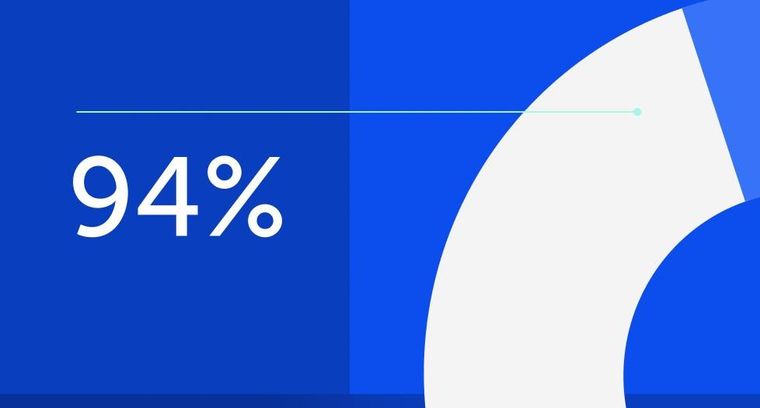
94% of researchers rate our articles as excellent or good
Learn more about the work of our research integrity team to safeguard the quality of each article we publish.
Find out more
MINI REVIEW article
Front. Sustain. Food Syst., 17 May 2019
Sec. Sustainable Food Processing
Volume 3 - 2019 | https://doi.org/10.3389/fsufs.2019.00038
This article is part of the Research TopicCellular Agriculture: Biotechnology for Sustainable FoodView all 11 articles
For decades, two-dimensional cell culture has been regarded as a major tool in cellular and molecular biology due to its simplicity, reproducibility and reliable nature. However, it is now recognized that 2D cell culture underrepresents the in vivo environment of living cells. The development and use of 3D scaffolds and biomaterials provide researchers an ability to more closely mimic the in vivo environment. However, many biomaterials are of animal origin, leading to variability, environmental and ethical concerns. Here we present three animal-free scaffolds: decellularized plant tissue, chitin/chitosan and recombinant collagen. Decellularized plant tissue provides a wide array of structures with varying biochemical, topographical and mechanical properties; chitin/chitosan-based scaffolds have shown synergistic bactericidal effects and improved cell-matrix interaction; and lastly, recombinant collagen has the potential to closely resemble native tissue, as opposed to the other two. These benefits, alongside potential scalability and tunability, open the door to applications beyond the biomedical realm, such as innovations in cellular agriculture and future food technologies.
Since the early 20th century, two-dimensional cell culture has been regarded as a reliable, simple and reproducible study of cellular behavior (Jedrzejczak-Silicka, 2017). However, a direct comparison between 2D and 3D cell culture is challenging due to dramatic differences in the cellular environment. In vivo, cells interact closely with other cells, a complex array of physical forces/stimuli, and biologically active extracellular matrix (ECM). In contrast, 2D cell culture is performed on a substrate with drastically different mechanical and biochemical properties (Figure 1). Comparisons between 2D and 3D cell culture have revealed significant differences in proliferation, differentiation, drug toxicity resistance, gene expression and protein synthesis (Huyck et al., 2012; Antoni et al., 2015; Ravi et al., 2015; Cavo et al., 2016; Fang and Eglen, 2017; Riedl et al., 2017). In order to overcome the gap between 2D cell culture and the 3D environment sensed by the cell in vivo, a plethora of natural and synthetic polymers, recombinant proteins, ceramics, and metal-composite scaffolds have been developed and reviewed previously (Carletti et al., 2011; O'Brien, 2011; Turnbull et al., 2018). Yet, in order to produce scaffolds with similar characteristics to those of the ECM, animal-derived polymers such as collagen are often considered as the gold standard. However, the dependence on animals have made them undesirable due to variability (Shoseyov et al., 2013), environmental (Kraham, 2017), and ethical concerns (Verbeke and Viaene, 2000). Moreover, the scalability and consumer acceptance of cultured meat products will rely on a disconnect from animal sources. Research into animal-free scaffolds has emerged as a potential source for consistent, chemically defined and low-cost materials.
Figure 1. Cells on 3D porous substrate vs. 2D substrate. Cells on 3D porous substrates can be found on the surface and interior, whereas cells on Petri dishes are bound to a 2D environment. Proteins naturally found in animal serum and those synthesized by cells adsorb to the surface of the material and facilitate cell adhesion. Through focal adhesion, adherent cells are able to interact with the substrate; therefore, the properties of the material (e.g., Stiffness) can influence the cell's behavior and morphology.
Synthetic or natural animal-free polymers such as cellulose (Huber et al., 2012; Hickey et al., 2018), chitin/chitosan (Jayakumar et al., 2011), alginate (Lee and Mooney, 2012), recombinant silk (Widhe et al., 2010), PLA (Serra et al., 2013), and PCL (Li et al., 2017) provide low cost, consistent and tunable scaffolds. In this concise review we have chosen to focus on chitin/chitosan, cellulose (bacterial and plant), and recombinant collagen and their use in tissue engineering and potential applications in cellular agriculture. The biomaterials chosen here meet the criteria for cellular agriculture applications, such as animal-free, abundant, biocompatible, versatile, provide nutritional benefits, and are already part of commonly consumed products. However, we recognize that many other biomaterials and scaffolding approaches do exist and, as above, we refer the reader to other topical reviews for a deeper examination of those strategies (Carletti et al., 2011; O'Brien, 2011; Derakhshanfar et al., 2018).
As tissue engineering and regenerative medicine continues to expand with promising results, the potential for novel food applications has arisen due to the similarity in techniques and approaches. Although meat/tissue has proven to be difficult to replicate in vitro due to its complex composition (muscle, nerve, water, minerals, growth factors, hormones, and Extracellular matrix proteins) (Listrat et al., 2016), the native structure of foods such as mushroom (Jo-Feeney et al., 2014) and jackfruit (John et al., 1992) have the potential to contribute the expected palatable properties of meat. In addition to rheological properties, these foods contribute nutritional benefits, such as insoluble fiber (McDougall et al., 1996; Cheung, 2013).
As the most abundant polymer in nature (1.5 × 1012 tons of total biomass) and the main component of plants, cellulose, a 1-4β D-glucose polymer has shown great potential as scaffolding material due its low cost, versatility and overall biocompatibility (O'Sullivan, 1997; Klemm et al., 2005). Cellulose hydrogels (Isobe et al., 2018), composites (Huber et al., 2012; Johns et al., 2018), functionalized plant cellulose (Modulevsky et al., 2014; Fontana et al., 2017) and decellularized plant tissue (Modulevsky et al., 2014; Gershlak et al., 2017) have been developed. This in turn shows the versatility of cellulose. Moreover, cellulose and its derivatives (e.g., Methylcellulose and 6-carboxycellulose) have been functionalized and blended with other materials to improve its mechanical, biological and chemical properties (Novotna et al., 2013; Thirumala et al., 2013; Fontana et al., 2017). Cellulose as a biomaterial has been extensively reviewed previously (Kalia et al., 2011; Hickey and Pelling, 2019). This section will emphasize on decellularized plant tissue and bacterial cellulose.
It was shown that decellularized apple hypanthium (Figure 2) can be used as a substrate for 3D cell culture. HeLa cells, 3T3 fibroblast, and C2C12 murine myoblast proliferated throughout the 3D matrix (Modulevsky et al., 2014). In order to decellularized the tissue, a surfactant, in this case SDS, was used to create pores in the plant cell membrane, leading to the release of cellular components (Brown and Audet, 2008; Modulevsky et al., 2014). The mechanical properties of these scaffolds which are known to influence cell behavior, have also been altered through functionalization and crosslinking (Modulevsky et al., 2014) and further shown to resemble skeletal (Modulevsky et al., 2014; Hickey et al., 2018) and cardiac muscle tissue (Gershlak et al., 2017). However, cellulose based scaffolds do lack a wide array of mammalian biochemical cues; thus, biofunctionalization or coating with functional surface proteins may be required for specific cell lines, especially in a serum-free environment (Hayman et al., 1985; Courtenay et al., 2017; Johns et al., 2018). It was noted, however, that the viability of C2C12 cells was not affected by the bare cellulose scaffold when compared to collagen and gelatin coating (Hickey et al., 2018). Nonetheless, seeding efficiency has been shown to be greatly improved with surface coating and functionalization (Modulevsky et al., 2014; Fontana et al., 2017; Hickey et al., 2018).
Figure 2. Preparation of cellulose scaffold. Macroscopic appearance of a freshly cut apple hypanthium tissue (A) and the translucent scaffold biomaterial post decellularization and absent of all native apple cells or cell debris (B). H & E staining of cross sectioned decellularized cellulose scaffold (C). The cell walls thickness and the absence of native apple cells following decellularization are shown. The 3D acellular highly porous cellulose scaffold architecture clearly revealed by SEM (D). Scale bar: A–B = 2 mm, C–D = 100 μm. Reprinted from Modulevsky et al. (2016).
An advantage of decellularized plant tissue is the wide array of natural topographies that can be used to study cellular behavior and potentially mimic in vivo conditions without long and costly processing (Modulevsky et al., 2014, 2016; Fontana et al., 2017). By utilizing the topographical cues present in the vascularization of stems and leaves, guided cell alignment was noted (Fontana et al., 2017). In this case, cell alignment was likely due to the confinement of cells within the vascularization channels. Alignment in cell culture is a highly desirable characteristic, especially in musculoskeletal research. By inducing alignment, researchers try to mimic the physiological state of myoblast and myotubes (Zhao et al., 2009; Bettadapur et al., 2016). In comparison to synthetic microchannel development techniques, such as 3D printing (Tijore et al., 2018), soft lithography (Glawe et al., 2005) and photolithography (Lee et al., 2006), the decellularized vascular bundle of plants depict a low cost, highly accessible and easy to use material.
The vascularization of a decellularized spinach leaf was postulated as a way to overcome the 100–200 μm diffusion limitations of 3D scaffolds (Gershlak et al., 2017). Yet, it's still unclear if cells growing outside of the vascularization tracts can benefit from the circulation of nutrients. However, as of now, the need for vascularization in decellularized plant is not a requirement. Cells are able to grow throughout the porous decellularized apple hypanthium without developing a necrotic center (Modulevsky et al., 2014; Hickey et al., 2018). Yet, a necrotic center is likely to develop in very large scaffolds which may possibly be required in food applications. The porosity of the apple also supported angiogenesis when implanted in vivo (Modulevsky et al., 2016). This observation will not necessarily extrapolate to other decellularized plant scaffolds due to their underlying native tissue geometry which makes plant species/tissue choice important (Gershlak et al., 2017). Although decellularization is depicted as a simple biomaterial development method, it lacks the customizability of “bottom-up” approaches, such as that of cellulose nanofibril scaffolds and cellulose composites with varying porosity, biological, and mechanical characteristics (Khan et al., 2016; Courtenay et al., 2017; Courtenay et al., 2018).
Cellulose is not only found in the plant kingdom, but is also produced by certain strains of bacteria, such as Acetobacterspp. (Schramm and Hestrin, 1954; Jonas and Farah, 1998). Although plant and bacterial cellulose share an identical α-cellulose structure, bacterial cellulose possess greater crystallinity, degree of polymerization and water holding capacity (Esa et al., 2014; Moniri et al., 2017). These attributes have been invaluable in a wide array of applications, including medical (Petersen and Gatenholm, 2011; Fürsatz et al., 2018), cosmetics (Pacheco et al., 2018) and food (Shi et al., 2014). The food applications include cultural desserts such as Nata de coco; and functional properties such as gelling agent, stabilizer and thickener (Shi et al., 2014). Moreover, bacterial cellulose has been used to incur juiciness and chewiness in emulsified meats (Lin and Lin, 2004).
An advantage of bacterial cellulose is the wide array of carbohydrate-rich by-products that have been used for its production (e.g., Wheat thin stillage, waste fiber sludge, pullulan fermentation waste water, beer culture broth) (Ha et al., 2008; Cavka et al., 2013; Revin et al., 2018; Zhao et al., 2018) and the wide array of chemical modifications that can be introduced to further improve biocompatibility and mechanical properties (Kurniawan et al., 2012; Saska et al., 2012; Lopes et al., 2014; Ostadhossein et al., 2015). The biocompatibility, low cost and nutritional attributes make this material a potential candidate for in vitro meat production.
As the second most abundant polymer in nature, chitin is found in the exoskeleton of arthropods (e.g., crab and shrimp) and fungi (Percot et al., 2003; Deguchi et al., 2015). In this review, fungal chitin is of interest due to the animal-free nature. Although the chitin sources referenced throughout this section are either not disclosed or declared to be animal derived (likely due to abundance) there is currently no reason to believe that it can't be replaced with fungi chitin (Bierhalz et al., 2016).
Through alkaline deacetylation, chitin is turned into chitosan (Rodríguez-Vázquez et al., 2015). The degree of deacetylation of chitin leads to physical, chemical, and biological changes, such as interaction with cells directly or with glycoproteins and proteoglycans through ionic complexes. In addition to the interaction with glycoproteins and proteoglycans, chitosan's resemblance to glycosaminoglycans has the potential of regulating and modulating bioactive factors (Madihally and Matthew, 1999; Yang, 2011; Chicatun et al., 2013). Moreover, it was also shown that chitosan can be blended with other polymers to further improve the mechanical properties with the aim of resembling native tissue (Zakhem et al., 2012; Hajiabbas et al., 2015).
Chitosan has shown to be a desirable material in tissue engineering due to its biocompatibility (Tamura et al., 2011; Croisier and Jérôme, 2013), antibacterial properties (Benhabiles et al., 2012), and accelerated healing rate on skin wounds (Tchemtchoua et al., 2011). It has been shown that chitosan and chitosan oligosaccharides provide a synergistic bactericidal effect on planktonic bacteria and biofilms when combined with antibiotics such cloxacillin (Decker et al., 2005; Breser et al., 2018) and sulfamethoxazole (Tin et al., 2009). Chitin and chitosan alone portrayed a bacteriostatic effect on gram negative bacteria, Escherichia coli, Vibrio cholerae, Shigella dysenteriae, and Bacteroides fragilis (Benhabiles et al., 2012). The synergistic effect with antibiotics and overall bacteriostatic properties are a desirable attribute for applications in cellular agriculture; as antibiotic use decreases social acceptability (Karavolias et al., 2018), has the potential to cause long term health problems, and increase the development of antimicrobial resistance (Thanner et al., 2016). However, to our knowledge, the antimicrobial potential of these compounds in long term cell mammalian cell culture has yet to be tested or verified.
Often materials for medical applications are segregated into permanent or temporary. The degradation of chitosan by lysozymes found in the body can be controlled through the degree of deacetylation (Muzzarelli, 1997; Tomihata and Ikada, 1997; Rodríguez-Vázquez et al., 2015). Degradability is not necessarily an undesirable characteristic, as degradation rates can be controlled; and the by-products have the potential to provide neuroprotective (Pangestuti and Kim, 2010) and anti-inflammatory properties (Azuma et al., 2015; Kim, 2018). Furthermore, biodegradable hydrogels with controlled degradation rates are expected to be a temporary matrix for adherent cells. The objective is to match matrix deposition by cells with the degradation rate of the scaffold (Berthod et al., 2006; Bitar and Zakhem, 2014; Ren et al., 2018). This not only applies to medical applications, but also to potential applications for in vitro meat production. A temporary matrix can allow for cellular ECM deposition with the end of goal of obtaining a scaffold with characteristics similar to that of native tissue.
The structure of certain types of mushroom provide a mouthfeel and umami flavor which resembles that of meat, often perceived as a vegan alternative (Jo-Feeney et al., 2014). Moreover, the cell wall components of mushroom contain chitin, 1-3-alpha-D-gucans and mannans, which confer nutritional benefits, such as dietary fiber (Cheung, 2013; Fernandes et al., 2015). The antimicrobial and nutritional properties, alongside its animal-free nature and abundancy, make chitin/chitosan-based scaffolds a potential substrate for cellular agriculture applications.
The well-known and extensively studied extracellular matrix protein, collagen, is often derived from bovine (Chan et al., 2016), porcine (Smith et al., 2000), and murine (Isobe et al., 2012) sources. Collagen type I, a fibrillar heterotrimeric protein composed of two α1(I) chains and one α2(I) chain, has been produced in numerous forms, including porous hydrogels, composites and a number of substrates with topographical cues and varying mechanical properties (Rich and Crick, 1955, 1961; Vernon et al., 2005; Stein et al., 2009; Antoine et al., 2014; Wang et al., 2016; Wu et al., 2018). Yet, variability (e.g., age and physiological state of donor), potential pathogen transmission, and contaminants including cytokines and growth factors have been a concern for this animal derived product (BANFIELD, 1956; Kohn and Rollerson, 1960; Keefe et al., 1992; Badylak and Gilbert, 2008; Shoseyov et al., 2013).
In order to overcome these issues, genetic engineering has led to the development of transgenic organisms capable of synthesizing the desired amino acid repeats. Through the insertion of COL1A1 and COL1A2 genes, the repeating amino acid sequence, Gly-X-Y, can be translated and transcribed. In this case, the X and Y often correspond to proline and hydroxyproline, respectively (Stein et al., 2009; An et al., 2014; Shoseyov et al., 2014). The repeating amino acid sequence leads to the triple helical conformation and specific thermal stability of collagen (Rich and Crick, 1955; Bella et al., 1995; An et al., 2014).
The production of procollagen through recombinant methods has been observed in bacteria (An et al., 2014), mammalian cells (Geddis and Prockop, 1993), insect cell culture (Myllyharju et al., 1997), yeast (Olsen et al., 2001), and plants (Stein et al., 2009; Xu et al., 2011). The introduction of COL1A1 and COL1A2 genes encode for the amino acid sequence. Yet, the post translational modifications are fundamental in the production of collagen with similar mechanical and biochemical properties to that of native collagen found in vivo. Procollagen for in vivo and in vitro use has been produced in a tobacco plant capable of expressing COL1A1 and COL1A2 proteins, alongside post translational modification proteins localized in the vacuole: Prolyl 4-hydrolysase (PH-4) alpha, PH-4beta and Lysine hydroxylase (LH1-3). P4H acts on the proline residues leading to directionality and thermal stability, whereas LH1-3 plays a role in collagen fibril formation and stabilization (Pihlajaniemi et al., 1991; Ruotsalainen et al., 2006; Shoseyov et al., 2013).
Although the production of recombinant collagen has proven to be difficult in part due to the need for post translational modification machinery natively found in mammalian cells (Werkmeister and Ramshaw, 2012; An et al., 2014), a fibrillar protein with a similar melting point and overall chemical structure to collagen has been observed and isolated in microbes, such as Streptococcus pyogenes. The protein's properties have been attributed to the presence of collagen like proteins, scl 1 and scl 2 (Lukomski et al., 2000, 2001; Yu et al., 2014). The production of this collagen like protein lacks the different biochemical cues found in vivo due to the lack of post translational modification; yet, the “blank slate” and gene customizability can be an attractive property for customization (Peng et al., 2010; An et al., 2014; Yu et al., 2014).
In order to fulfill the demand for recombinant collagen, yield optimization has been a major target. Standardized comparison has been difficult to accomplish due to the properties of the final product which are influenced by the level and presence of post translational modification proteins. Collagen production in plants, more specifically, tobacco, has been considered to be the most promising. Production of up to 200 mg of recombinant human type I procollagen per kg of fresh leaves (20 g/L reported by Werkmeister and Ramshaw, 2012) has been achieved through the vacuole targeted enzymes and genes (Stein et al., 2009). The biocompatibility of procollagen from transgenic tobacco plants was shown in vitro and in vivo (Shilo et al., 2013; Willard et al., 2013). In vitro, an increase in cell proliferation of human epidermal keratinocytes was noted when compared to bovine collagen (Willard et al., 2013). Bacteria collagen (Peng et al., 2010) and recombinant collagen produced in yeast (Liu et al., 2007;2008) have also shown in vivo and in vitro biocompatibility.
Collagen type I is not the only ECM protein that has been produced recombinantly. Other types of collagen (e.g., Type II and III) (Myllyharju et al., 2000; Pakkanen et al., 2003; Ruottinen et al., 2008), tropoelastin (Martin et al., 1995), and fibronectin (Staunton et al., 2009) fragments have also been produced recombinantly.
Collagen production in transgenic tobacco plants, yeast and/or bacteria has the potential to alleviate issues encountered through the use of animal derived biomaterials. Subsequently, the animal-free nature and similarity to native collagen can be a major step forward in the development of in-vitro meat, especially if producers wish to replicate the characteristics of native tissue.
Here we present three biomaterials that have shown promising results in tissue engineering and that can be translated to cellular agriculture applications in large part due to their abundance, animal-free nature and current food applications. Moreover, the wide array of natural topographies and dietary fiber found in plants, alongside the antimicrobial and rheological properties of chitin/chitosan further extend their potential in cell culture and cellular agriculture. However, these materials do lack the biochemical cues found in native mammalian extracellular matrices, leading to a need for functionalization. This need further increases the complexity of the process, reducing the scalability potential. On the other hand, the emergence of recombinant collagen extracted from plants has important advantages as a scaffold in its own right or if used to functionalize the surfaces of the materials above. Furthermore, these materials have been modified, either as microspheres or bulk, to possess the porosity necessary for diffusion of nutrients through dynamic or static bioreactors (Oh et al., 2009; Wu et al., 2011; García Cruz et al., 2012; Varley et al., 2017; Huang et al., 2018; Specht et al., 2018). In order to scale an animal-free product with similarities to that of native animal tissue, the need for fetal bovine serum, cost-effective engineering processes, antibiotic dependence, scaffold development, and cell line(immortalized vs. primary and cell co-culture) needs to be addressed (Specht et al., 2018; Stephens et al., 2018; Lynch and Pierrehumbert, 2019). It's currently believed that scaffolding will play a crucial role in the scalability of cultured meat. Therefore, the aim of this review was to summarize three animal-free materials with properties (e.g., rheological, nutritional and biological) that will likely be desirable in scaffolding for cultured meat applications. Yet, we wish to remind the reader that scaffolding is only one component of a much larger endeavor; and the scalability potential of the methods presented here is still unknown, and for some unlikely. Readers are encouraged to refer to Stephens et al. (2018) and Specht et al. (2018) for an overview on cellular agriculture and the major challenges.
SC wrote, revised, and edited manuscript. AP revised and edited manuscript.
This work was supported by the New Harvest grant number 0005. New Harvest is a 501c3 non-profit research institute based in the US. SC was also supported by the Queen Elizabeth II graduate scholarship in science and technology (QEII—GSST). AP gratefully acknowledges generous support from the Natural Sciences and Engineering Research Council (NSERC) Discovery Grant and Canada foundation for Innovation grant.
SC and AP are inventors on a patent application concerning the use of plant-derived cellulose for tissue engineering applications filed by the University of Ottawa.
The authors declare that the research was conducted in the absence of any commercial or financial relationships that could be construed as a potential conflict of interest.
An, B., Kaplan, D. L., and Brodsky, B. (2014). Engineered recombinant bacterial collagen as an alternative collagen-based biomaterial for tissue engineering. Front. Chem. 2:40. doi: 10.3389/fchem.2014.00040
Antoine, E. E., Vlachos, P. P., and Rylander, M. N. (2014). Review of collagen I hydrogels for bioengineered tissue microenvironments: characterization of mechanics, structure, and transport. Tissue Eng. Part B Rev. 20, 683–696. doi: 10.1089/ten.TEB.2014.0086
Antoni, D., Burckel, H., Josset, E., and Noel, G. (2015). Three-dimensional cell culture: a breakthrough in vivo. Int. J. Mol. Sci. 16, 5517–5527. doi: 10.3390/ijms16035517
Azuma, K., Osaki, T., Minami, S., and Okamoto, Y. (2015). Anticancer and anti-inflammatory properties of chitin and chitosan oligosaccharides. J. Funct. Biomater. 6, 33–49. doi: 10.3390/jfb6010033
Badylak, S. F., and Gilbert, T. W. (2008). Immune response to biologic scaffold materials. Semin. Immunol. 20, 109–116. doi: 10.1016/j.smim.2007.11.003
BANFIELD, W. G. (1956). Age changes in the swelling capacity of the human achilles tendon. J. Gerontol. 11:372. doi: 10.1093/geronj/11.4.372
Bella, J., Brodsky, B., and Berman, H. M. (1995). Hydration structure of a collagen peptide. Structure 3, 893–906. doi: 10.1016/S0969-2126(01)00224-6
Benhabiles, M. S., Salah, R., Lounici, H., Drouiche, N., Goosen, M. F. A., and Mameri, N. (2012). Antibacterial activity of chitin, chitosan and its oligomers prepared from shrimp shell waste. Food Hydrocoll. 29, 48–56. doi: 10.1016/j.foodhyd.2012.02.013
Berthod, F., Germain, L., Tremblay, N., and Auger, F. A. (2006). Extracellular matrix deposition by fibroblasts is necessary to promote capillary-like tube formation in vitro. J. Cell. Physiol. 207, 491–498. doi: 10.1002/jcp.20584
Bettadapur, A., Suh, G. C., Geisse, N. A., Wang, E. R., Hua, C., Huber, H. A., et al. (2016). Prolonged culture of aligned skeletal myotubes on micromolded gelatin hydrogels. Sci. Rep. 6:28855. doi: 10.1038/srep28855
Bierhalz, A. C., Westin, C. B., and Moraes, Â. M. (2016). Comparison of the properties of membranes produced with alginate and chitosan from mushroom and from shrimp. Int. J. Biol. Macromol. 91, 496–504. doi: 10.1016/j.ijbiomac.2016.05.095
Bitar, K. N., and Zakhem, E. (2014). Design strategies of biodegradable scaffolds for tissue regeneration. Biomed. Eng. Comput. Biol. 6, 13–20. doi: 10.4137/BECB.S10961
Breser, M. L., Felipe, V., Bohl, L. P., Orellano, M. S., Isaac, P., Conesa, A., et al. (2018). Chitosan and cloxacillin combination improve antibiotic efficacy against different lifestyle of coagulase-negative staphylococcus isolates from chronic bovine mastitis. Sci. Rep. 8, 5081–5013. doi: 10.1038/s41598-018-23521-0
Brown, R. B., and Audet, J. (2008). Current techniques for single-cell lysis. J. R. Soc. Interface 5(Suppl. 2), S131–S138. doi: 10.1098/rsif.2008.0009.focus
Carletti, E., Motta, A., and Migliaresi, C. (2011). “Scaffolds for tissue engineering and 3D cell culture,” in 3D Cell Culture. Methods in Molecular Biology (Methods and Protocols), Vol. 695, ed J. Haycock (New York, NY: Humana Press), 17–39.
Cavka, A., Guo, X., Tang, S. J., Winestrand, S., Jönsson, L. J., and Hong, F. (2013). Production of bacterial cellulose and enzyme from waste fiber sludge. Biotechnol. Biofuels 6:25. doi: 10.1186/1754-6834-6-25
Cavo, M., Fato, M., Peñuela, L., Beltrame, F., Raiteri, R., and Scaglione, S. (2016). Microenvironment complexity and matrix stiffness regulate breast cancer cell activity in a 3D in vitro model. Sci. Rep. 6:35367. doi: 10.1038/srep35367
Chan, E. C., Kuo, S., Kong, A. M., Morrison, W. A., Dusting, G. J., Mitchell, G. M., et al. (2016). Three dimensional collagen scaffold promotes intrinsic vascularisation for tissue engineering applications. PLoS ONE 11:e0149799. doi: 10.1371/journal.pone.0149799
Cheung, P. C. K. (2013). Mini-review on edible mushrooms as source of dietary fiber: Preparation and health benefits. Food Sci. Hum. Wellness 2, 162–166. doi: 10.1016/j.fshw.2013.08.001
Chicatun, F., Pedraza, C. E., Muja, N., Ghezzi, C. E., McKee, M. D., and Nazhat, S. N. (2013). Effect of chitosan incorporation and scaffold geometry on chondrocyte function in dense collagen type I hydrogels. Tissue Eng. Part A 19, 2553–2564. doi: 10.1089/ten.TEA.2013.0114
Courtenay, J. C., Deneke, C., Lanzoni, E. M., Costa, C. A., Bae, Y., Scott, J. L., et al. (2018). Modulating cell response on cellulose surfaces; tunable attachment and scaffold mechanics. Cellulose 25, 925–940. doi: 10.1007/s10570-017-1612-3
Courtenay, J. C., Johns, M. A., Galembeck, F., Deneke, C., Lanzoni, E. M., Costa, C. A., et al. (2017). Surface modified cellulose scaffolds for tissue engineering. Cellulose 24, 253–267. doi: 10.1007/s10570-016-1111-y
Croisier, F., and Jérôme, C. (2013). Chitosan-based biomaterials for tissue engineering. Eur. Polym. J. 49, 780–792. doi: 10.1016/j.eurpolymj.2012.12.009
Decker, E., Von Ohle, C., Weiger, R., Wiech, I., and Brecx, M. (2005). A synergistic chlorhexidine/chitosan combination for improved antiplaque strategies. J. Periodont. Res. 40, 373–377. doi: 10.1111/j.1600-0765.2005.00817.x
Deguchi, S., Tsujii, K., and Horikoshi, K. (2015). In situ microscopic observation of chitin and fungal cells with chitinous cell walls in hydrothermal conditions. Sci. Rep. 5:11907. doi: 10.1038/srep11907
Derakhshanfar, S., Mbeleck, R., Xu, K., Zhang, X., Zhong, W., and Xing, M. (2018). 3D bioprinting for biomedical devices and tissue engineering: a review of recent trends and advances. Bioactive Mater. 3, 144–156. doi: 10.1016/j.bioactmat.2017.11.008
Esa, F., Tasirin, S. M., and Rahman, N. A. (2014). Overview of bacterial cellulose production and application. Agric. Agricult. Sci. Proc. 2, 113–119. doi: 10.1016/j.aaspro.2014.11.017
Fang, Y., and Eglen, R. M. (2017). Three-dimensional cell cultures in drug discovery and development. SLAS Discov. Adv. Life Sci. R D 22, 456–472. doi: 10.1177/1087057117696795
Fernandes, Â., Barreira, J. C. M., Antonio, A. L., Morales, P., Férnandez-Ruiz, V., Martins, A., et al. (2015). Exquisite wild mushrooms as a source of dietary fiber: analysis in electron-beam irradiated samples. LWT Food Sci. Technol. 60, 855–859. doi: 10.1016/j.lwt.2014.10.050
Fontana, G., Gershlak, J., Adamski, M., Lee, J. S., Matsumoto, S., Le, H. D., et al. (2017). Biofunctionalized plants as diverse biomaterials for human cell culture. Adv. Healthc. Mater. 6:1601225. doi: 10.1002/adhm.201601225
Fürsatz, M., Skog, M., Sivlér, P., Palm, E., Aronsson, C., Skallberg, A., et al. (2018). Functionalization of bacterial cellulose wound dressings with the antimicrobial peptide ε-poly-L-lysine. Biomed. Mater. 13:025014. doi: 10.1088/1748-605X/aa9486
García Cruz, D. M., Salmerón-Sánchez, M., and Gómez-Ribelles, J. L. (2012). Stirred flow bioreactor modulates chondrocyte growth and extracellular matrix biosynthesis in chitosan scaffolds. J. Biomed. Mater. Res. Part A 100A, 2330–2341. doi: 10.1002/jbm.a.34174
Geddis, A. E., and Prockop, D. J. (1993). Expression of human COL1A1 gene in stably transfected HT1080 cells: the production of a thermostable homotrimer of type I collagen in a recombinant system. Matrix 13, 399–405. doi: 10.1016/S0934-8832(11)80045-4
Gershlak, J., Hernandez, S., Fontana, G., Perreault, L., Hansen, K., Larson, S., et al. (2017). Crossing kingdoms: using decellularized plants as perfusable tissue engineering scaffolds. Biomaterials 125, 13–22. doi: 10.1016/j.biomaterials.2017.02.011
Glawe, J. D., Hill, J. B., Mills, D. K., and McShane, M. J. (2005). Influence of channel width on alignment of smooth muscle cells by high-aspect-ratio microfabricated elastomeric cell culture scaffolds. J. Biomed. Mater. Res. Part A 75, 106–114. doi: 10.1002/jbm.a.30403
Ha, J. H., Shehzad, O., Khan, S., Lee, S. Y., Park, J. W., Khan, T., et al. (2008). Production of bacterial cellulose by a static cultivation using the waste from beer culture broth. Korean J. Chem. Eng. 25, 812–815. doi: 10.1007/s11814-008-0134-y
Hajiabbas, M., Mashayekhan, S., Nazaripouya, A., Naji, M., Hunkeler, D., Rajabi Zeleti, S., et al. (2015). Chitosan-gelatin sheets as scaffolds for muscle tissue engineering. Artificial Cells Nanomed. Biotechnol. 43, 124–132. doi: 10.3109/21691401.2013.852101
Hayman, E. G., Pierschbacher, M. D., Suzuki, S., and Ruoslahti, E. (1985). Vitronectin—a major cell attachmentpromoting protein in fetal bovine serum. Exp. Cell Res. 160, 245–258. doi: 10.1016/0014-4827(85)90173-9
Hickey, R. J., Modulevsky, D. J., Cuerrier, C. M., and Pelling, A. E. (2018). Customizing the shape and microenvironment biochemistry of biocompatible macroscopic plant-derived cellulose scaffolds. ACS Biomater. Sci. Eng. 4, 3726–3736. doi: 10.1021/acsbiomaterials.8b00178
Hickey, R. J., and Pelling, A. E. (2019). Cellulose biomaterials for tissue engineering. Front. Bioeng. Biotechnol. 7:45. doi: 10.3389/fbioe.2019.00045
Huang, L., Xiao, L., Jung Poudel, A., Li, J., Zhou, P., Gauthier, M., et al. (2018). Porous chitosan microspheres as microcarriers for 3D cell culture. Carbohydr. Polym. 202, 611–620. doi: 10.1016/j.carbpol.2018.09.021
Huber, T., Müssig, J., Curnow, O., Pang, S., Bickerton, S., and Staiger, M. P. (2012). A critical review of all-cellulose composites. J. Mater. Sci. 47, 1171–1186. doi: 10.1007/s10853-011-5774-3
Huyck, L., Ampe, C., and Van Troys, M. (2012). The XTT cell proliferation assay applied to cell layers embedded in three-dimensional matrix. Assay Drug Dev. Technol. 10, 382–392. doi: 10.1089/adt.2011.391
Isobe, N., Komamiya, T., Kimura, S., Kim, U., and Wada, M. (2018). Cellulose hydrogel with tunable shape and mechanical properties: from rigid cylinder to soft scaffold. Int. J. Biol. Macromol. 117, 625–631. doi: 10.1016/j.ijbiomac.2018.05.071
Isobe, Y., Kosaka, T., Kuwahara, G., Mikami, H., Saku, T., and Kodama, S. (2012). Oriented collagen scaffolds for tissue engineering. Materials 5, 501–511. doi: 10.3390/ma5030501
Jayakumar, R., Chennazhi, K. P., Srinivasan, S., Nair, S. V., Furuike, T., and Tamura, H. (2011). Chitin scaffolds in tissue engineering. Int. J. Mol. Sci. 12, 1876–1887. doi: 10.3390/ijms12031876
Jedrzejczak-Silicka, M. (2017). History of Cell Culture in New Insights Into Cell Culture Technology, Sivakumar Joghi Thatha Gowder. IntechOpen. Available online at: https://www.intechopen.com/books/new-insights-into-cell-culture-technology/history-of-cell-culture (accessed August 8, 2018).
Jo-Feeney, M., Miller, A. M., and Roupas, P. (2014). Mushrooms—biologically distinct and nutritionally unique: exploring a “Third food kingdom”. Nutr. Today 49, 301–307. doi: 10.1097/NT.0000000000000063
John, P. J., Sarvamangala, G. K., and Narasiham, P. (1992), Textural sensory attributes of curried raw jack fruit, fried pressure cooked. J. Food Qual. 15, 295–302. doi: 10.1111/j.1745-4557.1992.tb00993.x
Johns, M. A., Bae, Y., Guimarães, F. E. G., Lanzoni, E. M., Costa, C. A. R., Murray, P. M., et al. (2018). Predicting ligand-free cell attachment on next-generation cellulose-chitosan hydrogels. ACS Omega 3, 937–945. doi: 10.1021/acsomega.7b01583
Jonas, R., and Farah, L. F. (1998). Production and application of microbial cellulose. Polym. Degrad. Stab. 59, 101–106. doi: 10.1016/S0141-3910(97)00197-3
Kalia, S., Dufresne, A., Cherian, B. M., Kaith, B. S., Avérous, L., Njuguna, J., et al. (2011). Cellulosebased bio- and nanocomposites: a review. Int. J. Polym. Sci. 2011, 1–35. doi: 10.1155/2011/837875
Karavolias, J., Salois, M. J., Baker, K. T., and Watkins, K. (2018). Raised without antibiotics: impact on animal welfare and implications for food policy, Transl. Anim. Sci. 2, 337–348. doi: 10.1093/tas/txy016
Keefe, J., Wauk, L., Chu, S., and DeLustro, F. (1992). Clinical use of injectable bovine collagen: a decade of experience. Clin. Mater. 9, 155–162. doi: 10.1016/0267-6605(92)90095-B
Khan, S., Ul-Islam, M., Ikram, M., Ullah, M. W., Israr, M., Subhan, F., et al. (2016). Three-dimensionally microporous and highly biocompatible bacterial cellulose–gelatin composite scaffolds for tissue engineering applications. RSC Adv. 6, 110840–110849. doi: 10.1039/C6RA18847H
Kim, S. (2018). Competitive biological activities of chitosan and its derivatives: Antimicrobial, antioxidant, anticancer, and anti-inflammatory activities. Int. J. Polym. Sci. 2018:1708172. doi: 10.1155/2018/1708172
Klemm, D., Heublein, B., Fink, H., and Bohn, A. (2005). Cellulose: fascinating biopolymer and sustainable raw material. Angew. Chem. 44, 3358–3393. doi: 10.1002/anie.200460587
Kohn, R. R., and Rollerson, E. (1960). Aging of human collagen in relation to susceptibility to the action of collagenase. J. Gerontol. 15, 10–14. doi: 10.1093/geronj/15.1.10
Kraham, S. J. (2017). “Environmental impacts of industrial livestock production,” in International Farm Animal, Wildlife and Food Safety Law, eds G. Steier and K. Patel (Cham: Springer), 3–40.
Kurniawan, H., Lai, J., and Wang, M. (2012). Biofunctionalized bacterial cellulose membranes by cold plasmas. Cellulose 19, 1975–1988. doi: 10.1007/s10570-012-9785-2
Lee, K. Y., and Mooney, D. J. (2012). Alginate: properties and biomedical applications. Prog. Polym. Sci. 37, 106–126. doi: 10.1016/j.progpolymsci.2011.06.003
Lee, P., Lin, R., Moon, J., and Lee, L. P. (2006). Microfluidic alignment of collagen fibers for in vitro cell culture. Biomed. Microdevices 8, 35–41. doi: 10.1007/s10544-006-6380-z
Li, J., Chen, M., Wei, X., Hao, Y., and Wang, J. (2017). Evaluation of 3D-printed polycaprolactone scaffolds coated with freeze-dried platelet-rich plasma for bone regeneration. Materials 10:831. doi: 10.3390/ma10070831
Lin, K. W., and Lin, H. Y. (2004). Quality characteristics of Chinese-style meatball containing bacterial cellulose (nata). J. Food Sci. 69, SNQ107–SNQ111. doi: 10.1111/j.1365-2621.2004.tb13378.x
Listrat, A., Lebret, B., Louveau, I., Astruc, T., Bonnet, M., Lefaucheur, L., et al. (2016). How muscle structure and composition influence meat and flesh quality. Sci. World J. 2016, 3182746–3182714. doi: 10.1155/2016/3182746
Liu, W., Merrett, K., Griffith, M., Fagerholm, P., Dravida, S., Heyne, B., et al. (2007,2008) Recombinant human collagen for tissue engineered corneal substitutes. Biomaterials 29, 1147–1158. doi: 10.1016/j.biomaterials.2007.11.011.
Lopes, T. D., Riegel-Vidotti, I. C., Grein, A., Tischer, C. A., and Faria-Tischer, P. C. (2014). Bacterial cellulose and hyaluronic acid hybrid membranes: Production and characterization. Int. J. Biol. Macromol. 67, 401–408. doi: 10.1016/j.ijbiomac.2014.03.047
Lukomski, S., Nakashima, K., Abdi, I., Cipriano, V. J., Ireland, R. M., Reid, S. D., et al. (2000). Identification and characterization of the scl gene encoding a group A streptococcus extracellular protein virulence factor with similarity to human collagen. Infect. Immun. 68, 6542–6553. doi: 10.1128/IAI.68.12.6542-6553.2000
Lukomski, S., Nakashima, K., Abdi, I., Cipriano, V. J., Shelvin, B. J., Graviss, E. A., et al. (2001). Identification and characterization of a second extracellular collagen-like protein made by group A streptococcus: control of production at the level of translation. Infect. Immun. 69, 1729–1738. doi: 10.1128/IAI.69.3.1729-1738.2001
Lynch, J., and Pierrehumbert, R. (2019). Climate impacts of cultured meat and beef cattle. Front. Sustain. Food Syst. 3:5. doi: 10.3389/fsufs.2019.00005
Madihally, S. V., and Matthew, H. W. T. (1999). Porous chitosan scaffolds for tissue engineering. Biomaterials 20, 1133–1142. doi: 10.1016/S0142-9612(99)00011-3
Martin, S. L., Vrhovski, B., and Weiss, A. S. (1995). Total synthesis and expression in escherichia coli of a gene encoding human tropoelastin. Gene 154, 159–166. doi: 10.1016/0378-1119(94)00848-M
McDougall, G. J., Morrison, I. M., Stewart, D., and Hillman, J. R. (1996). Plant cell walls as dietary fibre: range, structure, processing and function. J. Sci. Food Agric. 70, 133–150.
Modulevsky, D. J., Cuerrier, C. M., and Pelling, A. E. (2016). Biocompatibility of subcutaneously implanted plant-derived cellulose biomaterials. PLoS ONE 11:e0157894. doi: 10.1371/journal.pone.0157894
Modulevsky, D. J., Lefebvre, C., Haase, K., Al-Rekabi, Z., and Pelling, A. E. (2014). Apple derived cellulose scaffolds for 3D mammalian cell culture. PLoS ONE 9:e97835. doi: 10.1371/journal.pone.0097835
Moniri, M., Boroumand Moghaddam, A., Azizi, S., Abdul Rahim, R., Bin Ariff, A., Zuhainis Saad, W., et al. (2017). Production and status of bacterial cellulose in biomedical engineering. Nanomaterials 7:257. doi: 10.3390/nano7090257
Muzzarelli, R. A. A. (1997). Human enzymatic activities related to the therapeutic administration of chitin derivatives. Cell. Mol. Life Sci. 53, 131–140. doi: 10.1007/PL00000584
Myllyharju, J., Lamberg, A., Notbohm, H., Fietzek, P. P., Pihlajaniemi, T., and Kivirikko, K. I. (1997). Expression of wild-type and modified proα chains of human type I procollagen in insect cells leads to the formation of stable [alpha1(I)]2alpha2(I) collagen heterotrimers and [alpha1(I)]3 homotrimers but not [alpha2(I)]3 homotrimers. J. Biol. Chem. 272, 21824–21830.
Myllyharju, J., Nokelainen, M., Vuorela, A., and Kivirikko, K. I. (2000). Expression of recombinant human type I-III collagens in the yeast pichia pastoris. Biochem. Soc. Trans. 28, 353–357. doi: 10.1042/0300-5127:0280353
Novotna, K., Havelka, P., Sopuch, T., Kolarova, K., Vosmanska, V., Lisa, V., et al. (2013). Cellulose-based materials as scaffolds for tissue engineering. Cellulose 20, 2263–2278. doi: 10.1007/s10570-013-0006-4
O'Brien, F. J. (2011). Biomaterials and scaffolds for tissue engineering. Mater. Today 14, 88–95. doi: 10.1016/S1369-7021(11)70058-X
Oh, S. K. W., Chen, A. K., Chen, X., Mok, Y., Lim, U., Chin, A., et al. (2009). Long-term microcarrier suspension cultures of human embryonic stem cells. Stem Cell Res. 2, 219–230. doi: 10.1016/j.scr.2009.02.005
Olsen, D. R., Leigh, S. D., Chang, R., McMullin, H., Ong, W., Tai, E., et al. (2001). Production of human type I collagen in yeast reveals unexpected new insights into the molecular assembly of collagen trimers. J. Biol. Chem. 276, 24038–24043. doi: 10.1074/jbc.M101613200
Ostadhossein, F., Mahmoudi, N., Morales-Cid, G., Tamjid, E., Navas-Martos, F. J., Soriano-Cuadrado, B., et al. (2015). Development of Chitosan/Bacterial cellulose composite films containing nanodiamonds as a potential flexible platform for wound dressing. Materials 8, 6401–6418. doi: 10.3390/ma8095309
O'Sullivan, A. C. (1997). Cellulose: the structure slowly unravels. Cellulose 4, 173–207. doi: 10.1023/A:1018431705579
Pacheco, G., Mello, C. V., Chiari-Andréo, B. G., Isaac, V. L. B., Ribeiro, S. J. L., Pecoraro, É., et al. (2018). Bacterial cellulose skin masks—properties and sensory tests. J. Cosmetic Dermatol. 17, 840–847. doi: 10.1111/jocd.12441
Pakkanen, O., Hämäläinen, E., Kivirikko, K. I., and Myllyharju, J. (2003). Assembly of stable human type I and III collagen molecules from hydroxylated recombinant chains in the yeast pichia pastoris. effect of an engineered C-terminal oligomerization domain foldon. J. Biol. Chem. 278, 32478–32483. doi: 10.1074/jbc.M304405200
Pangestuti, R., and Kim, S. (2010). Neuroprotective properties of chitosan and its derivatives. Mar. Drugs 8, 2117–2128. doi: 10.3390/md8072117
Peng, Y. Y., Yoshizumi, A., Danon, S. J., Glattauer, V., Prokopenko, O., Mirochnitchenko, O., et al. (2010). A streptococcus pyogenes derived collagen-like protein as a non-cytotoxic and non-immunogenic crosslinkable biomaterial. Biomaterials 31, 2755–2761. doi: 10.1016/j.biomaterials.2009.12.040
Percot, A., Viton, C., and Domard, A. (2003). Optimization of chitin extraction from shrimp shells. Biomacromolecules 4, 12–18. doi: 10.1021/bm025602k
Petersen, N., and Gatenholm, P. (2011). Bacterial cellulose-based materials and medical devices: current state and perspectives. Appl. Microbiol. Biotechnol. 91, 1277–1286. doi: 10.1007/s00253-011-3432-y
Pihlajaniemi, T., Myllyl,ä, R., and Kivirikko, K. I. (1991). Prolyl 4-hydroxylase and its role in collagen synthesis. J. Hepatol. 13, S2–S7. doi: 10.1016/0168-8278(91)90002-S
Ravi, M., Paramesh, V., Kaviya, S. R., Anuradha, E., and Solomon, F. D. P. (2015). 3D cell culture systems: advantages and applications. J. Cell. Physiol. 230, 16–26. doi: 10.1002/jcp.24683
Ren, X., Chen, C., Hou, Y., Huang, M., Li, Y., Wang, D., et al. (2018). Biodegradable chitosan-based composites with dual functions acting as the bone scaffold and the inflammation inhibitor in the treatment of bone defects. Int. J. Polym. Mater. Polym. Biomater. 67, 703–710. doi: 10.1080/00914037.2017.1376196
Revin, V., Liyaskina, E., Nazarkina, M., Bogatyreva, A., and Shchankin, M. (2018). Cost-effective production of bacterial cellulose using acidic food industry by-products. Braz. J. Microbiol. 49, 151–159. doi: 10.1016/j.bjm.2017.12.012
Riedl, A., Schlederer, M., Pudelko, K., Stadler, M., Walter, S., Unterleuthner, D., et al. (2017). Comparison of cancer cells in 2D vs 3D culture reveals differences in AKT-mTOR-S6K signaling and drug responses. J. Cell Sci. 130, 203–218. doi: 10.1242/jcs.188102
Rodríguez-Vázquez, M., Vega-Ruiz, B., Ramos-Zúñiga, R., Saldaña-Koppel, D. A., and Quiñones-Olvera, L. F. (2015). Chitosan and its potential use as a scaffold for tissue engineering in regenerative medicine. Biomed Res. Int. 2015:821279. doi: 10.1155/2015/821279
Ruotsalainen, H., Sipil,ä, L., Vapola, M., Sormunen, R., Salo, A. M., Uitto, L., et al. (2006). Glycosylation catalyzed by lysyl hydroxylase 3 is essential for basement membranes. J. Cell Sci. 119(Pt 4), 625–635. doi: 10.1242/jcs.02780
Ruottinen, M., Bollok, M., Kögler, M., Neubauer, A., Krause, M., Hämäläinen, E., et al. (2008). Improved production of human type II procollagen in the yeast pichia pastoris in shake flasks by a wireless-controlled fed-batch system. BMC Biotechnol. 8:33. doi: 10.1186/1472-6750-8-33
Saska, S., Teixeira, L. N., Tambasco de Oliveira, P., Minarelli Gaspar, A. M., Lima Ribeiro, S. J., Messaddeq, Y., et al. (2012). Bacterial cellulose-collagen nanocomposite for bone tissue engineering. J. Mater. Chem. 22, 2212–22112. doi: 10.1039/c2jm33762b
Schramm, M., and Hestrin, S. (1954). Factors affecting production of cellulose at the air/ liquid interface of a culture of acetobacter xylinum. J. Gen. Microbiol. 11, 123–129. doi: 10.1099/00221287-11-1-123
Serra, T., Planell, J. A., and Navarro, M. (2013). High-resolution PLA-based composite scaffolds via 3-D printing technology. Acta Biomater. 9, 5521–5530. doi: 10.1016/j.actbio.2012.10.041
Shi, Z., Zhang, Y., Phillips, G. O., and Yang, G. (2014). Utilization of bacterial cellulose in food. Food Hydrocoll. 35, 539–545. doi: 10.1016/j.foodhyd.2013.07.012
Shilo, S., Roth, S., Amzel, T., Harel-Adar, T., Tamir, E., Grynspan, F., et al. (2013). Cutaneous wound healing after treatment with plant-derived human recombinant collagen flowable gel. Tissue Eng. Part A 19, 1519–1526. doi: 10.1089/ten.TEA.2012.0345
Shoseyov, O., Posen, Y., and Grynspan, F. (2013). Human Recombinant Type I Collagen Produced in Plants. Tissue Eng. Part A 19, 1527–1533. doi: 10.1089/ten.TEA.2012.0347
Shoseyov, O., Posen, Y., and Grynspan, F. (2014). Human collagen produced in plants: more than just another molecule. Bioengineered 5, 49–52. doi: 10.4161/bioe.26002
Smith, M., McFetridge, P., Bodamyali, T., Chaudhuri, J. B., Howell, J. A., Stevens, C. R., et al. (2000). Porcine-derived collagen as a scaffold for tissue engineering. Food Bioprod. Proc. 78, 19–24. doi: 10.1205/096030800532680
Specht, E. A., Welch, D. R., Rees Clayton, E. M., and Lagally, C. D. (2018). Opportunities for applying biomedical production and manufacturing methods to the development of the clean meat industry. Biochem. Eng. J. 132, 161–168. doi: 10.1016/j.bej.2018.01.015
Staunton, D., Millard, C., Aricescu, A., and Campbell, I. (2009). “Preparation of recombinant fibronectin fragments for functional and structural studies,” in Extracellular Matrix Protocols. Methods in Molecular Biology (Methods and Protocols), Vol. 522, eds S. Even-Ram and V. Artym (New York, NY: Humana Press), 73–99.
Stein, H., Wilensky, M., Tsafrir, Y., Rosenthal, M., Amir, R., Avraham, T., et al. (2009). Production of bioactive, post-translationally modified, heterotrimeric, human recombinant type-I collagen in transgenic tobacco. Biomacromolecules 10, 2640–2645. doi: 10.1021/bm900571b
Stephens, N., Di Silvio, L., Dunsford, I., Ellis, M., Glencross, A., and Sexton, A. (2018). Bringing cultured meat to market: technical, socio-political, and regulatory challenges in cellular agriculture. Trends Food Sci. Technol. 78, 155–166. doi: 10.1016/j.tifs.2018.04.010
Tamura, H., Furuike, T., Nair, S. V., and Jayakumar, R. (2011). Biomedical applications of chitin hydrogel membranes and scaffolds. Carbohydr. Polym. 84, 820–824. doi: 10.1016/j.carbpol.2010.06.001
Tchemtchoua, V. T., Atanasova, G., Aqil, A., Filée, P., Garbacki, N., Vanhooteghem, O., et al. (2011). Development of a chitosan nanofibrillar scaffold for skin repair and regeneration. Biomacromolecules 12, 3194–3204. doi: 10.1021/bm200680q
Thanner, S., Drissner, D., and Walsh, F. (2016). Antimicrobial Resistance in Agriculture. MBio 7:e02227–e02215. doi: 10.1128/mBio.02227-15
Thirumala, S., Gimble, J. M., and Devireddy, R. V. (2013). Methylcellulose based thermally reversible hydrogel system for tissue engineering applications. Cells 2, 460–475. doi: 10.3390/cells2030460
Tijore, A., Irvine, S. A., Sarig, U., Mhaisalkar, P., Baisane, V., and Venkatraman, S. (2018). Contact guidance for cardiac tissue engineering using 3D bioprinted gelatin patterned hydrogel. Biofabrication 10:025003. doi: 10.1088/1758-5090/aaa15d
Tin, S., Sakharkar, K. R., Lim, C. S., and Sakharkar, M. K. (2009). Activity of chitosans in combination with antibiotics in pseudomonas aeruginosa. Int. J. Biol. Sci. 5, 153–160. doi: 10.7150/ijbs.5.153
Tomihata, K., and Ikada, Y. (1997). In vitro and in vivo degradation of films of chitin and its deacetylated derivatives. Biomaterials 18, 567–575. doi: 10.1016/S0142-9612(96)00167-6
Turnbull, G., Clarke, J., Picard, F., Riches, P., Jia, L., Han, F., et al. (2018). 3D bioactive composite scaffolds for bone tissue engineering. Bioactive Mater. 3, 278–314. doi: 10.1016/j.bioactmat.2017.10.001
Varley, M. C., Markaki, A. E., and Brooks, R. A. (2017). Effect of rotation on scaffold motion and cell growth in rotating bioreactors. Tissue Eng. Part A 23, 522–534. doi: 10.1089/ten.TEA.2016.0357
Verbeke, W. A. J., and Viaene, J. (2000). Ethical challenges for livestock production: meeting consumer concerns about meat safety and AnimalWelfare. J. Agric. Environ. Ethics 12, 141–151. doi: 10.1023/A:1009538613588
Vernon, R. B., Gooden, M. D., Lara, S. L., and Wight, T. N. (2005). Microgrooved fibrillar collagen membranes as scaffolds for cell support and alignment. Biomaterials 26, 3131–3140. doi: 10.1016/j.biomaterials.2004.08.011
Wang, X., Wang, G., Liu, L., and Zhang, D. (2016). The mechanism of a chitosan-collagen composite film used as biomaterial support for MC3T3-E1 cell differentiation. Sci. Rep. 6:39322. doi: 10.1038/srep39322
Werkmeister, J. A., and Ramshaw, J. A. M. (2012). Recombinant protein scaffolds for tissue engineering. Biomed. Mater. 7:012002. doi: 10.1088/1748-6041/7/1/012002
Widhe, M., Bysell, H., Nystedt, S., Schenning, I., Malmsten, M., Johansson, J., et al. (2010). Recombinant spider silk as matrices for cell culture. Biomaterials 31, 9575–9585. doi: 10.1016/j.biomaterials.2010.08.061
Willard, J. J., Drexler, J. W., Das, A., Roy, S., Shilo, S., Shoseyov, O., et al. (2013). Plant-derived human collagen scaffolds for skin tissue engineering. Tissue Eng. Part A 19, 1507–1518. doi: 10.1089/ten.TEA.2012.0338
Wu, X., Peng, C., Huang, F., Kuang, J., Yu, S., Dong, Y., et al. (2011). Preparation and characterization of chitosan porous microcarriers for hepatocyte culture. Hepatobil. Pancr. Dis. Int. 10, 509–515. doi: 10.1016/S1499-3872(11)60086-6
Wu, Y. J., Chen, T., Chen, I., Kuo, S. M., and Chuang, C. W. (2018). Developing highly porous collagen scaffolds by using alginate microsphere porogens for stem cell cultures. Mater. Lett. 223, 120–123. doi: 10.1016/j.matlet.2018.04.039
Xu, X., Gan, Q., Clough, R. C., Pappu, K. M., Howard, J. A., Baez, J. A., et al. (2011). Hydroxylation of recombinant human collagen type I alpha 1 in transgenic maize co-expressed with a recombinant human prolyl 4- hydroxylase. BMC Biotechnol. 11:69. doi: 10.1186/1472-6750-11-69
Yang, T. (2011). Chitin-based materials in tissue engineering: applications in soft tissue and epithelial organ. Int. J. Mol. Sci. 12, 1936–1963. doi: 10.3390/ijms12031936
Yu, Z., An, B., Ramshaw, J. A. M., and Brodsky, B. (2014). Bacterial collagen-like proteins that form triple-helical structures. J. Struct. Biol. 186, 451–461. doi: 10.1016/j.jsb.2014.01.003
Zakhem, E., Raghavan, S., Gilmont, R. R., and Bitar, K. N. (2012). Chitosan-based scaffolds for the support of smooth muscle constructs in intestinal tissue engineering. Biomaterials 33, 4810–4817. doi: 10.1016/j.biomaterials.2012.03.051
Zhao, H., Xia, J., Wang, J., Yan, X., Wang, C., Lei, T., et al. (2018). Production of bacterial cellulose using polysaccharide fermentation wastewater as inexpensive nutrient sources. Biotechnol. Biotechnol. Equip. 32, 350–356. doi: 10.1080/13102818.2017.1418673
Keywords: 3D cell culture, animal-free, cellulose, chitin, recombinant proteins, chitosan
Citation: Campuzano S and Pelling AE (2019) Scaffolds for 3D Cell Culture and Cellular Agriculture Applications Derived From Non-animal Sources. Front. Sustain. Food Syst. 3:38. doi: 10.3389/fsufs.2019.00038
Received: 14 November 2018; Accepted: 30 April 2019;
Published: 17 May 2019.
Edited by:
Marianne Jane Ellis, University of Bath, United KingdomReviewed by:
James C. Courtenay, University of Bath, United KingdomCopyright © 2019 Campuzano and Pelling. This is an open-access article distributed under the terms of the Creative Commons Attribution License (CC BY). The use, distribution or reproduction in other forums is permitted, provided the original author(s) and the copyright owner(s) are credited and that the original publication in this journal is cited, in accordance with accepted academic practice. No use, distribution or reproduction is permitted which does not comply with these terms.
*Correspondence: Andrew E. Pelling, YUBwZWxsaW5nbGFiLm5ldA==
Disclaimer: All claims expressed in this article are solely those of the authors and do not necessarily represent those of their affiliated organizations, or those of the publisher, the editors and the reviewers. Any product that may be evaluated in this article or claim that may be made by its manufacturer is not guaranteed or endorsed by the publisher.
Research integrity at Frontiers
Learn more about the work of our research integrity team to safeguard the quality of each article we publish.