- 1Laboratory of Food Microbiology and Food Preservation, Faculty of Bioscience Engineering, Ghent University, Ghent, Belgium
- 2Institute of Food Safety and Analytical Science, Nestlé Research Centre, Lausanne, Switzerland
- 3Laboratory of Food Microbiology and Biotechnology, Department of Industrial Biological Sciences, Ghent University, Kortrijk, Belgium
Berries represent an important fresh food commodity but are also used as dried or frozen ingredients added to many foods. Due to a number of foodborne outbreaks linked to frozen berries, the microbial safety of berry products has become a major food safety issue. The effect of gaseous ozone against selected strains was investigated in the present study at a laboratory scale. Among all the tested conditions, a 30 min treatment with 6% wt/wt (ca. 80 g/m3) gaseous ozone delivered the highest reductions on fresh strawberries: 2.1, 1.5, 1.8, and 3.3-log reductions were achieved for Salmonella, E. faecium, MNV-1 and MS2, respectively. For frozen strawberries, a short exposure of 5 min to ozone gas resulted in 1.6, 0.7, 0.7, and 1.8-log reductions of Salmonella, E. faecium, MNV-1 and MS2, respectively. Salmonella appeared to be more sensitive than its surrogate E. faecium. Compared to MS2, MNV-1 was more resistant and thus may represent as a more suitable surrogate for human norovirus in validating gas treatment efficiency. No sensorial differences were detected after ozone treatment. Inactivation results using gaseous ozone on frozen berries was reported for the first time in the present study. Guidelines on reporting gaseous ozone data were proposed to help ensure the reproducibility and experimental transparency in inactivation studies. Data collected in the present study support the potential use of gaseous ozone as a sustainable decontamination technology for frozen strawberries at different stages of processing. Further optimization of ozone exposure in a pilot plant scale setting is needed to facilitate the future application of gaseous ozone during minimal processing of berries.
Introduction
Berries represent an important fresh food commodity, which are produced and consumed in high volumes due to their perceived health benefits (Goodburn and Wallace, 2013; Van Boxstael et al., 2013). During the fruit season, berries are manually harvested and consumed as fresh, but also used as dried or frozen ingredients added to many foods. With a minimal use of physical interventions to control the foodborne pathogens, microbial contamination is considered to be the most important food safety issue in berry products (Van Boxstael et al., 2013; Bouwknegt et al., 2015; Li et al., 2015; Jacxsens et al., 2017). In the past decade, the consumption of frozen berries in Europe showed a steep increase (Tavoschi et al., 2015), meanwhile numbers of foodborne outbreaks have been reported. 26 virus outbreaks were reported on frozen berries in Europe from 2005 to 2013 (EFSA (European Food Safety Authority), 2014; Tavoschi et al., 2015). During the same period, a total of 20 foodborne outbreaks reported in the United States were traced back to berries (CCDC, 2013). The largest frozen strawberries outbreak happened in Germany in 2012, which resulted in 10,950 illnesses, exemplifying the risk of large outbreaks in the era of global food trade (Bernard et al., 2014). These outbreaks related with frozen berries reveal not only the influences of these products on public health and economic loss, also highlight out the urgent need to identify effective prevention measures that can be used to increase the safety of frozen berries.
Different decontamination measures have been investigated to control the foodborne pathogens on berries (Gulati et al., 2001; Lou et al., 2011; Li and Wu, 2013; Ramos et al., 2013; Pyatkovskyy et al., 2017). Ozone acquired the generally recognized as safe status (GRAS) in 1982 (O'Donnell et al., 2012) and has been used as a decontamination tool in the food and beverage industry. With its high oxidation potential, it has been demonstrated to be effective against foodborne pathogens when used as a liquid sanitizer (Guzel-Seydim et al., 2004; Hirneisen et al., 2011). When used in gas phase, the existing application is to apply ozone gas at low concentrations in cold storage rooms to control the growth of spoilage microorganisms on food products or in processing facility to reduce the environmental bacteria colonization rather than to inactivate or remove foodborne pathogens (Palou et al., 2002; Tzortzakis et al., 2007; Eglezos and Dykes, 2018).
Several publications are available which describe the use of gaseous ozone to inactivate foodborne pathogens. However, more information is needed to thoroughly evaluate the effectiveness and feasibility of using gaseous ozone to improve the microbial safety of frozen berries. Studies on baby spinach showed 1-log reduction of E. coli and Listeria spp. after being exposed to 10 ppm ozone gas for 2 min (Wani et al., 2015). As ozone sensitivity is commodity dependent, it is important to establish optimal application conditions for each food commodity (Karaca, 2010). For berries, Bialka and Demirci (2007a,c) investigated the effect of gaseous ozone on bacterial pathogens (Salmonella spp. and E. coli O157:H7). It is reported that gaseous ozone is most effective against these pathogens on raspberries, followed by blueberries, and the least effective on strawberries. As for the effect of gaseous ozone on viruses, Predmore et al. (2015) demonstrated ca. 2.5 and 4.0-log reductions of Murine norovirus-1 and Tulane virus respectively on fresh strawberry surfaces after treatment with 6 % wt/wt ozone for 30 min. In contrast, (Brié et al., 2018) reported more than a 3-log reduction of Murine norovirus-1 after a 1 min exposure to 3 ppm gaseous ozone; and limited effect was found on Hepatitis A virus. However, it is noted from the published data that different experimental set ups and units expressing gaseous ozone dose were used, which makes it difficult to compare the decontamination effect of gaseous ozone reported in different publications. Therefore, we here proposed guidelines on how to report gaseous ozone data and investigated the inactivation effect of gaseous ozone on fresh and more importantly on frozen strawberries against bacterial strains (Salmonella, Enterococcus faecium) and human norovirus surrogates. The effect of ozone concentration, treatment time and sensorial impact on strawberries were also reported.
Method and Materials
Strain Preparation
Three Salmonella enterica strains and one Enterococcus faecium strain (ATCC 8459) were used in this study. Salmonella enterica Typhimurium (LFMFP S883) is an environmental isolate kindly provided by Prof Venter from Pretoria University and deposited to LFMFP-Ghent University culture collection. Salmonella enterica Thompson (RM1987), a clinical isolate from a cilantro outbreak and Salmonella enterica Typhimurium (SL1344), a well-characterized streptomycin resistant strain, were kindly provided by Dr. Maria Brandl (ARS USDA). E. faecium (ATCC 8459) is a reference strain widely used in inactivation studies as a surrogate for Salmonella spp. (Bianchini et al., 2014). To prepare the inoculum, a loopful of stock culture (at −80°C) was cultured in 10 ml of brain heart infusion (BHI; Oxoid) broth at 37°C for 24 h. This was followed by another sub-culture in fresh BHI broth for a second overnight incubation. A cocktail of three Salmonella strains was prepared by combining the same amount (e.g., 1 ml) of each culture to yield a final concentration of 108 CFU/ml. The same concentration (108 CFU/ml) was reached for E. faecium at stationary phase.
Virus surrogates were used to study the efficiency of gaseous ozone against human norovirus, considering the difficulties in cultivating human norovirus (Ettayebi et al., 2016). Murine norovirus-1 (MNV-1, CW1) and bacteriophage MS2 (ATCC 15597-B1) were used in the present study. MNV-1 strain, Passage 7, (originally supplied by Prof. H. W. Virgin, Washington University, St. Louis, MO, USA), was propagated on RAW 264.7 cells at a M.O.I. of 0.05 and stored at −80°C until use. The titer of MNV-1 (ca. 107 PFU/ml) was determined by standard plaque assay technique, as described previously (Wobus et al., 2004). Working stock of MS2 bacteriophage strain was prepared as described in ISO 10705-1 using E. coli K12 (ATCC 23631) strain as a bacterial host (ISO, 1995) and stored at −80°C until use. The titer of MS2 was determined by double layer plaque assay, which was ca. 1010 PFU/ml. Plaque assay protocols were described previously by Zhou et al. (2017).
Sample Preparation and Enumeration
Fresh strawberries were purchased from a local market in Ghent, Belgium. Strawberries were rinsed with tap water and the ones with no surface defects were selected for experiments. For each sample, 25 ± 1 g of strawberries (2–3 strawberries depending on the batch, cut into half to reach 25 g if necessary) were inoculated with a total of 100 μl inoculum distributed over 15 spots (a total of 300 μl was used for MNV, distributed over 45 spots) and dried in a biosafety cabinet for 1 h. Frozen samples were prepared by transferring the inoculated samples to an instant freezer (Techfrost JOF-23, Italy) for 1 h (internal temperature −18°C) prior to ozone treatment. Inoculated-fresh or frozen samples with no further treatment were used as control groups. For the enumeration of bacteria, samples were tenfold diluted in a filtered stomach bag with 225 ml Buffered Peptone Water (BPW; Oxoid) and stomached for 1 min. Viable cell populations were determined by plating serial dilutions on Xylose Lysine Desoxycholate Agar (XLD; Oxoid) and Slanetz and Bartley medium (SB; Oxoid) for the enumeration of Salmonella and E. faecium, respectively. XLD plates were incubated at 37°C for 24 h and SB plates were incubated at 37°C for 48 h before counting. For MNV-1 and MS2, viruses were recovered by adding 50 ml vegetable buffer (100 mM Tris, 50 mM glycine, 3% (m/v) beef extract, 50 mM MgCl2; pH 9.5) and quantified by standard plaque assay methods as mentioned above.
Generation, Delivery, and Removal of Gaseous Ozone
Generation of Ozone
Generation of ozone was performed in a laboratory set up as shown in Figure 1. Ozone was generated from high purity oxygen using a corona discharge ozone generator (Ozomat COM-AD-02, Anseros). The overall gas flow rate was set at 1 L/min and controlled by a mass flow meter (model No. SN-32649-12, Cole–Parmer). Ozone concentrations were measured every second using the ozone analyzer (GM-OEM, Anseros). The stainless-steel ozone chamber (192 × 240 × 300 mm) was connected to the generator by Teflon tubes with two L-shape valves allowing to regulate the path of ozone as needed (Figure 1).
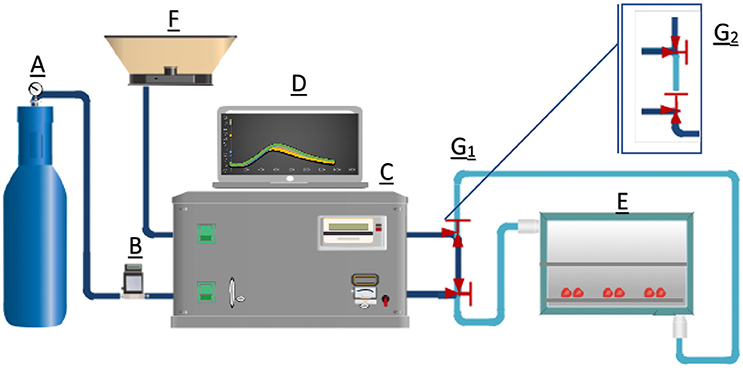
Figure 1. Diagram of gaseous ozone treatment setting. It includes an oxygen tank (A), a flow rate meter (B, 0–1 L/min), an ozone generator (C, 0–100% capacity), an ozone concentration monitor (D), an ozone reaction chamber (E), a ventilation hood (F), valves controlling the path of gas (G1,G2) and teflon connection tubes.
Delivery of Ozone
Delivery of ozone was controlled by two valves. When the valves were in position as shown in Figure 1G1, no gas was sent into the reaction chamber. As a result, concentration of ozone leaving the generator was measured and defined as the inlet gaseous ozone concentration. Once both valves were switched to the second position (Figure 1G2), ozone gas was sent to the chamber for treatment. Concentration of ozone leaving the chamber was measured and defined as the outlet gaseous ozone concentration. Prior to each run, the inlet ozone concentration was measured for 1 min before switching to the measurement of the outlet ozone concentration.
Removal of Ozone
was necessary to ensure safe working conditions for the laboratory staff. After the generator was turned off, oxygen gas or nitrogen gas was sent into the reaction chamber to dilute or remove the residual ozone.
Gaseous Ozone Concentration
Inlet ozone was measured by the ozone monitor and expressed in the unit of g/m3 under the standard temperature of 273.3 K (Kelvin) and the standard pressure of 101.3 kPa (Kilopascal). The following conversions of ozone concentration into different units were used:
Total ozone exposure (R, gram ozone per kilogram of strawberries) was calculated based on the concentration of inlet ozone (Cm, g/m3), flow rate (Q, l/min), treatment time (t, min), and weight of strawberries per trial (m, kg):
Gaseous Ozone Treatment
To determine the effect of ozone applied at different concentrations for different durations, fresh strawberries were treated with 1% wt/wt ozone in oxygen (ca. 14 g/m3) and 6% wt/wt ozone in oxygen (ca. 80 g/m3) for 5 and 30 min. For each trial, 75 g of inoculated fresh strawberries were loaded into the ozone chamber for treatment. After each treatment, residual ozone was flushed using oxygen (1 L/min) until the outlet concentration was below 5 g/m3. To evaluate the sensitivity of tested strains to the feed gas (pure oxygen) and ambient environment, groups of oxygen treated samples (40 min) were included.
To determine the effect of ozone on fresh and frozen strawberries at the same treatment condition, a total of 100 g strawberries (50 g frozen and 50 g fresh) were treated with 6% ozone for 5 min. The residual ozone in this experiment was flushed away with nitrogen gas at a higher flow rate, which eliminated the ozone residue within 1 min to shorten the treatment time. Fresh strawberries were considered as internal reference control to facilitate future comparison between the two settings (oxygen and nitrogen flushing). All the experiments were performed at ambient temperature. Temperature and relative humidity were measured by Easylog (EL-USB-2-Lcd, Lascar Electronics UK).
Sensory Trial
The sensory analysis was performed on non-inoculated fresh samples treated with 6% ozone for 5 min using a triangle test. Fresh strawberries were used as reference groups. Samples were stored at 4°C for 3 h, kept at room temperature for 45 min and de-calyxed before serving. During the evaluation, the panelists were presented with three coded samples, two of which were identical and another one was different. Twenty-two panelists were instructed to identify the odd sample and record the answer. Data was acquired through individual questionnaires.
Statistical Analysis
Different treatments were performed in triplicate (independent biological replicates on separate days). Microbial counts were transformed to log values to compare the results or calculate the log reductions. Differences between treatments was tested for their significance (P < 0.05) using SPSS Statistics 23 for Windows (SPSS Inc.). The Shapiro-Wilk test and Levene test were used to assess the normality and the equality of variance (P ≥ 0.05) of each data set, respectively. Independent t-tests were used to evaluate the differences between fresh and frozen strawberries. One-way analysis of variance (ANOVA) was used to test if there were any significant differences in results from different ozone concentrations and treatment durations, followed by the Tukey's honestly significant difference (HSD) as a post hoc test.
Results
Ozone Gas Profile
Ozone concentration was monitored for each treatment. The total ozone exposure was calculated using formula (2). Examples of typical ozone profiles during treatments of fresh strawberries are shown in Figure 2. The generator was turned off when the defined treatment time was reached, which corresponded to the highest concentration of ozone inside the reaction chamber. The chamber was kept closed while flushing with oxygen to remove the residual ozone until the outlet ozone concentration was below 5 g/m3 (Figure 2A). Hence, the final durations during which samples (75 g/trial) were in contact with ozone gas were 10.5, 39.9, 23.8, and 55.4 min on average, for the treatments at 1% for 5 min, 1% for 30 min, 6% for 5 min, and 6% for 30 min, respectively. The total ozone exposure was around 0.93, 6.00, 5.47, and 32.27 g/kg, respectively (Figure 2B). Experiments were performed at ambient temperature (22°C) and relative humidity inside the chamber was between 60 and 70%. A similar approach was followed for the second experimental protocol with 100 g of strawberries (fresh and frozen) treated with 6% ozone for 5 min. For this protocol, nitrogen gas was used to flush the residual ozone and the total treatment time was reduced to 6 min, which resulted in a total ozone exposure of 4.10 g/kg on average. Experimental temperature was on ca. 2°C lower than the first protocol and relative humidity decreased from 50 to 40% during the 5 min treatment.
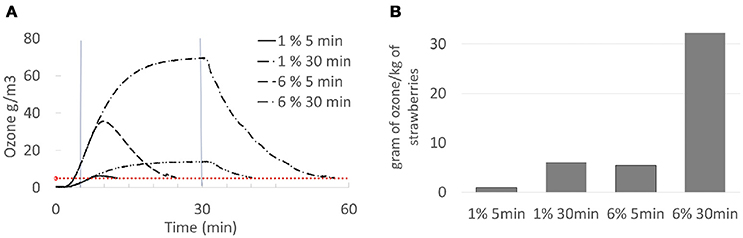
Figure 2. Profile of ozone concentration (A), the horizontal straight dashed line represents the level of 5 g/m3 of ozone, the vertical straight lines represent treatment of 5 min and 30 min, respectively. Total ozone exposure (B) during the treatments of fresh strawberries.
Effect of Gaseous Ozone at Different Concentrations and Durations
Fresh strawberries inoculated with Salmonella, E. faecium, MNV-1 and MS2 were treated with 1 and 6% ozone gas followed by oxygen flushing. Initial concentrations of strains recovered from fresh strawberries (control samples) were 6.53 ± 0.82, 6.39 ± 0.10, 6.20±0.10, and 4.17±0.71 log CFU/g for Salmonella, E.faecium, MS2 and MNV-1, respectively. Reductions of bacterial strains treated with different amounts of ozone are shown in Figure 3. For both bacterial strains, treatment with pure oxygen for 40 min had a minor effect (<0.4-log reduction) on the inoculated microbial load. Treatment with ozone gas at 6% for 30 min showed the highest reduction for both strains. The treatment with ozone achieved reductions of Salmonella ranging from 1.0 to 2.1-log. E. faecium appeared to be more resistant, with reductions ranging from 0.5 to 1.5-log. Extending treatment time at the higher concentration (6%) resulted in significant increases in the inactivation effect (P < 0.05) on bacterial strains. Results of viral reduction on fresh strawberries are also presented in Figure 3. Treatment with oxygen for 40 min resulted in a 0.7 ± 0.4-log reductions of MS2, while having no effect on MNV-1 when compared to the control group (P > 0.05). Highest reductions were achieved at 6% 30 min: 3.3-log for MS2 and 1.8-log for MNV-1. Treatments with 1% 5 min, 1% 30 min, and 6% 5 min resulted in reductions of 1.8, 2.1, and 2.2-log on MS2, respectively. MNV-1 showed more resistance under the same conditions with reductions of 1.1, 1.6, and 1.1-log, respectively. For all the tested strains, increasing the treatment duration at a certain ozone concentration or increasing the concentration without changing the treatment time enhanced the inactivation effect, but no proportional relationship was found between log reduction and total ozone exposure. For example, at the same concentration (1 or 6%), the ozone dose of the 30 min treatment was over five times higher compared to the dose of the 5 min treatment, while the inactivation was one to two times higher at most.
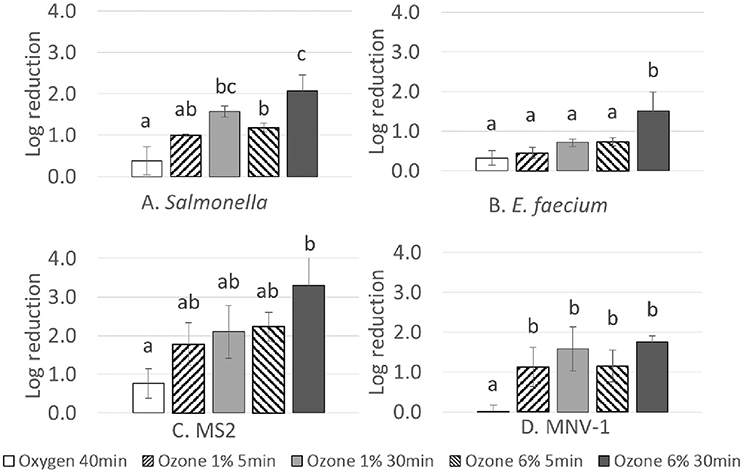
Figure 3. Log reductions of Salmonella, E. faecium, MS2 and MNV-1 on fresh strawberries under different gas treatment conditions with oxygen flushing (relative humidity: 60–70%). Each data bar represents an average value from independent replicates. Error bars indicate standard deviations. Lower case letters represent significant difference between treatments within each strain. Bars sharing no identical letter are significantly different from each other (P < 0.05).Total ozone exposure doses were around 0.93, 6.00, 5.47, and 32.27 grams of ozone per kilogram of strawberries for treatments at 1% for 5 min, 1% for 30 min, 6% for 5 min, and 6% for 30 min, respectively.
Effect of Gaseous Ozone on Fresh and Frozen Strawberries
After testing treatments with different amounts of ozone, the effect of gaseous ozone on frozen strawberries was evaluated using one selected condition. Fresh and frozen strawberries inoculated with Salmonella, E. faecium, MNV-1 and MS2 were treated with 6% ozone for 5 min, followed by nitrogen flushing for 1 min. Instead of log reduction, concentration of strains before and after ozone treatment are presented in Figure 4. Inoculated samples without gas treatment are presented as control groups, which were significantly higher than the treated groups for all the tested strains (P < 0.05). On frozen samples, the control level of Salmonella was about 1.5-log lower compared to the fresh ones due to the freezing process. Gaseous ozone treatment reduced Salmonella by 1.0 and 1.6-log on fresh and frozen strawberries, respectively. E. faecium was more resistant, 0.5 and 0.7-log reduction were achieved on fresh and frozen strawberries, respectively. For MNV-1, an average reduction of 0.7-log was obtained after treatment with 6% ozone for 5 min with no significant difference (P < 0.05) between fresh and frozen samples. For MS2, exposure to 6% ozone for 5 min resulted in a 0.9-log reduction on fresh strawberries and a 1.8-log reduction on frozen strawberries. Overall, the gaseous ozone showed more effect on frozen strawberries compared to the fresh strawberries, but the difference was only statistically significant for Salmonella (P = 0.04) and MS2 (P = 0.03).
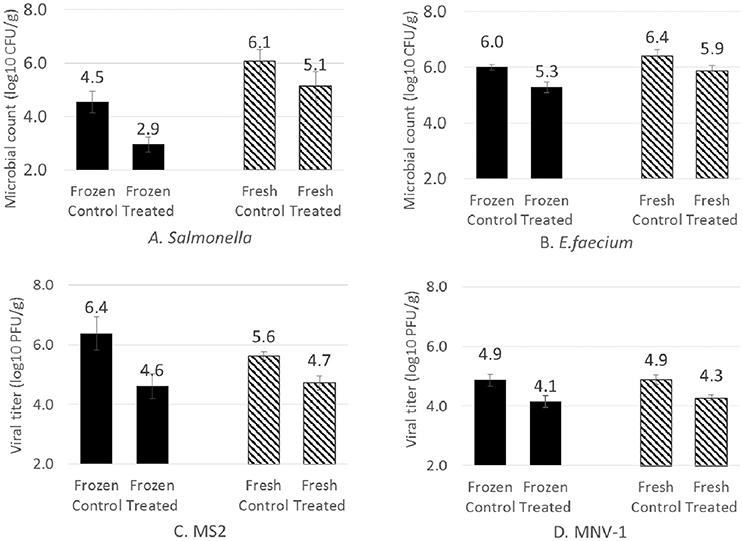
Figure 4. Concentrations of different strains on fresh and frozen strawberries before and after gaseous ozone treatment at 6% for 5 min, followed by 1 min nitrogen flushing (relative humidity: 40–50%). Each data bar represents an average value from independent replicates. Error bars indicate standard deviations. Total ozone exposure dose was 4.10 grams of ozone per kilogram of strawberries.
Comparison Between Two Settings (Oxygen and Nitrogen Flushing)
A comparison was made between fresh strawberries treated in the first protocol (oxygen flushing: Figure 3-ozone 6 % 5 min) and the second protocol (nitrogen flushing: Figure 4-Fresh) to evaluate the difference in inactivation resulting from the two settings. Oxygen was initially used to flush away the residual ozone, however, with the limited flow rate the flushing duration could take up to 40 min. Thus, the 40 min oxygen treatment (Figure 3) was included as a “worst case scenario” treatment condition to evaluate the effect of oxygen (as mentioned above, only a minor effect of < 0.4 log reduction was observed). Subsequently, the experiment was modified by substituting oxygen gas with nitrogen gas at a higher flow rate, which could flush away the residual ozone in 1 min. For both protocols, the effect of flushing gas was shown to be insignificant and the inactivation measured is thus mainly due to the ozone exposure. Log reductions on fresh strawberries of all tested strains using the oxygen flushing protocol (RH: 60–70%) were higher than the values using the nitrogen flushing protocol (RH: 40–50%). This could be explained by the fact that there was a difference in the relative humidity inside the treatment chamber and the total ozone exposure dose was 5.47 g/kg using the first protocol (oxygen) compared to 4.10 g/kg using the latter (nitrogen).
Sensory Results
Based on a chi-square distribution chart, the minimal number of correct responses required to reject the assumptions of “no difference” is 12 (significance at α = 0.05, number of panelists N = 22). In our experiment, the panel obtained 11 correct answers. Thus, no difference in taste and appearance was detected between ozone-treated and non-treated samples. Figure 5 shows a visual comparison between ozone-treated and non-treated fresh strawberries. No visual differences were found on strawberry flesh, but the treated samples showed certain degrees of de-colorization on the green calyxes. During the industrial production of frozen strawberries, samples are normally de-calyxed before being transferred to the individually quick frozen process. Hence, the de-colorization on the green part raises no potential application issues if ozone gas is used for decontamination of fresh strawberries intended to be frozen or on frozen strawberries.
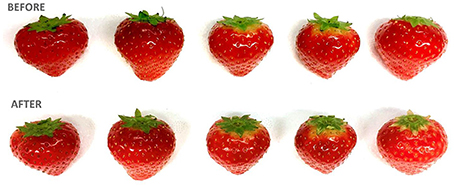
Figure 5. Visual changes of fresh strawberries and their calyxes before and after exposure to ozone gas at 0%, 1% for 5 min, 1% for 30 min, 6% for 5 min, and 6% for 30 min (from left to right).
Discussion and Conclusion
The microorganisms tested showed different susceptibilities to gaseous ozone. For bacteria, there are divergent conclusions on the sensitivity of Gram-positive and Gram-negative strains to ozone treatment (Khadre et al., 2001; Pascual et al., 2007; Horvitz and Cantalejo, 2014). Inactivation mechanism of ozone technology is mainly attributed to the oxidation reaction on different cellular components of the microorganism. Ozone molecules primarily come into contact with the cell surface and are able to attack various cell membrane components and damage the cell content constituents with increasing exposure time. Thus, theoretically, ozone should cause more damage on the cell wall of Gram-negative bacteria compared to Gram-positive bacteria (Khadre et al., 2001). Indeed, in our study, Salmonella appeared to be more sensitive than E. faecium. When comparing with other published results using gaseous ozone on strawberries (Bialka and Demirci, 2007b), our data on Salmonella showed higher reductions, which could be resulted from the use of selective media. Selective media was used in the present study considering strawberries had a total aerobic counts level of ca. 103 CFU/g. Concerns regarding stressed cells and nonculturable response should be further investigated to confirm the inactivation effect of gaseous ozone treatment (Dinu et al., 2009). For viruses, it was reported that ozone damaged the protein capsid but the RNA remained intact after the treatment (Pascual et al., 2007; Zhang et al., 2011; Predmore et al., 2015). In the present study, the reduction obtained for MNV-1 (1.8-log) was slightly lower than the one reported by Predmore et al. (2015) (ca. 2.0-log) under the same treatment condition (6% 30 min). Compared to MNV-1, MS2 was less resistant to gas treatment. Similar results on the susceptibility of MS2 to ozone treatment have been reported previously (Hall and Sobsey, 1993; Komanapalli and Lau, 1998; Khadre et al., 2001). Thus, MS2 may not be a suitable surrogate for norovirus when evaluating the effect of ozone gas treatment. It is recommended that more foodborne virus surrogates are investigated to further clarify the variability of viruses to gaseous ozone.
Interestingly, the results showed that increasing gaseous ozone treatment duration and concentration can improve the inactivation effect, but no proportional relationship was found. A similar observation was reported by Bialka et al. (2008) in a modeling study showing that the inactivation curve of Salmonella during gaseous ozone treatment followed a non-linear regression. Tailing effect was observed with the increase in treatment time. The assumption is that a certain population of bacteria remains resistant to ozone due to the adaptation of microorganisms to certain stresses (Van Boekel, 2002; Wani et al., 2015, 2016). Additionally, the flow rate in the present study was slow (1 L/min) which may limit the gas exchange on the strawberries' surfaces. Hence, flow rate is identified as a contributing factor in the inactivation effect.
Gaseous ozone was found to be more effective against microorganisms on frozen strawberries than fresh strawberries. To maintain the strawberries in a frozen state, only a short treatment time (5 min) was used in the present study. While the treated strawberries remain frozen, the relative humidity of the fruit surface may have changed and temperature difference indeed existed between the surface of frozen and fresh samples. Han et al. (2002) reported that the log reduction of E. coli O157:H7 on green peppers increased (from 1.0 to 3.5 log) with the increase of relative humidity (from 60 to 90%). The potential effect of relative humidity was also observed in our experiments on fresh strawberries treated under two experimental settings. Prior exposure to freezing process may also decrease the resistance of pathogens to gaseous ozone treatment. If verified, this could provide a possibility of combining other intervention strategies with gaseous ozone treatment to enlarge the inactivation effect. In summary, further experiments with adjusted relative humidity and different temperatures need to be performed to provide more information regarding the potential application of gaseous ozone on frozen berries at different stages during the production.
Although aqueous ozone was reported to be 5 times more efficient than gaseous ozone (Bialka and Demirci, 2007a), the application of gaseous ozone has more potential over aqueous ozone in the processing of berry products. For strawberries and blueberries, water washing or the use of liquid sanitizers can help to remove pathogens from the fruit surface but may also have negative effects on the quality and shorten the shelf life. In case of raspberries, being a very soft fruit not amenable to washing, gaseous ozone treatment is the only decontamination option. Furthermore, gaseous ozone may penetrate more easily into the hidden spots on the surfaces of these soft fruits. Ozone gas is already commercially used in cold storage rooms of fruit and vegetables (Horvitz and Cantalejo, 2014) to delay the outgrowth of spoilage yeast and fungi. This application uses a low concentration of ozone gas applied for a long time (days). In contrast, in the present study we aim at the inactivation rather than the growth inhibition of pathogens and we demonstrate that this application is feasible on both on fresh and frozen strawberries. Thus, this technology has the potential to be applied to frozen fruit during (intermediate) storage. It will contribute to the sustainability of the global frozen berry trade which is currently impacted by occasional outbreaks and loss of consumer trust in microbiological safety of these products. Other studies reported that gaseous ozone showed synergistic effects when combined with other technologies (vacuum cooling, pressured air or pulsed light beam) (Bialka et al., 2008; Shynkaryk et al., 2016). This warrants that further information is collected regarding the use of the gaseous ozone as part of a combination technology.
No sensorial impact was found on strawberry flesh using gaseous ozone treatment. Other studies on the color analysis of strawberries before and after ozone treatment also showed that the redness remained unchanged, while the calyxes became dry and bleached (Bialka and Demirci, 2007c; Pascual et al., 2007). During the preparation of strawberries intended to be frozen, samples are usually de-calyxed. Hence, the de-colorization on the green part raises no potential application issues for gaseous ozone being used on berries prior to or after freezing as a decontamination treatment. In contrast, other studies using gaseous ozone on leafy greens showed that the concentration and treatment duration needed to be strictly controlled to maintain the required product quality. Wani et al. (2016) concluded that the maximum ozone exposure to avoid quality defects for baby spinach and lettuce to avoid quality defects was 0.002 g/m3 for 10 min, which was much lower than the lowest concentration (14 g/m3) we used in the present study. Very importantly, (Onopiuk et al., 2017) reported that the beneficial properties of strawberries, such as vitamin C content and antioxidant capacity, remained unchanged after ozonation. Overall, the application of gaseous ozone will not affect the taste or visual quality of fresh and frozen strawberries.
Ozone can simply be generated from gas feed containing oxygen using UV-light or corona-discharge. However, within the limited number of publications on this topic, different units were used and experimental settings were sometimes poorly described (Tzortzakis and Chrysargyris, 2017). Hence, it is recommended that full details on the experimental set-up and design should be provided to increase the comparability and reproducibility of gaseous ozone inactivation data. The ozone concentration should be expressed in g/m3 or mg/L (weight of ozone in oxygen or air), or percentage of ozone by weight or volume to avoid the confusion of using ppm, which is normally used to describe the ozone concentration in water. Air-tight systems are required for gaseous ozone treatment since the inhalation of high concentration of ozone gas is dangerous (Glowacz and Rees, 2016). Thus, there is an inevitable time period needed to build up to and remove a certain amount of ozone gas in a closed environment to ensure workers' safety. This highlights the importance of reporting the efficiency of ozone gas transfer from the generator to the reaction chamber (come-up time). In order to compare, repeat, evaluate and apply the experimental findings in due time, primary guidelines are proposed which should be followed when reporting the data: (1) report the ozone concentration in the appropriate unit; (2) report the flow rate and type of feeding gas; (3) include the size of the treatment chamber; (4) report and optimize the sample size; (5) specify the come up and contact time; (6) describe the protocol for ozone removal and its duration; (7) document or control the relative humidity and temperature during the treatment.
In conclusion, gaseous ozone demonstrated an inactivation effect on fresh and frozen strawberries at all treatment conditions on four tested strains. To date, this is the first paper reporting inactivation results on frozen strawberries using gaseous ozone treatment. Taking into consideration that ozone gas is easily generated and has the benefit of being a treatment generating no chemical residues, it can be considered a very promising and sustainable processing technology applicable to fresh or frozen berries to control the number of foodborne pathogens. By optimizing the ozone exposure conditions, there is a potential to further increase the decontamination effect. To facilitate the future industrial application of gaseous ozone during minimal processing of berries, it would be of interest to investigate the survival or inactivation of foodborne pathogens or their surrogates on batches of berries in temperature and humidity-controlled storage rooms with prolonged ozone exposure.
Author Contributions
All the authors provided substantial contributions to the conception or design of the work. ZZ designed and performed all the laboratory research and drafted the work. SZ, FD, and MU revised the work critically. FC and IS provided the approval for publication of the content.
Conflict of Interest Statement
The authors declare that the research was conducted in the absence of any commercial or financial relationships that could be construed as a potential conflict of interest.
Acknowledgments
We would like to thank Michael Chys and Siméon Bourdoux for the technical supports regarding the ozone equipment and Ramize Xhaferi for her help in the microbial analysis.
References
Bernard, H., Faber, M., Wilking, H., Haller, S., Höhle, M., Schielke, A., et al. (2014). Large multistate outbreak of norovirus gastroenteritis associated with frozen strawberries, Germany, 2012. Eurosurveillance 19, 13–21. doi: 10.2807/1560-7917.ES2014.19.8.20719
Bialka, K. L., and Demirci, A. (2007a). Decontamination of Escherichia coli O157:H7 and Salmonella enterica on blueberries using ozone and pulsed UV-light. J. Food Sci. 72, M391–M396. doi: 10.1111/j.1750-3841.2007.00517.x
Bialka, K. L., and Demirci, A. (2007b). Efficacy of aqueous ozone for the decontamination of Escherichia coli O157:H7 and Salmonella on raspberries and strawberries. J. Food Prot. 70, 1088–1092. doi: 10.4315/0362-028X-70.5.1088
Bialka, K. L., and Demirci, A. (2007c). Utilization of gaseous ozone for the decontamination of Escherichia coli O157:H7 and Salmonella on raspberries and strawberries. J. Food Prot. 70, 1093–1098. doi: 10.4315/0362-028X-70.5.1093
Bialka, K. L., Demirci, A., and Puri, V. M. (2008). Modeling the inactivation of Escherichia coli O157:H7 and Salmonella enterica on raspberries and strawberries resulting from exposure to ozone or pulsed UV-light. J. Food Eng. 85, 444–449. doi: 10.1016/j.jfoodeng.2007.08.007
Bianchini, A., Stratton, J., Weier, S., Hartter, T., Plattner, B., Rokey, G., et al. (2014). Use of Enterococcus faecium as a surrogate for Salmonella enterica during extrusion of a balanced carbohydrate-protein meal. J. Food Prot. 77, 75–82. doi: 10.4315/0362-028X.JFP-13-220
Bouwknegt, M., Verhaelen, K., Rzezutka, A., Kozyra, I., Maunula, L., von Bonsdorff, C.-H., et al. (2015). Quantitative farm-to-fork risk assessment model for norovirus and hepatitis a virus in European leafy green vegetable and berry fruit supply chains. Int. J. Food Microbiol. 198, 50–58. doi: 10.1016/j.ijfoodmicro.2014.12.013
Brié, A., Boudaud, N., Mssihid, A., Loutreul, J., Bertrand, I., and Gantzer, C. (2018). Inactivation of murine norovirus and hepatitis a virus on fresh raspberries by gaseous ozone treatment. Food Microbiol. 70, 1–6. doi: 10.1016/j.fm.2017.08.010
CCDC (2013). Foodborne Outbreak Online Database. Centers for Disease Control and Prevention. (Accessed June, 2017). Available Online at: http://wwwn.cdc.gov/foodborneoutbreaks/Default.aspx.
Dinu, L.-D., Delaquis, P., and Bach, S. (2009). Nonculturable response of animal enteropathogens in the agricultural environment and implications for food safety. J. Food Prot. 72, 1342–1354. doi: 10.4315/0362-028X-72.6.1342
EFSA (European Food Safety Authority) (2014). Scientific opinion on the risk posed by pathogens in food of non-animal origin. Part 2 (Salmonella and Norovirus in berries). EFSA J. 12:3706. doi: 10.2903/j.efsa.2014.3706
Eglezos, S., and Dykes, G. A. (2018). Reduction of environmental listeria using gaseous ozone in a cheese processing facility. J. Food Prot. 81, 795–798. doi: 10.4315/0362-028X.JFP-17-361
Ettayebi, K., Crawford, S. E., Murakami, K., Broughman, J. R., Karandikar, U., Tenge, V. R., et al. (2016). Replication of human noroviruses in stem cell–derived human enteroids. Science 353, 1387–1393. doi: 10.1126/science.aaf5211
Glowacz, M., and Rees, D. (2016). The practicality of using ozone with fruit and vegetables. J. Sci. Food Agric. 96, 4637–4643. doi: 10.1002/jsfa.7763
Goodburn, C., and Wallace, C. A. (2013). The microbiological efficacy of decontamination methodologies for fresh produce: a review. Food Control 32, 418–427. doi: 10.1016/j.foodcont.2012.12.012
Gulati, B. R., Allwood, P. B., Hedberg, C. W., and Goyal, S. M. (2001). Efficacy of commonly used disinfectants for the inactivation of calicivirus on strawberry, lettuce, and a food-contact surface. J. Food Prot. 64, 1430–1434. doi: 10.4315/0362-028X-64.9.1430
Guzel-Seydim, Z. B., Greene, A. K., and Seydim, A. (2004). Use of ozone in the food industry. LWT-Food Sci. Tech. 37, 453–460. doi: 10.1016/j.lwt.2003.10.014
Hall, R. M., and Sobsey, M. D. (1993). Inactivation of hepatitis a virus and MS2 by ozone and ozone-hydrogen peroxide in buffered water. Water Sci. Technol. 27, 371–378. doi: 10.2166/wst.1993.0377
Han, Y., Floros, J., Linton, R., Nielsen, S., and Nelson, P. (2002). Response surface modeling for the inactivation of Escherichia coli O157: H7 on green peppers (Capsicum annuum) by ozone gas treatment. J. Food Sci. 67, 1188–1193. doi: 10.1111/j.1365-2621.2002.tb09475.x
Hirneisen, K. A., Markland, S. M., and Kniel, K. E. (2011). Ozone inactivation of norovirus surrogates on fresh produce. J. Food Prot. 74, 836–839. doi: 10.4315/0362-028X.JFP-10-438
Horvitz, S., and Cantalejo, M. (2014). Application of ozone for the postharvest treatment of fruits and vegetables. Crit. Rev. Food Sci. Nutr. 54, 312–339. doi: 10.1080/10408398.2011.584353
ISO (1995). Water Quality: Detection and Enumeration of Bacteriophages. 1. Enumeration of F-Specific RNA Bacteriophages. ISO 10705-1. Geneva: International organization for standardization.
Jacxsens, L., Uyttendaele, M., Luning, P., and Allende, A. (2017). “Food safety management and risk assessment in the fresh produce supply chain,” in IOP Conference Series: Materials Science and Engineering. (Bristol: IOP Publishing), 012020.
Karaca, H. (2010). Use of ozone in the citrus industry. Ozone Sci. Eng. 32, 122–129. doi: 10.1080/01919510903520605
Khadre, M., Yousef, A., and Kim, J. G. (2001). Microbiological aspects of ozone applications in food: a review. J. Food Sci. 66, 1242–1252. doi: 10.1111/j.1365-2621.2001.tb15196.x
Komanapalli, I., and Lau, B. (1998). Inactivation of bacteriophage k, Escherichia coli, and Candida albicans by ozone. Appl. Microbiol. Biotechnol. 49, 766–769. doi: 10.1007/s002530051244
Li, D., Keuckelaere, A., and Uyttendaele, M. (2015). Fate of foodborne viruses in the “farm to fork” chain of fresh produce. Compr. Rev. Food Sci. Food Saf. 14, 755–770. doi: 10.1111/1541-4337.12163
Li, Y., and Wu, C. (2013). Enhanced inactivation of Salmonella Typhimurium from blueberries by combinations of sodium dodecyl sulfate with organic acids or hydrogen peroxide. Food Res. Intl. 54, 1553–1559. doi: 10.1016/j.foodres.2013.09.012
Lou, F., Neetoo, H., Chen, H., and Li, J. (2011). Inactivation of a human norovirus surrogate by high-pressure processing: effectiveness, mechanism, and potential application in the fresh produce industry. Appl. Environ. Microbiol. 77, 1862–1871. doi: 10.1128/AEM.01918-10
O'Donnell, C., Tiwari, B. K., Cullen, P., and Rice, R. G. (2012). Ozone in Food Processing. Chichester: John Wiley and Sons.
Onopiuk, A., Półtorak, A., Wyrwisz, J., Moczkowska, M., Stelmasiak, A., Lipinska, A., et al. (2017). Impact of ozonisation on pro-health properties and antioxidant capacity of ‘Honeoye’strawberry fruit. CyTA-J. Food 15, 58–64. doi: 10.1080/19476337.2016.1212273
Palou, L., Crisosto, C. H., Smilanick, J. L., Adaskaveg, J. E., and Zoffoli, J. P. (2002). Effects of continuous 0.3 ppm ozone exposure on decay development and physiological responses of peaches and table grapes in cold storage. Posthar. Biol. Technol. 24, 39–48. doi: 10.1016/S0925-5214(01)00118-1
Pascual, A., Llorca, I., and Canut, A. (2007). Use of ozone in food industries for reducing the environmental impact of cleaning and disinfection activities. Trends Food Sci. Tech. 18, S29–S35. doi: 10.1016/j.tifs.2006.10.006
Predmore, A., Sanglay, G., Li, J., and Lee, K. (2015). Control of human norovirus surrogates in fresh foods by gaseous ozone and a proposed mechanism of inactivation. Food Microbiol. 50, 118–125. doi: 10.1016/j.fm.2015.04.004
Pyatkovskyy, T., Shynkaryk, M., Yousef, A., and Sastry, S. K. (2017). Fresh produce sanitization by combination of gaseous ozone and liquid sanitizer. J. Food Eng. 210, 19–26. doi: 10.1016/j.jfoodeng.2017.03.031
Ramos, B., Miller, F., Brandão, T. R., Teixeira, P., and Silva, C. L. (2013). Fresh fruits and vegetables—an overview on applied methodologies to improve its quality and safety. Innovative Food Sci. Emerg. Technol. 20, 1–15. doi: 10.1016/j.ifset.2013.07.002
Shynkaryk, M. V., Pyatkovskyy, T. I., Yousef, A. E., and Sastry, S. K. (2016). Gaseous ozone treatment of baby spinach within the existing production chain for inactivation of Escherichia coli O157: H7. J. Food Eng. 191, 10–18. doi: 10.1016/j.jfoodeng.2016.06.025
Tavoschi, L., Severi, E., Niskanen, T., Boelaert, F., Rizzi, V., Liebana, E., et al. (2015). Food-borne diseases associated with frozen berries consumption: a historical perspective, European Union, 1983 to 2013. Eurosurveillance 20:21193. doi: 10.2807/1560-7917.ES2015.20.29.21193
Tzortzakis, N., and Chrysargyris, A. (2017). Postharvest ozone application for the preservation of fruits and vegetables. Food Rev. Intl. 33, 270–315. doi: 10.1080/87559129.2016.1175015
Tzortzakis, N., Singleton, I., and Barnes, J. (2007). Deployment of low-level ozone-enrichment for the preservation of chilled fresh produce. Posthar. Biol. Technol. 43, 261–270. doi: 10.1016/j.postharvbio.2006.09.005
Van Boekel, M. A. (2002). On the use of the Weibull model to describe thermal inactivation of microbial vegetative cells. Int. J. Food Microbiol. 74, 139–159. doi: 10.1016/S0168-1605(01)00742-5
Van Boxstael, S., Habib, I., Jacxsens, L., De Vocht, M., Baert, L., Van de Perre, E., et al. (2013). Food safety issues in fresh produce: bacterial pathogens, viruses and pesticide residues indicated as major concerns by stakeholders in the fresh produce chain. Food Control 32, 190–197. doi: 10.1016/j.foodcont.2012.11.038
Wani, S., Barnes, J., and Singleton, I. (2016). Investigation of potential reasons for bacterial survival on ‘ready-to-eat’leafy produce during exposure to gaseous ozone. Posthar. Biol. Technol. 111, 185–190. doi: 10.1016/j.postharvbio.2015.08.007
Wani, S., Maker, J. K., Thompson, J. R., Barnes, J., and Singleton, I. (2015). Effect of ozone treatment on inactivation of Escherichia coli and Listeria sp. on spinach. Agriculture 5, 155–169. doi: 10.3390/agriculture5020155
Wobus, C. E., Karst, S. M., Thackray, L. B., Chang, K. O., Sosnovtsev, S. V., Belliot, G., et al. (2004). Replication of Norovirus in cell culture reveals a tropism for dendritic cells and macrophages. PLoS Biol. 2:e432. doi: 10.1371/journal.pbio.0020432
Zhang, H. Q., Barbosa-Cánovas, G. V., Balasubramaniam, V. B., Dunne, C. P., Farkas, D. F., and Yuan, J. T. (2011). Nonthermal Processing Technologies for Food. Chichester: John Wiley and Sons.
Zhou, Z., Zuber, S., Cantergiani, F., Butot, S., Li, D., Stroheker, T., et al. (2017). Inactivation of viruses and bacteria on strawberries using a levulinic acid plus sodium dodecyl sulfate based sanitizer, taking sensorial and chemical food safety aspects into account. Int. J. Food Microbiol. 257, 176–182. doi: 10.1016/j.ijfoodmicro.2017.06.023
Keywords: gaseous ozone, norovirus, Salmonella, non-thermal processing, frozen strawberries, inactivation, guidelines
Citation: Zhou Z, Zuber S, Cantergiani F, Sampers I, Devlieghere F and Uyttendaele M (2018) Inactivation of Foodborne Pathogens and Their Surrogates on Fresh and Frozen Strawberries Using Gaseous Ozone. Front. Sustain. Food Syst. 2:51. doi: 10.3389/fsufs.2018.00051
Received: 22 May 2018; Accepted: 26 July 2018;
Published: 21 August 2018.
Edited by:
Joshua B. Gurtler, Agricultural Research Service (USDA), United StatesReviewed by:
Joseph D. Eifert, Virginia Tech, United StatesAudrey Kreske, Restaurant Brands International, Inc., United States
James Patrick Folsom, Lonza, United States
Copyright © 2018 Zhou, Zuber, Cantergiani, Sampers, Devlieghere and Uyttendaele. This is an open-access article distributed under the terms of the Creative Commons Attribution License (CC BY). The use, distribution or reproduction in other forums is permitted, provided the original author(s) and the copyright owner(s) are credited and that the original publication in this journal is cited, in accordance with accepted academic practice. No use, distribution or reproduction is permitted which does not comply with these terms.
*Correspondence: Zijin Zhou, emlqaW4uemhvdUB1Z2VudC5iZQ==