, 43–50. doi: 10.1016/j.agee.2005.12.007
Barberi, P., Burgio, G., Dinelli, G., Moonen, A. C., Otto, S., Vazzana, C., et al. (2010). Functional biodiversity in the agricultural landscape: relationships between weeds and arthropod fauna. Weed Res. 50, 388–401. doi: 10.1111/j.1365-3180.2010.00798.x
Benton, T. G., Vickery, J. A., and Wilson, J. D. (2003). Farmland biodiversity: is habitat heterogeneity the key? Trends Ecol. Evol. 18, 182–188. doi: 10.1016/S0169-5347(03)00011-9s
Boatman, N., Jones, N., Garthwaite, D., Bishop, J., Pietravalle, S., Harrington, P., et al. (2007). Evaluation of the Operation of Environmental Stewardship. Final Report to Defra, Project No. MA01028, Central Science Laboratory.
Bommarco, R., Kleijn, D., and Potts, S. G. (2013). Ecological intensification: harnessing ecosystem services for food security. Trends Ecol. Evol. 28, 230–238. doi: 10.1016/j.tree.2012.10.012
Borenstein, M., Hedges, L. V., Higgins, J. P. T., and Rothstein, H. R. (2009). Introduction to Meta-Analysis. Chichester: Wiley & Sons.
Bowler, D. E., Heldbjerg, H., Fox, A. D., de Jong, M., and Böhning-Gaese, K. (2019). Long-term declines of European insectivorous bird populations and potential causes. Conserv. Biol. 33, 1120–1130. doi: 10.1111/cobi.13307
Boys, E., (ed.). (2014). Encyclopaedia of Pests and Natural Enemies. Agriculture and Horticulture Development Board.
Cardenas, A. M., and Hidalgo, J. M. (2007). Application of the mean individual biomass (MIB) of ground beetles (coleoptera, carabidae) to assess the recovery process of the guadiamar green corridor (southern Iberian Peninsula). Biodiver. Conserv. 16, 4131–4146. doi: 10.1007/s10531-007-9211-5
Chiverton, P. A., and Sotherton, N. W. (1991). The effects on beneficial arthropods of the exclusion of herbicides from cereal crop edges. J. Appl. Ecol. 28, 1027–1039. doi: 10.2307/2404223
Clarke, J. H., Cook, S. K., Harris, D., Wiltshire, J. J. J., Henderson, I. G., Jones, N. E., et al. (2007). The SAFFIE Project Report. Boxworth: ADAS.
DEFRA (2010). Scoping Study to Investigate the Development of a Chick-Food Index. Project code PS2358. DEFRA. Available online at: http://randd.defra.gov.uk/Default.aspx?Menu=Menu&Module=More&Location=None&Completed=0&ProjectID=16999 (accessed March 10, 2019).
Dietrick, E. J. (1961). An improved backpack motorised fan for suction sampling of insects. J. Econ. Entomol. 54, 394–395. doi: 10.1093/jee/54.2.394
Ewald, J. A., and Aebischer, N. J. (2000). Trends in pesticide use and efficacy during 26 years of changing agriculture in southern England. Environ. Monitor. Assess. 64, 493–529. doi: 10.1023/A:1006295917190
Ewald, J. A., Wheatley, C. J., Aebischer, N. J., Moreby, S. J., Duffield, S. J., Crick, H. Q., et al. (2015). Influences of extreme weather, climate and pesticide use on invertebrates in cereal fields over 42 years. Glob. Change Biol. 21, 931–3950. doi: 10.1111/gcb.13026
Foster, S. P., Paul, V. L., Slater, R., Warren, A., Denholm, I., Field, L. M., et al. (2014). A mutation (L1014F) in the voltage-gated sodium channel of the grain aphid, Sitobion avenae, is associated with resistance to pyrethroid insecticides. Pest Manage. Sci. 70, 1249–1253. doi: 10.1002/ps.3683
Fried, G., Petit, S., Dessaint, F., and Reboud, X. (2009). Arable weed decline in Northern France: crop edges as refugia for weed conservation? Biol. Conserv. 142, 238–243. doi: 10.1016/j.biocon.2008.09.029
Gaba, S., Chauvel, B., Dessaint, F., Bretagnolle, V., and Petit, S. (2010). Weed species richness in winter wheat increases with landscape heterogeneity. Agric. Ecosyst. Environ. 138, 318–323. doi: 10.1016/j.agee.2010.06.005
Garibaldi, L. A., Carvalheiro, L. G., and Vaissière, B. E. (2016). Mutually beneficial pollinator diversity and crop yield outcomes in small and large farms. Science 351, 388–391. doi: 10.1126/science.aac7287
Gontijo, L. M. (2018). Engineering natural enemy shelters to enhance conservation biological control in field crops. Biol. Cont. 130, 155–163. doi: 10.1016/j.biocontrol.2018.10.014
Greenberg, C. H., and McGrane, A. (1996). A comparison of relative abundance and biomass of ground-dwelling arthropods under different forest management practices. For. Ecol. Manage. 89, 31–41. doi: 10.1016/S0378-1127(96)03868-6
Griffiths, G. J., Holland, J. M., Bailey, A., and Thomas, M. B. (2008). Efficacy and economics of shelter habitats for conservation biological control. Biol. Cont. 45, 200–209. doi: 10.1016/j.biocontrol.2007.09.002
Grim, T. (2006). An exceptionally high diversity of hoverflies (Syrphidae) in the food of the reed warbler (Acrocephalus scirpaceus). Biol. Bratisl. 61, 235–239. doi: 10.2478/s11756-006-0036-6
Hald, A. B. (1999). The impact of changing the season in which cereals are sown on the diversity of the weed flora in rotational fields in Denmark. J. Appl. Ecol. 36, 24–32. doi: 10.1046/j.1365-2664.1999.00364.x
Hallmann, C. A., Sorg, M., Jongejans, E., Siepel, H., Hofland, N., Schwan, H., et al. (2017). More than 75 percent decline over 27 years in total flying insect biomass in protected areas. PLoS ONE 12:e0185809. doi: 10.1371/journal.pone.0185809
Hawes, C., Haughton, A. J., Bohan, D. A., and Squire, G. R. (2009). Functional approaches for assessing plant and invertebrate abundance patterns in arable systems. Basic Appl. Ecol. 10, 34–42. doi: 10.1016/j.baae.2007.11.007
Heimpel, G., and Jervis, M. (2005). “Does floral nectar improve biological control?” in Plant Provided Food for Carnivorous Insects: A Protective Mutualism and its Applications, eds F. Wäckers, P. van Rijn, and J. Bruin (Cambridge: Cambridge University Press), 267–304.
Henderson, I. G., Holland, J. M., Storkey, J., Lutman, P., Orson, J., and Simper, J. (2012). Effects of the proportion and spatial arrangement of un-cropped land on breeding bird abundance in arable rotations. J. Appl. Ecol. 49, 883–891. doi: 10.1111/j.1365-2664.2012.02166.x
Herzog, F., Günter, M., Hofer, G., Jeanneret, P., Pfiffner, L., Schläpfer, F., et al. (1970). Restoration of agro-biodiversity in Switzerland. WIT Trans. Ecol. Environ. 46:10.
Hodge, I., and Reader, M. (2010). The introduction of entry level Stewardship in England: extension or dilution in agri-environment policy? Land Use Policy 27, 270–282. doi: 10.1016/j.landusepol.2009.03.005
Holland, J. M., Birkett, T., and Southway, S. (2009). Contrasting the farm-scale spatio-temporal dynamics of boundary and field overwintering predatory beetles in arable crops. Biocontrol 54, 19–33. doi: 10.1007/s10526-008-9152-2
Holland, J. M., Hutchison, M. A. S., Smith, B., and Aebischer, N. J. (2006). A review of invertebrates and seed-bearing plants as food for farmland birds in Europe. Ann. Appl. Biol. 148, 49–71. doi: 10.1111/j.1744-7348.2006.00039.x
Holland, J. M., Perry, J. N., and Winder, L. (1999). The within-field spatial and temporal distribution of arthropods in winter wheat. Bull. Entomol. Res. 89, 499–513. doi: 10.1017/S0007485399000656
Holland, J. M., Smith, B. M., Birkett, T. C., and Southway, S. (2012). Farmland bird invertebrate food provision in arable crops. Ann. Appl. Biol.160, 66–75. doi: 10.1111/j.1744-7348.2011.00521.x
Holland, J. M., Smith, B. M., Southway, S. E., Birkett, T. C., and Aebischer, N. J. (2008). The effect of crop, cultivation and seed addition for birds on surface weed seed densities in arable crops during winter. Weed Res. 48, 503–511. doi: 10.1111/j.1365-3180.2008.00663.x
Holland, J. M., Storkey, J., Lutman, P. J. W., Birkett, T. C., Simper, J., and Aebischer, N. J. (2014). Utilisation of agri-environment scheme habitats to enhance invertebrate ecosystem service providers. Agric. Ecosyst. Environ. 183, 103–109. doi: 10.1016/j.agee.2013.10.025
Holland, J. M., Winder, L., Woolley, C., Alexander, C. J., and Perry, J. N. (2004). The spatial dynamics of crop and ground active predatory arthropods and their aphid prey in winter wheat. Bull. Entomol. Res. 94, 419–431. doi: 10.1079/BER2004323
Holmes, R. J., and Froud-Williams, R. J. (2005). Post-dispersal weed seed predation by avian and non-avian predators. Agric. Ecosyst. Environ. 105, 23–27. doi: 10.1016/j.agee.2004.06.005
Huang, H., Deng, J., Lan, Y., Yang, A., Deng, X., Wen, S., et al. (2018). Accurate weed mapping and prescription map generation based on fully convolutional networks using UAV imagery. Sensors 18:3299. doi: 10.3390/s18103299
Jones, N. E., and Smith, B. M. (2007). Effects of selective herbicide treatment, row width and spring cultivation on weed and arthropod communities in winter wheat. Aspects Appl. Ecol. 81, 39–46.
Keenleyside, C., Allen, B., Hart, K., Menadue, H., Stefanova, V., Prazan, J., et al. (2011). Delivering Environmental Benefits Through Entry Level Agri-Environment Schemes in the EU. Report Prepared for DG Environment, Project ENV.B.1/ETU/2010/0035. Institute for European Environmental Policy, London.
Lanz, B., Dietz, S., and Swanson, T. (2018). The expansion of modern agriculture and global biodiversity decline: an integrated assessment. Ecol. Econo. 144, 260–277. doi: 10.1016/j.ecolecon.2017.07.018
Marshall, E. J. P., and Arnold, G. M. (1994). Weed seed banks in arable fields under contrasting pesticide regimes. Ann. Appl. Biol. 125, 349–360. doi: 10.1111/j.1744-7348.1994.tb04975.x
Marshall, E. J. P., Brown, V. K., Boatman, N. D., Lutman, P. J. W., Squire, G. R., and Ward, L. K. (2003). The role of weeds in supporting biological diversity within crop fields. Weed Res. 43, 77–89. doi: 10.1046/j.1365-3180.2003.00326.x
Moreby, S. J., and Sotherton, N. W. (1997). A comparison of some important chick-food insect groups found in organic and conventionally-grown winter wheat fields in southern England. Biol. Agric. Horticult. 15, 51–60. doi: 10.1080/01448765.1997.9755181
Morris, A. J., Holland, J. M., Smith, B., and Jones, N. E. (2004). Sustainable arable farming for an improved environment (SAFFIE): managing winter wheat sward structure for Skylarks Alauda arvensis. Ibis 146, 155–162. doi: 10.1111/j.1474-919X.2004.00361.x
Nentwig, W. (1982). Why do certain insects escape from a spiders web? Oecologia 53, 412–417. doi: 10.1007/BF00389023
Newton, I. (2004). The recent declines of farmland bird populations in Britain: an appraisal of causal factors and conservation actions. Ibis 146, 579–600. doi: 10.1111/j.1474-919X.2004.00375.x
Norris, R. F., and Kogan, M. (2005). Ecology of interactions between weeds and arthropods. Ann. Rev. Entomol. 50, 479–503. doi: 10.1146/annurev.ento.49.061802.123218
Pearson, D. E., Icasatti, N. S., Hierro, J. L., and Bird, B. J. (2014). Are local filters blind to provenance? Ant seed predation suppresses exotic plants more than natives. PLoS ONE 9:e103824. doi: 10.1371/journal.pone.0103824
Petit, S., Boursault, A., and Bohan, D. A. (2014). Weed seed choice by carabid beetles (Coleoptera: Carabidae): linking field measurements with laboratory diet assessments. Eur. J. Entomol. 111, 615–620. doi: 10.14411/eje.2014.086
Petit, S., Boursault, A., Le Guilloux, M., Munier-Jolain, N., and Reboud, X. (2011). Weeds in agricultural landscapes. Rev. Agron. Sustain. Dev. 31, 309–317. doi: 10.1051/agro/2010020
Potts, G. R. (1991). “The environmental and ecological importance of cereal fields,” in The Ecology of Temperate Cereal Fields, eds L. G. Firbank, N. Carter, J. F. Darbyshire, and G. R. Potts (Oxford: Blackwell Scientific Publications), 3–21.
Potts, G. R., and Aebischer, N. J. (1991). “Modelling the population dynamics of the Grey Partridge: conservation and management,” in Bird Population Studies: Their Relevance to Conservation and Management, eds C. M. Perrins, J. -D. Lebreton, and G. J. M. Hirons (Oxford: Oxford University Press), 373–390.
Potts, G. R., Ewald, J. A., and Aebischer, N. J. (2010). Long-term changes in the flora of the cereal ecosystem on the Sussex Downs, England, focusing on the years 1968–2005. J. Appl. Ecol. 47, 215–226. doi: 10.1111/j.1365-2664.2009.01742.x
Pywell, R. F., Heard, M. S., Woodcock, B. A., Hinsley, S., Ridding, L., Nowakowski, M., et al. (2015). Wildlife-friendly farming increases crop yield: evidence for ecological intensification. Proc. R. Soc. B 282:20151740. doi: 10.1098/rspb.2015.1740
Richner, N., Holderegger, R., Linder, H. P., and Walter, T. (2015). Reviewing change in the arable flora of Europe: a meta-analysis. Weed Res. 55, 1–13. doi: 10.1111/wre.12123
Robinson, R. A., and Sutherland, W. J. (2002). Post-war changes in arable farming and biodiversity in Great Britain. J. Appl. Ecol. 39, 157–176. doi: 10.1046/j.1365-2664.2002.00695.x
Rogers, L. E., Hinds, W. T., and Buschbom. (1975). A general weight vs. length relationship for insects. Ann. Entomol. Soc. Am. 69, 387–389. doi: 10.1093/aesa/69.2.387
Ryszkowski, L., and Kark, K. (1977). Variabiity in biomass of epigeic insects in agricultural landscape. Ekol. Polska 25, 501–517.
Sánchez-Bayo, F., and Wyckhuys, K. A. (2019). Worldwide decline of the entomofauna: a review of its drivers. Biol. Conserv. 232, 8–27. doi: 10.1016/j.biocon.2019.01.020
Seifert, C., Leuschner, C., and Culmsee, H. (2015). Arable plant diversity on conventional cropland—The role of crop species, management and environment. Agric. Ecosyst. Environ. 213, 151–163. doi: 10.1016/j.agee.2015.07.017
Shewry, P. R., and Hey, S. J. (2015). The contribution of wheat to human diet and health. Food Energy Secur. 4, 178–202. doi: 10.1002/fes3.64
Siemann, E., Tilman, D., Haarstad, J., and Ritchie, M. (1998). Experimental tests of the dependence of arthropod diversity on plant diversity. Am. Nat. 152, 738–750. doi: 10.1086/286204
Smith, B., Holland, J., Jones, N., Moreby, S., Morris, A. J., and Southway, S. (2009). Enhancing invertebrate food resources for skylarks in cereal ecosystems: how useful are in-crop agri-environment scheme management options? J. Appl. Ecol. 46, 692–702. doi: 10.1111/j.1365-2664.2009.01638.x
Sotherton, N. W. (1991). “Conservation headlands: a practical combination of intensive cereal farming and conservation,” in The Ecology of Temperate Cereal Fields, eds L. G. Firbank, N. Carter, J. F. Darbyshire, and G. R. Potts (Oxford: Blackwell Scientific Publications), 373–397.
Southwood, T. R. E., and Cross, D. J. (1969). The ecology of the partridge. J. Anim. Ecol. 38, 497–509. doi: 10.2307/3030
Stoate, C. (2017). The Allerton Project's first 25 years: a rich seam of evidence to support farmland conservation. Brit. Wildlife 28, 392–397.
Storkey, J., Brooks, D., Haughton, A., Hawes, C., Smith, B. M., and Holland, J. M. (2013). Using functional traits to quantify the value of plant communities to invertebrate ecosystem service providers in arable landscapes. J. Ecol. 101, 38–46. doi: 10.1111/1365-2745.12020
Storkey, J., and Cussans, J. W. (2007). Reconciling the conservation of in-field biodiversity with crop production using a simulation model of weed growth and competition. Agric. Ecosyst. Environ. 122, 173–182. doi: 10.1016/j.agee.2006.12.031
Storkey, J., Meyer, S., Still, K. S., and Leuschner, C. (2011). The impact of agricultural intensification and land-use change on the European arable flora. Proc. R. Soc. Biol. Sci. 279, 1421–1429. doi: 10.1098/rspb.2011.1686
Storkey, J., and Neve, P. (2018). What good is weed diversity? Weed Res. 58, 239–243. doi: 10.1111/wre.12310
Storkey, J., and Westbury, D. B. (2007). Managing arable weeds for biodiversity. Pest Manag. Sci. 63, 517–523. doi: 10.1002/ps.1375
Sutcliffe, O. L., and Kay, Q. O. (2000). Changes in the arable flora of central southern England since the 1960s. Biol. Conserv. 93, 1–8. doi: 10.1016/S0006-3207(99)00119-6
Swanton, C. J., Weaver, S., Cowan, P., Acker, R. V., Deen, W., and Shreshta, A. (1999). Weed thresholds: theory and applicability. J. Crop Prod. 2, 9–29. doi: 10.1300/J144v02n01_02
Thomas, S. R., Goulson, D., and Holland, J. M. (2001). Resource provision for farmland gamebirds: the value of beetle banks. Ann. Appl. Biol. 139, 111–118. doi: 10.1111/j.1744-7348.2001.tb00135.x
Tooley, J., and Brust, J. (2002). “Weed seed predation by carabid beetles,” in The Agroecology of Carabid Beetles, ed J. M. Holland (Andover, MA: Intercept Limited).
Tschumi, M., Albrecht, M., Bärtschi, C., Collatz, J., Entling, M. H., and Jacot, K. (2016). Perennial, species-rich wildflower strips enhance pest control and crop yield. Agric. Ecosyst. Environ. 220, 97–103. doi: 10.1016/j.agee.2016.01.001
Veres, A., Petit, S., Conord, C., and Lavigne, C. (2013). Does landscape composition affect pest abundance and their control by natural enemies? A review. Agric. Ecosyst. Environ. 166, 110–117. doi: 10.1016/j.agee.2011.05.027
Vickery, J. A., Feber, R. E., and Fuller, R. J. (2009). Arable field margins managed for biodiversity conservation: a review of food resource provision for farmland birds. Agric. Ecosyst. Environ. 133, 1–13. doi: 10.1016/j.agee.2009.05.012
Walker, K. J., Critchley, C. N. R., Sherwood, A. J., Large, R., Nuttall, P., Hulmes, S., et al. (2006). Effectiveness of new agri-environment schemes in conserving arable plants in intensively farmed landscapes. Biol. Conserv. 129, 192–206. doi: 10.1016/j.biocon.2005.10.034
Westerman, P. R., Hofman, A., Vet, L. E. M., and Van Der Werf, W. (2003). Relative importance of vertebrates and invertebrates in epigeaic weed seed predation in organic cereal fields. Agric. Ecosyst. Environ. 95, 417–425. doi: 10.1016/S0167-8809(02)00224-4
Keywords: invertebrates, farmland biodiversity conservation, functional biodiversity, arable weeds, farmland bird conservation
Citation: Smith BM, Aebischer NJ, Ewald J, Moreby S, Potter C and Holland JM (2020) The Potential of Arable Weeds to Reverse Invertebrate Declines and Associated Ecosystem Services in Cereal Crops. Front. Sustain. Food Syst. 3:118. doi: 10.3389/fsufs.2019.00118
Received: 21 June 2019; Accepted: 09 December 2019;
Published: 10 January 2020.
Edited by:
Stacy Michelle Philpott, University of California, Santa Cruz, United StatesReviewed by:
John Michael Lynch, University of Oxford, United KingdomJustin Andrew Johnson, University of Minnesota Twin Cities, United States
Copyright © 2020 Smith, Aebischer, Ewald, Moreby, Potter and Holland. This is an open-access article distributed under the terms of the Creative Commons Attribution License (CC BY). The use, distribution or reproduction in other forums is permitted, provided the original author(s) and the copyright owner(s) are credited and that the original publication in this journal is cited, in accordance with accepted academic practice. No use, distribution or reproduction is permitted which does not comply with these terms.
*Correspondence: Barbara M. Smith, YmFyYmFyYS5zbWl0aCYjeDAwMDQwO2NvdmVudHJ5LmFjLnVr