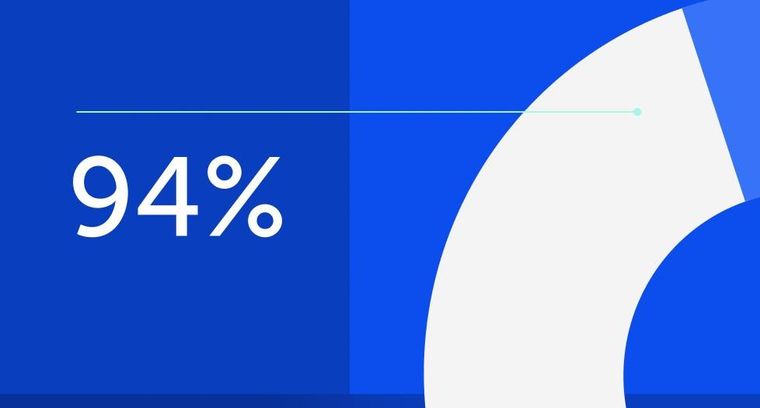
94% of researchers rate our articles as excellent or good
Learn more about the work of our research integrity team to safeguard the quality of each article we publish.
Find out more
ORIGINAL RESEARCH article
Front. Sustain. Food Syst., 24 September 2019
Sec. Agroecology and Ecosystem Services
Volume 3 - 2019 | https://doi.org/10.3389/fsufs.2019.00077
This article is part of the Research TopicMeasuring Interactions among Ecosystem Services in Agricultural LandscapesView all 7 articles
Privately owned rangelands in the western US support many ecosystem services and are threatened by financial incentives favoring conversion to housing development and more intensive forms of agriculture. Recognizing this threat, the impact investment community has identified rangeland management as a potential investing strategy to produce financial returns while preserving or enhancing the ecosystem services provided by intact rangelands. This strategy is based primarily on the notion that a capital-intensive conversion from continuous to rotational grazing can financially sustain rangelands through a combination of increased productivity and potentially monetized ecosystem service flows. The potential for these gains is supported by compelling anecdotal evidence, yet a robust body of scientific literature based on rigorous field experiments has not supported those claims, nor produced transferrable estimates of the benefits provided by rotational grazing of livestock (particularly cattle in the western US). Therefore, to demonstrate investment viability and measure investment success, impact investors will likely need to address these well-documented disconnects through some combination of monitoring and process-based modeling. This study examines the extent to which existing modeling tools are up to this task, by assessing the ability of two process-based models to represent four specific rotational grazing benefits put forth by impact investors, using a ranch in northeastern Wyoming as a case study. Using the Soil Water Assessment Tool, we simulated high magnitude changes in the water balance from surface runoff to evapotranspiration, which may be a benefit or negative impact depending on context (Benefit 1). We simulated a decrease in soil water storage under rotational grazing, which directly contradicts the outcome assumed in investment literature (Benefit 2). Using the InVEST beta Rangeland Production Model (based on the Century ecosystem model), we simulated increased biomass productivity (Benefit 3) under rotational grazing, which enhanced animal performance under some management parameters but negatively impacted it in others (Benefit 4). We conclude that the impact investing community will likely find greater success through a shift to objective-oriented ranch management, rather than a specific focus on rotation, and will also need additional investment in science and monitoring to demonstrate benefits.
Rangelands comprise roughly 31% of the United States' total land area with the majority of these rangelands located in the arid and semiarid western portion of the country (Havstad et al., 2007), where more than half are privately owned (Maczko et al., 2011). Many of these rangeland ecosystems are unique in that they can be managed for grazing and thereby simultaneously be kept in productive agriculture while maintaining native vegetation and soil cover, in contrast to other more intensive forms of cropping (Brown and MacLeod, 2011). Such functional and intact rangeland ecosystems are linked to a diversity of ecosystem services (ES) benefiting people living in the western US (Havstad et al., 2007; Maczko et al., 2011; Teague et al., 2013), including the provisioning of recreational opportunities, food and fiber (via ranching), carbon sequestration, water security, and wildlife habitat (Havstad et al., 2007). However, pressure from changing demographics and demands (Maczko et al., 2011; Reeves et al., 2018) is creating strong financial incentives for the sale of privately owned rangelands for housing development and more intensive forms of agriculture (Huntsinger and Hopkinson, 1996; Goldstein et al., 2011; Maczko et al., 2011; Wetzel et al., 2012; Cameron et al., 2014). This transformation of rangeland ecosystems in the form of both fragmentation and conversion has a substantial impact on the vitality of rural economies, wildlife and bird habitat, recreational opportunities, and carbon storage, among other benefits (Resnik et al., 2006; Havstad et al., 2007; Maczko et al., 2011; Reeves et al., 2018).
To the extent market forces are capturing social preferences, the pressures driving conversion of rangelands would indicate that there is a greater social value provided by homes and cropland, and this may very well be the case in many areas. However, in contrast to the benefits of conversion, many of the ecosystem services being provided by intact rangelands are under- or un-priced, which could lead to more conversion than socially preferred if all costs and benefits were fully accounted for (Maczko et al., 2011). Additionally, the dynamics of conversion raise issues of equity and hold the potential to pit wealth held by those external to rangeland communities against the interest of local economies. The appropriate balance between these considerations is a matter of policy, economics, and politics. However, any negotiation of outcomes in that space needs to be underpinned by a solid understanding of the benefits (and potential negative impacts) associated with preserving functioning rangelands, so that they can be put on a more level playing field via financial or regulatory incentives (Havstad et al., 2007).
For those interested in preserving functioning rangelands, one prominent path to counterbalance the market forces driving conversion of intact rangelands—and the ES they provide—is to increase the financial returns to owning and managing them (e.g., Cheatum et al., 2011). There are several publicly funded programs in the US, including parts of the 2014 Farm Bill like the Agricultural Conservation Easement Program (ACEP), which currently compensates ranchers for maintaining rangeland ecosystems and providing ES to stakeholders (Goldstein et al., 2011). Under ACEP, ranchers are provided tax incentives for conservation in the form of conservation easements (NRCS, 2014). Long-standing approaches like land-use planning, regulatory efforts, and land trusts will continue to play an important role as well; however, these approaches all have disadvantages from a feasibility, scalability, and political acceptability standpoint (Sheridan, 2007; Goldstein et al., 2011).
Stemming from the recognition of these limitations, there is growing concern that neither existing public support programs nor traditional land use management approaches are likely sufficient to meet the problem of rangeland fragmentation and conversion with the urgency perceived by those who advocate for it (Gage et al., 2016). Furthermore, the expansion of these programs—particularly publicly funded ones—may be challenging for several reasons, including taxpayer unwillingness to pay for ecosystem services that have historically been provided for free, and political concerns about the use of public funds for agricultural operations on private lands (Sheridan, 2007; Goldstein et al., 2011).
At the same time, there has been increasing interest in the role private finance may be able to play in sustaining rangelands and rangeland ES (Montalto et al., 2007; AlphaMundi SAIF, 2010; Pons et al., 2013; NatureVest EKO Asset Management Partners, 2014; Culp et al., 2015; Held, 2017). Impact investment, which is more broadly focused on environmental and social returns in addition to expected financial gains (Brest and Born, 2013), could fill gaps in public funding or missing markets to enable rewards for good stewardship that leads to sustained provision of ES (Pons et al., 2013). To date, impact investment interest in private rangelands has focused on payments linked to the establishment and maintenance of what is termed a “rotational grazing” system, where cattle are grazed more intensively for short periods, in theory mimicking more natural grazing patterns of a migrating herd and providing ecological benefits (Hodgson, 1979; Pons et al., 2013; Culp et al., 2015; Lang et al., 2017), which we discuss in more detail in the paragraphs below. For example, a US$20 million pilot deal focused primarily in eastern Montana aims to regenerate “soil, water, biodiversity and carbon sequestration as well as reduction in feed costs, fuel and labor costs reduced by 50%” through financing the land management arm of the Savory Institute, Grasslands LLC (Pons et al., 2013). Preliminary investment ideas for the Colorado River Basin that focus on grazing management also cite the Savory Grazing Method (one method for grazing management that emphasizes rotational grazing) as justification to financially incentivize some system of rotational grazing management (Culp et al., 2015). In the case of the Colorado system, the provision of an economic incentive for transition to rotational grazing management is suggested to generate “improvements in the water cycle,” increases in groundwater capture, forage composition, and forage production (Culp et al., 2015).
In the impact investment literature reviewed as part of this study, rotational grazing systems are positioned in contrast to other grazing systems, including continuous grazing systems. Investors provide the upfront capital to facilitate conversion from continuous to rotational grazing, and then generate financial returns based on a combination of monetized ecological benefits and increases in ranching productivity. The split between the two sources of returns, as well as between investors and rangeland managers, is determined by the context and contracting structure that is unique to each case. For example, Pons et al. (2013) outline a pathway where investors provide capital and then obtain financial returns in the form of improved crop and livestock production derived from increased forage productivity and soil health. A parallel strategy is outlined by Culp et al. (2015) where investors provide capital to convert from continuous to “regenerative” grazing (i.e., an intensive rotational system), after which several benefits to both landowner and investor are derived, including improved forage quality and livestock capacity and corresponding improved income from the sale of higher quality or a higher quantity of livestock. Both examples reflect the larger desire to find “win-wins” where relevant stakeholders receive a diversity of ES from conserved rangelands, and investors obtain a reasonable return on their investment.
The keen interest from private capital and the purported benefits of rotational systems raise the question of why conversion to rotational systems has not been more widespread to date. We suggest that there are two main reasons for the lack of large-scale adoption in the rangeland management community. First, the transition to a rotational system is expensive and rangeland managers may not have the capital required to facilitate the transition, despite being optimistic about the benefits of this transition—this is the gap that private finance would then fill. Although limited financial data on this subject was available, capital investment is required to subdivide existing pastures into smaller pastures and build water infrastructure for livestock where it may not have previously existed, among other expenditures (Beetz and Rinehart, 2010). This cost, despite the potential perception of benefits, may be prohibitive, particularly in the absence of available credit. Second, and perhaps more crucially, managers or lenders may be unconvinced that rotational grazing can deliver on all the benefits promised by its proponents because anecdotal and scientific evidence on these benefits is inconclusive, as we elaborate below. Impact investors presumably believe that lack of access to capital is the more significant of these barriers. However, based on the literature review conducted as part of this study, we believe that the inconclusiveness of experimental results to date warrant further consideration as well.
In justifying grazing management interventions, the impact investment literature commonly cites holistic range management and the work of Allan Savory (Pons et al., 2013; Culp et al., 2015; Lang et al., 2017), who is arguably one of the most prominent advocates for rotational grazing. However, a variety of rotational systems exist, and the suggestion that some form of rotational grazing provides better economic and environmental returns has received considerable attention from the academic community since the 1930s (Morgan, 1933; Hodgson et al., 1934; Briske et al., 2008; Barnes and Hild, 2013). Specific rotational grazing approaches vary with respect to variables like stocking rate, stocking density, rotations per year, grazing days, and length of grazing deferment, and many land managers who have embraced various forms of rotational grazing management report seeing dramatic evidence of improved productivity and profitability [case studies in Rangelands 2013 special issue, 35 (5)]. This includes some cases where increasing the overall stocking density of a property resulted in maintaining or enhancing ecological condition [for example, as measured by altered ratio of warm- to cool-season grasses; case studies in Rangelands 2013 special issue, 35 (5)].
At the same time, experimental studies in the literature generally fail to reproduce these benefits (Briske et al., 2008). Table 1 summarizes a targeted literature review of four benefits that are common to the investment literature (Pons et al., 2013; Culp et al., 2015; Lang et al., 2017). The selected outcomes constitute a mix of intermediate outcomes and outcomes that may be directly monetized or otherwise provide a benefit, specifically: (1) changes in the water balance in favor of increased water availability for uses people value (Pons et al., 2013); (2) increases in groundwater capture (Pons et al., 2013; Culp et al., 2015; Lang et al., 2017); (3) improvements in forage productivity; and (4) improvements in animal performance. These studies, which are discussed in greater detail in the Literature Review Supplement, generally show results that are mixed at best.
Table 1. Summary of literature reviewed for this study, broken out by primary benefit examined (if a study examines multiple relevant benefits, it will appear more than once).
The lack of rigorous experimental evidence substantiating these anecdotal claims creates challenges for those looking to predict the benefits of an investment in rotational grazing, or evaluate them ex-post. The ability to do at least one of these is likely to be necessary to secure investment and, depending on contract structure, to attribute returns in an impact investing context (Barnes and Hild, 2013). Yet, the existence of such a significant body of compelling evidence and individual rancher experience exists makes it difficult to conclude that the benefits of rotational grazing management are simply an illusion (Sayre, 2001; While, 2008; Teague et al., 2011; Barnes and Hild, 2013; Norton et al., 2013). While failing to find strong support for the advocated benefits, our literature review also indicates there are several overarching factors that make comparing rotational and continuous grazing systems difficult, meaning the effects could be real but challenging to reproduce experimentally.
First, there may be meaningful differences between experimental and working conditions: many ranches have large pastures that are heterogeneous in terms of productivity and pasture composition (Barnes and Hild, 2013). Such heterogeneity compared to experimental plots may inhibit the ability to reproduce anecdotal findings because experimental plots may be limited in their replication of actual pasture or property conditions. Second, the rigidity of experimental controls may limit the ability of these studies to replicate on-the-ground practices such as adaptive management, designed to be dynamically responsive to changing conditions and goals (Briske et al., 2008; Teague et al., 2008). Third, the unpredictability of precipitation makes replication of management conditions difficult: In practice, the adequate recovery period to allow vegetation to regrow before being grazed must often be variable in response to weather, level of defoliation, and timing of defoliation (Grissom and Steffens, 2013). These differences in grazing management regimes make them difficult to test in a formal, experimental setting.
The results of our literature review portray, at best, a mixed picture for the benefits of rotational grazing (see citations in Table 1). Yet, there are glimmers of hope within the set of formal empirical studies, and the anecdotal evidence remains compelling to many. Seemingly more persuaded by the favorable pieces of evidence, the impact investing community has grown increasingly interested in meeting the strong demand for supporting rangeland ecosystems. Additionally, producers are interested in the potential for enhanced income from increased productivity, supplemental income from direct payments for ES, and recognition for stewardship (Goldstein et al., 2011). To move from concept to action, any proposed impact investment will need to convince risk-bearing parties of the likelihood and potential magnitude of benefits, before the investment is made. Evaluation is also likely necessary after the investment, since ex-post evaluation may form a basis for payment, and even if it does not, new investment is unlikely to follow in the absence of a growing evidence base that early investments achieve their goals in practice.
Given the complexities involved and the likely infeasibility of deploying empirical trials with enough statistical power alongside each impact investment, we believe some form of process-based modeling will be necessary to assess benefits. Process-based models integrate pre-existing established theoretical relationships, constrained by parameters and available data (Curtis et al., 1992). They can therefore serve two roles in the rangeland impact investing sphere. First, as “virtual laboratories,” they allow an analyst and stakeholders to conduct numerous “experiments,” which in turn can help clarify the implications of various assumptions and parameters, including exploration of whether the institution of a rotational grazing program alone is sufficient to generate the proposed benefits, and whether potential trade-offs between outcomes of interest might exist (Bankes, 1993). Second, process-based modeling is a way to test our ability to evaluate the hypothesized impacts of changes in grazing management under realistic conditions—e.g., on actual ranches, with the data that would be available in the process of assessing a candidate impact investment.
Our study therefore tests the ability of two candidate models to capture the selected benefits derived from the institution of a rotational grazing system as suggested in impact investment literature at a representative study site in northeastern Wyoming1. To make the results of this study more relevant for impact investors, we examined modeling tools for which data and model parameterizations are readily available in the rural Western US, which we believe reflects realistic data constraints that will be faced for this type of effort in the region. Using this low data requirement as a guidepost, we selected two models—the Soil Water Assessment Tool (SWAT) and the InVEST beta Rangeland Production model (hereafter, RPM), which were constructed to operate independently of each other, but with harmonized overlapping inputs where relevant. Focusing on the benefits highlighted by the impact investment literature, we parameterized the models to compare a rotational system and continuous system, and tested whether they exhibited differences in the water balance, groundwater capture, forage productivity, and animal performance post-treatment (e.g., after the institution of a rotational grazing system). We find that their ability to do so is quite limited, illuminating some fundamental trade-offs between desired benefits, and suggesting that where benefits are real, a combination of more sophisticated models and more detailed data and monitoring will be necessary to reliably detect them.
We constructed our modeling effort to reflect characteristics of the Ucross ranch, a site in northwestern Wyoming that was selected for its representativeness of properties impact investors are likely to target and because it has experienced beneficial changes as a result of changes in management. Ucross is characteristic of many cattle operations in the arid and semi-arid Great Plains and has basic documentation of grazing and landscape changes dating back to the early 2000s (Graham, 2014). Ucross is also situated in the same ecoregion (Northwestern Great Plains) and a similar ecological site precipitation zone as the Grasslands LLC pilot project referred to earlier for impact investment.
Ucross is an 8,500 ha property located in northeastern Wyoming adjacent to the Big Horn Mountains and at the juncture of the Alpine Middle Rockies upland and the Northwestern Great Plains lowland (Kuhn et al., 2017). The property is situated within the larger 2,849 km2 Clear Creek Watershed (hydrologic unit code: 10090206), which ranges in elevation from roughly 4,000 m above sea level in the alpine uplands to roughly 1,000 m above sea level in the agriculturally productive lowlands. In the Clear Creek Watershed, ~75% of the land cover is rangeland and 18% is forest. The remaining portion of the watershed is comprised primarily of irrigated pasture, agricultural lands, and wetlands. The climate is typical of semi-arid systems with lows ranging from −39°C to highs of 40°C and an average annual temperature of 7.4°C (Kuhn et al., 2017). Annual precipitation ranges from 37 cm to 76 cm, the majority of which falls as snow at higher elevations. Lower portions of the Clear Creek Watershed, including the Ucross ranch, sit within the 25.4–35.6 cm Northern Plains ecological site precipitation zone (NRCS, 2019). Like many arid and semi-arid systems in this part of the western U.S., the seasonal hydrograph is driven primarily by spring runoff (Kuhn et al., 2017).
Ucross has been managed as a working cattle ranch dating back to the 1880s. In the mid-1960s, the property was purchased by the Apache Corporation. In the early 2000s after seeing declining plant productivity, increased erosion, and noxious weed invasion, ranch management underwent a dramatic shift that emphasizes a grazing duration of 14 days or fewer per pasture and a 90-day standard deferment between grazing events (Graham, 2014). With this change in management, operators observed several beneficial effects with respect to both ranch-level productivity and ecological function. The ranch-level stocking rate was doubled, percent bare ground was reduced, yearly forage biomass production increased, desirable grass species increased, and more streams were observed to contain water year-round (Graham, 2014). We provide more information of prior management in Supplementary Material under the Study Site Supplement.
Our study was designed to test the ability of existing modeling tools to demonstrate four key outcomes that investors anticipate occurring when ranchers transition from a continuous grazing system to a rotational one. We selected two models—the Soil Water Assessment Tool (SWAT) and the InVEST beta Rangeland Production model (hereafter, RPM) to simulate differences in the water balance, groundwater capture, forage productivity, and animal performance after the institution of a rotational grazing system. As illustrated in Figure 1, these modeling efforts share substantial common inputs based on the study site characteristics but were conducted in tandem and are therefore not directly linked by endogenous feedbacks. For instance, the output parameters from the InVEST beta RPM are not used as input for SWAT model, though in theory a more fully coupled system would better capture the effects of an intervention. However, as noted above, our intent is first to test the adequacy of existing models, in part to assess whether added complexity would have practical value.
Figure 1. Modeling and analysis workflow. Models share common inputs where feasible but are run without feedbacks. SWAT examines a single grazing characterization represented in a spatially explicit manner on the Ucross landscape, while InVEST RPM is run to explore various management strategies on a single representative grazing area. Both models are run under rotational and continuous management to allow a focus on the impact of management strategy.
In the section below, we first outline the SWAT modeling strategy and then outline the RPM modeling strategy. Because no concrete guidance on how to define rotational grazing was indicated in the investment literature, we tested grazing in two ways, to capitalize on the relative strengths of each model. First, with the SWAT model, a rotational grazing scenario was constructed to mimic the actual management at Ucross to the extent possible, modeling the rotation (or not) of cattle across specific individual pastures. Second, with the RPM model, we tested a broader range of scenarios to compare results from different rotational grazing regimes, while limited to a representative Ucross pasture.
In all cases, we assess the impacts of rotational grazing against a continuous baseline, meaning that when appropriate, the results are presented as the rotational grazing result minus the continuous grazing result. Thus, when a result is negative it indicates a net decrease in water, biomass, or diet sufficiency resulting from the transition from continuous to rotational grazing and correspondingly when a result is positive, it indicates a net increase in water, biomass, or diet sufficiency resulting from the transition.
We selected SWAT for the hydrologic modeling component of this study because it is a reliable predictive tool that can be used in areas with limited input data (Stehr et al., 2009; Neitsch et al., 2011; Arnold et al., 2012; Nyeko, 2015). SWAT is a physically based, semi-distributed catchment scale model that has been widely used to quantify the effects of different land management practices on hydrologic, sediment, and nutrient dynamics over a daily, monthly, or yearly time-step (Neitsch et al., 2011). Over the past 30 years, SWAT has been well-documented as a capable tool for tackling water resources management challenges with a strong land-use component. For more detailed information about SWAT's architecture see Arnold et al. (2012) and Neitsch et al. (2011).
We used SWAT to test whether treatment lead to the simulation of the hydrologic outcomes put forth in the impact investment literature. In the absence of specific practices and parameters from that literature, we elected to compare a single representative grazing strategy, derived from a combination of Ucross ranch management input and literature values, against a continuous grazing baseline. This allowed us to test whether modeling a representative rotational grazing strategy for the study site under realistic conditions of data availability can simulate desired outcomes in the water balance and in groundwater capture.
The SWAT calibration used the only source of observed streamflow data available, which was from a USGS stream gage (No. 06317000 on Clear Creek near Arvada, Wyoming) to define a complete calibration watershed (henceforth referred to as the Clear Creek watershed) that contained Ucross (please see the SWAT Model Supplement in Supplementary Material). Nash Sutcliffe Efficiency (NSE) was calculated to be 0.69, goodness-of-fit (R2) was calculated at 0.75, Root Mean Square Error (RMSE was ±223 cm, RMSE-observation standard deviation ratio (RSR) was 0.55, and PBIAS was −19.4%. According to the criteria for monthly timestep models put forth by Moriasi et al. (2007), these performance statistics constitute a “Good” result with respect to RSR and NSE. When the log of observed and simulated flows was taken to reduce the sensitivity of NSE to extreme flows (Krause et al., 2005), NSE improved to 0.83. Although the calculated PBIAS was poorer than NSE or RSR, it constitutes a “Satisfactory” result according to Moriasi et al. (2007).
Once the Clear Creek watershed SWAT model was calibrated and validated, the resulting parameters were used to create a nested SWAT model for scenario analysis at the Ucross property using detailed land use information from Glick et al. (2016). That is, a calibrated watershed level SWAT model was further refined within the Ucross boundaries2. The delineation of this smaller area using the land cover information from Glick et al. (2016) resulted in the subdivision of the property into 36 subbasins. Using a combination of the suitability of the land classification type (e.g., range) and information from Ucross management, 16 of the 36 subbasins were determined to contain HRU's suitable for grazing operations within SWAT (see Figure 2). In this way, grazing was restricted to areas that were grazed on the Ucross property (based on best available information—e.g., animals in the model were prohibited from grazing areas that were not grazed by animals in real life). A similar approach to the representation of rotational grazing operations by subbasins was applied in Park et al. (2017).
Figure 2. Map of the grazed subbasins within the Ucross property used for setting up the rotational and continuous grazing scenarios.
Following the creation of the nested model, we constructed two different grazing scenarios to represent continuous and rotational grazing. These scenarios were constructed using a combination of information provided by Ucross management and values from literature specifically aimed at improving data input for grazing scenario setup in SWAT (Almendinger and Murphy, 2005). Inputs for both scenarios are summarized in Table 2 below, with additional information in the SWAT Model Supplement and Supplemental Tables 1, 2. The average input curve number was 79 for the continuous scenario and 78.8 for the rotational scenario. For more information on how these scenarios were derived, see the SWAT Model Supplement in Supplementary Material.
Table 2. Input information for SWAT grazing scenario setup where * denotes information sources from discussions with Ucross management and ** denotes information sourced from Almendinger and Murphy (2005) for grazing inputs in SWAT.
The results of the rotational and continuous scenarios were used to generate an annual average water balance for the watershed, which included simulated totals for precipitation, surface runoff, infiltration, evapotranspiration (ET), evaporation from the shallow aquifer, aquifer recharge, lateral flow, and return flow. We assessed changes in these simulated totals by taking the difference between rotational and continuous results and dividing them by total precipitation. SWAT produces multiple groundwater relevant outputs that may be of interest to impact investment since they may be indicators of baseflow relevant for dry season water demand or riparian habitat. For this study we focus specifically on soil water, as a measurement of the simulated change in soil water storage and by extension, an indicator of whether the model simulated increased groundwater capture, which we interpret to be of most interest in impact investment literature. We also focused on percolation, which examines water that percolates past the root zone to assess differences in deeper groundwater storage. Finally, to examine whether a conversion to rotational management at a watershed scale would result in benefits that merit consideration from a water resources perspective, we calculated the volumetric difference in simulated changes across the grazed portion of the Ucross property (31.28 km2) as well as an extrapolated value based on the area of rangeland within the larger Clear Creek watershed (1,339 km2 or 47% of 2,849 km2). While the impact of rotational grazing will vary spatially, this latter value provides an approximation of the total changes that might be observed if rotational grazing were adopted in all relevant areas across the watershed.
In parallel with the SWAT modeling, we used the InVEST beta RPM to investigate the impact of rotational grazing on forage biomass and animal performance. RPM is a process-based model that simulates forage production, energy demands of grazing animals, and animal diet selection from commonly available input data (see full model description in the RPM Supplement in Supplementary Material). By coupling the Century ecosystem model (version 4.6; Parton, 1996) with a basic ruminant physiology model adapted from the Australian feeding standard (CSIRO, 2007), the Rangeland Production model captures the broad impacts of management decisions such as duration and intensity of grazing on the viability of livestock production systems. While the low data requirements of the RPM render it much less precise than existing traditional ranch management models (e.g., Corson et al., 2007; Rotz et al., 2013), several features of the model make it relevant to address the impacts of rotational grazing described above. These include differentiation of the nutritional quality of live vs. senesced vegetation; dynamic diet selection limited by both forage availability and quality; and nonlinear impacts of grazing intensity on forage regrowth after defoliation.
RPM predicts forage growth in terms of quantity (biomass) and quality (crude protein content) and estimates the viability of livestock production given that level of forage production. The model uses the Century ecosystem model (Parton, 1996), a monthly time-step ecosystem model developed for use in managed grasslands, to predict above- and belowground carbon and nutrient pools including forage biomass and protein content. The core equations of the coupled ruminant submodel, which describe forage intake, energy and protein supplied by the diet, and energy and protein requirements for maintenance, were adapted from the animal biology submodel of GRAZPLAN (Freer et al., 1997; see the RPM Supplement in Supplementary Material for full animal submodel equations).
While it does not track livestock weight, meat, and milk production explicitly, RPM instead uses the metric of diet sufficiency to characterize animal performance given predicted forage biomass and quality. The diet sufficiency metric describes, for each modeled timestep, the extent to which energy intake of the animal diet meets or exceeds maintenance energy needs. It is calculated as the ratio of total intake of metabolizable energy from the diet (MEintake) to energy requirements of maintenance (MEreq) (Equation 1).
A diet sufficiency value greater than one indicates that the herd is expected to gain weight; a value less than one indicates that the diet is insufficient to maintain current weight or condition and the herd is expected to be losing weight. The livestock herd is simulated as a static collection of age/sex classes, since uncertainty about the exact herd demographics over a landscape makes tracking the growth and consequent change in feeding requirements of an individual unrealistic. For the simulations conducted here, we simulated a single age/sex class representing growing heifers (Table 3). The monthly timestep of the Century model restricted our rotation scenarios to grazing and rest periods of 1 month or longer, but within that constraint we tested a range of ranch-level stocking rates and number of pastures, as described below.
We used the Middle Alkire pasture of Ucross ranch as the simulation setting for the initial test of modeling capability to capture the hypothesized benefits of impact investment in rangeland productivity and animal performance. Model inputs and data sources are summarized in Table 3. In contrast to the SWAT modeling, all model inputs described a single representative pasture in Ucross; for continuous scenarios, this pasture was grazed season-long, while for rotational scenarios, the pasture was replicated in multiple instances which were each grazed for 1 month in sequence. By utilizing this representative pasture instead of the multiple pastures of Ucross that differ in size, topography, and pasture composition, we isolated the effect of timing and duration of grazing. Animals in both continuous and rotational scenarios grazed only in months 4–10.
The stocking density of each pasture in continuous and rotational scenario was calculated from the total area of all pastures and the total herd size. For example, under hypothetical conditions of 100 animals grazing 100 ha, the stocking density in one pasture grazed season-long (the continuous scenario) would be 1 animal per ha. In rotational scenarios, pasture-level stocking density differed according to the number of pastures simulated. With two pastures, the stocking density during each month of grazing under the same ranch-level stocking rate was 100 animals per 50 ha (2 animals per ha). In rotational scenarios, the animal herd was rotated between pastures in sequence. For example, with 3 pastures, the herd was allocated to pasture 1 in month 1 of grazing, pasture 2 in month 2, pasture 3 in month 3, and back to pasture 1 in month 4. Therefore, with a greater number of simulated pastures, each pasture received a longer rest period but also experienced a greater pasture-level stocking density when grazed.
We ran the model for 5 years (60 monthly time steps) under continuous and rotational grazing, both following a spin-up under continuous grazing scheme as described by Parton et al. (1993). We contrasted the effect of total stocking rate and grazing/rest period duration by testing a range of number of pastures in rotational scenarios, and a range of ranch-level stocking rates including the empirical stocking rate of 0.51 animals per ha (Graham, 2014). For each scenario, we recorded residual pasture biomass and animal performance at each monthly time step, according to the selected diet. In rotational scenarios, pasture biomass was calculated as the average residual biomass across all pastures, including the pasture that was grazed in the current timestep and those that were rested.
The composition of the water balance remains largely unchanged when comparing rotational grazing to a continuous baseline (Figure 3 below). Water balance components show in Figure 3 include lateral flow, groundwater contribution to the stream, percolation, and deep aquifer storage; all of which remain largely unchanged. The primary difference in the transition from continuous to rotational appears to be the shift of 3.5% of average annual precipitation from surface runoff to ET.
Figure 3. Differences in average annual total water balance components over the simulation period (2003–2009). The primary impact is a shift from surface runoff to ET, which also decreases water yield. Soil and groundwater are generally unaffected or experienced slightly negative impacts relative to the direction hoped for by impact investors.
The magnitude of impacts from watershed-wide treatment may be substantial and negative in value (Table 4, Column 6; summarizing changes in the water balance at the ranch and when extrapolated to relevant parts of the watershed). For instance, under a rotational grazing system surface runoff is approximately 572 ae-ft lower over the course of the year and ET is approximately 630 ae-ft higher over the course of the year for the entire Ucross property. Although these results indicate the potential for high magnitude changes, our model did not indicate that the forgone surface runoff corresponded with a simulated increase in percolation and recharge and by extension, the potential for increased on-farm water capture (though this may be a limitation of the curve number approach, see discussion below). Instead, our SWAT model indicates that approximately 91% (572 ae-ft of 630 ae-ft) of the foregone surface runoff is evapotranspired under the rotational scenario; a tradeoff that is also observed in the results presented in annual water balance ratio under baseline scenarios in Park et al. (2017). While likely not to be considered a beneficial hydrologic outcome, this would be consistent with an increase in forage production.
Table 4. Volumetric totals and comparisons for water balance parameters throughout the season at the ranch and watershed scale.
Scenario results indicated a net mean monthly decrease of around 8 mm in soil water storage compared to rotational grazing, with a minimum decline of ~1 mm and a maximum decline of ~14 mm. Distributed across the entire Ucross property, this equates to the loss of approximately 203 ae-ft in soil water storage per month on average with a low of 25 ae-ft and a high of 330 ae-ft per month.
Simulated differences in percolation exhibited a high number of outliers at the monthly level, with a spread from 2 to −4 mm with a simulated monthly median value of around 0 mm. This supports a conclusion that overall changes in percolation are negligible, though month-specific effects show higher variance and with changes in both directions. These results relate to the higher trampling rate per unit area in the rotational grazing scenario, which compacts the soil and likely lowers both water storage capacity and percolation. Figure 4 indicates the distribution of changes in monthly soil water and changes in monthly percolation.
Figure 4. Distribution of monthly simulated differences in soil water and percolation based on the two grazing scenarios. Change in monthly parameters is calculated by subtracting continuous results from rotational results for both soil water and percolation, meaning that when the value is negative there was more soil water or percolation under the continuous scenario and vice versa.
RPM simulations showed that while the range of pasture biomass across pastures in rotational scenarios was large, average pasture biomass across grazed and rested pastures was consistently higher in rotational scenarios than in continuous (Figure 5). This increase in forage biomass due to the grazing schedule was larger under higher ranch-level stocking rates, although forage biomass declined with increasing stocking rate under both continuous and rotational scenarios, as would be expected. When animals were rotated among a greater number of pastures, enforcing both a higher pasture-level stocking density and a longer rest period under rotation, the increase in forage biomass compared to the continuously grazed scenario was also larger (e.g., reading across rows of subplots in Figure 5).
Figure 5. Monthly simulated standing biomass in continuous (solid line) vs. rotational grazing (dashed line) simulations. Sub-plots show number of pastures (2–10) in rotation, and total ranch-level stocking rate (0.36–0.66 animals/ha). All simulations were run for 5 years, but for clarity only the final 12 months are shown here. Intra-annual patterns were similar across all 5 years (see Supplemental Figure 5 for full results across all simulated years).
In rotational scenarios, the entire herd grazed in one pasture during a month-long grazing period, while other pastures rested; therefore with higher number of pastures, the pasture-level stocking density during the grazing period was 2–10 times the ranch-level stocking rate for both continuous and rotational scenarios (Figure 5). While the grazing period in rotational scenarios was always 1 month long, with higher number of pastures the rest period was also longer.
In contrast to average forage biomass, animal performance did not show a consistent direction of response to rotational grazing as compared to continuous (Figure 6). Instead, the impact of rotation on diet sufficiency differed both with total stocking rate and with the number of pastures used in rotational scenarios. While animal performance was slightly higher than continuous grazing across stocking rate levels when animals were rotated between just two pastures, the selected diet often did not meet maintenance needs in rotational scenarios that used 10 pastures. That is, predicted diet sufficiency was often <1 in rotational scenarios with 10 pastures, indicating that the available forage was inadequate to maintain current weight or condition. This is likely due to the extremely high pasture-level stocking density in grazed pastures when 10 pastures were used (i.e., 10 times the ranch-level stocking rate, from 3.6 to 6.6 animals per ha in the pasture being grazed). While average biomass across pastures was high (Figure 5), the available forage in the one pasture being grazed was often not sufficient to meet the animals' dietary requirements (see Supplementary Figure 4).
Figure 6. Animal performance, as measured by diet sufficiency, in continuous (solid line) vs. rotational grazing (dashed line) simulations. All simulations were run for 5 years, but for clarity only the final 12 months are shown here. Intra-annual patterns were similar across all 5 years (see Supplemental Figure 6 for full results across all simulated years).
As expected, total stocking rate had a clear impact on the ability of animals to meet their energy needs regardless of rotation strategy (e.g., diet sufficiency tended to dip below one more often at higher stocking rates), but our results also show that rotational grazing ameliorated or exacerbated this effect depending on the number of pastures used. Animals rotated between just two pastures at a high stocking rate had greater performance than animals grazed continuously, but animals rotated among 10 pastures under the same high stocking rate experienced much lower performance than those grazing continuously (contrasting columns in bottom row of subplots, Figure 6). The energy shortfall indicated by the low diet sufficiency values in the more extreme rotation scenarios likely reflect conditions of scarcity more extreme than a typical manager would tolerate, an issue of realism that we return to in the discussion.
Our two complementary modeling efforts were designed to test the ability of existing tools to demonstrate four benefits consistently cited by impact investment as potential benefits associated with the switch from a continuous to a rotational grazing system. Figure 7 provides a qualitative summary of our findings. In brief, we find that the consistency and reliability of simulated outcomes of treatment are not as clear cut as may be required to serve the impact investing community. For overall water balance, the shift from runoff to ET is significant but whether it is a benefit is context dependent, while for groundwater the shift is consistent but small and in the opposite direction of what is preferred by impact investors. For biomass and animal outcomes, the impacts are a clear function of management choices within the rotational system, showing real but modest potential benefit at the lower stocking densities.
Figure 7. Summary of findings for four selected benefits, including qualitative assessment of direction of effect (e.g., positive, zero, or negative) of rotational grazing based on the simulation results.
There are, however, several caveats to these findings applicable to both models. One relates to the limited exploration of all the various types of rotational grazing that may be used by a manager, and limited ability to represent any adaptive, responsive management. We recognize that grazing plans often include ad hoc modifications made by managers based on range condition or other relevant observations. The responsiveness of managers to variability in conditions has been put forward as a critical component of successful grazing systems, with respect to the generation of desired environmental and hydrologic benefits (Barnes and Hild, 2013). To fully capture adaptive management in either model is likely to require large amounts of site-specific data and extensive expert knowledge of the system, which equates to time and money that may or may not be available. Additionally, while we harmonized inputs to the extent possible, our modeling effort does not dynamically link the hydrologic, biomass, and animal outcomes into a single integrated model, a challenge where future work could be particularly helpful in more fully measuring tradeoffs and benefits. We imagine that this would not require additional data, but would add complexity and as a result, more uncertainty in results.
With that said, our models were constructed to test our best approximation of actual grazing management at Ucross in SWAT and a range of range of rotational grazing regimes using RPM. In addition, we believe that the types of challenges we faced with respect to the construction of realistic grazing scenarios in terms of both input data challenges and actual scenario construction are likely to be confronted as part of any pre- or post-investment evaluation in the rural western US. In the sections below we discuss these issues in more detail, broken out by simulated hydrologic benefits and simulated forage/animal performance benefits.
Our results do not demonstrate hydrological outcomes supporting the proposition that rotational grazing will simultaneously provide hoped-for “wins” for impact investors and ranchers, relevant stakeholders, and the environment. While our simulated water balance outcomes did indicate the potential for high-magnitude differences in surface runoff and ET, whether this constitutes a benefit or not will be viewed differently in different contexts and by different stakeholders. Such an assessment requires considering the value of water for a specific system, which means that continuous or rotational grazing may be more beneficial depending on the specific considerations. For instance, if water is needed for aquatic habitat, reducing surface water runoff may pose a problem for maintaining minimum ecological flows or supporting adjacent wetlands. However, if reduction in surface water runoff increases water available for forage production and prevents erosion of topsoil, that may mean that the effect of the rotational program is a net positive.
The only other published study utilizing SWAT to investigate grazing management was conducted by Park et al. (2017). Our results generally track average annual water balance ratios reported by Park et al. under the baseline scenario with respect to decreased surface runoff and, to a lesser extent, increased ET under rotational grazing. However, extrapolation of these results is somewhat limited by different average annual precipitation: average annual precipitation at the Park et al. (2017) study site (86.4–96.5 cm) is more than triple annual average precipitation at Ucross (25.4–35.6 cm). Park et al. (2017) also examine flood risk reduction attributable to reduced surface water runoff and do find some benefit from rotational grazing.
With respect to groundwater, simulated results indicated that rotational grazing resulted in a net decrease in soil water and no overall change in percolation. We expect that this difference is largely linked to differences in trampling rate; because per unit area trampling is higher under rotational grazing, infiltration and soil water capture decline. While this does not suggest that the groundwater benefits derived from chipping of hooves (Pons et al., 2013) or churning of sod (Culp et al., 2015) do not occur, SWAT does not capture them, which would limit its utility in evaluating a program that assumes benefits from these mechanisms. Additionally, although the direction of the effect was clearly negative, the simulated difference was relatively small in comparison with differences in ET and surface runoff—so even if the sign were reversed, it may still not be of sufficient magnitude to be relevant for an impact investment.
An additional limiting factor is that we were only able to calibrate our models against surface water data, due to lack of data available for soil moisture and groundwater. Although we believe that this constraint is likely to be typical of the systems targeted for impact investing, the lack of calibration data is particularly problematic in the context of simulated groundwater results, where measured data about soil moisture content in particular would have strengthened confidence in our simulated groundwater results. The other broader limitation is the lack of overall data about how different grazing systems explicitly control temporary and long-term groundwater storage. More work to assess this relationship—and refine its representation in process-based models—could be done using geophysical measurements designed to explicitly measure changes in groundwater storage before and after different grazing treatments. This type of field study could also potentially improve the representation of this relationship in models like SWAT. Given that the SWAT model did not support the significance of the anticipated benefits to groundwater, more field data collection or the use of remote sensing data as a proxy for groundwater (e.g., Danvir et al., 2018) is likely necessary to justify investment in rotational grazing specifically for groundwater capture.
Results from the RPM showed that pasture biomass was consistently higher in rotational scenarios, supporting the purported benefits of rotational grazing management in terms of forage production. Our tests of rotation among 2–10 pastures showed that rotation among higher numbers of pastures tended to increase forage biomass. At higher stocking rates, however, this increase in average biomass came at a large cost to animal performance. This apparent tradeoff arose in the model because forage availability in the one pasture being grazed was insufficient to meet the animals' energetic needs while other pastures were left completely ungrazed; Supplementary Figure 4 shows that grazed pastures in rotational scenarios were frequently grazed to very low biomass, while biomass in rested pastures was high but unavailable to the animals. At high stocking rates, the higher average biomass across pastures in the rotational scenarios was largely an outcome of less forage being consumed overall, possibly in addition to the stimulative effect of high-intensity grazing on forage growth (McNaughton, 1979).
Because it is a model designed to be applied over large areas and with lightweight data requirements, RPM is limited in several ways that may obscure its representation of rotational grazing management. First, while the model assumes that increased stocking density automatically increases the level of defoliation of all plants, at certain levels an increase in stocking density may simply increase the likelihood that any particular plant will be defoliated (Teague et al., 2008). Second, one potential driver of the beneficial impacts of rotation was not considered here: many anecdotal reports of increased ranch productivity with rotational grazing include improved pasture composition (Grissom and Steffens, 2013). Parameterizing RPM to simulate multiple pasture species, while possible, constitutes a heavy data burden. The model necessitates the abstraction of a single pasture or property, which in reality contains a multitude of forage plants differing in availability, palatability, nutritive content, and maturity, to a single point-based estimate of forage biomass and quality. This level of abstraction is reasonable given the need to apply analysis tools rapidly and over broad spatial scales, but it leaves out some heterogeneity that may underlie managers' power to enhance productivity (Barnes and Hild, 2013; Fuhlendorf et al., 2017).
For example, managers who report improved forage productivity after implementing rotational grazing likely do not follow the fixed, monthly rotation intervals that our simplified experimental setup required. Instead, managers who achieve improved ranch-level productivity and resilience tend to adopt flexible, adaptive management strategies that are tailored to the specific conditions and management goals for the property that they manage (Ortega-S et al., 2013). A substantial body of empirical literature suggests that total stocking density and the timing of defoliation relative to precipitation are the primary drivers of the impact of livestock grazing on productivity (Vermeire et al., 2008). By skillful manipulation of these levers, managers may achieve dramatic increases in forage productivity. However, rotational grazing per se does not necessarily connote optimal manipulation of stocking density and timing of grazing relative to precipitation.
The simulated RPM results with respect to animal diet sufficiency were more varied than the forage productivity results. The results given here, though driven by a highly simplified representation of rotational grazing management, show that rotation can under some circumstances enhance animal performance, while hampering it under other conditions. While rotational scenarios generally had higher biomass than scenarios including continuous grazing, it also tended to lower animal performance if pushed outside a certain management regime. Indeed, as mentioned above, some of the benefits of rotation to pasture biomass were driven by the inability of the animals to meet their nutritional needs when concentrated into very small pastures. The RPM results indicating strong and consistent impacts of total stocking rate on forage- and animal-related outcomes, alongside the inconsistencies in the difference between rotational and continuous scenarios, confirm that total stocking rate and precipitation are more powerful drivers of productivity than rotational grazing per se.
Many of the same caveats discussed in relation to biomass productivity apply to simulated animal diet sufficiency results as well. It is difficult to simulate adaptive management behavior in an experimental setting, which is likely to impact not only simulated biomass productivity but also simulated animal diet sufficiency. For example, our model exhibited a cost to animals at higher stocking rates that would likely be corrected by an observant manager before widespread costs in the form of livestock nutrient deficiencies or mortality were ever realized. Implementing this kind of judgment and experience-based decision-making in a simplified model is difficult and likely subjective (e.g., may vary from manager to manager, be dependent on risk tolerance, etc.).
Despite the limitations of our simplified and rigid approach, a strength of our modeling analysis is that other factors which are known to mediate the impact of management on forage biomass, such as pasture species composition, timing of defoliation relative to precipitation, topographical heterogeneity, and manager behavior (Briske et al., 2008), were excluded from analysis. Our highly simplified method shows that even under the most rigorously controlled experimental setting, the impacts of rotation are implementation dependent, with the implication that there is at least some (albeit limited) operating room where rotational grazing can modestly benefit biomass and diet sufficiency.
The impact investment literature reviewed for this study is generally premised on benefits associated with conversion from continuous to rotational grazing. Given this presumption, the mixed results of the modeling presented here, combined with the findings of our academic literature review, should give pause to those seeing large promise in impact investment for rangelands. For hydrologic benefits, the model we tested did not simulate those commonly cited as justification for impact investment after the institution of a rotational grazing systems. In the case of RPM, our modeling did show real but modest benefits in a limited range of management practices, while exhibiting stark negative outcomes for animal performance in others. For both categories of services, our results highlight the potential for tradeoffs that do not appear to be fully appreciated in impact investment literature to date. For instance, the highlighted (inverse) link between surface runoff and ET indicates that increasing the consumptive water use for biomass gain has a potentially significant impact on surface water delivery to downstream users and for the benefit of aquatic habitat. Our results further highlight trade-offs in which aspects of the water balance can be enhanced at the same time, in particular they show the challenges of simultaneously increasing ET (for forage production), increasing groundwater capture, and maintaining surface water delivery. Although the magnitudes of impacts for key modeled outcomes varies significantly, our results make clear that realizing certain desired benefits may come at a cost to others.
However, while we feel our models are indeed capturing some fundamental trade-offs, it is also quite possible that the science—as encapsulated in the accessible models we used—is inadequate to evaluate the subtle benefits of rangeland investment, nor represent more nuanced management practices that would actually be applied under impact investment (see below). Importantly, while we can provide context, this study alone cannot partition between true negligible effects vs. inadequacy of the models. Yet understanding model outcomes and capabilities remains important, because models are likely to be relied upon at some stage of investment evaluation, perhaps in tandem with other methods such as remote sensing and more extensive field data collection. We do not believe it is likely these latter methods will fully supplant model-based assessment due to combinations of cost, scalability, and challenges in extracting signal through noise on shorter spatial and time scales—but blending the approaches by using models to guide data collection or using additional data to constrain models may provide a better evaluation toolkit.
Impact investment in rangelands has the potential to fill an unmet demand for capital and provide additional resources to incentivize the management of intact rangeland ecosystems—an effort which process-based modeling can contribute to in substantive and important ways. These findings do not suggest there is no promising path forward for investment in rangelands to protect valuable ES and provide financial benefits to landowners. Rather, they imply that the interventions, benefit streams, and evaluation methodologies need to be more precisely defined and also evaluated against each other. As impact investors consider next steps, we suggest they would benefit from developing investment and evaluation strategies that more fully incorporate the mixed findings and empirical challenges within the significant body of scientific literature examining rotational grazing. Based on the demonstrated shortcomings of existing tools to clearly link rotational grazing with several key benefits, we suggest that impact investment could create more targeted and quantifiable outcomes if impact investment strategy remains more agnostic as to the specific type of grazing system employed to generate these outcomes, focusing instead on incentivizing owners to manage adaptively for scientifically-backed outcomes.
This is not the first study to call in to question the need for an emphasis beyond rotational vs. continuous grazing management and is not likely to be the last. Teague et al. (2008) outline the flawed nature of the entire contrast built around rotational vs. continuous grazing management, arguing that focus should rather be placed on adaptive management grounded in planning, flexibility in stocking density, monitoring of grazing levels, and avoidance of grazing during critical points in the season, among others. Hinging investment specifically on the superiority of rotational grazing neglects potentially more meaningful emphasis on good and objective-oriented management. By better incorporating these opportunities in to impact investment strategy, our hope is that rangeland owners and investors will be well-prepared to work collaboratively to further protect these important systems and the diversity of ES they provide.
The raw data supporting the conclusions of this manuscript will be made available by the authors, without undue reservation, to any qualified researcher.
BG, VK, and RC-K conceived the research. AK built the original model for the Clear Creek Watershed and the nested SWAT model capturing Ucross and represent grazing scenarios. BG refined and extended these models to evaluate the selected outcomes. VK and RC-K created the RPM model and VK developed and ran the RPM model for the Ucross property. RR advised the project and provided expert input for the RPM model. BB advised and structured later stages of the research and manuscript development. BG led writing of the manuscript, with contributions from VK and BB. RC-K, RR, and AK provided significant input and review.
BG, VK, RC-K, and BB were funded by a grant from the Ishiyama Foundation. AK was funded by the Ucross High Plains Stewardship Initiative.
RC-K is an author on this publication and serves as an editor for the special collection to which this article has been submitted. BG's family is active in the Wyoming ranching community, including a role in management of the Ucross study site from 2000 to 2008.
The remaining authors declare that the research was conducted in the absence of any commercial or financial relationships that could be construed as a potential conflict of interest.
The authors would like to thank the following individuals for their time and thoughtfulness: Leon Szeptycki for guiding questions, provisioning of funding, and thoughtful review, P. James-Dennedy Frank for guidance on technical components of SWAT, Todd Graham for sharing data from the Ucross property, Charlie Bettigole for introductions, data, and feedback, Michelle Downey for introductions, data, and feedback, Sabrina Szeto for assistance with data, and Jennie Muir-Gordon for providing expert input.
The Supplementary Material for this article can be found online at: https://www.frontiersin.org/articles/10.3389/fsufs.2019.00077/full#supplementary-material
1. ^Wyoming Water Development Office Wyoming State Water Plan. Available online at: http://waterplan.state.wy.us/waterfacts.html (accessed March 11, 2019).
2. ^Ucross Foundation: Ranch History. Available online at: http://www.ucrossfoundation.org/land-stewardship/ucross-ranch/ (accessed December 3, 2018).
Almendinger, J. E., and Murphy, M. (2005). Land-Use Change and Agricultural Practices in the Willow River Watershed, Western Wisconsin, 1992-2004. Science Museum of Minnesota, 26.
AlphaMundi SAIF (2010). Advisory Solutions for People and Planet Presentation at Impact Finance Luncheon Market Overview at the ISAG Forum Geneva, 18 February 2011. Available online at: http://isa-gva.org/wp-content/uploads/2011/02/AlphaMundi-2011.02.18-ISAG1.pdf (accessed November 19, 2018).
Arnold, J. G., Moriasi, D. N., Gassman, P. W., Abbaspour, K. C., White, M. J., Srinivasan, R., et al. (2012). SWAT: model use, calibration, and validation. Trans. ASABE 55, 1491–1508. doi: 10.13031/2013.42256
Bankes, S. (1993). Exploratory modeling for policy analysis. Operat. Res. 41, 435–449. doi: 10.1287/opre.41.3.435
Barnes, M., and Hild, A. (2013). Foreword: strategic grazing management for complex creative systems. Rangelands 35, 3–5. doi: 10.2111/RANGELANDS-D-13-00052.1
Barnes, M., and Howell, J. (2013). Multiple-paddock grazing distributes utilization across heterogeneous mountain landscapes: a case study of strategic grazing management. Rangelands 35, 52–61. doi: 10.2111/RANGELANDS-D-13-00019.1
Beetz, A., and Rinehart, L. (2010). The National Sustainable Agriculture Information Service, 1–12. Available online at: www.attra.ncat.org
Brest, P., and Born, K. (2013). When can impact investing create real impact. Stanford Soc. Innov. Rev. 11, 22–31.
Briske, D. D., Derner, J. D., Brown, J. R., Fuhlendorf, S. D., Teague, W. R., Havstad, K. M., et al. (2008). Rotational grazing on rangelands: reconciliation of perception and experimental evidence. Rangel. Ecol. Manag. 61, 3–17. doi: 10.2111/06-159R.1
Brown, J., and MacLeod, N. (2011). A site-based approach to delivering rangeland ecosystem services. Rangeland J. 33, 99–108. doi: 10.1071/RJ11006
Cameron, D. R., Marty, J., and Holland, R. F. (2014). Whither the Rangeland?: protection and conversion in California's Rangeland ecosystems. PLoS ONE 9:e103468. doi: 10.1371/journal.pone.0103468
Cheatum, M., Casey, F., Alvarez, P., and Parkhurst, B. (2011). Payments for Ecosystem Services: A California Rancher Perspective. Washington, DC: Nicholas Institute for Environmental Policy Solutions; Duke University.
Corson, M. S., Rotz, C. A., Skinner, R. H., and Sanderson, M. A. (2007). Adaptation and evaluation of the integrated farm system model to simulate temperate multiple-species pastures. Agric. Syst. 94, 502–508. doi: 10.1016/j.agsy.2007.01.003
Costin, A. (1980). Runoff and soil and nutrient losses from an improved pasture at Ginninderra, Southern Tablelands, New South Wales. Aust. J. Agric. Res. 31:533. doi: 10.1071/AR9800533
Culp, P., Bayon, R., Scott, J., and Melton, T. (2015). Investing for Impact in the Colorado River Basin With support from the Walton Family Foundation. Available online at: http://encouragecapital.com/wp-content/uploads/2015/09/Liquid-Assets-Full-Report-Web1.pdf (accessed March 13, 2019).
Curtis, B., Kellner, M. I., and Over, J. (1992). Process modeling. Commun. ACM 35, 75–90. doi: 10.1145/130994.130998
Dadkhah, M., and Gifford, G. F. (1980). Influence of vegetation, rock cover, and trampling on infiltration rates and sediment production 1. J. Am. Water Resour. Assoc. 16, 979–986. doi: 10.1111/j.1752-1688.1980.tb02537.x
Danvir, R., Simonds, G., Sant, E., Thacker, E., Larsen, R., Svejcar, T., et al. (2018). Upland bare ground and riparian vegetative cover under strategic grazing management, continuous stocking, and multiyear rest in new Mexico mid-grass prairie. Rangelands 40, 1–8. doi: 10.1016/j.rala.2017.12.004
Derner, J. D., and Hart, R. H. (2007). Grazing-induced modifications to peak standing crop in northern mixed-grass prairie. Rangeland Ecol. Manage. 60, 270–276. doi: 10.2111/1551-5028(2007)60[270:GMTPSC]2.0.CO;2
Derner, J. D., Hart, R. H., Smith, M. A., and Waggoner, J. W. (2008). Long-term cattle gain responses to stocking rate and grazing systems in northern mixed-grass prairie. Livest. Sci. 117, 60–69. doi: 10.1016/j.livsci.2007.11.011
Freer, M., Moore, A. D., and Donnelly, J. R. (1997). GRAZPLAN: decision support systems for Australian grazing enterprises—II. The animal biology model for feed intake, production and reproduction and the GrazFeed DSS. Agricultural Systems, 54, 77–126.
Fuhlendorf, S. D., Fynn, R. W., McGranahan, D. A., and Twidwell, D. (2017). “Heterogeneity as the basis for rangeland management,” in Rangeland Systems, ed D. D. Briske (Cham: Springer), 169–196.
Gage, A. M., Olimb, S. K., and Nelson, J. (2016). Plowprint: tracking cumulative cropland expansion to target grassland conservation. Great Plains Res. 26, 107–116. doi: 10.1353/gpr.2016.0019
Ganskopp, D., and Bohnert, D. (2001). Nutritional dynamics of 7 northern Great Basin grasses. J. Range Manage. Arch., 54, 640–647. doi: 10.2458/azu_jrm_v54i6_ganskopp
Glick, H. B., Routh, D., Bettigole, C., Seegmiller, L., Kuhn, C., and Oliver, C. D. (2016). Modeling the effects of horizontal positional error on classification accuracy statistics. Photogramm. Eng. Remote Sens. 82, 789–802. doi: 10.14358/PERS.82.10.789
Goldstein, J. H., Presnall, C. K., López-Hoffman, L., Nabhan, G. P., Knight, R. L., Ruyle, G. B., et al. (2011). Beef and beyond: paying for ecosystem services on western US rangelands. Rangelands 33, 4–13. doi: 10.2111/1551-501X-33.5.4
Goodloe, S. (1969). Short duration grazing in Rhodesia. J. Range Manag. 22:369. doi: 10.2307/3895844
Graham, T. (2014). A Voice of the New Agrarianism. Available online at: http://ranchadvisory.com/images/uploads/Ucross_Ranch__Todd_Graham.pdf (accessed February 2, 2018).
Grissom, G., and Steffens, T. (2013). Case study: adaptive grazing management at Rancho Largo Cattle Company. Rangelands 35, 35–44. doi: 10.2111/RANGELANDS-D-13-00015.1
Havstad, K. M., Peters, D. P. C., Skaggs, R., Brown, J., Bestelmeyer, B., Fredrickson, E., et al. (2007). Ecological services to and from rangelands of the United States. Ecol. Econ. 64, 261–268. doi: 10.1016/j.ecolecon.2007.08.005
Heitschmidt, R. K., Dowhower, S. L., and Walker, J. W. (1987). Some effects of a rotational grazing treatment on quantity and quality of available forage and amount of ground litter. J. Range Manage. (1987) 40, 318–321. doi: 10.2307/3898728
Held, L. (2017). Impact Investors Flock to Sustainable Agriculture. GreenBiz. Available online at: https://www.greenbiz.com/article/impact-investors-flock-sustainable-agriculture (accessed March 13, 2019).
Hepworth, K. W., Test, P. S., Hart, R. H., Waggoner, J. W., and Smith, M. A. (1991). Grazing systems, stocking rates, and cattle behavior in Southeastern Wyoming. J. Range Manag. 44:259. doi: 10.2307/4002954
Hobbs, N. T., Schimel, D., Owensby, C. E., and Ojima, D. S. (1991). Fire and grazing in the tallgrass prairie: contingent effects on nitrogen budgets. Ecology 72, 4, 1374–1382. doi: 10.2307/1941109
Hodgson, J. (1979). Nomenclature and definitions in colorimetry. Grass Forage Sci. 34, 11–17. doi: 10.1111/j.1365-2494.1979.tb01442.x
Hodgson, R. E., Grunder, M. S., Knott, J. C., and Ellington, E. V. (1934). A comparison of rotational and continuous grazing of pastures in Western Washington. Wash. Agr. Exp. Stn. Bull. 294, l–36.
Huntsinger, L., and Hopkinson, P. (1996). Sustaining rangeland landscapes: a social and ecological process. J. Range Manag. 49, 167–173. doi: 10.2307/4002689
Kamstra, L. D. (1973). Seasonal changes in quality of some important range grasses. J. Range Manage. Arch. 26, 289–291. doi: 10.2307/3896580
Krause, P., Boyle, D. P., and Bäse, F. (2005). Comparison of different efficiency criteria for hydrological model assessment. Adv. Geosci. 5, 89–97. doi: 10.5194/adgeo-5-89-2005
Kuhn, C., Bettigole, C., Glick, H. B., Seegmiller, L., Oliver, C. D., and Raymond, P. (2017). Patterns in stream greenhouse gas dynamics from mountains to plains in northcentral Wyoming. J. Geophys. Res. Biogeosci. 122, 2173–2190. doi: 10.1002/2017JG003906
Lang, K., Humphreys, J., Rodinciuc, A., Baker, S., Kron, J., and Pearce, A. (2017). Impact Investing in Sustainable Food and Agriculture Across Asset Classes. Available online at: http://www.trilliuminvest.com/wp-content/uploads/2017/05/Investing-in-Sustainable-Food-and-Agriculture.pdf (accessed November 30, 2018).
Lang, R. D. (1979). The effect of ground cover on surface runoff from experimental plots [soil erosion]. J. Soil Conserv. Serv. New South Wales 35, 108–114.
Maczko, K., Tanaka, J. A., Breckenridge, R., Hidinger, L., Heintz, H. T., Fox, W. E., et al. (2011). Rangeland ecosystem goods and services: values and evaluation of opportunities for ranchers and land managers. Rangelands 33, 30–37. doi: 10.2307/41319863
McNaughton, S. J. (1979). Grazing as an optimization process: grass-ungulate relationships in the Serengeti. Am. Nat. 113, 691–703. doi: 10.1086/283426
McNaughton, S. J. (1984). Grazing lawns: animals in herds, plant form, and coevolution. Am. Nat. 124, 863–886. doi: 10.1086/284321
Montalto, F., Behr, C., Alfredo, K., Wolf, M., Arye, M., and Walsh, M. (2007). Rapid assessment of the cost-effectiveness of low impact development for CSO control. Landsc. Urban Plan. 82, 117–131. doi: 10.1016/j.landurbplan.2007.02.004
Morgan, D. O. (1933). The effect of heavy stocking on the worm burden under a system of rotational grazing. J. Helminthol. 11, 169–180. doi: 10.1017/S0022149X00001838
Moriasi, D. N., Arnold, J. G., Van Liew, M. W., Bingner, R. L., Harmel, R. D., and Veith, T. L. (2007). Model evaluation guidelines for systematic quantification of accuracy in watershed simulations. Trans. ASABE 50, 885–900. doi: 10.13031/2013.23153
Mulholland, B., and Fullen, M. A. (1991). Cattle trampling and soil compaction on loamy sands. Soil Use Manage. 7, 189–193. doi: 10.1111/j.1475-2743.1991.tb00873.x
Murphy, S. R., Lodge, G. M., and Harden, S. (2004). Surface soil water dynamics in pastures in northern New South Wales. 3. Evapotranspiration. Aust. J. Exp. Agric. 44:571. doi: 10.1071/EA03041
NatureVest and EKO Asset Management Partners. (2014). Investing in Conservation: A Landscape Assessment of an Emerging Market. The Nature Conservancy.
Neitsch, S. L., Arnold, J. G., Kiniry, J. R., and Williams, J. R. (2011). Soil and Water Assessment Tool Theoretical Documentation Version 2009. Available onlin at: https://swat.tamu.edu/media/99192/swat2009-theory.pdf (accessed November 20, 2018).
Norton, B. E., Barnes, M., and Teague, R. (2013). Grazing management can improve livestock distribution: increasing accessible forage and effective grazing capacity. Rangelands 35, 45–51. doi: 10.2111/RANGELANDS-D-13-00016.1
NRCS (2019). Ecological Site Description System. Available online at: https://esis.sc.egov.usda.gov/ESDReport/fsReport.aspx?id=R058BY166WY&rptLevel=general&approved=yes&rptType==regular (accessed March 14, 2019).
Nyeko, M. (2015). Hydrologic modelling of data scarce basin with SWAT model: capabilities and limitations. Water Resour. Manag. 29, 81–94. doi: 10.1007/s11269-014-0828-3
Ortega-S, J. A, and Lukefahr, S. D. (2013). Optimum stocking rate, monitoring, and flexibility. Rangelands Arch. 35, 5, 22–27. doi: 10.2111/RANGELANDS-D-13-00021.1
Owens, L. B., and Shipitalo, M. J. (2009). Runoff quality evaluations of continuous and rotational over-wintering systems for beef cows. Agric. Ecosyst. Environ. 129, 482–490. doi: 10.1016/j.agee.2008.11.003
Park, J.-Y., Ale, S., and Teague, W. R. (2017). Simulated water quality effects of alternate grazing management practices at the ranch and watershed scales. Ecol. Modell. 360, 1–13. doi: 10.1016/j.ecolmodel.2017.06.019
Parton, W. J. (1996). “The CENTURY model,” in Evaluation of Soil Organic Matter Models, eds D. S. Powlson, P. Smith, and J. U. Smith (Berlin: Springer), 283–291.
Parton, W. J., Scurlock, J. M. O., Ojima, D. S., Gilmanov, T. G., Scholes, R. J., Schimel, D. S., et al. (1993). Observations and modeling of biomass and soil organic matter dynamics for the grassland biome worldwide. Glob. Biogeochem. Cycles, 7, 785–809.
Pons, E., Long, M.-A., and Pomares, R. (2013). Promoting Sustainable Food Systems through Impact Investing. Available online at: https://scholar-google-com.stanford.idm.oclc.org/scholar?q=Pons%2C%20Elena%2C%20Maud-Alison%20Long%2C%20and%20Raul%20Pomares.%202013.%20Promoting%20Sustainable%20Food%20System%20Through%20Impact%20Investing.%20San%20Francisco (accessed November 13, 2018).
Pronger, J., Campbell, D. I., Clearwater, M. J., Rutledge, S., Wall, A. M., and Schipper, L. A. (2016). Low spatial and inter-annual variability of evaporation from a year-round intensively grazed temperate pasture system. Agric. Ecosyst. Environ. 232, 46–58. doi: 10.1016/j.agee.2016.07.011
Reeves, M. C., Krebs, M., Leinwand, I., Theobald, D. M., and Mitchell, J. E. (2018). United States Department of Agriculture Rangelands on the Edge: Quantifying the Modification, Fragmentation, and Future Residential Development of U.S. Rangelands. Available online at: https://www.fs.fed.us/rm/pubs_series/rmrs/gtr/rmrs_gtr382.pdf (accessed November 30, 2018).
Resnik, J., Wallace, G., Brunson, M., and Mitchell, J. (2006). Open spaces, working places. Rangelands 4–9.
Rotz, C. A., Corson, M. S., Chianese, D. S., Montes, F., Hafner, S. D., and Coiner, C. U. (2013). The Integrated Farm System Model: Reference Manual, Version 4.0 [Internet] (Pasture Systems and Watershed Management Research Unit, Agricultural Research Service, USDA). Available online at: http://afrsweb.usda.gov/SP2UserFiles/Place/19020500/Reference%20Manual.pdf
Sayre, N. (2001). The New Ranch Handbook: A Guide to Restoring Western Rangelands. Available online at: www.quiviracoalition.org (accessed November 19, 2018).
Sheridan, T. E. (2007). Embattled ranchers, endangered species, and urban sprawl: the political ecology of the New American West. Annu. Rev. Anthropol. 36, 121–138. doi: 10.1146/annurev.anthro.36.081406.094413
Stehr, A., Debels, P., Arumi, J. L., Romero, F., and Alcayaga, H. (2009). Combining the Soil and Water Assessment Tool (SWAT) and MODIS imagery to estimate monthly flows in a data-scarce Chilean Andean basin. Hydrol. Sci. J. 54, 1053–1067. doi: 10.1623/hysj.54.6.1053
Sterling, S. M., Ducharne, A., and Polcher, J. (2013). The impact of global land-cover change on the terrestrial water cycle. Nat. Clim. Chang 3, 385–390. doi: 10.1038/nclimate1690
Teague, R., Provenza, F., Kreuter, U., Steffens, T., and Barnes, M. (2013). Multi-paddock grazing on rangelands: why the perceptual dichotomy between research results and rancher experience? J. Environ. Manage. 128, 699–717. doi: 10.1016/j.jenvman.2013.05.064
Teague, W. R., Dowhower, S. L., Baker, S. A., Haile, N., DeLaune, P. B., and Conover, D. M. (2011). Grazing management impacts on vegetation, soil biota and soil chemical, physical and hydrological properties in tall grass prairie. Agric. Ecosyst. Environ. 141, 310–322. doi: 10.1016/j.agee.2011.03.009
Teague, W. R., Provenza, F., Norton, B., Steffens, T., Barnes, M., Kothmann, M., et al. (2008). “Benefits of multi-paddock grazing management on rangelands:limitations of experimental grazing research and knowledge gaps,” in Grasslands: Ecology, Management and Restoration, ed H. G. Schroder (Hauppauge, NY: Nova Science Publishers), 41–80.
Thurow, T. L. (1991). Chapter 6 Hydrology and Erosion. Available online at: http://cnrit.tamu.edu/rlem/textbook/Chapter6.htm (accessed January 15, 2019).
Thurow, T. L., Blackburn, W. H., and Taylor, C. A. Jr. (1988). Infiltration and interrill erosion responses to selected livestock grazing strategies, Edwards Plateau, Texas. J. Range Manage. 41, 296–302. doi: 10.2307/3899382
United States Department of Agriculture National Resources Conservation Service (USDA NRCS). (2014). Agricultural Conservation Easement Program. Available online at: http://www.nrcs.usda.gov/wps/portal/nrcs/main/national/programs/easements/acep/
Vermeire, L. T., Heitschmidt, R. K., and Haferkamp, M. R. (2008). Vegetation response to seven grazing treatments in the Northern Great Plains. Agric. Ecosyst. Environ. 125, 111–119. doi: 10.1016/j.agee.2007.12.003
Warren, S. D., Thurow, T. L., Blackburn, W. H., and Garza, N. E. (1986). The influence of livestock trampling under intensive rotation grazing on soil hydrologic characteristics. J. Range Manage. 39, 491–495. doi: 10.2307/3898755
Wetzel, W. C., Lacher, I. L., Swezey, D. S., Moffitt, S. E., and Manning, D. T. (2012). Analysis reveals potential rangeland impacts if Williamson Act eliminated. Calif. Agric. 66, 131–136. doi: 10.3733/ca.v066n04p131
While, C. (2008). Revolution on the Range: The Rise of a New Ranch in the American West (Island Press/Shearwater Books). Available online at: https://books.google.com/books?hl=en&lr=&id=kMuZHQX_UxUC&oi=fnd&pg=PR4&dq=White,+C.+2008.+Revolution+on+the+range:+the+rise+of+a+new+ranch+in+the+American+West.+Washington,+DC,+USA:+Island+Press.+248+p.&ots=y8uLHMHt1m&sig=PF8xgbu5GRpvUaSvFRLTAReQIy8#v=one (accessed November 19, 2018).
Keywords: impact investment, ecosystem services, grazing management, forage productivity, water availability
Citation: Gordon BL, Kowal VA, Khadka A, Chaplin-Kramer R, Roath R and Bryant BP (2019) Existing Accessible Modeling Tools Offer Limited Support to Evaluation of Impact Investment in Rangeland Ecosystem Services. Front. Sustain. Food Syst. 3:77. doi: 10.3389/fsufs.2019.00077
Received: 03 May 2019; Accepted: 06 September 2019;
Published: 24 September 2019.
Edited by:
Eike Luedeling, University of Bonn, GermanyReviewed by:
Neelendra K. Joshi, University of Arkansas, United StatesCopyright © 2019 Gordon, Kowal, Khadka, Chaplin-Kramer, Roath and Bryant. This is an open-access article distributed under the terms of the Creative Commons Attribution License (CC BY). The use, distribution or reproduction in other forums is permitted, provided the original author(s) and the copyright owner(s) are credited and that the original publication in this journal is cited, in accordance with accepted academic practice. No use, distribution or reproduction is permitted which does not comply with these terms.
*Correspondence: Beatrice L. Gordon, Ymxnb3Jkb25Ac3RhbmZvcmQuZWR1
Disclaimer: All claims expressed in this article are solely those of the authors and do not necessarily represent those of their affiliated organizations, or those of the publisher, the editors and the reviewers. Any product that may be evaluated in this article or claim that may be made by its manufacturer is not guaranteed or endorsed by the publisher.
Research integrity at Frontiers
Learn more about the work of our research integrity team to safeguard the quality of each article we publish.