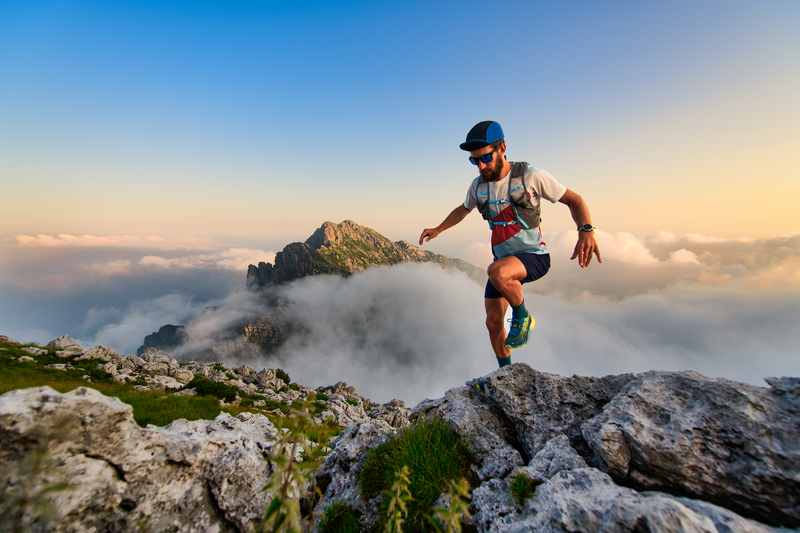
95% of researchers rate our articles as excellent or good
Learn more about the work of our research integrity team to safeguard the quality of each article we publish.
Find out more
REVIEW article
Front. Sustain. Food Syst. , 18 February 2019
Sec. Sustainable Food Processing
Volume 3 - 2019 | https://doi.org/10.3389/fsufs.2019.00007
This article is part of the Research Topic Nanotechnology applied to Food and Agriculture View all 7 articles
Bacterial cellulose (BC) has been produced for a number of applications, mainly focused on the biomedical area. Although there is a variety of interesting applications of BC for food and food packaging, only a few have been explored to the moment, since the high cost of BC production is usually considered as a limiting factor. On the other hand, several cost-effective culture media have been proposed, contributing to reduce BC production costs. This article overviews the bioprocess conditions that affects BC production and the main possible applications of BC for food and food packaging purposes.
Bacterial cellulose (BC) is a naturally occurring nanomaterial produced as an exopolysaccharide by some bacteria, such as those from the genus Komagataeibacter (former Gluconacetobacter) cultivated in a medium with carbon and nitrogen sources (Rajwade et al., 2015). Compared with other genera, Komagataeibacter is usually the genus of choice for research and food applications, due to its higher BC yield and purity (Ruka et al., 2012). Membranes with different network structure and mechanical properties were reported to be obtained from different strains of the genus Komagataeibacter (Chen et al., 2018).
The biosynthesis of BC was firstly observed by ancient Chinese producing kombucha tea, a fermented beverage produced by a symbiotic colony of acetic acid bacteria and yeast embedded within a cellulose mat formed at the surface of the beverage (Marsh et al., 2014). Some possible explanations of the cellulose formation by these microorganisms are that BC is formed as a self-defense mechanism to protect bacteria from the damaging effects of UV light or to help bacteria float at the air-liquid interface in order to secure sufficient oxygen supply (Reiniati et al., 2017). The BC biosynthesis is a strictly regulated process with multi-step reactions, involving several individual enzymes, catalytic complexes, and regulatory proteins. It is possible to divide the BC synthesis in two intermediary steps: (I) the intracellular formation of 1,4-β-glucan chains, and (II) the assembly and crystallization of cellulose chains. In this last step, the cellulose chains are extruded outside the cell and self-assembled into fibrils (Figure 1A). The polymerization and crystallization mechanisms are not very well-understood, and it is the limiting step in the biosynthesis (Chawla et al., 2009; Lee et al., 2014; Reiniati et al., 2017).
Although it shares the same molecular formula (C6H10O5)n with plant celluloses, BC is free of lignin, hemicelluloses, and pectin, which are normally present in plant-derived celluloses; thus BC purification is an easy, low energy process (Huang et al., 2014), whereas purification of plant celluloses usually requires harsh chemicals. The spatial fibril arrangement provides crystallinity indexes up to 85% (Siró and Plackett, 2010). Other unique properties of BC are higher degree of polymerization, and remarkable tensile properties due to its web-like network structure (Iguchi et al., 2000; Tsouko et al., 2015; Paximada et al., 2016b). When compared to plant-cellulose, BC fibers also have higher specific area (Sulaeva et al., 2015), higher water holding capacity (holding up to hundreds of times its weight in water), and longer drying time (Meftahi et al., 2009). Moreover, its production do not require harsh chemical treatments for cellulose isolation and purification (Shi et al., 2014).
The high aspect ratio of the fibrils provides BC with a high surface area, which results in a high water holding capacity, water molecules being tightly bound to the hydroxyl groups within the cellulose chains (Gelin et al., 2007). The abundance of reactive groups within BC structure also provides it with a variety of possible modifications (either in situ or ex situ) for the introduction of functionalities to fulfill specific requirements (Siró and Plackett, 2010), making it a remarkable material with tailor-made properties for a number of applications. Moreover, the high porosity combined to the high surface area make BC a suitable material for physical interaction with antimicrobials and other active compounds (Shah et al., 2013).
The yield and properties of BC depend on several factors, including the bacterial strain used, the culture medium composition, and the operational conditions applied during the cultivation process. The composition of the culture medium determines the material morphology and the physical properties of the resulting material, which affects the range of possible applications.
BC has been mainly used for biomedical applications such as materials for tissue engineering, wound dressing, artificial skin and blood vessels, and carriers for drug delivery (Rajwade et al., 2015; Tsouko et al., 2015). Particularly, “never-dried” BC membranes have been used for wound dressing applications, thanks to their remarkable tensile properties, water holding capacity, biocompatibility, and high porosity and permeability to gases, allowing them to maintain a suitable moist environment, and to absorb wound exudates (Czaja et al., 2006; Sulaeva et al., 2015).
There are still many challenges for commercial low-cost production of BC, including the lack of efficient fermentation systems (with low BC yields), the high capital investment and high operating costs, which explains why BC commercialization is still very incipient, and focused on high-value niche markets. Widening BC effective applications to other areas such as food and food packaging require efforts for optimization of the whole process, including evaluation of culture media compositions, fermentation systems, genetic engineering, and post-production modification (Gama and Dourado, 2018). On the other hand, many studies have focused on reducing its production costs by proposing cost-effective fermentation media to replace the expensive Hestrin and Schramm (HS) conventional medium. Several researches have been focused on the use of alternative cheap media, such as food industry by-products (Jozala et al., 2015; Fan et al., 2016; Molina-Ramírez et al., 2018; Revin et al., 2018) and cashew tree gum (Pacheco et al., 2017). Such alternative sources of nutrient media could be an interesting option to produce BC for food and food packaging applications, which do not require such a high purity degree as those required for biomedical applications.
This article overviews the bioprocess conditions that affects BC production and the main possible applications of BC as food ingredients as well as for packaging purposes.
The development of cost-effective production of BC involves the choice of bacterial strain as well as the selection of bioprocess conditions. BC may be produced by static cultivation or stirred cultivation. For commercial production, large scale, semi-continuous, or continuous fermentation methods are more suitable to meet the demand. Anyway, the maximum production of BC is always the objective, with suitable shape and properties for the intended application (Lin et al., 2013a). The effects of cultivation type (static or agitated; batch, or fed batch process), the bacterial strain and the culture medium (complex or industrial waste) need to be carefully evaluated in order to obtain BC with yields high enough to improve its feasibility for food and food packaging applications.
BC can be produced from the species of Achromobacter, Alcaligenes, Aerobacter, Agrobacterium, Azotobacter, Gluconacetobacter, Pseudomonas, Rhizobium, Sarcina, Dickeya, and Rhodobacter (Lin et al., 2013a). However, the major producers of BC belong to the Komagataeibacter genus (former Gluconacetobacter), which is widely used for BC production mainly due to its capacity to metabolize a wide range of carbon/nitrogen sources (Lee et al., 2014; Ul-Islam et al., 2017).
The development of genetic engineered strains is also a potential strategy that has been studied for enhancing BC production and yield as well as to improve its structural features (Ul-Islam et al., 2017). Recently, a strain of Komagataeibacter rhaeticus that can grow in low-nitrogen conditions and produce cellulose at high yields was isolated, its genome was sequenced, and a synthetic biology toolkit was developed for genetic engineering studies. This toolkit provided the characterization data necessary for the engineering of K. rhaeticus iGEM, which enabled to design a system that allows tunable control over native cellulose production and to produce novel patterned and functionalized cellulose-based biomaterials (Florea et al., 2016). The development of genetically engineered strains also allowed the increase of BC production in a low oxygen environment, which can facilitate the use of static culture for production of BC (Liu et al., 2018).
BC production requires a glucose-rich culture medium with other nutrients sources, resulting in high production costs, which limits BC potential applications (Hungund et al., 2013). The conventional culture medium used for BC production is the Hestrin and Schramm (HS) medium, which contains glucose, peptone and yeast extract as carbon, and nitrogen sources (Cacicedo et al., 2016). Nevertheless, alternative culture media containing fruit juices (Kurosumi et al., 2009; Hungund et al., 2013), sugar cane molasse (Keshk and Sameshima, 2006; Tyagi and Suresh, 2016), brewery waste (Shezad et al., 2010), among others (Ul-Islam et al., 2017) has been investigated for BC production.
The evaluation of fruit juices including orange, pineapple, apple, Japanese pear, and grape for BC production by Acetobacter xylinum NBRC 13693 showed that orange and Japanese pear juices were suitable medium for BC production, resulting in increased yields of BC (Kurosumi et al., 2009). Other examples of fruit juice as culture medium, including pineapple, pomegranate, muskmelon, water melon, tomato, orange, and also molasses, starch hydrolysate, sugarcane juice, coconut water, coconut milk have been also used as alternative carbon sources for bacterial cellulose production (Hungund et al., 2013). The suitability of using molasses from sugar industries as cheap carbon source for production of BC with characteristics similar to those obtained using more expensive carbon sources such as glucose or fructose has also been demonstrated (Tyagi and Suresh, 2016).
The use of additives into the growth medium, including organic acids, carbohydrates, and ethanol has been reported to improve BC production (Ul-Islam et al., 2017). A recent work on BC production by Komagataeibacter medellinensis using HS modified medium in the presence of ethanol and acetic acid (both at 0.1 wt%) revealed an improvement in BC yield up to 279%. However, the crystallinity index (%), the degree of polymerization, and maximum rate of degradation temperatures decreased considerably (Molina-Ramírez et al., 2018).
One of the current challenges limiting the application of BC in a broader and larger scale is related to bioprocess developments to increase its production yields. The process conditions used for BC production also affects directly its properties and, consequently, its potential applications. Reactor design as well as proper monitoring and control of process variables are considered as key factors toward optimizing BC yields (Ul-Islam et al., 2017). Independent of using static or agitated cultivation methods, in order to improve BC yield, the most important process variables that should be optimized and controlled during the cultivation process includes pH, oxygen supply, and temperature (Lee et al., 2014).
Optimum pH for cell growth and BC production depends on the bacterial strain, being usually between 4.0 and 7.0 (Reiniati et al., 2017). Monitoring and controlling the pH to attain maximum BC yield is recommended since the pH of the culture medium could vary as a function of time due to the accumulation of secondary metabolites, such as gluconic, acetic, or lactic acids that are produced during the consumption of sugars and nitrogen sources (Lee et al., 2014). The effects of different pH in the range of 3.5–7.5 on BC production by Acetobacter xylinum 0416 revealed that the dried weight of BC was 60% higher when the pH of the medium was controlled compared with uncontrolled pH. In addition, A. xylinum 0416 growth rate decreased around 30% when pH value dropped from 5.05 to 3.56, due the formation of acetic acid as a by-product during fermentation process (Zahan et al., 2015).
Another important process variable to control during cultivation is aeration. The BC-producing bacteria are highly aerobic, so a suitable oxygen supply is crucial (Wu and Li, 2015). While low dissolved oxygen content hinders bacteria growth and BC production, a high oxygenation can favor the production of gluconic acid (Lee et al., 2014; Ul-Islam et al., 2017). The control of the temperature during cultivation for BC production is also important, since it can have an effect on BC yield and properties as well. The effect of temperature on BC production by Komagataeibacter xylinus B-12068 showed that the optimal temperature range for cell growth and BC production is rather narrow 28–30°C, while the physiological temperature range is wide (20–37°C) (Volova et al., 2018).
Static cultivation is a simple and widely used method of BC production. The culture medium (usually placed into shallow trays) is inoculated, which results in the formation of the BC pellicle, which floats due to the entrapped CO2 bubbles generated by the bacteria (Lin et al., 2013a). The cultivation usually requires 5–20 days, until the BC sheet nearly fills the tray, since the BC production depends on the area of the air/liquid interface (Lin et al., 2013a).
In traditional static cultivation, vessels of diverse shapes and sizes can be used, so that the BC membrane takes the shape of the material where it was grown. This is an advantage when a material with a predefined shape is required, which has been widely used in regenerative medicine. Such a property has not been well-explored for food applications, but it might be used to produce nata-like products with different shapes, e.g., for children.
On the other hand, the traditional static culture is time-demanding, with low productivity, which may hinder its industrial application (Lin et al., 2013a). The cellulose membrane produced in the medium tends to entrap the bacteria, which limits their oxygen supply, and the nutrients are constantly consumed so that their concentration decreases over time, which limits the BC production (Esa et al., 2014). Fed-batch cultivation is an interesting strategy to overcome this problem. A study conducted by Shezad et al. (2009) showed that the addition of new alternative medium culture during cultivation in a continuous process regime increased two to three times the yield process in fed-batch cultivation compared to batch cultivation.
Recently, bioreactors that produce BC in a higher yield under nearly static conditions have been developed to produce BC sheets–such as Horizontal Lift Reactor (Kralisch et al., 2009), rotary biofilm contactor (Kim et al., 2007), and aerosol bioreactor (Hornung et al., 2007).
In agitated cultivation, oxygen is continuously mixed into the medium, so the BC is produced with enhanced yield compared with static culture, which contributes to cost reduction (Ul-Islam et al., 2015). The agitated fermentation process may lead to several forms of cellulose, from fibrous suspension to spheres and pellets (Esa et al., 2014), the shape and size depending on the applied rotational speed (Ul-Islam et al., 2015).
Although the BC yield in agitated culture is usually considered to be higher than in static culture, a major drawback of agitation is the increased probability of mutation of cellulose-producing cells into cellulose-negative mutants under the high turbulence and shear stress (Park et al., 2004; Kim et al., 2007). Thus, different reactors have been proposed to enhance BC productivity while preventing the formation of cellulose-negative subpopulations (Ul-Islam et al., 2017). Additionally, supplementing the culture medium with ethanol has been reported to prevent the accumulation of those mutants, increasing BC production (Son et al., 2001).
Stirred-tank bioreactors have been frequently used to produce BC in fibrous form. However, the crystallinity, elastic modulus, and degree of polymerization of the fibrous BC was reported to be lower than those of pellicular BC, as a result of the shear stress upon agitation (Watanabe et al., 1998). In stirred tank bioreactors, the fibrous BC suspension with high cell density produces a high viscosity, which limits the oxygen transfer, requiring a higher agitation power, which results in high energy consumption (Shoda and Sugano, 2005).
An alternative type of fermentation reactor is airlift bioreactor, in which oxygen is continuously transferred from the bottom of the reactor to the culture medium, providing a suitable oxygen supply (Ul-Islam et al., 2015). The process is more energy efficient and involves less shear stress when compared to stirred-tank reactors (Wu and Li, 2015). An airlift bioreactor for BC production was firstly reported by Chao et al. (1997). Different configuration of airlift bioreactors have been proposed since, such as a modified airlift bioreactor proposed by Wu and Li (2015) with a series of net plates, in order to produce BC in pellicular form. The resulting membranes presented higher water-holding capacity than that of BC produced by static cultivation, and the elastic modulus was reported to be manipulated by varying the number of net plates.
The downstream processing (Figure 1B) involves two steps: the separation of the BC produced from the culture medium and the purification of the biopolymer. The BC can be removed from the culture medium applying simple procedures, in spite of the cultivation system used. For the agitated cultivation the BC can be removed by filtration or centrifugation, and for static cultures the BC film produced in the liquid-air interface can be simply harvested. However, the recovered BC from the broth is not pure, since it contains some impurities like remaining cells and nutrients, thus it needs to be purified before application. The most widely used procedure for BC purification is the alkali treatment with NaOH or KOH. Care should be taken with the alkali treatment because concentrated solutions may transform the cellulose and modify its mechanical properties (Chawla et al., 2009; Reiniati et al., 2017). The level of purification required will depend on the type of application, whereas BC for medical application requires a more meticulous procedure to remove the impurities than the ones for food and food packaging application.
The downstream processing of BC can also include a drying step. There are different methods of drying the BC including drying at room temperature, oven drying, freeze drying, and supercritical drying. The drying process of BC changes its characteristics and properties, thus it should be chosen according to the final application of the material (Zeng et al., 2014; Vasconcellos and Farinas, 2018). It is important to note that the downstream processing for BC is usually easier and cheaper when compared to the procedures required to purify plant-derived cellulose. Nevertheless, the choice of BC downstream processing can impact the final characteristics and price of the material, and therefore should be selected based on its desired application.
BC may be used in different forms, including intact membranes (or fibers or pellets, depending on the production method), disassembled BC, and BC nanocrystals (BCNC). For most food and food packaging applications, BC should be combined to other components, which may be accomplished by different methods (Figure 2).
Figure 2. Techniques to mix BC with other components for food and food packaging applications. (A) Impregnation; (B) Disassembly; (C) Acid hydrolysis; (D) In situ nanocomposites. (Adapted from: Azeredo et al., 2017; reproduced with the permission of Elsevier).
BC membranes may be immersed into dispersions containing other components (Figure 2A). The main advantage of this impregnation approach is that the steps of membrane disintegration are avoided, making the process somewhat simpler. The main disadvantage, on the other hand, is the impossibility of changing the material shape after fermentation, limiting applications such as coatings. Nata-de-coco cubes, for instance, are immersed into syrups for commercialization. Impregnation have also been used to produce films (Zhu et al., 2010; Chang et al., 2012; Ul-Islam et al., 2012).
Alternatively, BC membranes may be disassembled (by physical and/or chemical methods) in order to be used as a suspension or in powder form, in formulations with other components (Figure 2B). This approach has some advantages. It is easier to combine into formulations, and the proportions of film components may be controlled more accurately. This approach has been used to produce ice cream (Guo et al., 2018) and films (Jiang et al., 2016; Viana et al., 2018).
BC nanocrystals (BCNC) are usually produced by acid hydrolysis, which removes most amorphous parts of cellulose, keeping needle-like crystals with nanosized diameters. Those may be used in several formulations (Figure 2C), including Pickering emulsions, or as a reinforcing phase in films, and coatings. Although the oral toxicity of BCNC has not been specifically studied, the oral gavage administration to rats of doses of up to 2,000 mg/kg of aqueous suspensions of plant cellulose nanocrystals resulted in no adverse effects (O'Connor et al., 2014).
Finally, in situ composites may be obtained by adding other components (usually another polymer) into the culture medium, making BC chains to grow forming a composite with the added component (Figure 2D).
BC is a dietary fiber, approved as a “generally recognized as safe” (GRAS) food by the US Food and Drug Administration (FDA) (Shi et al., 2014). As a food ingredient, one of the main advantages of BC is its appeal for dietetic foods, due to its indigestibility by humans (Mohite and Patil, 2014). Moreover, it favors the intestinal transit (as other dietary fibers), besides contributing to the mouthfeel (Fontana et al., 2017). The main applications of BC for the food industry (Figure 3) are described below.
BC has long been used as the raw material for a juicy and chewy dessert from Philippines called nata-de-coco, which is basically produced from BC grown from coconut water enriched with several carbohydrates and amino acids, cut into cubes, and immersed in a sugar syrup (Iguchi et al., 2000). Natural uncontrolled pre-fermentation processes have been increasingly used, but they represent a food safety risk because of the possible concomitant growth of pathogenic bacteria (Zhang et al., 2017).
Adaptations of the process may be used to produce similar products, such as nata-de-pina (with pineapple juice), the flavors being controlled by the culture medium source (Iguchi et al., 2000; Jozala et al., 2016). Apart from being consumed by themselves, nata products may be added as texture-rich cubes to beverages, yogurts, and jellies.
Although the Philippines are still still the main nata-de-coco producer, the country has faced problems, which have resulted in decreased production and exportation of the product since 2011, when the highest exportation (more than 6,000 tons, about US$ 6.6 billion) was recorded (Piadozo, 2016). The problems that the country have faced include: the high cost of coconut milk, which has been aggravated by infestation of coconut trees by the coconut scale insect; the adverse effects of extreme temperatures on bacteria activity and resulting nata quality; and competition from other coconut-producing countries, which have improved the process.
Actually, a variety of nata-like products may be developed to address different requirements by the consumers. Different shapes may be obtained by using different vessels; colors and flavors may be customized by impregnating BC with different fruit juices, syrups, etc. Finally, even texture may be changed by further processing techniques such as conventional oven drying or freeze drying, producing foamy or crunchy products. Thus, BC has an under-explored potential as a raw material for novel low-calorie desserts and snacks, for which there has been an increasing demand.
Fats have important roles on physical, rheological, and textural properties of a variety of foods. In cakes, just to mention an example, fats are responsible for keeping air cells into the oil-in-water emulsion, contributing to the volume, softness, and flavor (Rios et al., 2018).
On the other hand, high fat intakes have been related to the development of several health problems including obesity, diabetes, high blood cholesterol levels, and heart diseases; so, many efforts have been directed to reduce the fat content of food products (Guasch-Ferré et al., 2015). Consumers have been increasingly aware of the harmful effects of high-fat diets, and have therefore demanded for reduced-fat counterparts to the products they usually consume. On the other hand, there is a strongly held view that low-fat foods are less desirable, since they frequently have inferior sensory properties when compared to their high-fat counterparts. Thus, an important challenge is to assure that the sensory properties of the product are kept as close as possible to those of their regular corresponding products. Thus, research efforts have been made in the last few decades concerning suitable fat replacing ingredients or systems (from both the structural and sensory points of view).
The aims of fat replacement are to mimic the fat functionality in different food products, while reducing the calorific value and/or avoiding the fat-related health problems. Fat replacement studies have been carried out using different hydrocolloids in a variety of products such as cakes (Rios et al., 2018), emulsified meat products (Kumar et al., 2016), and cheeses (Aydinol and Ozcan, 2018).
BC have been used as a fat replacer in meatballs (Lin and Lin, 2004). One hand, the use of 20% BC (completely replacing the added fat of the product) resulted in dramatic adverse effects such as cooking losses and softening, impairing the product acceptance. On the other hand, the addition of 10% BC (reducing to a half the added fat content of meatballs) resulted in similar sensory properties and shelf stability to the control (regular meatballs).
The fat replacement by BC in a surimi product resulted in increased gel strength and water-holding capacity due to its enhanced network structure (Lin et al., 2011). However, no sensory tests were carried out for comparison of acceptance of the BC-containing surimi with the regular one.
A possibility that deserves a specific study is the substitution of partially hydrogenated oils (PHOs) with some modified BC, since PHOs lost the GRAS status by the FDA, and a number of possible replacers have been prospected (Wang et al., 2016).
Apart from substituting fats, BC was demonstrated to offer a variety of other health benefits, related to its action as a dietary fiber. Hypolipidemic and hypocholesterolemic effects of BC have been reported in hamsters, and those effects were significantly higher than those of plant cellulose (Chau et al., 2008). Increased fecal weight and decreased transit time were also reported in rats, when compared to a control group which did not receive BC (Okiyama et al., 1993).
The preparation of meat analogs by using BC with Monascus extract has been suggested (Sheu et al., 2000; Purwadaria et al., 2010). Monascus purpureus is a mold that produces yellow, orange and/or red polyketide pigments as well as antihypercholesterolemic agents such as monacolin and mevinolin (Sheu et al., 2000; Kim and Ku, 2018). Fermenting BC with this mold resulted in colored BC products, the color being affected by the carbon and nitrogen sources used for fermentation (Sheu et al., 2000). The colored composite has been presented as a raw material for novel functional foods, mainly meat analogs (Ochaikul et al., 2006; Purwadaria et al., 2010). The color of the composite was quite resistant to washing, autoclaving, freezing, or acidification (Sheu et al., 2000).
A meat analog produced from Monascus-BC complex would have a number of market appeals, namely, those related to BC as dietary fiber, the cholesterol-lowering effect from Monascus as well as the non-animal origin, which would make the product a suitable substitute to meat products for consumers with dietary restrictions (Ullah et al., 2016).
Solid colloidal particles have the ability to adsorb oil-water emulsion interfaces, stabilizing droplets against coalescence by forming a strong monolayer at the interface (Hu et al., 2015; Paximada et al., 2016b). Such emulsions are usually referred to as Pickering emulsions. As in the case of classical emulsions stabilized by surfactants (emulsifiers), the stabilization of the droplets of the discontinuous phase takes place by adsorption of the solid particles at the surface of droplets. However, the mechanism for the anchoring of the solid particles at the oil-water interface is different, based on partial wetting of the particle surfaces by oil and water; therefore, the solid particles do not have to be amphiphilic (Chevalier and Bolzinger, 2013), although an amphiphilic character is typically required (Kalashnikova et al., 2012).
BC and bacterial cellulose nanocrystals (BCNC) are hydrophilic due to the high density of hydroxyl groups on their surfaces (Dankovich and Gray, 2011), while hydrophobic interactions result from the crystalline organization and extensive hydrogen bonding of chains, making them amphiphilic (Lindman et al., 2010; Kalashnikova et al., 2012; Yan et al., 2017), property that have been applied to stabilize surfactant-free Pickering emulsions (Kalashnikova et al., 2011, 2012; Yan et al., 2017).
BC has shown better capacity to stabilize oil in water (O/W) emulsions (containing 10 wt% olive oil) when compared to commercial cellulose derivatives (HPMC and CMC), which was ascribed to the strong network formed by the BC fibrils adsorbed to the oil droplets (Paximada et al., 2016b). Even if BC emulsions presented the largest droplets (d3, 2 = 26 μm), they showed the best stability to coalescence, with lowest serum index (SI = 3%).
In another study, the same group (Paximada et al., 2016a) prepared O/W emulsions (with 5 wt% olive oil) using whey protein isolate (WPI, 2–5 wt%) as surfactant and BC (0–1 wt%) as stabilizer. At lower BC concentrations (0.5–0.7 wt%), the emulsion was unstable, but 1 wt% BC promoted stable emulsions, avoiding coalescence.
When comparing BC and BCNC for their emulsion stabilization performances in O/W emulsions (with olive oil), Yan et al. (2017) reported that BCNCs presented higher thermal stability as well as better emulsifying performance, which was ascribed not only to their size, but also to their highly negative zeta potential derived from the sulfate groups formed on sulfuric acid hydrolysis.
BC may be used for several applications including thickening, gelling, and water binding. It has been demonstrated to increase the gel strength of tofu, to prevent cocoa precipitation in a chocolate beverage, and to keep beverage viscosity after a heat treatment (Okiyama et al., 1993).
BC was compared to xanthan gum (XG) and locust bean gum (LBG) as an alternative and cheaper thickener (Paximada et al., 2016a). A lower BC concentration (0.1%, in contrast to 0.7% XG and 1% LBG) was required to obtain the same shear viscosity (yield stress), indicating that BC may be regarded as a good alternative thickener food applications.
Ice cream is a globally consumed and appreciated product. Structurally (Figure 4A), it consists of a complex matrix of fat globules, ice crystals, air bubbles, protein-hydrocolloid structures, and a continuous phase (serum) of unfrozen water with dissolved sugars, proteins, and salts (Daw and Hartel, 2015). Fats have a vital role on structure and texture of ice cream. Whipping and freezing promote partial coalescence of fat droplets, forming a 3D network which surrounds the air cells formed on whipping, supporting their structure, thus contributing to shape retention (Varela et al., 2014; Petrut et al., 2016). Moreover, fats contribute to the lubrication sense and flavor richness (Tekin et al., 2017). Proteins are also structural components with functions related to emulsification (adsorbing to fat globules during homogenization), whipping (contributing to formation of air bubbles), and water binding (enhancing viscosity, increasing the meltdown time, and reducing iciness) (Varela et al., 2014). Sugars control hardness by reducing the freezing temperature of the mix; stabilizers and emulsifiers regulate ice crystal growth, contributing to a uniform texture (Tekin et al., 2017). Upon temperature increases or fluctuations, the ice crystals melt, and the network of partial coalesced fat globules collapses (Petrut et al., 2016).
Figure 4. (A) Schematic structure of an ice cream; (B) proposed schematic structure of an ice cream containing NFBC as a fat replacer and rheology modifier, with reduced iciness, and air cell structure kept by NFBC.
Different potential functions of BC as an ice cream ingredient may be mentioned (Figure 4B), namely, as structure/texture modifier (increasing viscosity and meltdown time, supporting the physical structure, contributing to shape retention upon temperature fluctuations), emulsion and foam stabilizer (contributing to keep the network of fat droplets surrounding the air cells), and fat replacer (in terms of structure and texture). However, only a few studies have evaluated one or another of those aspects, and none was found studying all of them.
Cellulose derivatives such as CMC are commonly used in ice cream formulations as stabilizers by holding water, increasing the mix viscosity, reducing ice and lactose crystal growth on storage, and providing resistance to melting (Granger et al., 2005; Guo et al., 2018). On the other hand, BC has a better emulsion stabilizing ability than vegetable celluloses (Paximada et al., 2016a), which remains to be explored for ice creams.
Okiyama et al. (1993) reported that BC allowed ice creams to retain their shape for at least 60 min at room temperature, while control ice cream (without BC) completely melted away over the same time. A more detailed study is required relating the melting process with the ice cream structure, texture, and emulsion stability.
Guo et al. (2018) evaluated the influences of BC/soy protein isolate (SPI) composites (with different BC/SPI ratios, from 1:20 to 1:5) as a partial or total cream (fat) replacer on an ice cream model. The thermal stability, texture, rheological, and emulsifying properties of SPI were improved by BC addition. The product with 20% BC/SPI (1:20) presented the most similar texture to the regular ice cream (with 30% cream) as well as improved melting resistance, with the additional advantage of the lower calorific value.
Double emulsions are multi-phase systems including single emulsions (water-in-oil or oil-in-water-W/O or O/W, respectively) dispersed into a third phase. The two basic types of double emulsions are water-in-oil-in-water (W/O/W) and oil-in-water-in-oil (O/W/O). The most common applications of double emulsions to foods are related to fat reduction. Double emulsions confer a better mouthfeel when compared to conventional low-fat formulations, mimicking better the texture of regular (non-low-fat) products (Tekin et al., 2017). No study was found using BC as stabilizer and/or rheology modifier of double emulsion ice creams.
Probiotics have been increasingly used in food applications to balance gut microflora and confer health benefits to the consumers. However, the survival of probiotics upon storage and transit through the gastro-intestinal system is usually poor (Chávarri et al., 2012).
Fijałkowski et al. (2016) immobilized probiotic strains of Lactobacillus spp by impregnating BC membranes with the probiotic bacteria, or by including the probiotic into the culture medium for BC production. The second method was the most effective in terms of protection of the probiotic against the effects of gastric juices and bile salts. On the other hand, this approach is incompatible with the usual method used to purify BC membranes after fermentation, which includes soaking in an alkali solution at temperatures typically higher than 100°C to remove remaining BC-producing bacteria cells (Fijałkowski et al., 2016). Anyway, the study demonstrated that BC is useful as an immobilization support for Lactobacillus cells, protecting them against gastric juices.
Pectin/BC composites were tested to protect Bacillus coagulans (Khorasani and Shojaosadati, 2016). The composites resulted in a high survival rate of B. coagulans after microwave drying and simulated digestion. Composites with higher BC contents resulted in higher survival on digestion. The composite with 20% pectin and 80% BC was considered as the one with the best combination between degree of protection and prebiotic score.
Unfortunately, the studies with BC-based probiotic-containing materials are usually focused only on the impact of technological parameters on bacterial survival, but not on evaluating the capacity of BC of releasing probiotic bacteria at the intestine so they can play their role. Future studies are required to address those issues, as the effective functionality of any probiotic product depends on the bacteria being released at their colonization site.
BC has been also used to immobilize enzymes that may be useful for food applications. Enzymes which have been already successfully immobilized for controlled release from BC include lipase (Wu et al., 2017), laccase (Chen et al., 2015), and lysozyme (Bayazidi et al., 2018).
Bio-based films and coatings are membranes produced from renewable materials, having at least two components: a matrix, which usually consists of a macromolecule able to form a cohesive network; and a plasticizer, usually required for reducing brittleness inherent to most matrices. Other components may be also incorporated, in order to improve the barrier and mechanical properties, or their resistance to moisture. Sometimes, different matrices are combined to produce a material with desirable properties from each one.
Films and coatings are usually produced as packaging materials, although edible films and coatings usually require a complementary external packaging, for hygienic reasons. Some edible free-standing films may be added with flavor components such as fruit purees, and those may also be applied as snacks or wraps for sushis and non-conventional sandwiches (Otoni et al., 2017).
Being food-grade, BC may be used as a matrix for films and coatings for food applications, including edible films, and coatings. Moreover, it may also be used to produce reinforcing bacterial cellulose nanocrystals (BCNC).
BC membranes present an entangled structure, with void spaces distributed through it, which allows the entrapment of other components (Malheiros et al., 2018). The impregnation approach (Figure 1A) has been applied in several studies on food films. The method is technically simple, but not suitable to form coatings on food surfaces or to produce films by continuous methods.
In some cases, the combination of BC with other polymers may bring about advantages related to specific properties of the other polymer. Considering the antimicrobial properties of chitosan, for instance, Lin et al. (2013b) produced BC-chitosan films by impregnating BC into a chitosan solution. The resulting composite film not only presented activity against Gram-negative and Gram-positive bacteria, but it also exhibited remarkably higher elastic modulus than neat BC films.
Crosslinking is a very interesting approach to improve the tensile and barrier performance of packaging films. Proteins are easier to be involved in crosslinking reactions than polysaccharides, since their amino groups are highly nucleophilic (Azeredo and Waldron, 2016). In order to promote crosslinking reactions to BC sheets, Chang et al. (2012) impregnated them with gelatin and immersed the composite sheet into different protein crosslinking agents. Compact interpenetrating BC/gelatin networks were formed, with enhanced tensile properties, mainly at higher gelatin contents.
BC have been used as a vehicle to gradually deliver antimicrobial agents onto food surfaces, contributing thus to extend microbial food stability.
Antimicrobial BC membranes have been obtained by Santos et al. (2018), by impregnating BC sheets into nisin. FTIR revealed the immobilization of nisin to BC, which was ascribed to linkings of amine groups of nisin to C6 carboxylic acid groups of BC. The immobilization resulted in activity against Gram-negative and Gram-positive bacteria. Similar films have been tested by Nguyen et al. (2008) on the surface of vacuum-packaged frankfurters, and were shown to be effective to control bacterial growth.
Active sausage casings were produced by impregnating BC tubes with ε-polylysine (ε-PL) (Zhu et al., 2010). The composite casings, besides presenting good tensile and barrier properties, exhibited antibacterial activity, extending the shelf life of sausages. The casings also presented good thermal stability, keeping activity against S. aureus after autoclaving at up to 121°C for 30 min.
BC membranes impregnated with antimicrobial peptides (bacteriocins) were proven to be more efficient to control the growth of Listeria monocytogenes when compared to free bacteriocins (Malheiros et al., 2018), suggesting that BC played a protecting role on the peptides.
Multilayer films containing an antimicrobial layer between two outer layers was the approach used by Jebel and Almasi (2016) to control the release rate of the antimicrobial agent (ZnO nanoparticles). The active layer was impregnated in a dispersion of the nanoparticles, dried, coated (on both sides) with wet BC membranes, and allowed to dry. The presence of ZnO nanoparticles enhanced the tensile properties and decreased the water vapor permeability of the films, besides providing the films with antibacterial activity.
BC membranes may also be loaded with a variety of active agents other than antimicrobial compounds, including antioxidants, oxygen scanvengers, and ethylene absorbers, acting thus in different ways as vehicles to substances which may promote extended stability of foods to several modes of degradation.
Impregnation has not been a common strategy to incorporate reinforcement agents into BC membranes, but Ul-Islam et al. (2012) have used this approach to form BC nanocomposites with montmorillonite (MMT). The nanoclay was adsorbed by the BC membrane, and resulted in increased tensile strength and enhanced thermal stability of the nanocomposite material.
Most BC applications for films and coatings involve the use of disassembled BC (in suspension or powder form, as in Figure 1B). Besides the aforementioned advantages, this approach (in contrast to impregnation) may be used to produce films by continuous methods (such as continuous casting), which are more suitable for industrial purposes, and to apply coatings directly on food surfaces.
The most common technique to form films from disassembled BC is the conventional lab casting, which consists on pouring a film-forming dispersion onto a suitable surface and making the solvent to evaporate. Another interesting technique is electrospinning, which is an electrodynamic process where a polymer solution contained in a syringe is spun by the application of a high voltage electric field to the tip of the needle connected to the syringe. As the resulting jet travels in the air toward the collector, the polymer charges cause whipping or bending of the jet, with consequent jet elongation and solvent evaporation, producing a thin fiber which is deposited on the grounded collector as a randomly oriented, non-woven mesh (Bhushani and Anandharamakrishnan, 2014). BC mats were made by Costa et al. (2012) by electrospinning acetylated BC. The electrospun mats exhibited more symmetrically nanoporous structure when compared to cast films mats, with pores oriented along the longitudinal direction of the fibers, which was ascribed to the stretching effect during electrospinning. Although BC-based films for food packaging purposes have not yet been obtained by this method, the technique should be explored in the future. On the other hand, the technique was already used for BCNC-reinforced films from polyhydroxybutyrate-co-hydroxyvalerate (PHBV) (Martínez-Sanz et al., 2016) and corn starch (Fabra et al., 2016).
Disassembled BC may be used for formulation with a variety of compounds, in a more versatile way than with the impregnation approach. Nanofibrillated BC (NFBC, produced by TEMPO oxidation and physical defibrillation) has been used (combined or not to pectin, in different proportions) to produce films with added fruit (guava and mango) purees (Viana et al., 2018). Higher NFBC/pectin ratios resulted in improved tensile properties, barrier to water vapor, and water resistance, which reflects the superior tensile, and barrier properties of NFBC when compared to pectin. The films could be used for a variety of applications in which fruit flavors would be appreciated, including food wraps or even fruit ribbons.
NFBC has been also combined to copper nanoparticles (CuNP), forming antibacterial films with polyvinyl alcohol (PVA) (Jiang et al., 2016). The hybrid NFBC-CuNP material was used as a template for CuNP controlled release. NFBC was proven effective to regulate copper release, improving the long-term antimicrobial properties of the films against E. coli.
A bottom-up technique may be used to combine BC to another biopolymer in situ. BC nanofibrils are allowed to grow in the presence of the second biopolymer added to the culture medium, producing self-assembled bionanocomposites. Depending on the properties of the biopolymer used, the pattern of BC structure may be changed. Xyloglucan, xylan, and alginate were reported to decrease BC crystallinity and to stimulate BC production (Zhou et al., 2007; Gu and Catchmark, 2012). The presence of pectin, gelatin, or carboxymethylcellulose (CMC) in the culture media resulted in enhanced mechanical properties when compared to the pure BC, which is ascribed to the binding of the other polysaccharides with BC, changing cellulose network structure and water binding capacity (Dayal and Catchmark, 2016).
An in situ process of growing BC in a medium with 5% PVA was compared to BC impregnation with a 5% PVA solution (Gea et al., 2010). The in situ process resulted in virtually unchanged BC fibril arrangement, and the composite exhibited improved transparency and elongation when compared to pure BC, while the other tensile properties were maintained, which was ascribed to the partial interruption of hydrogen bonds within the BC network. The impregnation process, on the other hand, resulted in aggregates which remarkably impaired the tensile properties of the films.
Fontes et al. (2018) produced BC mats modified in situ with CMC. The presence of CMC increased the viscosity of the culture medium, affecting the biosynthesis of BC, and also reduced the porosity of the resulting materials. The CMC coated the BC nanofibers, which resulted in decrease of elastic modulus as the degree of substitution (DS) of CMC increased.
Another form of using BC as a phase for composites is by isolating their crystalline regions, obtaining bacterial cellulose nanocrystals (BCNC). Acid hydrolysis is most usually the technique of choice for BCNC isolation (Fabra et al., 2016; Salari et al., 2018), although enzymatic hydrolysis with cellulase may be used as well (George et al., 2011).
BCNCs were incorporated into a chitosan dispersion along with silver nanoparticles (AgNPs), to produce nanocomposite films (Salari et al., 2018). Hydrogen bonds were formed between chitosan and BCNC, and crystalline peaks appeared in X-ray diffractograms, resulting in enhanced barrier and tensile properties of the films, besides the antibacterial activity provided by the AgNPs.
BCNCs were also used as reinforcement to thermoplastic corn starch films (Fabra et al., 2016). The best performance (in terms of barrier and tensile properties) was achieved with a BCNC loading of 15 wt%. Nanocomposite PVA films also presented improved properties (thermal stability, tensile strength and elastic modulus) from BCNC addition, when compared to the control film from pure PVA (George et al., 2011).
Emulsion films from polyssacaride-lipid or protein-lipid combinations have been suggested to combine advantages of different components, namely, the tensile and gas barrier effects of polyssacarides (or proteins), and the barrier to water vapor of lipids. The addition of lipids to hydrophilic film forming formulations is an interesting approach for food applications which require a low water vapor permeability. On the other hand, the presence of lipids usually impairs the transparency and tensile properties of films (Rodrigues et al., 2014; Galus, 2018). Thus, the use of BCNC acting both as an emulsion stabilizer and a reinforcement agent sounds as an interesting approach, which has not been reported so far.
Other than nata-like products, there is a wide number of potential applications of BC for food industry as well as for food packaging purposes. Although they have not been suitably explored to the moment, because of economic reasons, a number of studies have indicated that the BC production costs tend to decrease, without compromising BC quality, mainly for food applications, which usually do not require a level of purity as high as those for biomedical applications. Moreover, the future looks very promising with novel technologies for BC production such as 3D printing, which has already been proven feasible to produce BC objects in any desired geometry (Shaffner et al., 2017).
Some technologies are still to be explored for processing BC for food purposes. Surface modifications to BC (by using plasma, for instance) are particularly promising, thanks to the abundance of chemically reactive sites within BC structure, allowing modifications to provide tailor-made surface functionalities (controlled by the choice of the plasma gas and other operational parameters), while keeping its core properties. Nitrogen-containing plasma, for instance, was proven to incorporate N-containing functional groups and increase BC porosity (Pertile et al., 2010). While the intended application of the study was for improving interaction with cells, a number of food applications could be explored, such as enhanced capacity for impregnation with active compounds, or increased gas permeability for films for fresh produce.
Although the commercial BC production for low-cost niche markets is still a challenge, and validations for most food applications are still required, there are reasons to believe that BC has a promising future as a unique ingredient for differentiated novel food products with sensory and health appeals, including edible films, and coatings.
CF, HB, VV, and AC addressed the introduction, bioprocess conditions for BC production, and the forms of BC. CF and VV also addressed the downstream processing, and revised the manuscript. HA addressed the food and food packaging applications, and revised the manuscript. Figures 1, 3 were created by VV, and Figures 2, 4, by HA.
The authors gratefully acknowledge the financial support of Brazilian Agricultural Research Corporation (EMBRAPA, Brazil, 03.14.04.007.00.00), the Nanotechnology Network for Research in Agriculture (Rede AgroNano, EMBRAPA, 01.14.03.001.03.00), Brazilian Agency for Industrial Research and Industrial (EMBRAPII, Brazil, PIFS-1802.0005), São Paulo Research Foundation (CePOF-FAPESP, 2013/07276-1; BIOEN-FAPESP 2016/10636-8), the Research Support Foundation of Ceará State (FUNCAP/CNPq PR2-0101-00023.01.00/15), Brazilian National Council for Scientific and Technological Development (CNPq- PVE 401182/2014-2), and Brazilian Higher Education Improvement Coordination (CAPES).
The authors declare that the research was conducted in the absence of any commercial or financial relationships that could be construed as a potential conflict of interest.
Author AC thanks São Paulo Research Foundation (FAPESP, Process # 2018/01835-2) and author VV thanks Brazilian Higher Education Improvement Coordination (CAPES) for their scholarship. Authors HA, HB, and CF thank the National Council for Scientific and Technological Development (CNPq, Brazil) for their Productivity Fellowships (PQ 302381/2016-3, DT 305169/2016-5, and PQ 303166/2016-9, respectively).
Aydinol, P., and Ozcan, T. (2018). Production of reduced fat Labneh cheese with inulin and β-glucan fibre based fat replacer. Int. J. Dairy Technol. 71, 362–371. doi: 10.1111/1471-0307.12456
Azeredo, H. M. C., Rosa, M. F., and Mattoso, L. H. C. (2017). Nanocellulose in bio-based food packaging applications. Ind. Crops Prod. 97, 664–671. doi: 10.1016/j.indcrop.2016.03.013
Azeredo, H. M. C., and Waldron, K. W. (2016). Crosslinking in polysaccharide and protein films and coatings for food contact–A review. Trends Food Sci. Technol. 52, 109–122. doi: 10.1016/j.tifs.2016.04.008
Bayazidi, P., Almasi, H., and Asl, A. K. (2018). Immobilization of lysozyme on bacterial cellulose nanofibers: characteristics, antimicrobial activity and morphological properties. Int. J. Biol. Macromol. 107, 2544–2551. doi: 10.1016/j.ijbiomac.2017.10.137
Bhushani, J. A., and Anandharamakrishnan, C. (2014). Electrospinning and electrospraying techniques: potential food based applications. Trends Food Sci. Technol. 38, 21–33. doi: 10.1016/tifs.2014.03.004
Cacicedo, M. L., Castro, M. C., Servetas, I., Bosnea, L., Boura, K., Tsafrakidou, P., et al. (2016). Progress in bacterial cellulose matrices for biotechnological applications. Bioresour. Technol. 213, 172–180. doi: 10.1016/j.biortech.2016.02.071
Chang, S.-T., Chen, L.-C., Lin, S.-B., and Chen, H.-H. (2012). Nano-biomaterials application: morphology and physical properties of bacterial cellulose/gelatin composites via crosslinking. Food Hydrocoll. 27, 137–144. doi: 10.1016/j.foodhyd.2011.08.004
Chao, Y. P., Sugano, Y., Kouda, T., Yoshinaga, F., and Shoda, M. (1997). Production of bacterial cellulose by Acetobacter xylinum with an air-lift reactor. Biotechnol. Tech. 11, 829–832. doi: 10.1023/A:1018433526709
Chau, C. F., Yang, P., Yu, C., and Yen, G. (2008). Investigation on the lipid-and cholesterol-lowering abilities of biocellulose. J. Agric. Food Chem. 56, 2291–2295. doi: 10.1021/jf7035802
Chávarri, M., Marañon, I., and Villarán, M. C. (2012). “Encapsulation technology to protect probiotic bacteria,” in Probiotics, ed E. C. Rigobelo (London: InTech), 501–540.
Chawla, P. R., Bajaj, I. B., Survase, S. A., and Singhal, R. S. (2009). Microbial cellulose: fermentative production and applications. Food Technol. Biotechnol. 47, 107–124.
Chen, L., Zou, M., and Hong, F. F. (2015). Evaluation of fungal laccase immobilized on natural nanostructured bacterial cellulose. Front. Microbiol. 6:1245. doi: 10.3389/fmicb.2015.01245
Chen, S.-Q., Lopez-Sanchez, P., Wang, D., Mikkelsen, D., and Gidley, M. J. (2018). Mechanical properties of bacterial cellulose synthesised by diverse strains of the genus Komagataeibacter. Food Hydrocoll. 81, 87–95. doi: 10.1016/j.foodhyd.2018.02.031
Chevalier, Y., and Bolzinger, M.-A. (2013). Emulsions stabilized with solid nanoparticles: pickering emulsions. Colloids Surf. A 439, 23–34. doi: 10.1016/j.colsurfa.2013.02.054
Costa, L. M. M., Olyveira, G. M., Basmaji, P., and Xavier Filho, L. (2012). Nanopores structure in electrospun bacterial cellulose. J. Biomater. Nanobiotechnol. 3, 92–96. doi: 10.4236/jbnb.2012.31012
Czaja, W., Krystynowicz, A., Bielecki, S., and Brown, Jr. R. M. (2006). Microbial cellulose—the natural power to heal wounds. Biomaterials 27, 145–151. doi: 10.1016/j.biomaterials.2005.07.035
Dankovich, T. A., and Gray, D. G. (2011). Contact angle measurements on smooth nanocrystalline cellulose (I) thin films. J. Adhes. Sci. Technol. 25, 699–708. doi: 10.1163/016942410X525885
Daw, E., and Hartel, R. W. (2015). (2015). Fat destabilization and melt-down of ice creams with increased protein content. Int. Dairy J. 43, 33–41. doi: 10.1016/j.idairyj.2014.12.001
Dayal, M. S., and Catchmark, J. M. (2016). Mechanical and structural property analysis of bacterial cellulose composites. Carbohydr. Polym. 144, 447–453. doi: 10.1016/j.carbpol.2016.02.055
Esa, F., Tasirin, S. M., and Rahman, N. A. (2014). Overview of bacterial cellulose production and application. Agric. Agric. Sci. Procedia 2, 113–119. doi: 10.1016/j.aaspro.2014.11.017
Fabra, M. J., López-Rubio, A., Ambrosio-Martín, J., and Lagaron, J. M. (2016). Improving the barrier properties of thermoplastic corn starch-based films containing bacterial cellulose nanowhiskers by means of PHA electrospun coatings of interest in food packaging. Food Hydrocoll. 61, 261–268. doi: 10.1016/j.foodhyd.2016.05.025
Fan, X., Gao, Y., He, W., Hu, H., Tian, M., Wang, K., et al. (2016). Production of nano bacterial cellulose from beverage industrial waste of citrus peel and pomace using Komagataeibacter xylinus. Carbohydr. Polym. 151, 1068–1072. doi: 10.1016/j.carbpol.2016.06.062
Fijałkowski, K., Peitler, D., Rakoczy, R., and Zywicka, A. (2016). Survival of probiotic lactic acid bacteria immobilized in different forms of bacterial cellulose in simulated gastric juices and bile salt solution. LWT Food Sci. Technol. 68, 322–328. doi: 10.1016/j.lwt.2015.12.038
Florea, M., Hagemann, H., Santosa, G., Abbott, J., Micklem, C. N., Spencer-Milnes, X., et al. (2016). Engineering control of bacterial cellulose production using a genetic toolkit and a new cellulose-producing strain. Proc. Natl. Acad. Sci. U.S.A. 1113, E3431–E3440. doi: 10.1073/pnas.1522985113.
Fontana, J. D., Koop, H. S., Tiboni, M., Grzybowski, A., Pereira, A., Kruger, C. D., et al. (2017). “New insights on bacterial cellulose,” in Food biosynthesis, ed. A.M. Grumezescu, A. M. Holban (Cambridge: Academic Press), 213–249. doi: 10.1016/C2016-0-00180-8
Fontes, M. L., Meneguin, A. B., Tercjak, A., Gutierrez, J., Cury, B. S. F., Santos, A. M., et al. (2018). Effect of in situ modification of bacterial cellulose with carboxymethylcellulose on its nano/microstructure and methotrexate release properties. Carbohydr. Polym., 179, 126–134. doi: 10.1016/j.carbpol.2017.09.061
Galus, S. (2018). Functional properties of soy protein isolate edible films as affected by rapeseed oil concentration. Food Hydrocoll. 85, 233–241. doi: 10.1016/j.foodhyd.2018.07.026
Gama, F. M. P., and Dourado, F. (2018). Bacterial nanocellulose: what future? Bioimpacts 8, 1–3. doi: 10.15171/bi.2018.01
Gea, S., Bilotti, E., Reynolds, C. T., Soykeabkeaw, N., and Peojs, T. (2010). Bacterial cellulose–poly(vinyl alcohol) nanocomposites prepared by an in-situ process. Mater. Lett. 64, 901–904. doi: 10.1016/j.matlet.2010.01.042
Gelin, K., Bodin, A., Gatenholm, P., Mihranyan, A., Edwards, K., and Strømme, M. (2007). Characterization of water in bacterial cellulose using dielectric spectroscopy and electron microscopy. Polymer 48, 7623–7631. doi: 10.1016/j.polymer.2007.10.039
George, J., Ramana, K. V., and Bawa, A. S. (2011). Bacterial cellulose nanocrystals exhibiting high thermal stability and their polymer nanocomposites. Int. J. Biol. Macromol. 48, 50–57. doi: 10.1016/j.ijbiomac.2010.09.013
Granger, C., Leger, A., Barey, P., Langendorff, V., and Cansell, M. (2005). Influence of formulation on the structural networks in ice cream. Int. Dairy J. 15, 255–262. doi: 10.1016/j.idairyj.2004.07.009
Gu, J., and Catchmark, J. M. (2012). Impact of hemicelluloses and pectin on sphere-like bacterial cellulose assembly. Carbohydr. Polym. 88, 547–557. doi: 10.1016/j.carbpol.2011.12.040
Guasch-Ferré, M., Babio, N., Martínez-González, M. A., Corella, D., Ros, E., Martín-Peláez, S., et al. (2015). Dietary fat intake and risk of cardiovascular disease and all-cause mortality in a population at high risk of cardiovascular disease. Am. J. Clin. Nutr. 102, 1563–1573. doi: 10.3945/ajcn.115.116046
Guo, Y., Zhang, X., Hao, W., Xie, Y., Chen, L., Li, Z., et al. (2018). Nano-bacterial cellulose/soy protein isolate complex gel as fat substitutes in ice cream model. Carbohydr. Polym. 198, 620–630. doi: 10.1016/j.carbpol.2018.06.078
Hornung, M., Ludwig, M., and Schmauder, H. P. (2007). Optimizing the production of bacterial cellulose in surface culture: a novel aerosol bioreactor working on a fed batch principle (Part 3). Eng. Life Sci. 7, 35–41. doi: 10.1002/elsc.200620164
Hu, Z., Ballinger, S., Pelton, R., and Cranston, E. D. (2015). Surfactant-enhanced cellulose nanocrystal pickering emulsions. J. Coll. Interf. Sci. 439, 139–148. doi: 10.1016/j.jcis.2014.10.034
Huang, Y., Zhu, C., Yang, J., Nie, Y., Chen, C., and Sun, D. (2014). Recent advances in bacterial cellulose. Cellulose 21, 1–30. doi: 10.1007/s10570-013-0088-z
Hungund, B., Prabhu, S., Shetty, C., Acharya, S., Prabhu, V., and Gupta, S. (2013). Production of bacterial cellulose from Gluconacetobacter persimmonis GH-2 using dual and cheaper carbon sources. J. Microb. Biochem. Technol. 5, 31–33. doi: 10.4172/1948-5948.1000095
Iguchi, M., Yamanaka, S., and Budhiono, A. (2000). Bacterial cellulose - a masterpiece of nature's arts. J. Mater. Sci. 35, 261–270. doi: 10.1023/A:1004775229149
Jebel, F. S., and Almasi, H. (2016). Morphological, physical, antimicrobial and release properties of ZnO nanoparticles-loaded bacterial cellulose films. Carbohydr. Polym. 149, 8–19. doi: 10.1016/j.carbpol.2016.04.089
Jiang, C., Oporto, G. S., Zhong, T., and Jaczynski, J. (2016). TEMPO nanofibrillated cellulose as template for controlled release of antimicrobial copper from PVA films. Cellulose 23, 713–722. doi: 10.1007/s10570-015-0834-5
Jozala, A. F., Lencastre-Novaes, L. C., Lopes, A. M., Santos-Ebinuma, V. C., Mazzola, P. G., Pessoa Jr, A., et al. (2016). Bacterial nanocellulose production and application: a 10-year overview. Appl. Microbiol. Biotechnol. 100, 2063–2072. doi: 10.1007/s00253-015-7243-4
Jozala, A. F., Pértile, R. A. N., Santos, C. A., Santos-Ebinuma, V. C., Seckler, M. M., Gama, F. M., et al. (2015). Bacterial cellulose production by Gluconacetobacter xylinus by employing alternative culture media. Appl. Microbiol. Biotechnol. 99, 1181–1190. doi: 10.1007/s00253-014-6232-3
Kalashnikova, I., Bizot, H., Cathala, B., and Capron, I. (2011). New Pickering emulsions stabilized by bacterial cellulose nanocrystals. Langmuir 27, 7471–7479. doi: 10.1021/la200971f
Kalashnikova, I., Bizot, H., Cathala, B., and Capron, I. (2012). Modulation of cellulose nanocrystals amphiphilic properties to stabilize oil/water interface. Biomacromolecules 13, 267–275. doi: 10.1021/bm201599j
Keshk, S., and Sameshima, K. (2006). The utilization of sugar cane molasses with/without the presence of lignosulfonate for the production of bacterial cellulose. Appl. Microbiol. Biotechnol. 72, 291–296. doi: 10.1007/s00253-005-0265-6
Khorasani, A. C., and Shojaosadati, S. A. (2016). Bacterial nanocellulose-pectin bionanocomposites as prebiotics against drying and gastrointestinal condition. Int. J. Biol. Macromol. 83, 9–18. doi: 10.1016/j.ijbiomac.2015.11.041
Kim, D., and Ku, S. (2018). Beneficial effects of Monascus sp. KCCM 10093 pigments and derivatives: a mini review. Molecules 23:E98. doi: 10.3390/molecules23010098
Kim, J. Y., Kim, J. N., Wee, Y. J., Park, D. H., and Ryu, H. W. (2007). Bacterial cellulose production by Gluconacetobacter sp. RKY5 in a rotary biofilm contactor. Appl. Biochem. Biotechnol. 137, 529–537. doi: 10.1007/s12010-007-9077-8
Kralisch, D., Hessler, N., Klemm, D., Erdmann, R., and Schmidt, W. (2009). White biotechnology for cellulose manufacturing—The HoLiR concept. Biotechnol. Bioeng. 105, 740–747. doi: 10.1002/bit.22579
Kumar, Y., Kairam, N., Ahmad, T., and Yadav, D. N. (2016). Physico chemical, microstructural and sensory characteristics of low-fat meat emulsion containing aloe gel as potential fat replacer. Int. J. Food Sci. Technol. 51, 309–316. doi: 10.1111/ijfs.12957
Kurosumi, A., Sasaki, C., Yamashita, Y., and Nakamura, Y. (2009). Utilization of various fruit juices as carbon source for production of bacterial cellulose by Acetobacter xylinum NBRC 13693. Carbohydr. Polym. 76, 333–335. doi: 10.1016/j.carbpol.2008.11.009
Lee, K. Y., Buldum, G., Mantalaris, A., and Bismarck, A. (2014). More than meets the eye in bacterial cellulose: biosynthesis, bioprocessing, and applications in advanced fiber composites. Macromol. Biosci. 14, 10–32. doi: 10.1002/mabi.201300298
Lin, K. W., and Lin, H. Y. (2004). Quality characteristics of Chinese-style meatball containing bacterial cellulose (Nata). J. Food Sci. 69, Q107–Q111. doi: 10.1111/j.1365-2621.2004.tb13378.x
Lin, S.-P., Calvar, I. L., Catchmark, J. F., Liu, J.-R., Demirci, A., and Cheng, K.-C. (2013a). Biosynthesis, production and applications of bacterial cellulose. Cellulose 20, 2191–22119. doi: 10.1007/s10570-013-9994-3
Lin, S. B., Chen, L. C., and Chen, H. H. (2011). Physical characteristics of surimi and bacterial cellulose composite gel. J. Food Proc. Eng. 34, 1363–1379. doi: 10.1111/j.1745-4530.2009.00533.x
Lin, W.-C., Lien, C.-C., Yeh, H.-J., Yu, C.-M., and Hsu, S.-H. (2013b). Bacterial cellulose and bacterial cellulose-chitosan membranes for wound dressing applications. Carbohydr. Polym. 94, 603–611. doi: 10.1016/j.carbpol.2013.01.076
Lindman, B., Karlstrom, G., and Stigsson, L. (2010). On the mechanism of dissolution of cellulose. J. Molec. Liquids 156, 76–81. doi: 10.1016/j.molliq.2010.04.016
Liu, M., Li, S., Xie, Y., Jia, S., Hou, Y., Zou, Y., and Zhong, C. (2018). Enhanced bacterial cellulose production by Gluconacetobacter xylinus via expression of Vitreoscilla hemoglobin and oxygen tension regulation. Appl. Microbiol. Biotechnol. 102, 1155–1165. doi: 10.1007/s00253-017-8680-z
Malheiros, P. S., Jozala, A. F., Pessoa, A. Jr., Vila, M. M. D. C., and Balcão, V. M. (2018). Immobilization of antimicrobial peptides from Lactobacillus sakei subsp. sakei 2a in bacterial cellulose: structural and functional stabilization. Food Pack. Shelf Life 17, 25–29. doi: 10.1016/j.fpsl.2018.05.001
Marsh, A. J., O'Sullivan, O., Hill, C., Ross, R. P., and Cotter, P. D. (2014). Sequence-based analysis of the bacterial and fungal compositions of multiple kombucha (tea fungus) samples. Food Microbiol. 38, 171–178. doi: 10.1016/j.fm.2013.09.003
Martínez-Sanz, M., Lopez-Rubio, A., Villano, M., Oliveira, C. S. S., Majone, M., Reis, M., et al. (2016). Production of bacterial nanobiocomposites of polyhydroxyalkanoates derived from waste and bacterial nanocellulose by the electrospinning enabling melt compounding method. J. Appl. Polym. Sci. 133:42486. doi: 10.1002/app.42486
Meftahi, A., Khajavi, R., Rashidi, A., Sattari, M., Yazdanshenas, M. E., and Torabi, M. (2009). The effects of cotton gauze coating with microbial cellulose. Cellulose 17, 199–204. doi: 10.1007/s10570-009-9377-y
Mohite, B. V., and Patil, S. V. (2014). A novel biomaterial: bacterial cellulose and its new era applications. Biotechnol. Appl. Biochem. 61, 101–110. doi: 10.1002/bab.1148
Molina-Ramírez, C., Castro, C., Zuluaga, R., and Gañán, P. (2018). Physical characterization of bacterial cellulose produced by Komagataeibacter medellinensis using food supply chain waste and agricultural by-products as alternative low-cost feedstocks. J. Polym. Environ. 26, 830–837. doi: 10.1007/s10924-017-0993-6
Nguyen, V. T., Gidley, M. J., and Dykes, G. A. (2008). Potential of a nisin-containing bacterial cellulose film to inhibit Listeria monocytogenes on processed meats. Food Microbiol. 25, 471–478. doi: 10.1016/j.fm.2008.01.00
Ochaikul, D., Chotirittikrai, K., Chantra, J., and Wutigornsombatkul, S. (2006). Studies on fermentation of Monascus purpureus TISTR 3090 with bacterial cellulose from Acetobacter xylinum TISTR 967. KMITL Sci. Tech. J. 6, 13–17.
O'Connor, B., Berry, R., and Goguen, R. (2014). “Commercialization of cellulose nanocrystal (NCC) production: a business case focusing on the importance of proactive ehs management,” in Nanotechnology Environmental Health and Safety, eds M. S. Hull and D. M. Bowman (Oxford:William Andrew), 225–246.
Okiyama, A., Motoki, M., and Yamanaka, S. (1993). Bacterial cellulose IV. Application to processed foods. Food Hydrocoll. 6, 503–511. doi: 10.1016/S0268-005X(09)80074-X
Otoni, C. G., Avena-Bustillos, R. J., Azeredo, H. M. C., Lorevice, M. V., Moura, M. R., Mattoso, L. H. C., et al. (2017). Recent advances on edible films based on fruits and vegetables—a review. Compreh. Rev. Food Sci. Food Safety 16, 1151–1169. doi: 10.1111/1541-4337.12281
Pacheco, G., Nogueira, C. R., Meneguin, A. B., Trovatti, E., Silva, M. C. C., Machado, R. T. A., et al. (2017). Development and characterization of bacterial cellulose produced by cashew tree residues as alternative carbon source. Ind. Crops Prod. 107, 13–19. doi: 10.1016/j.indcrop.2017.05.026
Park, J. K., Hyun, S. H., and Jung, J. Y. (2004). Conversion of G. hansenii PJK into non-cellulose producing mutants according to the culture condition. Biotechnol. Bioprocess Eng. 9, 383–388. doi: 10.1007/BF02933062
Paximada, P., Koutinas, A. A., and Mandala, I. (2016a). Effect of bacterial cellulose addition on physical properties of WPI emulsions. Comparison with common thickeners. Food Hydrocoll. 54, 245–254. doi: 10.1016/j.foodhyd.2015.10.014
Paximada, P., Tsouko, E., Kopsahelis, N., Koutinas, A. A., and Mandala, I. (2016b). Bacterial cellulose as stabilizer of o/w emulsions. Food Hydrocoll. 53, 225–232. doi: 10.1016/j.foodhyd.2014.12.003
Pertile, R. A. N., Andrade, F. K., Alves J.r., C., Gama, M. (2010). Surface modification of bacterial cellulose by nitrogen-containing plasma for improved interaction with cells. Carbohydr. Polym. 82, 692–698. doi: 10.1016/j.carbpol.2010.05.037
Petrut, R. F., Danthine, S., and Blecker, C. (2016). Assessment of partial coalescence in whippable oil-in-water food emulsions. Adv. Colloid Interface Sci. 229, 25–33. doi: 10.1016/j.cis.2015.12.004
Piadozo, M. E. S. (2016). “Nata de coco industry in the Philippines,” in Bacterial Nanocellulose – From Biotechnology to Bio-Economy, ed M. Gama, F. Dourado, S. Bielecki (London: Elsevier), 215–229.
Purwadaria, T., Gunawan, L., and Gunawan, A. W. (2010). The production of nata colored by Monascus purpureus J1 pigments as functional food. Microbiol. Indones. 4, 6–10. doi: 10.5454/mi.4.1.2
Rajwade, J. M., Paknikar, K. M., and Kumbhar, J. V. (2015). Applications of bacterial cellulose and its composites in biomedicine. Appl. Microbiol. Biotechnol. 99, 2491–2511. doi: 10.1007/s00253-015-6426-3
Reiniati, I., Hrymak, A. N., and Margaritis, A. (2017). Recent developments in the production and applications of bacterial cellulose fibers and nanocrystals. Crit. Rev.Biotechnol. 37, 510–524. doi: 10.1080/07388551.2016.1189871
Revin, V., Liyaskina, E., Nazarkina, M., Bogatyreva, A., and Shchankin, M. (2018). Cost-effective production of bacterial cellulose using acidic food industry by-products. Brazil. J. Microbiol. 49, 151–159. doi: 10.1016/j.bjm.2017.12.012
Rios, R. V., Garzón, R., Lannes, S. C. S., and Rosell, C. M. (2018). Use of succinyl chitosan as fat replacer on cake formulations. LWT 96, 2660–2265. doi: 10.1016/j.lwt.2018.05.041
Rodrigues, D. C., Caceres, C. A., Ribeiro, H. L., Abreu, R. F. A., Cunha, A. P., and Azeredo, H. M. C. (2014). Influence of cassava starch and carnauba wax on physical properties of cashew tree gum-based films. Food Hydrocoll. 38, 147–151. doi: 10.1016/j.foodhyd.2013.12.010
Ruka, D. R., Simon, G. P., and Dean, K. M. (2012). Altering the growth conditions of Gluconacetobacter xylinus to maximize the yield of bacterial cellulose. Carbohydr. Polym. 89, 613–622. doi: 10.1016/j.carbpol.2012.03.059
Salari, M., Khiabani, M. S., Mokarram, R. R., Ghanbarzadeh, B., and Kafil, H. S. (2018). Development and evaluation of chitosan based active nanocomposite films containing bacterial cellulose nanocrystals and silver nanoparticles. Food Hydrocoll. 84, 414–423. doi: 10.1016/j.foodhyd.2018.05.037
Santos, C. A., Santos, G. R., Soeiro, V. S., Santos, J. R., Rebelo, M. A., Chaud, M. V., et al. (2018). Bacterial nanocellulose membranes combined with nisin: a strategy to prevent microbial growth. Cellulose 25, 6681–6689. doi: 10.1007/s10570-018-2010-1
Shaffner, M., Rühs, P. A., Coulter, F., Kilcher, S., and Studart, A. R. (2017). 3D printing of bacteria into functional complex materials. Sci. Adv. 3:eaao6804. doi: 10.1126/sciadv.aao6804
Shah, N., Ul-Islam, M., Khattak, W. A., and Park, J. K. (2013). Overview of bacterial cellulose composites: a multipurpose advanced material. Carbohydr. Polym. 98, 1585–1598. doi: 10.1016/j.carbpol.2013.08.018
Sheu, F., Wang, C. L., and Shyu, Y. T. (2000). Fermentation of Monascus purpureus on bacterial cellulose-nata and the color stability of Monascus-nata complex. J. Food Sci. 65, 342–345. doi: 10.1111/j.1365-2621.2000.tb16004.x
Shezad, O., Khan, S., Khan, T., and Park, J. K. (2009). Production of bacterial cellulose in static conditions by a simple fed-batch cultivation strategy. Korean J. Chem. Eng. 26, 1689–1692. doi: 10.1007/s11814-009-0232-5
Shezad, O., Khan, S., Khan, T., and Park, J. K. (2010). Physicochemical and mechanical characterization of bacterial cellulose produced with an excellent productivity in static conditions using a simple fed-batch cultivation strategy. Carbohydr. Polym. 82, 173–180. doi: 10.1016/j.carbpol.2010.04.052
Shi, Z., Zhang, Y., Phillips, G. O., and Yang, G. (2014). Utilization of bacterial cellulose in food. Food Hydrocoll. 35, 539–545. doi: 10.1016/j.foodhyd.2013.07.012
Shoda, M., and Sugano, Y. (2005). Recent advances in bacterial cellulose production. Biotechnol. Bioprocess Eng. 10, 1–8. doi: 10.1007/BF02931175
Siró, I., and Plackett, D. (2010). Microfibrillated cellulose and new nanocomposite materials: a review. Cellulose 17, 459–494. doi: 10.1007/s10570-010-9405-y
Son, H.-J., Heo, M.-S., Kim, Y.-G., and Lee, S.-J. (2001). Optimization of fermentation conditions for the production of bacterial cellulose by a newly isolated Acetobacter. Biotechnol. Appl. Biochem. 33, 1–5. doi: 10.1042/BA20000065
Sulaeva, I., Henniges, U., Rosenau, T., and Potthast, A. (2015). Bacterial cellulose as a material for wound treatment: Properties and modifications. A review. Biotechnol. Adv. 33, 1547–1571. doi: 10.1016/j.biotechadv.2015.07.009
Tekin, E., Sahin, S., and Sumnu, G. (2017). Physicochemical, rheological, and sensory properties of low-fat ice cream designed by double emulsions. Eur. J. Food Sci. Technol. 119:1600505. doi: 10.1002/ejlt.201600505
Tsouko, E., Kourmenta, C., Ladakis, D., Kopsahelis, N., Mandala, I., Papanikolaou, S., et al. (2015). Bacterial cellulose production from industrial waste and by-product streams. Int. J. Mol. Sci. 16, 14832–14849. doi: 10.3390/ijms160714832
Tyagi, N., and Suresh, S. (2016). Production of cellulose from sugarcane molasses using Gluconacetobacter intermedius SNT-1, optimization and characterization. J. Clean. Prod. 112, 71–80. doi: 10.1016/j.jclepro.2015.07.054
Ul-Islam, M., Khan, T., and Park, J. K. (2012). Nanoreinforced bacterial cellulose-montmorillonite composites for biomedical applications. Carbohydr. Polym. 89, 1189–1197. doi: 10.1016/j.carbpol.2012.03.093
Ul-Islam, M., Khan, T., Ullah, M. W., and Park, J. K. (2015). Bacterial cellulose composites: Synthetic strategies and multiple applications in bio-medical and electro-conductive field. Biotechnol. J. 10, 1847–1861. doi: 10.1002/biot.201500106
Ul-Islam, M., Ullah, M. J., Khan, S., Shah, N., and Park, J. K. (2017). Strategies for cost-effective and enhanced production of bacterial cellulose. Int. J. Biol. Macromol. 102, 1166–1173. doi: 10.1016/j.ijbiomac.2017.04.110
Ullah, H., Santos, H. A., and Khan, T. (2016). Applications of bacterial cellulose in food, cosmetics and drug delivery. Cellulose 23, 2291–2314. doi: 10.1007/s10570-016-0986-y
Varela, P., Pintor, A., and Fiszman, S. (2014). How hydrocolloids affect the temporal oral perception of ice cream. Food Hydrocoll. 36, 220–228. doi: 10.1016/j.foodhyd.2013.10.005
Vasconcellos, V. M., and Farinas, C. S. (2018). The effect of the drying process on the properties of bacterial cellulose films from Gluconacetobacter Hansenii. Chem. Eng. Trans. 64, 145–150. doi: 10.3303/CET1864025
Viana, R. M., Sá, N. M. S. M., Barros, M. O., Borges, M. F., and Azeredo, H. M. C. (2018). Nanofibrillated bacterial cellulose and pectin edible films added with fruit purees. Carbohydr. Polym. 196, 27–32. doi: 10.1016/j.carbpol.2018.05.017
Volova, T. G., Prudnikova, S. V., Sukovatyi, A. G., and Shishatskaya, E. I. (2018). Production and properties of bacterial cellulose by the strain Komagataeibacter xylinus B-12068. Appl. Microbiol. Biotechnol. 102, 7417–7428. doi: 10.1007/s00253-018-9198-8.
Wang, F. C., Gravelle, A. J., Blake, A. I., and Marangoni, A. G. (2016). Novel trans fat replacement strategies. Curr. Opin. Food Sci. 7, 27–34. doi: 10.1016/j.cofs.2015.08.006
Watanabe, K., Tabuchi, M., Morinaga, Y., and Yoshinaga, F. (1998). Structural features and properties of bacterial cellulose produced in agitated culture. Cellulose 5, 187–200. doi: 10.1023/A:1009272904582
Wu, S.-C., and Li, M.-H. (2015). Production of bacterial cellulose membranes in a modified airlift bioreactor by Gluconacetobacter xylinus. J. Biosci. Bioeng. 120, 444–449. doi: 10.1016/j.jbiosc.2015.02.018
Wu, S.-C., Wu, S.-M., and Su, F.-M. (2017). Novel process for immobilizing an enzyme on a bacterial cellulose membrane through repeated absorption. J. Chem. Technol. Biotechnol. 92, 109–114. doi: 10.1002/jctb.4994
Yan, H., Chen, X., Song, H., Li, J., Feng, Y., Shi, Z., et al. (2017). Synthesis of bacterial cellulose and bacterial cellulose nanocrystals for their applications in the stabilization of olive oil pickering emulsion. Food Hydrocoll. 72, 127–135. doi: 10.1016/j.foodhyd.2017.05.044
Zahan, K. A., Pa'e, N., and Muhamad, I. I. (2015). Monitoring the effect of pH on bacterial cellulose production and acetobacter xylinum 0416 growth in a rotary discs reactor. Arab. J. Sci. Eng. 40, 1881–1885. doi: 10.1007/s13369-015-1712-z
Zeng, M. L., Laromaine, A., and Roig, A. (2014). Bacterial cellulose films: influence of bacterial strain and drying route on film properties. Cellulose 21, 4455–4469. doi: 10.1007/s10570-014-0408-y
Zhang, J., Yang, Y., Deng, J., Wang, Y., Hu, Q., Li, C., et al. (2017). Dynamic profile of the microbiota during coconut water prefermentation for nata de coco production. LWT Food Sci. Technol. 81, 87–93. doi: 10.1016/j.lwt.2017.03.036
Zhou, L. L., Sun, D. P., Hu, L. Y., Li, Y. W., and Yang, J. Z. (2007). Effect of addition of sodium alginate on bacterial cellulose production by Acetobacter xylinum. J. Ind. Microbiol. Biotechnol. 34, 483–489. doi: 10.1007/s10295-007-0218-4
Keywords: nanocellulose, nanofibrils, nata-de-coco, emulsions, edible films, impregnation
Citation: Azeredo HMC, Barud H, Farinas CS, Vasconcellos VM and Claro AM (2019) Bacterial Cellulose as a Raw Material for Food and Food Packaging Applications. Front. Sustain. Food Syst. 3:7. doi: 10.3389/fsufs.2019.00007
Received: 05 October 2018; Accepted: 25 January 2019;
Published: 18 February 2019.
Edited by:
Miguel Cerqueira, Laboratório Ibérico Internacional de Nanotecnologia (INL), PortugalReviewed by:
Guadalupe Virginia Nevárez-Moorillón, Autonomous University of Chihuahua, MexicoCopyright © 2019 Azeredo, Barud, Farinas, Vasconcellos and Claro. This is an open-access article distributed under the terms of the Creative Commons Attribution License (CC BY). The use, distribution or reproduction in other forums is permitted, provided the original author(s) and the copyright owner(s) are credited and that the original publication in this journal is cited, in accordance with accepted academic practice. No use, distribution or reproduction is permitted which does not comply with these terms.
*Correspondence: Henriette M. C. Azeredo, aGVucmlldHRlLmF6ZXJlZG9AZW1icmFwYS5icg==
Disclaimer: All claims expressed in this article are solely those of the authors and do not necessarily represent those of their affiliated organizations, or those of the publisher, the editors and the reviewers. Any product that may be evaluated in this article or claim that may be made by its manufacturer is not guaranteed or endorsed by the publisher.
Research integrity at Frontiers
Learn more about the work of our research integrity team to safeguard the quality of each article we publish.