- 1Manaaki Whenua Landcare Research, Lincoln, New Zealand
- 2School of Physical and Chemical Sciences, University of Canterbury, Christchurch, New Zealand
- 3Institute of Environmental Science and Research Limited, Christchurch, New Zealand
Biowastes can enhance the establishment of New Zealand (NZ) native vegetation, particularly on degraded land, where biological or physicochemical deficiencies limit plant growth. We identified critical success factors influencing selection and use of biowastes for a) growing native plants and b) rehabilitating native ecosystems. These were: weed competition; resilience (especially to drought and storms); ecosystem succession; and soil microbiome responses to elevated Nitrogen, Phosphorus, Organic Matter (OM) and contaminants (including Trace Elements). These factors determine the selection, timing and methods of biowaste application, selection of plant species and target ecosystems, and post-application management of receiving sites. Commonly planted native NZ species benefit from biowastes application where soils have depleted OM or sites with surface crusting, erosion, or high exposure to wind and temperature fluctuations. Commonly planted native species display luxury uptake of macro-nutrients, even on soils that are low-fertility under agricultural standards. Therefore, the growth responses to additional nutrients may be small. In contrast, most exotic weeds outcompete NZ species in high fertility soils. Therefore, biowaste application should not result in excessive nutrient availability. This can be achieved by using blends that contain a high-carbon biowaste, such as wood-waste, which immobilizes some macronutrients in the short term. We recommend long-term research using mixed-species field trials to identify biowastes and application methods that together support development of resilient native ecosystems. In particular, research should determine the role of biowastes on the beneficial mycorrhizae and native soil fauna. Application methods that enhance heterogeneity may help retain and/or build responsive microbiomes while conserving “native” microbiomes to deliver sustainable, rehabilitated native ecosystems.
Introduction
New Zealand (NZ) is re-establishing large areas into native species and ecosystems, especially on land that is marginal for pasture or plantation forestry due to low productivity or high environmental risks associated with grazing stock or clear-fell harvesting of trees (Davis et al., 2013). Recent initiatives have included “million tree” projects in NZ's largest city1 and on Te Runanga o Ngai Tahu farms (tribally owned), and a nationwide “One Billion Trees Programme”2. Native seedlings are being planted into newly-fenced riparian areas on farms to help improve water quality under the 2018 Good Farming Action Plan for Water Quality3 and into low-producing areas of farms to produce high-value honey from mānuka (Leptospermum scoparium). Native biodiversity is recognized as contributing to a wide range of ecosystem services on farmland (Maseyk et al., 2019). Finally, significant areas of non-native plantation forests are being converted at harvest to permanent native forests, especially under new National Environmental Standards for Plantation Forestry which restrict planting areas that are highly vulnerable to erosion when clear-fell harvested.
Most agricultural landscapes in NZ contain little native vegetation. Repeated burning following Polynesian settlement in the thirteenth century reduced the original closed-forest cover by nearly 40% in one of the most rapid and complete landscape transformations in the world (McWethy et al., 2010). Following European arrival, most of the remaining native plant cover was removed from lowland NZ and replaced with farms, non-native forests, and urban areas (Ministry for the Environment, 2000; Walker et al., 2006; Ministry for the Environment Statistics New Zealand, 2015). Intensification of farming in recent decades has steadily removed residual native biodiversity in these cleared areas through clearing, draining, plowing and/or irrigation. In addition, inorganic Phosphate (P) fertilizers and lime has been applied because most NZ soils are naturally acidic and have sub-optimal levels of available soil P for growing pastures, crops, and non-native plantation trees (Will, 1985; McLaren and Cameron, 1996). Less than 10% of indigenous vegetation cover remains in fertile lowland areas (Walker et al., 2006), and in the Canterbury plains (a large, agricultural landscape) the number of native plant and bird species seen while driving over 150 km may be “counted on one hand” (Norton and Reid, 2013).
Biowastes comprise “unwanted material” of biological origin such as products of sewage treatment (Sanchez et al., 2009; Guo et al., 2014), animal effluents (Paavola et al., 2006), crop residues, and sawdust. Disposal of biowastes can be expensive e.g., disposal to landfill (Güereca et al., 2006), and environmentally damaging if disposed to waterways (Correa et al., 2006), incinerated (Werther and Ogada, 1999), or injudiciously applied to land with increased risks to human health (Pritchard et al., 2010). However, some biowastes can be beneficially applied to land to improve soil fertility (Esperschuetz et al., 2016). In NZ, social attitudes and indigenous values restrict use of biowastes containing human effluent for food (Ataria et al., 2016). Linked to this are NZ industry constraints on the application of sewage wastes. For example, Fonterra, a NZ company that is the world's largest dairy exporter, does not allow suppliers to use feed grown with municipal biosolids. Resistance to reusing biosolids in NZ is also partly due to a lack of precedent, as NZ farmers have tended to use inorganic fertilizers, particularly P, to support pasture and crop growth. The result is that NZ landfills about 59% of its biosolids (ANZBP, 2017) contrasting with Europe and Asia, where there is a long history of recycling of organic wastes—including human wastes from cities—to agricultural land rural as fertilizers. NZ also contrasts with neighbor Australia, which uses most of its biosolids productively; 75% in agriculture in 2017.
Potentially, biowastes could be used to re-establish native vegetation on land that is physically, biologically or chemically degraded (Gutiérrez-Ginés et al., 2017b). The latter driver has been proposed since at least 2002 in NZ (O'Connor et al., 2002); more recently by Dickinson et al. (2015), Esperschuetz et al. (2017b), and Gutiérrez-Ginés et al. (2019). Native plant species are increasingly grown for honey, timber, oils, fiber, and medicinal extracts4. Biowaste application could increase productivity and/or qualities of such harvested native plants and enhance carbon sequestration and/or ecosystem rehabilitation.
In this review, we aim to identify the critical success factors influencing selection and use of biowastes for (a) growing native plants and (b) rehabilitating native terrestrial ecosystems. First, we describe the characteristics of NZ biowastes, then look at ways biowastes are used to support plant growth and land production. Given the lack of field data, we draw on inorganic fertilizer trials with native species and ecosystems (Langer et al., 1999; Norton et al., 2013; Franklin et al., 2015; Simcock and Ross, 2017) to support directly-relevant biowastes research. The greatest number of NZ studies that can inform long-term biowaste use are in non-native plantation forests. Most of these plantations were established to stabilize and produce revenue from “waste” land with low productivity due to low OM and highly vulnerability to erosion if denuded, for example, Raw Soils on coastal sand dunes in Horowhenua (The Pot), Canterbury (Bottle Lake) and Nelson (Rabbit Island) regions, and Pumice Soils of the Central North Island.
We then focus on how native plants and other components of native ecosystems such as soil animals and mycorrhizae may benefit from, or be adversely impacted by, different biowastes and application methods. The interactions between species, particularly with competing weed species, are reported. Most studies investigating the interactions between biowastes and NZ native plants have been conducted in highly controlled glasshouse and laboratory trials (e.g., Xue et al., 2012; Waterhouse et al., 2014; Gutiérrez-Ginés et al., 2017b; Seyedalikhani et al., 2019 with few field studies Parker, 2011; Xue et al., 2015. Most research has used municipal biosolids from three urban areas (Auckland, Kaikoura, or Christchurch), although these biowastes have changed as treatment processes and inputs changed. We conclude by identifying critical knowledge gaps and recommend research to fill these gaps.
Characteristics and General Effects of New Zealand Biowastes
The potential benefits from biowaste application depend on the nature of the biowaste, how it is applied, and the receiving soils and site conditions. As an agricultural and horticultural exporter, NZ generates a wide range of organic byproducts: fruit, vineyard and orchard waste materials, and animal-processing wastes, including those from the marine industry, notably mussel and oyster shell. Common high-volume biowastes with low solids content are dairy-shed effluents, municipal effluents, and discharges from agricultural industries such as wool scouring-, dairy- and meat-processors, along with their human equivalents (gray water and land-based effluent fields). Biowastes investigated for beneficial land application include urban organic wastes (road sweepings and road catch-pit contents, garden wastes) and crop residues (e.g., straw or oat husk), including those used as bedding for farm animals such as poultry or cattle.
Although all biowastes contain OM, the properties of individual biowastes are strongly influenced by source materials (especially concentrations of trace elements, TE trace elements (including trace elements) and emerging organic contaminant, EOCs), and the type and extent of processing, including composting and removal or addition of water (Table 1). There is greater concern over biowaste derived from municipal wastewater systems because of the wider range of novel contaminants, for example new biocides and pharmaceutical products entering consumer markets (e.g., Triclosan, nano-silver, microplastics) (Muñoz et al., 2009). Industrial effluents also change, for example, Hart and Speir (1992) reported process changes at a Canterbury meat-works that elevated inorganic sulfur (S), sodium (Na), chlorides, and chromium to levels that degraded receiving soils, and reversed the benefits of decades of previous biowaste applications.
Wastewater
Wastewaters typically have low concentrations of contaminants compared with solid waste, since they are composed of approx. 99% water and 1% solids (UN-Water, 2015). Such wastes are irrigated at application rates that may maximize disposal rates within environmental limits to limit the land area required for individual factories and cities to dispose of waste products. Environmental limits include avoiding excessive leaching of N and P and/or inducing anaerobic conditions that impact on plant health. In New Zealand, municipal liquid wastes are applied using surface or subsurface irrigation at frequencies ranging from daily to weekly. Wastewaters typically have low concentration of N and P, but daily or weekly application can equate to large annual kg/ha inputs. For example, O'Connor et al. (2002) reported sewage wastewater, with 40 and 9 g/m3 N and P, respectively, was applied at annual rates of about 500 kg N/ha and 110 kg P/ha. Some wastewaters can have high Na or potassium (K) concentrations or build up to high concentrations if applied at high rates (Haynes and Naidu, 1998).
Solids and Slurries
Biowaste solids include slurries, semi-liquid residues with more than 20–25% solids by dry weight, composts, and plant residues which vary in key nutrients and contaminants (Table 1). Biosolids in particular can have a wide range of moisture contents depending on processing and storage methods; hence one product can vary from slurry to solids. However, all these materials are too dense to be irrigated, so may be applied using spreaders on vehicles. As nutrient-density increases, such materials are economic to use further from source, as cartage costs are lower relative to their nutrient or amendment value, but they are still relatively less economic to transport long distances than most chemical fertilizers. Biowastes with high solids content have the advantage of being more easily applied at the high rates needed to ameliorate degraded soil OM. Weber et al. (2012) describe the value of repeated municipal sludge applications for rehabilitation of infertile, mined land to plantation forests in Waikato. At the same site, sustainable pasture rehabilitation required a one-off basal application of at least 100 dry t/ha of composted municipal biosolids (Simcock and Xue, 2017). Some NZ biosolids are treated by alkaline stabilization, in which waste materials such as lime, cement kiln dust, coal or fly-ash are added to stabilize carbon and reduce leaching of TEs (trace elements) (notably Zn). This process is also reported to reduce N fertilizer value by increasing NH3 volatilization (Wang et al., 2008), and hence contributing to green-house gas emissions.
Solid biowastes include wood wastes (Table 1) generated by large volumes of exported logs, and bark from log storage and processing areas processed with other agricultural biowastes to produce high-value growing media after composting, screening, and blending. Such plantation forest biowastes have particular value as “clean” materials (i.e., with low contaminants such as HM or EOC) that can facilitate co-disposal of other biowastes. For example, Feitjie and Popay (1992) developed horticultural growing media (3%N, 0.02%P, 0.06% K) and soil amendments from pine bark and sheep blood, and from fish waste products (Feitje and Petrie, 1992). Other silvicultural and forest processing byproducts include sawdust and paper mill sludges; post peelings, and untreated timbers shredded for re-use as urban landscaping mulches and applied at 5–10-cm depth. Such biowastes have minimal seasonal variation compared with materials derived from municipal green wastes and street sweepings in areas with exotic deciduous trees that dominate many urban areas (Depree, 2008).
Impacts of Biowastes on New Zealand Soils
Influence of Biowastes on Soil Carbon and Nutrients
Biowastes can increase soil carbon (Wang et al., 2008). Through the processes of immobilization and sorption, this carbon may increase plant available N, P, K, and S (McLaren and Cameron, 1996) unless these elements are present in high concentrations in the biowaste itself (Esperschuetz et al., 2016). Most land-based municipal wastewater sites in NZ have low initial carbon contents, so they respond to relatively moderate levels of biowaste additions. The duration of soil response is influenced by stability of the biowaste. Composted material has a proportionately greater effect on soil carbon because it has a higher proportion of stable carbons and lower proportion of easily decomposed (labile) carbon. Microbiological activity/turnover in receiving soils also influences the soil carbon response, as does the “readiness” of the soil microbial community.
NZ has large areas of soils with high surface charge due to high Al oxides; these Allophanic Soils can maintain substantially higher “base” concentrations of soil carbon (Sparling and Schipper, 2004). The high surface charge in these soils and Oxidic Soils (which have high Fe oxides) also moderates plant responses by moderating the readily, plant-available , commonly reported in New Zealand literature as “Olsen P.” The strength of response to applied P is indicated by “ retention,” or anion retention capacity (McLaren and Cameron, 1996; Drewry et al., 2013). Low P limits growth of many non-native plants in New Zealand, particularly legumes, as most NZ soils are naturally deficient (Olsen P 0–5 mg/kg). NZ target Olsen P for agricultural pasture production are 20–35 mg/kg for sedimentary soils (Mackay et al., 2013).
The liming effect of some biowaste can help counter effects of acidification. However, the main biowastes studied in NZ are weakly to strongly acidic with the impact on soil pH influenced by soil buffering capacity. For example, a municipal biowaste with pH 4.3 strongly acidified a Raw Sand with low buffering potential (initial Total Carbon 0.13% w/w) as soil pH dropped from 8.8 to 5.2 (Gutiérrez-Ginés et al., 2017b). However, the same biosolid added to a well-buffered Orthic Brown Soil with initial 3.9% Total Carbon increased pH from 3.9 to 4.9. Acidification is most severe in biowastes with low C:N ratios and high N loading rates. The potential leaching losses of N associated with high N loading rates can be mitigated by co-disposal with woody wastes such as biochar (Knowles et al., 2011) or sawdust (Paramashivam et al., 2016, 2017). In the latter, microbe-extraction of N can be so effective that plant growth is suppressed. For example, blending 3 t/ha sawdust with 1,250 kg N/ha biosolids reduced ryegrass biomass by about 25% compared with unamended municipal biosolids (Esperschuetz et al., 2016). High inorganic N in biowastes can also enhance TE mobility, particularly Zn and Cd, which have less affinity for organic matter than Cr and Pb (Smith et al., 1992).
Waste waters with high Na or K concentrations can reduce soil infiltration rates if soil dispersion causes loss of structure until Na or K is leached (Haynes and Naidu, 1998). However, soil dispersion only affects permeability when the receiving soils have an appreciable clay content (Hart and Speir, 1992). Infiltration can also be impeded if wastewater physically clog soil pores or cause biological growths (Bedbabis et al., 2014).
Influence of Soil Water and Oxygen on Plant Response to Biowaste
The benefits of wastewaters for plant growth are influenced by the frequency, volumes, and timing of application relative to plant water demand. The water in wastewaters and slurries benefits plant growth when application mitigates moisture stress and extends growth periods. These effects may be in addition to those generated from biowaste (Roberts et al., 1992). However, in the absence of moisture or nutrient stress, plant responses may be absent, for example Dixon et al. (2007) found no yield benefit from three types of mulches placed at 10-cm depth (composts, post peelings or bark chips), likely because the orchard soil was fertile and the avocados were irrigated. Plant growth responses may also not occur if biowastes exacerbate plant water demand and water is limiting (Clinton and Leckie, 2002). They reported lack of an anticipated growth response of 6-year-old pines to high rates of biosolids (800 kg N/ha equivalent) may have been masked by earlier onset of drought stress because the high N increased transpiration.
Biowastes are unlikely to have any beneficial effect where soils are, or become, waterlogged unless the method of biowaste application will fracture soils to improve drainage (e.g., biowaste injection). Sims et al. (1994) and Simcock and Xue (2017) reported Eucalyptus and Pinus radiata growth were reduced when biowastes were applied under anerobic conditions. In the former, coppicing severely reduced tree water uptake, necessitating reduced wastewater application rates until tree water use (and root development) recovered. In the latter, a combination of poor soil physical condition and low slope created poor drainage that prevented tree response to improved fertility from the biowaste. Adverse soil physical conditions were also proposed as major limitations to native karamu (Coprosma robusta) growth in a field study where growth did not respond to N or P, despite glasshouse trials indicating marked responses to these nutrients (Davis and Langer, 1997). Surface mulches applied to depths that suppress evaporation may also exacerbate the duration and severity of anoxic soil conditions in the underlying soils, as can wastewaters (Atkinson et al., 1992).
Using Biowastes for Growing Native Vegetation
Methods of Establishing Native Vegetation
Before discussing the effects of biowastes on native plants and ecosystems, it is useful to understand the methods used to establish native NZ vegetation since the late 1980s as this influences the success of biowaste use. The dominant establishment method is planting nursery-raised seedlings (Pollock, 1986; Porteous, 1993; Peters and Clarkson, 2010) as native woody species are typically poorly represented in seed banks in most areas where native plants are established (i.e., farms and cities). Planting is the only practical option where competition from non-native species is intense enough to prevent successful seeding. Ineffective control of competing vegetation is the most common reason for the failure of native plantings; Will (1985) and Sullivan et al. (2009) report adverse effects of weed competition on native plantings and plantation trees, respectively. NZ's naturalized weed flora exceeds the number of native species, and non-native plants dominate nearly all lowland environments because they are more effective dispersers, seed earlier, and grow faster and/or taller. Many non-native plants are also N fixers (e.g., gorse, broom, acacia, hakea, clovers, lotus) and/or are better adapted to living with mammals by having prickles or thorns, or through being able to regrow after intensive browsing (Wardle, 1991).
Where weed competition is intense, taller native seedlings (>50 cm) are planted as these require less frequent “releasing” (Pardy et al., 1992). Weeds compete both above ground, for space and light, and below ground for nutrients and moisture, with the latter being the most damaging in terms of survival and growth (Will, 1985). Such competition is typically managed at planting by placing fertilizer below the root ball to avoid stimulating weeds, and at smaller sites organic mulches are spread to conserve water and slow weed establishment. Organic mulches are produced from cleared trees, plantation by-products, packing cases or green wastes. However, at large revegetation scales, mulching is not practical. Instead, establishment techniques from plantation forestry using pre- and post-plant herbicides are often adopted. Native planting densities of 2,500 to 10,000 plants/ha are used to achieve early canopy closure and weed suppression, higher than the 400–1,000 plants/ha of plantation forestry. Plantation forestry also uses facultative living mulches (often cereals, grasses or herbaceous legumes) to suppress weed growth and stabilize soils, but this approach is currently under-utilized in native plantings, along with bare-rooted plants and pre-planting cultivation treatments (e.g., ripping or spot mounding) used in plantation forestry to enhance drainage and mitigate compaction.
Response of NZ Native Plants to Fertilizers
Native NZ plants are generally, but not exclusively, adapted to soils with low available N and P so growth responses to added nutrients are often low (Stevenson and Smale, 2005; Dickinson et al., 2015; Franklin et al., 2015, Appendix). The magnitude of response is influenced by the severity of nutrient deficiency and the availability of soluble N and P in amendments. Most native plant growth experiments show a plateau in plant response to increasing fertility, and this plateau occurs at a lower concentration than for agricultural species typically used for comparison. For example, Franklin et al. (2015) added increasing amounts of N as urea to native plants. The eight native species were tolerant to highly elevated N, from 200 to 1,600 kg/ha, as they were able to assimilate N into foliage through “luxury uptake.” Luxury uptake occurs when a plant takes up nutrients in excess of levels that increase plant growth; it is a feature of plants that grow slowly and/or in low-fertility conditions or where growth is limited by other nutrients (Iversen et al., 2010). Luxury uptake as a response to applied fertilizer has also been reported for P in manuka by Hall (1977), where it was regarded as representing a substantial P reserve for growing seedlings. Low background fertility conditions are typical of earlier NZ fertilizer trials on mine tailings, which typically have negligible OM and very low N. This research showed above-ground growth of some woody native species responded strongly to treatments containing N but not for treatments with only P (karamu, Coprosma robusta and kahikatea, Dacrycarpus dacrydioides), whereas other woody species (red beech, Fuscospora fusca and marble leaf, Carpodetus serratus) responded more strongly to applied P (Langer et al., 1999). In a linked trial, only foliar N increased, with concentrations in plants grown in replaced topsoil more than double that of plants on infertile mine tailings. Glasshouse trials with kānuka (Kunzea serotina) in a low fertility soil also showed increased growth with the addition of N but not with additional P (Dollery, 2017).
Response of Native Plants to Nutrients Applied as Biowastes
Responses of native plants to biowastes are also influenced by baseline soil fertility and differ between plant species. Overall biomass and height responses are strongest in lowest fertility soils and for biosolids with the highest available N (Reis et al., 2017; Seyedalikhani et al., 2019), i.e., anaerobic digested biosolids with high and low rather than mature, stockpiled biosolids with low . Biomass increases can be dramatic where baseline soils are extremely infertile, e.g., Reis et al. (2017) reported a 40-fold increase in mānuka biomass from low-fertility sand amended with 90 t/ha of aged stockpiled biosolids. Vermi-composts offer benefits of a slower N and P release that may better match the lower demand of native plants. Xue et al. (2012, 2016) report pot trials amending pumice subsoil (0.02% C, 0.01% N, 0.005% total P) with vermi-composts made from septic tank or dairy-shed solid waste and horticultural factory greenwastes. The vermi-composts (1.6 to 2.1%N and 0.34 to 0.38%P) supplied adequate P for seedling mānuka and totara (Podocarpus totara) growth and were at least as effective as Di-ammonium phosphate. However, vermi-compost did not supply adequate P for the much faster-growing (non-native) radiata pine seedlings, which had two to ten times greater dry weights than the native species.
A positive height and/or biomass growth response in native species is usually linked to a lower shoot: root ratio (Waterhouse et al., 2014; Seyedalikhani et al., 2019). For example, only above-ground biomass of tussock (Chionochloa rubra) and mānuka responded to 20% v/v biosolids addition, not root biomass, even though the control was an acidic, infertile mine soil (Seyedalikhani et al., 2019). Native monocots, for example harakeke (flax, Phormium spp.), toetoe (Austroderia spp.), ti kouka (Cordyline australis), and sedges (Carex species) generally respond more strongly to fertilizers than native woody plants (dicotyledons) (Parker, 2011; Gutiérrez-Ginés et al., 2017b).
Pot trials indicate biowaste-stimulated increases in biomass of kānuka (Kunzea ericoides) and mānuka retain essential oil qualities (a potentially lucrative industry) at application rates equivalent to over 2,500 kg N/ha (Seyedalikhani et al., 2019). Native plants can deliver specific ecosystem benefits, for example, mānuka limits amplification of some HM contaminants typically elevated in biowastes; Morrell (1997) identified low foliar bioaccumulation of TE in native plants as a particular benefit in trials using municipal biosolids to revegetate toxic, base-metal mine tailings.
Effects of Native Plants on Biowastes
Some native species, notably kānuka and mānuka, also mitigate microbiological pathogens present in some biowastes (Prosser et al., 2016). In vitro and lysimeter studies show mānuka also inhibits nitrification processes in soil, potentially reducing leaching; both actions could enhance protection of surface and ground water (Esperschuetz et al., 2017a). Recent field experiments are testing the efficacy of planting these species into riparian areas of farms and areas used for municipal wastewater-disposal. Early results of Escherichia coli, bromide, and dye tracing suggest enhanced bacterial bypass flow under mānuka and kānuka and predict that this enhanced preferential flow reduces the risk of surface pathogen runoff compared with pasture (Mishra, 2018). Further, this bypass flow route directs surface-applied pathogens toward the anti-microbial root compounds, increasing the chance of pathogen reduction in soils. Some native plants, such as mānuka, preferentially seek such enriched areas (Reis et al., 2017; Gutiérrez-Ginés et al., 2019) so could take advantage of discrete placement of high-nutrient biowastes.
Responses of Native Ecosystems to Biowastes
Weeds
Pot and field trials investigating responses of individual native plants to a range of biowastes have shown above-ground biomass increases with the increased availability of N and/or P if these are limiting in the soil, but no root biomass response. Further, the N or P concentration at which native plants uptake luxury amounts of nutrients without necessarily increasing growth rates is lower than common “control” species such as (non-native) grasses, crops or pine trees. Therefore, in the presence of competition, native plants are generally disadvantaged by improved soil chemical fertility. Dollery (2017) reported fertilization (of 470 kg P/ha) and organic amendments had either negligible or detrimental effects on native species due to stimulation of competition from non-native weeds to develop. This research was unusual in reporting the impact on native moss species; there was a strong relationship between increase in exotic grasses and decrease in moss cover, as mosses are vulnerable to shading by grass. But increasing fertility can advantage natives where levels “release” natives from growth limitations but are low enough to limit growth of competing weeds, e.g., an Olsen P of 16 mg/kg for native species in pasture (Franklin et al., 2015). Weed control to allow native seedlings to grow is particularly critical in northern and lowland areas due to competing vine, shrub and tree weeds (Ross and Crequer, 2006; Sullivan et al., 2009). Specifically, control of pasture (grasses and herbaceous, particularly Lotus conrniculatus) was critical in the first 1–3 years to allow natives to establish, then control of woody weeds until canopy closure. The need for sympathetic post-application management was also highlighted by Phillips (1996) in a review of 11 Australian mines and quarries using biosolids for revegetation.
Native plants establishing from seeds are particularly vulnerable to competition. Langer et al. (1999) reported native karamu, koromiko (Hebe salicifolia) and mānuka seed could be established by broadcasting, and seedlings that survived the first winter responded strongly to fertilizer (N and P). However, the fertilizer also stimulated the growth of competitive herbaceous species such as Yorkshire fog (Holcus lantanus), lotus (Lotus corniculatus), and Himalayan honeysuckle (Leycesteria formosa) and plants that then smothered the natives. In some cases pasture grasses may be deliberately encouraged to suppress taller, more competitive weeds (as “green manure or green mulch”). They concluded native seeding only had potential as a revegetation technique for sites with low weed competition. Intense weed competition from pasture species was also reported by Douglas et al. (2007) in their research attempting seeding of native species into farmland.
Competition from non-native species can be controlled by manipulating the timing and/or placement of biowastes or native plants, or by weed removal using herbicides or physical methods. Biowaste mulches are effective at control competition between planted native seedlings and common light-demanding weeds. Benefits depends on the depth, texture and rate of decomposition of the mulch, the characteristics of weed species and the drainage status of the soils. Balasubramaniamm et al. (1998) reported a range of mulches effectively suppressed weeds in NZ vineyards; Pratt (1999) reported bark was better at suppressing weeds over 2 years than shorter-lived mulches. Where native forest or shrubland is a target, many organic mulches can also enhance conditions for natural seed regeneration under the forest canopy once decomposed, thereby supporting longer-term ecological succession.
Mycorrhizae
Most NZ native plants form symbiotic associations with Arbuscular mycorrhizal fungi (AMF) (Williams, 2011) which enable them to access N and P directly from organic sources and can play an essential role in water and nutrient uptake (Davis et al., 2013). Although NZ has only four woody plant genera known to form ecto-mycorrhizal (ECM) associations in the field (Orlovich and Cairney, 2004), these are critical seral species (Leptospermum scoparium and Kunzea spp.) and forest canopy components (southern beech, Nothofagus spp., Lophonzonia sp.). In contrast, the ECM fungal diversity is high; for example, there are over 200 species that are likely linked with southern beech species (McKenzie et al., 2000). Mycorrhizas are essential for P uptake and growth of manuka and southern rata (Metrosideros umbellata) seedlings in soils where low P-status limits growth (Hall, 1977). Pot trials growing southern beech in soils with low available P showed seedlings that developed mycorrhizas grew more rapidly than those that did not (Johnston et al., 2003). Some ecto-mycorrhizae protect plants from root pathogens (Johnston et al., 2003) either through forming a dense, compact mantle (Peterson et al., 2004) or by producing antifungal compounds (Duchesne et al., 1989). They may also enhance plant resistance to climatic stresses like drought by enlarging surface area explored for water or moderating impacts of pollutants (Read, 2002). Lack of suitable ECM fungi appears to limit spread of southern beech from forest margins (Wardle, 1991), and ECM fungi also facilitate invasions of NZ native ecosystems by exotic species, for example Pinus contorta into tussock ecosystems and (Douglas fir) Pseudotsuga meziesii into southern beech forests (Orlovich and Cairney, 2004).
The response of mycorrhizae to biowastes is influenced by the concentration and type of nutrients supplied. For example, moderate amounts of N fertilizer enhanced vesicular—arbuscular mycorrhizal infection in a low-P status soil, whereas applying P fertilizer reduced infection Hall (1977). Mycorrhizae are generally suppressed in nutrient-rich conditions. Hence, Waterhouse (2014) reported that unweathered municipal biosolids reduced AMF colonization of L. scoparium, whereas trials using vermi-composted, stabilized biowastes with lower available N and P had no significant effect on Ecto- or Arbuscular mycorrhizae (Xue et al., 2016). Further, Last et al. (1987) suggest ecto-mycorrhizal species adapted to soils with low OM contents are unlikely to be competitive in soils with high levels of carbon.
Native Soil Organisms
Degraded soils typically have depleted soil fauna, due to lower OM contents, lower OM inputs (stressed plant growth), and degraded physical conditions. For example, earthworm numbers in rehabilitated lignite mine soils were initially <10% of the population of undisturbed soils (Widdowson and McQueeb, 1990). In New Zealand, even agricultural soils that are not degraded are profoundly modified from their natural state with elevated soil N and depleted native soil invertebrate fauna (Kim et al., 2017). Hence, invertebrates of leaf litter and native earthworms have been proposed as useful indicators of successful forest restoration in New Zealand (Smith et al., 2016).
New Zealand has a diverse, distinctive group of over 179 species of endemic earthworms (Kim et al., 2017) that are also important food for native animals such as Powelliphanta spp. snails and kiwi (Apteryx spp.) birds. Some native earthworms are highly tolerant to acidic conditions (down to ca pH 4, Boyer and Wratten, 2010). However, these earthworms are intolerant of soluble N, as are non-native earthworms, for example, a 200 kg/ha split-application of urea fertilizer reduced non-native worm numbers by up to 40% on rehabilitated soils (Widdowson and McQueeb, 1990). The impact of biowaste therefore depends on chemical contaminants in the biowaste (such as ammonia or trace elements), baseline soil limitations (organic matter, contaminants), and the degree to which these are alleviated by a specific biowaste. For example, mortality of native earthworms over 3 months incubation was reduced from 42% to zero when acidic topsoils were amended with municipal biosolids at 1:4 ratio (Waterhouse et al., 2014). In a similar incubation experiment using aged biosolids and two native Maoridrilus species, mortality of 40% in an unamended soil was considered due to lack of OM (food), and all worms died in 100% biosolids treatment (Kim et al., 2017). However, adding suitable types and rates of biowaste to soils can increase OM contents and improve soil physical conditions both directly, and indirectly, through benefitting earthworms that in turn enhance soil physical and chemical fertility (Ross and Widdowson, 1985; Boyer and Wratten, 2010; Kim et al., 2017).
Critical Factors That Determine Successful Use of Biowastes for NZ Plants and Ecosystems
To date, most NZ biowaste research has aimed to identify either the maximum rate of biowaste that can be applied with acceptable environmental impacts (to minimize land required for disposal) or the amount of biowaste needed to achieve a production outcome equivalent to inorganic fertilizer application (Sharp, 1992). These approaches are applicable to situations where native plants are being established for production, carbon sequestration or microbiological attenuation purposes. However, there is also value in selecting biowastes types and rates to achieve specific, beneficial ecosystem services, production and ecological rehabilitation outcomes that enhance the establishment, resilience and succession of native ecosystems.
Four critical factors need to be considered when selecting a biowaste or biowastes and method/s of application to achieve the selected native NZ plant and ecosystem outcomes:
• Weed competition;
• Ecosystem succession;
• Resilience (especially to drought and storms); and,
• Soil microbiome responses to elevated N, P, OM and contaminants.
These factors are summarized in Figure 1, and should be considered in the selection of the specific biowastes, timing and methods of biowaste application, the selection of plant species and target ecosystems, and the post-application management of receiving sites. The three latter factors are most important where an aim of biowaste use is ecosystem rehabilitation and less important where native plants are used for carbon sequestration or production (other than for food).
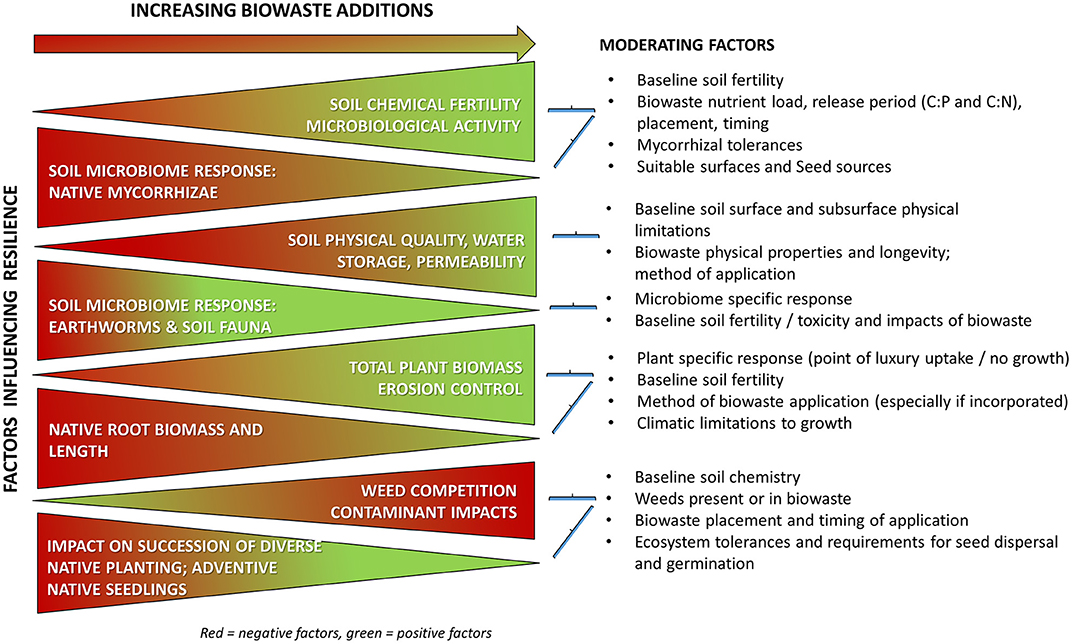
Figure 1. Summary of generalized effects of biowastes on main factors that determine success of restoration/native ecosystem restoration in New Zealand. The review discusses the evidence and moderating factors (right hand side) that influence the extent and type of response. Resilience is critical and influenced by all these factors.
Weed Competition
Control of herbaceous weeds is critical to allow native establishment in situations where biowastes stimulate plant growth, for example by overcoming nutrient limitations. Biowaste application rates at these sites should consider the availability (form) of N and P applied, and not be based on total N or P; the latter is typically limited to 200 kg N/ha /annum, while available P is strongly influenced by soil mineralogy. This approach may allow higher rates of low-concentration biowastes to be applied and encourage composting, vermi-composting or co-disposal of more concentrated biowastes with high C:N material (sawdust, arborist mulch or shredded bark). This is particularly valuable where soils are degraded and large volumes of biowastes (100–400 dry t/ha) are needed to enhance soil moisture holding capacity and soil organic contents.
When natives are established with, or into, non-native pastures, herbicides are commonly used to create bare planting areas and to kill weeds that compete with native plants. An alternative or complementary approach is to use biowastes as a mulch (Dollery et al., 2019) to gain added benefits of soil moisture conservation (if applied to sufficient depth and when soils are moist) and surface temperature moderation. Mulches can be selected to create a leaf-litter layer and favorable seed bed following decomposition. Weed competition can also be managed using a temporary “green-mulch” of non-native grasses or cereals; these have the added advantage of high N uptake to quickly sequester N into plant biomass and rapid stabilization of erosion-prone surfaces. Native planting can then be delayed until available N levels decrease to levels at which native plants are more competitive and the green mulch has died-off (if annual species), is less competitive, or is killed.
Ecosystem Succession
Consideration of succession pathways is a critical factor where biowastes are used for native ecosystem rehabilitation, because many NZ plants are long lived (hundreds of years) and rehabilitation usually focuses on just establishing common seral plants (Dickinson et al., 2015; Simcock and Ross, 2017). Coarse biowastes such as logs and stumps have a unique role in facilitating succession as decomposing logs provide a substrate onto which seedlings can establish that cannot compete on the forest floor (Wardle, 1991). Such biowastes and finer organic mulches can also create sheltered, humid, and more stable microclimates in the initial establishment phases. Together, surrogate humus layers and coarse wood also provide habitats for a diverse range of native, endemic invertebrates otherwise absent from newly planted sites for decades. Branches and logs can also be used to physically protect plants from mammal browse, allowing establishment of palatable species (Forbes, 2015).
Resilience
Biowastes usually supply one or more primary benefits for plant growth, delivered by enhanced available N and P. However, because a positive height and/or biomass growth response in native species is usually linked to a lower shoot: root ratio (Waterhouse et al., 2014; Seyedalikhani et al., 2019), biowaste applications that stimulate a large above-ground growth are likely to reduce resilience on native plants to drought and windthrow (storms). Vulnerability to drought is increased where biowastes encourage development of a near-surface root mat, for example by regular surface application of wastewater (Sims et al., 1994) or use of surface mulches with fertilizer (Pratt, 1999). However, organic biowaste mulches are commonly used to enhance native seedling resilience to drought during establishment by reducing water loss directly (by reducing evaporation from soil) and indirectly (by suppressing growth of competing weeds). Mulches also enhance resilience when they overcome soil surface physical limitations, thereby reducing runoff and enhancing recharge of soil moisture by rainfall (Stewart et al., 1992). Organic mulches and compost amendments are also widely used to increase resilience of earthworked-slopes against erosion, both directly (by providing a physical cover and reducing runoff) and indirectly, by enhancing mechanical reinforcement of soil by roots (Donn et al., 2015).
Coarse mulches including logs and stumps also create a rough, erosion resistant topography with stable, sheltered microsites (Simcock and Ross, 2017). At exposed sites, the reducing wind exposure by creating a rough hummocky surface had a greater positive impact on growth than applying fertilizer for the woody koromiko (Hebe salicifolia) and mountain beech (Fuscospora cliffortioides), but not for the more wind-tolerant monocotyledons flax (Phormium spp.) and toetoe (Austroderia spp.) (Theinhardt, 2003). Thus, spreading such coarse woody material over soils amended with nutrient-rich biosolids could enable the full potential growth response of native plants at degraded or highly exposed sites. Other NZ mine-rehabilitation research consistently shows such provision of an erosion-resistant root zone underpins native plant survival and growth (Simcock and Ross, 2017).
Methods of biowaste application that involve cultivation can benefit plant resilience in physically-degraded soils, by increasing rooting depth and volume (Donn et al., 2015). Such considerations are increasingly important in a future of climate change and where biowaste additions are large enough, or initial soil conditions poor enough, to substantially change soil chemical fertility, plant-available water storage, and/or root volumes. Biowaste applications, especially large, one-off applications of vermi-composted or similar biowaste with relatively slow nutrient release profile appear best-suited for increasing native plant establishment where soils are chemically and/or physically degraded. This may reduce the risks associated with higher above-ground biomass and reduced below-ground roots. Where wastewaters are irrigated, plant resilience can be enhanced by manipulating frequency and depth to minimize surface rooting, overcome the severity of soil seasonal moisture deficit overcome, and/or avoid inducing anaerobic soil conditions.
Soil Microbiome Response
The final critical success factor that influences the successful use of biowastes with NZ plants and ecosystems is soil microbiome responses to elevated HM and contaminants (including biological contaminants). There is little data on which to set concentration limits for contaminants such as HMs or EOC where the primary goal of biowaste amendment is ecosystem rehabilitation. Once added to soil, some contaminants are difficult to remove. Long-term NZ research on 3-yearly applications of municipal sludge to a sandy Raw Soil, with 900 mm rainfall and 2–4 m depth groundwater, concluded biowastes improved soil carbon levels, site fertility (N and P), and site productivity, without causing significant adverse impact on the receiving environment (Wang et al., 2004; Xue et al., 2015). Suitable ecosystems for higher-nutrient biosolids include those naturally adapted to high inputs of guano, such as those adjacent to seabird colonies. Such systems would provide a baseline to compare effects of enhanced foliar nutrition on palatability of plants to insects, other browsers or disease; factors that have received little attention.
New Zealand ecosystems that are adapted to low available N and P are likely to be transformed by biowaste applications that elevate the availability of these nutrients. Because biowastes contain organic-bound N and P, the duration during which nutrients such as P, K, Mg, and Ca are available for plant growth is extended and potential for N leaching is reduced (Horrocks et al., 2013; Esperschuetz et al., 2017a). Partly-stabilized biowastes, such as vermi-composts with moderate levels of available N and P, and large proportions of slowly-mineralisable N and P, also offer solutions as they are more likely to match release to native plant demand, which is lower than for arable crops or plantation forestry. In these biosolids, the surge of microbial activity is completed, and OM metabolized aerobically. This not only reduces the nutrient pulse compared with raw (anoxic/anaerobic) sludges, but also reduces the risks associated with HM leaching.
While a spike in available N may be reduced by adding carbon-rich biowastes, and by leaching and harvesting plant biomass, there is little potential for P-sensitive ecosystems to recover to natural levels of available P. An exception may be soils with high anion retention that could be cultivated. Elevated P is likely to have long-term effects on soil mycorrhizae, and there is little understanding of NZ native mycorrhizal relationships in native ecosystems. Elevated available P facilitates the establishment of a range of aggressive, N-fixing weed species. Together, these vulnerabilities mean some low-fertility native ecosystems are not suitable targets for rehabilitation or for the application of nutrient-rich biowastes or for the long-term application of low-concentration biowastes (e.g., wastewaters).
Some emerging contaminants in municipal biowastes, such as anti-depressants, have been shown to have individual and cumulative impacts on terrestrial ecosystem components in European and North American studies (Whitlock et al., 2018). No equivalent studies on NZ species have been carried out using NZ-relevant contaminants and concentrations. Last, some biowastes are a potential source of non-native plants, animals and micro-organisms, especially where wastes contain viable propagules or are stored before transport in contact with soil or in the open; municipal sludges and composts have transferred tomatoes, violets, and non-native earthworms between sites.
At a fine scale, heterogeneous application methods that take advantage of the scavenging ability of some native plants may be method to retain or enhance a diverse soil microbiome. Methods to create variation in rooting environments can include physical treatments and placement of different biowastes. At a larger scale, the underlying importance of such habitat heterogeneity to support biodiversity heterogeneity is emphasized internationally, but is only beginning to be implemented in New Zealand rehabilitation. Enhancing heterogeneity may also enhance resilience to stresses, including long-term climatic change and short-term weed pressures—a critical factor for successful native restoration.
Conclusions and Research Recommendations
Biowastes have important physicochemical- and ecological- roles for the rehabilitation of native NZ ecosystems. Eighty million years of isolation in the absence of mammals (except bats and seals) in NZ has resulted in high rates of endemism for plants (80%), birds (71%), insects (80%), and fungi (50%), along with complex inter-relationships. Most NZ native tree and shrub species have symbiotic mycorrhizal associations—an adaptation to soils with low available N and P, but relatively high soil OM; native plants are therefore commonly stress-tolerators (Grime, 2001). To facilitate increased beneficial use of biowaste for re-establishing these unique ecosystems, it is critical to characterize each biowaste and the receiving soil using both total element concentrations and biologically “available” concentrations. The key biosolids parameters that influence plant and soil animal responses are the concentration of soluble (plant-available) N, P, and toxic materials, especially HMs if soil pH favors HMs in soil solution. Phyto-toxicity is mediated by pH and surface area, the latter is in turn influenced by OM and clay mineralogy. The nature of the biowaste also informs the application method that can be used to maximize specific benefits. For example, “pre-shocking” small areas to develop a microbiome able to process and respond to biowastes (especially HMs, N, and EOCs), may help to improve resilience while conserving “native” microbiomes. Similarly, heterogeneous application methods, which take advantage of the scavenging ability of some native plants, may be another way to retain a diverse microbiome.
Biowastes may increase the establishment and growth of native species grown directly for timber, oils, and fiber by overcoming nutrient or water limitations, or indirectly, by suppressing competition. Biowastes that enhance readily plant-available N and P typically increase above-ground plant biomass, but the effects are moderated by initial soil conditions; in soils that are not degraded, native species may take up luxury amounts of nutrients but not increase above-ground biomass. Because below-ground (root) biomass is typically unchanged, root: shoot ratios decrease. Biowastes generally enhance soil carbon sequestration rates, water-holding capacity and macro-porosity if applied in large enough amounts and/or where soils initially have low organic contents. Such outcomes are enhanced by biowastes with high proportions of stable carbon (i.e., composted or vermi-composted materials) and/or mixing into soil. Benefits from any biowaste are likely to be greatest where root zones have depleted OM, weak structure, have formed crusts, low cation exchange and/or low available N and P as long as soil aeration is maintained at levels that meet the requirements of the selected plant/ecosystem.
Biowastes may also be applied in ways that overcome specific adverse effects and accentuate specific benefits; for example, tree waste can be incorporated into soil as compost to improve nutrient supply, and break surface crusts, and/or applied as a surface mulch to reduce water loss and suppress competing plants in the short term, and/or used as logs (i.e., unmulched) to create sheltered microhabitats. While numerous NZ studies describe these physicochemical benefits, there is little information on the ecological effects resulting from increased fertility or the impact of withholding wastewaters or sludges when sites reach such levels of N, P, or contaminants that applications cease.
This study identified the following critical factors that influence the successful use of biowastes with NZ plants and ecosystems:
• Weed competition—manipulating the application (rate, nutrient load, timing and/or placement) of biowastes to optimize nutrient supply and limit weed growth. This can include using mulches to control competition between planted native seedlings and common, light-demanding weeds;
• Resilience (especially to drought and storms)—surface application of biowastes can help conserve moisture by increasing infiltration, reducing runoff and reducing evapotranspiration. However, if surface-rooting is encouraged and/or root: shoot ratio is decreased (i.e., larger above-ground biomass) resilience of native plants to stresses such as drought is reduced, particularly if irregular irrigation is disrupted;
• Ecosystem succession—species dominance and succession can be manipulated by the availability of nutrients applied through biowastes as species that respond with faster growth rates (rather than luxury uptake into leaves) are advantaged by higher nutrient levels. Succession can also be manipulated by using biowastes such as logs, which provide stable, sheltered sites in the short term and medium-term germination sites. Such coarse wood can also be used to physically exclude browsing mammals that otherwise prevent establishment of palatable plant species; and,
• Soil microbiome responses to elevated N, P, HM, and contaminants (including biological contaminants)—some native plant- and ecosystem-specific mycorrhizal associations can be inhibited by elevated nutrients (either, or both, N and P). Similarly, contaminants such as HMs or EOC in certain biowastes can negatively impact the microbiome.
These factors, and the characteristics of the different biowastes, should be considered in the selection, timing and methods of biowaste application, the selection of plant species and target ecosystems, and the post-application management of receiving sites. Field trials are needed to test techniques developed in glasshouse trials that reduce nutrient pulses and leaching losses from biowastes. These will facilitate high-enough, one-off (basal) applications to deliver soil physical benefits. Vermi-composted biowastes have potential in this area. Field confirmation of laboratory trials indicating if oil, wood and fiber quality is maintained under high biowaste application rates are also needed. Where possible, trials should be maintained long enough to capture OM transformations, as OM degrades and ecosystem changes as plant succession occurs.
New Zealand has the potential to move beyond a “biowaste disposal” approach and, instead, select biowastes to achieve specific, beneficial ecosystem services. To achieve this requires research both on factors that influence development of whole-ecosystem rehabilitation where NZ-natives are planted, and research on how, when and where different biowastes can be applied to enhance ecosystem rehabilitation. Target ecosystems should be identified and explored. While rehabilitation of many NZ ecosystem may require a careful balance with nutrient loads provided by biowastes to manage weed competition, rehabilitation of some ecosystems may be achieved with higher-nutrient biosolids. For example, naturally fertile forests associated with seabird colonies that were present pre-European/Maori throughout NZ but are now rare. Use of biowastes for ecosystem rehabilitation also requires greater understanding of the tolerances and vulnerabilities of both native invertebrates and mycorrhizae to combinations of nutrients, HM, biocides, and EOCs. This includes understanding effects (including of mixtures), the concentrations at which they occur and investigating biowaste combinations and application methods that mitigate adverse effects.
Author Contributions
All authors listed have made a substantial, direct and intellectual contribution to the work, and approved it for publication.
Funding
The project was conducted as part of the Center of Integrated Biowaste Research with financial support provided by the Institute of Environment Science and Research Ltd. through the Strategic Science Investment Fund from the Ministry of Business, Innovation and Employment, New Zealand (Grant: C03X1701).
Conflict of Interest
The authors declare that the research was conducted in the absence of any commercial or financial relationships that could be construed as a potential conflict of interest.
Supplementary Material
The Supplementary Material for this article can be found online at: https://www.frontiersin.org/articles/10.3389/fsufs.2019.00085/full#supplementary-material
Footnotes
1. ^https://www.aucklandcouncil.govt.nz/mayor-of-auckland/mayor-priorities/protecting-our-environment/Pages/million-trees.aspx
2. ^https://www.mpi.govt.nz/funding-and-programmes/forestry/planting-one-billion-trees/
3. ^http://www.fedfarm.org.nz/FFPublic/Policy2/National/Good_Farming_Practice-Action_Plan_for_Water_Quality_2018.aspx.
4. ^https://www.landcareresearch.co.nz/resources/data/nga-tipu-whakaoranga-maori-plant-use-database.
References
Adams, J. A., Warren, H. H., and Cameron, K. C. (1992). “Potential nitrogen and phosphorus leaching from a sandy soil under Pinus radiata following amendment with municipal sewage sludge,” in The Use of Wastes and Byproducts as Fertilisers and Soil Amendments for Pasture and Crops, eds P. E. H. Gregg and L. D. Currie. Occasional Report No. 6 (Palmerston North: Fertilizer and Lime Research Centre, Massey University, 69–90.
ANZBP (2017). New Zealand Biosolids Statistics. Australian and New Zealand Biosolids Partnership. Available online at: https://www.biosolids.com.au/guidelines/new-zealand-biosolids-statistics/ (accessed December 11, 2017).
Ataria, J., Baker, V., Goven, J., Langer, E. R., Leckie, A., Ross, M., et al. (2016). From Tapu to Noa - Maori Cultural Views on Biowastes Management: A Focus on Biosolids. Centre for Integrated biowaste Research Report. Available online at: http://www.cibr.org.nz/assets/Uploads/Newsletter-Thumb/CIBR-From-Tapu-to-Noa.pdf (accessed September 24, 2019).
Atkinson, D. S., van der Mespel, G. J., and Popay, A. I. (1992). “Effectiveness of whey for controlling grass grub,” in The Use of Wastes and Byproducts as Fertilisers and Soil Amendments for Pastures and Crops, eds P. E. H. Gregg and L. D. Currie. Occasional Report No. 6 (Palmerston North: Fertiliser and Lime Research Centre, Massey University).
Balasubramaniamm, R., Scott, K., Agnew, R., and McDonald, A. (1998). “Options for waste stream utilisation for sustainable viticulture,” in Proceedings of the Technical Session No 17, eds H. Wang and J. M. Carnus (Blenheim: NZ Land Treatment Collective).
Bedbabis, S., Ben Rouina, B., Boukhris, M., and Ferrara, G. (2014). Effect of irrigation with treated wastewater on soil chemical properties and infiltration rate. J. Environ. Manag. 133, 45–50. doi: 10.1016/j.jenvman.2013.11.007
Boyer, S., and Wratten, S. D. (2010). The potential of earthworms to restore ecosystem services after opencast mining – a review. Basic Appl. Ecol. 11, 196–203. doi: 10.1016/j.baae.2009.12.005
Clinton, P., and Leckie, A. (2002). “The fate of N in municipal biosolids applied to central South Island radiata pine plantations,” in New Zealand Land Treatment Collective: Proceedings for the 2002 Annual Conference, eds. H. Wang and J. Lavery (Whangamata).
Correa, R. S., White, R. E., and Weatherley, A. J. (2006). Risk of nitrate leaching from two soils amended with biosolids. Water Resour. 33, 453–462. doi: 10.1134/S0097807806040117
Creasy, G. L., LealPerez, G., Crawford, M., Ibbotson, L., Tompkins, J., Creasy, K., et al. (2007). Influence of Natural Reflective Mulch on Pinot Noir Grape and Wine Quality. Sustainable Farming Fund Report Project 03/110. Centre for Viticulture and Oenology, Lincoln University.
Davis, M., Dickie, I., Paul, T., and Carswell, F. (2013). Is Kanuka and Manuka establishment in grassland constrained by mycorrhizal abundance? N. Zeal. J. Ecol. 37, 172–177. Available online at: https://newzealandecology.org/nzje/3084
Davis, M. R., and Langer, E. R. (1997). “Giles Creek Fertiliser Response of Coprosma robusta and Nothofagus fusca seedlings. Rehabilitation of indigenous forest after mining, West Coast,” in Science for Conservation 54 (Wellington: Department of Conservation), 38–47.
Depree, C. (2008). Contaminant Characterisation and Toxicity of Road Sweepings and Catchpit Sediments: Towards More Sustainable Reuse Options. Land Transport NZ Research Report 345. Wellington: Land Transport New Zealand, 114.
Dickinson, N., Marmiroli, M., Das, B., McLaughlin, D., Leung, D., and Robinson, B. (2015). Endemic plants as browse crops in agricultural landscapes of New Zealand. Agroecol. Sust. Food Syst. 39, 224–242. doi: 10.1080/21683565.2014.967438
Dixon, T., Elmsly, T. A., Fields, F. P., Smith, D. B., Mandemaker, A. J., Greenwood, A. G., et al. (2007). “What, when, where and how much mulch should be applied to ‘Hass’ avocado trees in the western Bay of Plenty,” in Annual Research Report, Vol. 7 (New Zealand Avocado Growers' Association Annual Research Report), 49–72. Available online at: http://www.avocadosource.com/Journals/NZAGA/NZAGA_2007/NZAGA_2007_06.pdf (accessed September 24, 2019).
Dollery, R. (2017). Ecological Restoration of Dryland Kānuka Communities in an Irrigated Agricultural Landscape. Doctor of Philosophy, Lincoln University.
Dollery, R., Li, S., and Dickinson, N. M. (2019). Nutrient-enriched soils and native N-fixing plants in New Zealand. J. Plant Nutr. Soil Sci. 182, 104–110. doi: 10.1002/jpln.201800482
Donn, S., Wheatley, R. E., McKenzie, B. M., Loades, K. W., and Hallett, P. (2015). Improved soil fertility from compost amendment increases root growth and reinforcement of surface soil on slopes. Ecol. Eng. 71, 458–465. doi: 10.1016/j.ecoleng.2014.07.066
Douglas, G. B., Dodd, M. B., and Power, I. L. (2007). Potential of direct seeding for establishing native plants into pastoral land in New Zealand. N. Zeal. Journal of Ecology 31, 143–153. Available online at: https://newzealandecology.org/nzje/2831
Drewry, J., Currane-Cournane, F., Taylor, M., Gray, C., and McDowell, R. (2013). “Olsen P methods and soil quality monitoring: are we comparing ‘apples with apples’?,” in Fertiliser and Lime Research Centre Annual Workshop. Occasional Report No. 6 (Palmerston North: Massey University).
Duchesne, L. C., Ellis, B. E., and Peterson, R. L. (1989). Disease suppression by the ecto-mycorrhizal fungus Paxillus involutus: contribution of oxalic acid. Can. J. Bot. 67, 2726–2730. doi: 10.1139/b89-351
Esperschuetz, J., Anderson, C., Bulman, S., Katamian, O., Horswell, J., Dickinson, N. M., et al. (2017a). Response of Leptospermum scoparium, Kunzea robusta and Pinus radiata to contrasting biowastes. Sci. Total Environ. 587–588, 258–265. doi: 10.1016/j.scitotenv.2017.02.134
Esperschuetz, J., Anderson, C., Bulman, S., Lense, O., Horswell, J., Dickinson, N., et al. (2016). Production of biomass crops using biowastes on low-fertility soil: 1. influence of biowastes on plant and soil quality. J. Environ. Qual. 45, 1960–1969. doi: 10.2134/jeq2015.12.0596
Esperschuetz, J., Balaine, N., Clough, T., Bulman, S., Dickinson, N. M., Horswell, J., et al. (2017b). The potential of L. scoparium, K. robusta and P. radiata to mitigate N-losses in silvopastural systems. Environ. Poll. 225, 12–19. doi: 10.1016/j.envpol.2017.03.042
Feitje, G., and Petrie, R. (1992). “From fish, forestry and freezing works to a fertile soil amendment,” in The Use of Wastes and Byproducts as Fertilisers and Soil Amendments for Pastures and Crops, eds P. E. H. Gregg and L. D. Currie. Occasional Report No. 6 (Palmerston North: Fertiliser and Lime Research Centre, Massey University).
Feitjie, G., and Popay, A. I. (1992). “From forest and freezing works to fertiliser,” in The Use of Wastes and Byproducts as Fertilisers and Soil Amendments for Pastures and Crops, eds P. E. H. Gregg and L. D. Currie. Occasional Report No. 6 (Palmerston North: Fertiliser and Lime Research Centre, Massey University).
Floate, M. J. S., and Enright, P. D. (1992). “Preliminary studies on the availability and use of organic wastes for the restoration of depleted soils in Central Otago,” in The Use of Wastes and Byproducts as Fertilisers and Soil Amendments for Pastures and Crops, eds P. E. H. Gregg and L. D. Currie. Occasional Report No. 6 (Palmerston North: Fertiliser and Lime Research Centre, Massey University).
Forbes, A. S. (2015). Non-harvest Pinus radiata plantations for forest restoration in New Zealand (Ph.D. thesis). New Zealand School of Forestry, College of Engineering, University of Canterbury.
Franklin, H. M., Dickinson, N. M., Esnault, C. J. D., and Robinson, B. H. (2015). Native plants and nitrogen in agricultural landscapes of New Zealand. Plant Soil 394, 407–420. doi: 10.1007/s11104-015-2622-2
Grime, J. P. (2001). Plant Strategies, Vegetation Processes, and Ecosystem Properties. Chichester: John Wiley and Sons.
Güereca, L. P., Gass,ó, S., Baldasano, J. M., and Jiménez-Guerrero, P. (2006). Life cycle assessment of two biowaste management systems for Barcelona, Spain. Resour. Conserv. Recycl. 49, 32–48. doi: 10.1016/j.resconrec.2006.03.009
Guo, J., Wang, W., Liu, X., Lian, S., and Zheng, L. (2014). Effects of thermal pre-treatment on anaerobic co-digestion of municipal biowastes at high organic loading rate. Chemosphere 101, 66–70. doi: 10.1016/j.chemosphere.2013.12.007
Gutiérrez-Ginés, M. J., Madejón, E., Lehto, N. J., McLenaghen, R. D., Horswell, J., Dickinson, N., et al. (2019). Response of a Pioneering Species (Leptospermum scoparium J. R.Forst. and G.Forst.) to heterogeneity in a low-fertility soil. Front. Plant Sci. 10:93. doi: 10.3389/fpls.2019.00093
Gutiérrez-Ginés, M. J., McIntyre, C., Lense, O., Mishra, M., Seyedalikhani, S., McLenaghen, R., et al. (2017a). A Lysimeter Experiment and Field Trial to Determine Options for the Beneficial Reuse of Wastewater From Duvauchelle and Akaroa, Banks Peninsula. Final Report ed. Lincoln University.
Gutiérrez-Ginés, M. J., Robinson, B. H., Esperschütz, J., Madejon, E., McLenaghen, R. D., and Horswell, J. (2017b). Potential use of biosolids to reforest degraded areas with New Zealand native vegetation. J. Environ. Qual. 46, 906–914. doi: 10.2134/jeq2017.04.0139
Hall, I. R. (1977). Effect of applied nutrients and endomycorrhizas on Metrosideros umbellata and Leptospermum scoparium. N. Zeal. J. Bot. 15, 481–484. doi: 10.1080/0028825X.1977.10432553
Hart, P. B. S., and Speir, T. (1992). “Agricultural and industrial effluent and wastes as fertilizers and soil amendments on New Zealand,” in The Use of Wastes and Byproducts as Fertilisers and Soil Amendments for Pasture and Crops, eds P. E. H. Gregg and L. D. Currie. Occasional Report No. 6 (Palmerston North: Fertilizer and Lime Research Centre, Massey University, 69–90.
Haynes, R. J., and Naidu, R. (1998). Influence of lime, fertilizer and manure applications on soil organic matter content and soil physical conditions: a review. Nutr. Cycl. Agroecosyst. 51, 123–137. doi: 10.1023/A:1009738307837
Horrocks, A. J., Tregurtha, C. S., Maley, S., and Meenken, E. D. (2013). “Compost SFF Final Report (2009–12),” in PFR SPTS (Lincoln: Plant and Food Research).
Iversen, C. M., Bridgham, S. D., and Kellogg, L. E. (2010). Scaling plant nitrogen use and uptake efficiencies in response to nutrient addition in peatlands. Ecology 91, 693–707. doi: 10.1890/09-0064.1
Johnston, P. R., Horner, I. J., and Beever, R. (2003). “Phytophthora cinnamomi in New Zealand's indigenous forests,” in ‘Phytophthora in Forests and Natural Ecosystems’. 2nd International IUFRO Working Party 7.02.09 Meeting, eds J. A. McComb, G. E. Hardy, and I. C. Tommerup (Albany, WA: Murdoch University Print, Perth).
Kim, Y. N., Robinson, B., Horswell, J., Boyer, S., and Dickinson, N. (2017). Impacts of endemic Maoridrilus Earthworms (Megascolecidae) in biosolids-amended soil. J. Environ. Qual. 46, 177–184. doi: 10.2134/jeq2016.06.0207
Knowles, O. A., Robinson, B. H., Contangelo, A., and Clucas, L. (2011). Biochar for the mitigation of nitrate leaching from soil amended with biosolids. Sci. Total Environ. 409, 3206–3210. doi: 10.1016/j.scitotenv.2011.05.011
Langer, E. R., Davis, M. R., and Ross, C. W. (1999). “Rehabilitation of lowland indigenous forest after mining in Westland,” in Science for Conservation. Wellington: Department of Conservation.
Last, F. T., Dighton, J., and Mason, P. A. (1987). Successions of sheathing mycorrhizal fungi. Trends Ecol. Evol. 2, 157–161. doi: 10.1016/0169-5347(87)90066-8
Luo, J., and Lindsey, S. (2001). “Irrigation of meat processing wastewater onto land,” in NZ Land Treatment Collective. 2001 Annual Conference. Invercagill: Technical Session 22.
Mackay, A., Dominati, E., and Taylor, M. (2013). Soil Quality Indicators: The Next Generation. Palmerston North: AgReserach Ltd.
Maseyk, F., Dominati, E., and Mackay, A. (2019). More than a ‘nice to have’: integrating indigenous biodiversity into agroecosystems in New Zealand. N. Zeal. J. Ecol. 43, 1–12. doi: 10.20417/nzjecol.43.20
McKenzie, E. H. C., Buchanan, P. K., and Johnston, P. R. (2000). Checklist of fungi on Nothofagus in New Zealand. N. Zeal. J. Bot. 38, 635–720. doi: 10.1080/0028825X.2000.9512711
McLaren, R. G., and Cameron, K. C. (1996). Soil Science. Sustainable Production and Environmental Protection. Auckland: Oxford University Press.
McLaren, R. G., Clucas, L. M., Speir, T. M., and van Schaik, A. P. (2007). Distribution and movement of nutrients and metals in a Pinus radiata forest soil following application of biosolids. Environ. Poll. 147, 32–40. doi: 10.1016/j.envpol.2006.08.027
McWethy, D. B., Whitlock, C., Wilmshurst, J. M., McGlone, M. S., Fromont, M., Li, X., et al. (2010). Rapid landscape transformation in South Island New Zealand following initial Polynesian settlement. Proc. Natl. Acad. Sci. U.S.A. 107, 21343–21348. doi: 10.1073/pnas.1011801107
Ministry for the Environment (2000). New Zealand Biodiversity Strategy 2000-2020. New Zealand: Ministry for the Environment. Available online at: https://www.doc.govt.nz/nature/biodiversity/nz-biodiversity-strategy-and-action-plan/ (accessed September 24, 2019).
Ministry for the Environment and Statistics New Zealand (2015). Environment Aotearoa 2015: Data to 2013. New Zealand: Ministry for the Environment.
Mishra, M. (2018). Interactions between Escherichia coli and the New Zealand native plants Leptospermum scoparium and Kunzea robusta (Ph.D.), Lincoln Univeristy.
Morrell, W. J. (1997). An assessment of the revegetation potential of base-metal tailings from the Tui Mine, Te Aroha, New Zealand. Doctor of Philosophy in Soil Science, Massey University.
Muñoz, I., Gómez-Ramos, M. J., Agüera, A., Fernández-Alba, A. R., García-Reyes, J. F., and Molina-Díaz, A. (2009). Chemical evaluation of contaminants in wastewater effluents and the environmental risk of reusing effluents in agriculture. TrAC Trends Anal. Chem. 28, 676–694. doi: 10.1016/j.trac.2009.03.007
Norton, D. A., Creedy, S., and Keir, D. (2013). Substrate modification for enhanced native forest restoration, Reefton. Ecol. Manag. Restor. 14, 147–150. doi: 10.1111/emr.12038
Norton, D. A., and Reid, N. (2013). Nature and Farming: Sustaining Native Biodiversity in Agricultural Landscapes. Melbourne, VIC: CSIRO Publishing
O'Connor, M. B., Crush, J. R., and Longhurst, R. D. (2002). “Applying sewage wastes to agricultural land – benefits and some limitations to acceptance,” in New Zealand Land Treatment Collective: Proceedings for the 2002 Annual Conference, eds H. W. J. Lavery and N. Z. Whangamata.
Orlovich, D. A., and Cairney, J. W. G. (2004). Ecto-mycorrhizal fungi in New Zealand: current perspectives and future directions. N. Zeal. J. Bot. 42, 721–738. doi: 10.1080/0028825X.2004.9512926
Paavola, T., Syväsalo, E., and Rintala, J. (2006). Co-digestion of manure and biowaste according to the EC Animal By-Products Regulation and Finnish national regulations. Water Sci. Technol. 53, 223–231. doi: 10.2166/wst.2006.253
Paramashivam, D., Clough, T. J., Dickinson, N. M., Horswell, J., Lense, O., and Robinson, B. H. (2016). Effect of pine waste and biochar on nitrogen mobility in biosolids. J. Environ. Qual. 45:360–367. doi: 10.2134/jeq2015.06.0298
Paramashivam, D., Dickinson, N. M., Clough, T. J., Horswell, J., and Robinson, B. H. (2017). Potential environmental benefits from blending biosolids with other organic amendments before application to land. J. Environ. Qual. 46, 481–489. doi: 10.2134/jeq2016.10.0421
Pardy, G. F., Bergin, D. O., and Kimberley, M. (1992). “Survey of native tree plantation,” in FRI Bulletin 175 (Rotorua: Forest Research Institute), 24.
Parker, R. W. (2011). “Biosolids as a soil amendment for the rehabilitation of disturbed land,” in Winds of Change: Land Treatment in Challenging Times, Annual Conference, 23-25 March 2011: New Zealand Land Treatment Collective (Palmerston North).
Peters, M., and Clarkson, B. (2010). Wetland Restoration: a Handbook for New Zealand Freshwater Systems. Lincoln: Manaaki Whenua Press.
Peterson, R. L., Massicotte, H. B., and Melville, L. H. (2004). Mycorrhizas: Anatomy and Cell Biology. Ottawa, ON: NRC Research Press.
Phillips, C. (1996). A manual of Good Practice for the Use of Biosolids in Land Reclamation. Australia. Melbourne, VIC: Australian Minerals and Energy Environment Foundation Occasional Paper no. 5.
Pollock, K. M. (1986). “Plant materials handbook for soil conservation: Vol. 3, native plants,” in Water and Soil Miscellaneous Publication (Water and Soil Directorate, Ministry ofWorks and Development), ed C. W. VanKraayenoord (Wellington), 65.
Porteous, T. (1993). Native Forest Restoration: A Practical Guide for Landowners. Queen Elizabeth the Second National Trust. Available online at: https://qeiinationaltrust.org.nz/wp-content/uploads/2018/02/Native-Forests-Restoration.jpg.pdf (accessed September 24, 2019).
Pratt, C. (1999). Factors affecting the establishment, growth and survival of native woody plant communities on the Canterbury Plain (New Zealand Master of Science), Lincoln: Lincoln Univeristy.
Pritchard, D. L., Penney, N., McLaughlin, M. J., Rigby, H., and Schwarz, K. (2010). Land application of sewage sludge (biosolids) in Australia: risks to the environment and food crops. Water Sci. Technol. 62, 48–57. doi: 10.2166/wst.2010.274
Prosser, J. A., Woods, R. R., Horswell, J., and Robinson, B. H. (2016). The potential in-situ antimicrobial ability of Myrtaceae plant species on pathogens in soil. Soil Biol. Biochem. 96, 1–3. doi: 10.1016/j.soilbio.2015.12.007
Read, D. J. (2002). “Towards ecological relevance – progress and pitfalls in the path towards an understanding of mycorrhizal functions in nature,” in Mycorrhizal Ecology, eds M. G. A. van der Heijden and I. Sanders (Berlin: Springer), 3–29. doi: 10.1007/978-3-540-38364-2_1
Reis, F. V. P., Gutiérrez-Ginés, M. J., Smith, C. M. S., Lehto, N. J., and Robinson, B. H. (2017). Mānuka (Leptospermum scoparium) roots forage biosolids in low fertility soil. Environ. Exp. Bot. 133, 151–158. doi: 10.1016/j.envexpbot.2016.10.012
Roberts, A. H. C., O'Connor, M. B., and Longhurst, R. (1992). “Wastes as nutrient plant sources: issues and options,” in The Use of Wastes and Byproducts as Fertilisers and Soil Amendments for Pasture and Crops, eds P. E. H. Gregg and L. D. Currie. Occasional Report No. 6 (Palmerston North: Massey University).
Ross, C. W., and Crequer, D. (2006). “Land rehabilitation at Golden Cross – 8 years after mine closure,” in Australian Institute of Mining and Metallurgy New Zealand Branch Conference (Waihi).
Ross, C. W., and Widdowson, J. P. (1985). Restoration research after open-cast mining in Southland. N. Zeal. Soil News 33, 163–169
Sanchez, M. E., Otero, M., Gómez, X., and Morán, A. (2009). Thermogravimetric kinetic analysis of the combustion of biowastes. Renew. Energy 34, 1622–1627. doi: 10.1016/j.renene.2008.11.011
Seyedalikhani, S., Esperschuetz, J., Dickinson, N. M., Hofmann, R., Breitmeyer, J., Horswell, J., et al. (2019). Biowastes to augment the essential oil production of Leptospermum scoparium and Kunzea robusta in low-fertility soil. Plant Physiol. Biochem. 137, 213–221. doi: 10.1016/j.plaphy.2019.02.008
Sharp, R. W. (1992). “The benefits and problems of the beneficial reuse of sewage sludge in New Zealand,” in The Use of Wastes and Byproducts as Fertilisers and Soil Amendments for Pasture and Crops, eds P. E. H. Gregg and L. D. Currie. Occasional Report No. 6 (Palmerston North: Fertilizer and Lime Research Centre, Massey University).
Simcock, R., and Ross, C. W. (2017). “Mine rehabilitation in New Zealand: overview and case studies,” in Spoil to Soil: Mine Site Rehabilitation and Revegetation, eds N. S. Bolan, M. B. Kirkham, and Y. S. Ok. (Boca Raton, FL: CRC Press. Taylor and Francis Group), 335–364. doi: 10.1201/9781351247337-24
Simcock, R. C., and Xue, J. (2017). Biosolids Assist Mine Rehabilitation to Plantation Forestry. Putting Waste to Work: Centre for Integrated Biowaste Research newsletter 15, 4–5. Available online at: http://www.cibr.org.nz/assets/Uploads/May-2017-Issue-16.pdf (accessed September 24, 2019).
Sims, R. E. H., Hodson, R. W., and Watters, M. P. (1994). “Root production of short rotation coppice eucalyptus grown under an effluent disposal system,” in New Zealand Land Treatment Collective. Proceedings of the Technical Session No. 11, eds S. Pandey and J. M. Carnus (Massey University).
Smith, C. M. S., Bowie, M. H., Hahner, J. L., Boyer, S., Kim, Y. N., Zhong, H. T., et al. (2016). Punakaiki Coastal Restoration Project: A Case Study for a Consultative and Multidisciplinary Approach in Selecting Indicators of Restoration Success for a Sand Mining Closure site, West Coast, New Zealand. CATENA 136, 91–103. doi: 10.1016/j.catena.2015.07.024
Smith, C. T., McMahon, S. D., Devoe, D. R., and Bowden, W. B. (1992). “Silvicultural and environmental aspects of wastewater sludge utilisation,” in The Use of Wastes and Byproducts as Fertilisers and Soil Amendments for Pasture and Crops, eds P. E. H. Gregg and L. D. Currie. Occasional Report No. 6 (Palmerston North: Fertilizer and Lime Research Centre, Massey University).
Sparling, G., and Schipper, L. (2004). Soil quality monitoring in New Zealand: trends and issues arising from a board-scale survey. Agric. Ecosyst. Environ. 104, 545–552. doi: 10.1016/j.agee.2003.11.014
Speir, T. W., Ross, D. J., and Tate, K. R. (1987). “The effects of nutrientrich agricultural effluents on two New Zealand soils,” in 4th International CIEC Symposium Agricultural Waste Management and Environmental Protection: 1987 (Braunschweig), 11–14.
Speir, T. W., Van Schaik, A., Percival, H. J., Close, M. E., and Pang, L. (2003). Heavy metals in soil, plants and groundwater following high-rate sewage sludge application to land. Water Soil Air Poll. 150, 319–358. doi: 10.1023/A:1026101419961
Stevenson, B. A., and Smale, M. C. (2005). Seed bed treatment effects on vegetation and seedling establishment in a New Zealand pasture one year after seeding with native woody species. Ecol. Manag. Restorat. 6, 124–131. doi: 10.1111/j.1442-8903.2005.00229.x
Stewart, D. P. C., Cameron, K. C., and Cornforth, I. S. (1992). “Evaluation of spent mushroom compost as a soil amendment,” in The Use of Wastes and Byproducts as Fertilisers and Soil Amendments for Pastures and Crops, eds P. E. H. Gregg and L. D. Currie. Occasional Report No. 6 (Palmerston North: Fertiliser and Lime Research Centre, Massey University).
Sullivan, J. J., Williams, P. A., Timmins, S. M., and Smale, M. C. (2009). Distribution and spread of environmental weeds along New Zealand roadsides. N. Zeal. J. Ecol. 33, 190–204. Available online at: https://newzealandecology.org/nzje/2904
Theinhardt, N. I. (2003). Plant restoration at an open cast coal mine, West Coast, New Zealand. M. Sc. in Forestry University of Canterbury, Christchurch.
UN-Water (2015). Wastewater Management – A UN-Water Analytical Brief. UN-Water. Available online at: https://www.unwater.org/publications/wastewater-management-un-water-analytical-brief/ (accessed September 24, 2019).
Walker, S., Price, R., Rutledge, D., Stephens, R. T. T., and Lee, W. G. (2006). Recent loss of indigenous cover in New Zealand. N. Zeal. J. Ecol. 30, 169–177. Available online at: https://newzealandecology.org/nzje/2313
Wang, H., Brown, S. L., Magesan, G. N., Slade, A. H., Quintern, M., Clinton, P. W., et al. (2008). Technological options for the management of biosolids. Environ. Sci. Pollut. Res. Int. 15, 308–317. doi: 10.1007/s11356-008-0012-5
Wang, H., Magesan, G. N., Kimberley, M. O., Payn, T. W., Wilks, P. J., and Fisher, C. R. (2004). Environmental and nutritional responses of a Pinus radiata plantation to biosolids application. Plant Soil 267, 255–262. doi: 10.1007/s11104-005-0107-4
Waterhouse, B. R. (2014). Conserving New Zealand's endemic carnivorous land snail Powelliphanta patrikensis through restoration of a functioning ecosystem. (Ph.D. thesis), Lincoln University.
Waterhouse, B. R., Boyer, S., Adair, K. L., and Wratten, S. D. (2014). Using municipal biosolids in ecological restoration: what is good for plants and soil may not be good for endemic earthworms. Ecol. Eng. 70, 414–421. doi: 10.1016/j.ecoleng.2014.06.021
Weber, P., Wildy, J., Crombie, F., Olds, W., Rossiter, P., Pizey, M., et al. (2012). “Waste or resource: Solid Energy's beneficial use of waste streams,” in WasteMINZ Conference, 18 October 2012. Available online at: https://www.wasteminz.org.nz/pubs/waste-or-resource-solid-energys-beneficial-use-of-waste-streams/.
Werther, J., and Ogada, T. (1999). Sewage sludge combustion. Progr. Energy Combust. Sci. 25, 55–116. doi: 10.1016/S0360-1285(98)00020-3
Whitlock, S. E., Pereira, M. G., Shore, R. F., Lane, J., and Arnold, K. E. (2018). Environmentally relevant exposure to an antidepressant alters courtship behaviours in a songbird. Chemosphere 211, 17–24. doi: 10.1016/j.chemosphere.2018.07.074
Widdowson, J. P., and McQueeb, D. (1990). “Rehabilitation after opencast mining in Southland,” in Issues in the Restoration of Disturbed Land, eds P. E. H. Gregg, R. B. Stewart, and L. D. Currie. Occasional Report No. 4 (Palmerston North: Fertiliser and Lime Research Centre, Massey University).
Will, G. (1985). “Nutrient deficiencies and fertilser use in NZ exotic forests,” in FRI Bulletin (Rotorua: Forest Research Institute, New Zealand Forest Service).
Williams, P. A. (2011). Secondary succession through non-native dicotyledonous woody plants in New Zealand. N. Zeal. Nat. Sci. 36, 73–91. Available online at: http://www.science.canterbury.ac.nz/nzns/issues/vol36-2011/williams.pdf
Xue, J., Bakker, M. R., Miln, S., Graham, D., Ross, C., and Horswell, J. (2016). “Application of vermi-composts from septic tank waste improved soil fertility, early growth and nutrition of native and exotic trees species,” in New Zealand Land Treatment Collective: Proceedings for the 2016 Annual Conference (Gisborne).
Xue, J., Graham, D., and Ross, M. (2012). “Response of radiata pine and totara seedlings to the addition of biosolids and vermicomposted biosolids under glasshouse conditions,” in New Zealand Land Treatment Collective: Proceedings for the 2012 Annual Conference (Tauranga), eds G. Gielen and M. Heaphy, 131–138.
Keywords: biowastes, New Zealand, rehabilitation, biosolids, biodiversity, ecosystems
Citation: Simcock R, Cavanagh J, Robinson B and Gutierrez-Gines MJ (2019) Using Biowastes to Establish Native Plants and Ecosystems in New Zealand. Front. Sustain. Food Syst. 3:85. doi: 10.3389/fsufs.2019.00085
Received: 27 May 2019; Accepted: 18 September 2019;
Published: 04 October 2019.
Edited by:
Paula Alvarenga, University of Lisbon, PortugalReviewed by:
Paula Madejón, Institute of Natural Resources and Agrobiology of Seville (CSIC), SpainLaura Zavattaro, University of Turin, Italy
Copyright © 2019 Simcock, Cavanagh, Robinson and Gutierrez-Gines. This is an open-access article distributed under the terms of the Creative Commons Attribution License (CC BY). The use, distribution or reproduction in other forums is permitted, provided the original author(s) and the copyright owner(s) are credited and that the original publication in this journal is cited, in accordance with accepted academic practice. No use, distribution or reproduction is permitted which does not comply with these terms.
*Correspondence: Robyn Simcock, c2ltY29ja3JAbGFuZGNhcmVyZXNlYXJjaC5jby5ueg==