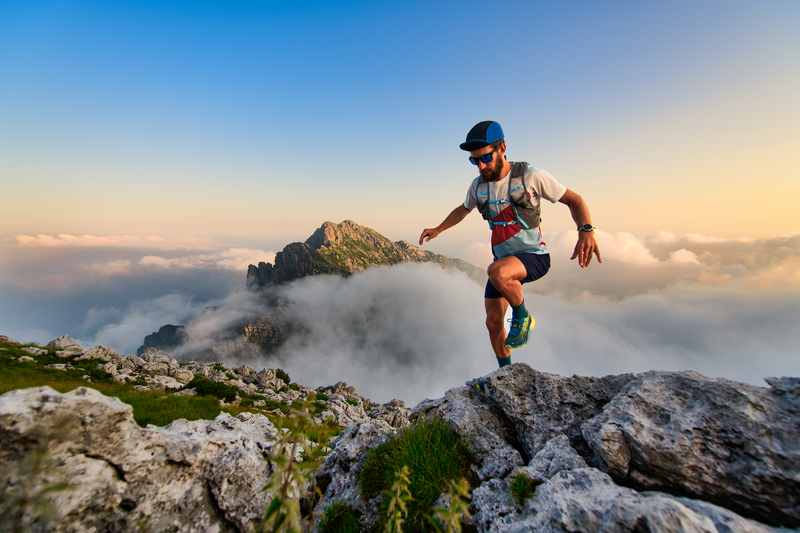
95% of researchers rate our articles as excellent or good
Learn more about the work of our research integrity team to safeguard the quality of each article we publish.
Find out more
ORIGINAL RESEARCH article
Front. Sustain. Food Syst. , 30 May 2018
Sec. Waste Management in Agroecosystems
Volume 2 - 2018 | https://doi.org/10.3389/fsufs.2018.00020
This article is part of the Research Topic New processes for Nutrient Recovery from Wastes View all 14 articles
Converting poultry litter (PL) to biochar and applying the biochar to cropland as a soil amendment may be a best approach for recovering nutrients from solid biowastes while minimizing nutrient runoff losses from the treated field. To evaluate the potential of PL-derived biochar as a slow-release phosphorus (P) fertilizer, the speciation, lability, and bioavailability of P in PL and the derived biochars were examined and compared. Raw PL and its derived biochars through 300–600°C slow pyrolysis were analyzed for total P (TP), inorganic P (IP), and organic P (OP) contents. The TP was fractionated into readily, generally, moderately, low, and non-labile pools by sequential extraction with different solutions. The TP was further assessed for bioavailability using batch extraction by water, Olsen, Bray-1, Mehlich-3, and 1 M HCl extractants. The P species in biochars were characterized using solid-state 31P nuclear magnetic resonance (NMR) techniques. The results indicate that during pyrolysis OP in PL was transformed to IP and water-soluble P to low labile forms such as hydroxyapatite and oxyapatite especially at higher temperature. Bray-1 and Mehlich-3 were appropriate extractants for evaluating the immediate to medium-term available and the long-term available P in biochar, respectively. Converting PL to biochar through ≤450°C pyrolysis significantly reduced the water-soluble proportion and the lability of P but did not compromise the long-term P bioavailability, resulting in a P-enriched, slow release soil amendment that would minimize the P runoff risks following field application. The promising results need to be further validated in soil-biochar-plant systems.
Poultry production (domestic fowl rearing for meat and eggs) is a crucial economic activity in nearly all countries to furnish affordable diet protein and suppress hunger. Intensive and concentrated bird rearing, however, generates vast volumes of litter waste (mixture of feces, sheddings, and bedding materials) that requires appropriate disposal (Bolan et al., 2010). The U.S. poultry industry, for example, generates annually more than 550 million dry tons of litter waste (Coker, 2017). Poultry litter (PL) contains high contents of organic carbon (OC, ~380 g kg−1) and the plant nutrients nitrogen (N, ~40 g kg−1), phosphorus (P, ~15 g kg−1), and potassium (K, ~38 g kg−1) (Guo et al., 2009a) and is predominantly disposed of through land application as an organic fertilizer. The P:N ratio (e.g., 1:2–1:3) of PL is much higher than the typical crop nutrient requirement (e.g., 1:6–1:8) (Sadras, 2005) and therefore, application of PL at N-based agronomic rates would result in over-fertilization of P, which is subject to runoff losses to natural water bodies. In regions with concentrated poultry production, repeated and excess application of PL to cropland has introduced substantial loads of P and N nutrients to local water systems, causing eutrophication, and general water quality degradation issues (NASA, 2016). Phosphorus exists in PL principally in labile forms (e.g., water-soluble, 0.5 M NaHCO3-extractable, and organic P) (Dou et al., 2000; Li et al., 2014). Up to 50% of the P in PL is water extractable (Dou et al., 2000) and can be rapidly released into the soil water via rainfall following land application (Guo et al., 2009b). To reduce the risks of P runoff from land-applied PL, it is critical to decrease the P water extractability and release rate of the organic fertilizer.
Biochar is a promising soil amendment capable of persistently enhancing soil quality through ameliorating soil physical, chemical, and biological properties (Guo et al., 2016). Converting agricultural byproducts and other organic residues to biochar and utilizing the biochar as a soil amendment may be a best management practice for recovering nutrients and sustaining soil quality. Research demonstrates the feasibility of manufacturing nutrients-enriched biochar by pyrolysis of PL for promoting crop growth (Chan et al., 2008; Revell et al., 2012; Mierzwa-Hersztek et al., 2016). Depending on the pyrolysis conditions especially the temperature and time duration, the yield of biochar ranged from 45 to 60 mass% of the feedstock PL (Song and Guo, 2012). Nearly 100% of the PL-P was recovered in biochar (Song and Guo, 2012). Pyrolysis significantly transformed the P in PL and reduced the water extractable portion of P from 19.5% of total P in raw PL to <7.0% in biochar. As the pyrolysis temperature was elevated from 300 to 600°C, the portion of 0.01 M HCl-extractable P in the PL-derived biochar products decreased from >55% of total P to <16% (Song and Guo, 2012). Pyrolysis at 400°C converted the labile P in raw PL to Mg/Ca phosphate minerals in biochar and reduced the water-soluble P from 2.95 g kg−1 in raw PL to 0.17 g kg−1 in biochar (Wang et al., 2015). In PL biochar, orthophosphate was predominant while organic phosphate (a major form of P in raw PL) was barely present. Furthermore, release of P from PL biochar in water and neutral soils was significantly slower and steadier than from raw PL (Wang et al., 2015). Relative to raw PL, the derived biochar is a slow P release fertilizer (Dai et al., 2016). To date the transformation processes of P in PL during pyrolysis have not been fully understood. The existing forms of P in PL-derived biochar are not clear, and the bioavailability of the biochar P lacks assessment. The objective of this study was to examine the transformation of P in speciation and bioavailability during converting PL to biochar under varying pyrolysis conditions, aiming to optimize the pyrolysis operation for producing a slow release yet efficiently available P soil amendment from animal wastes.
Poultry litter was procured from an industrial facility in Seaford, DE that processes raw PL collected from local broiler farms into a marketable fertilizer product via a series of physical and mechanical operations. The PL predominantly in <4 mm granules contained 92.3 wt% of dry matter and 7.7 wt% of moisture. The dry matter was comprised of 71.5 wt% organic constituents and 28.5 wt% ash minerals. On the dry mass basis the PL contained total N 30.7, total P 13.7, and total K 41.8 g kg−1. More nutrient composition information of the PL can be found in Table 1 of Song and Guo (2012). The PL was used as received.
The PL was converted to biochar through slow pyrolysis at varied peak temperatures, with duplicate trials performed at each selected temperature. Briefly, 650 g of the granular PL were weighed into a metal canister (11 cm i.d. × 13 cm height) to its volume at loose packing. The canister was covered by its metal lid that had a 5-mm hole in the center. The canister was placed in an Isotemp muffle furnace (Thermo Fisher Scientific, Inc., Suwanee, GA) and heated at a pre-determined temperature (300–600°C) until the pyrolysis was complete, as indicated by no further visible smokes escaping from the furnace. The furnace was able to raise its internal temperature at 20°C/min and maintain a pre-set constant temperature with continuous power supply. Pyrolysis of PL inside the canister started when the furnace temperature reached above 250°C, signified by visible smokes (pyrolysis vapors) emitted out of the 4-cm gas vent in the top panel of the furnace. It took 130–370 min for the pyrolysis of PL in the canister to be complete, the time shortened as the peak pyrolysis temperature increased in the range of 300–600°C.
Once the complete pyrolysis was achieved, the furnace was switched off, and the canister was taken out and cooled to the room temperature with immediate sealing of the lid hole with a piece of metal tape. The biochar in the canister was then transferred into a Ziploc plastic bag and stored in a dark cabinet at 22°C prior to further characterization. More details of the biochar preparation methods can be found in Song and Guo (2012). The peak temperatures 300, 350, 400, 450, 500, 550, and 600°C were used to produce biochars from PL through pyrolysis operations varying in temperature, the most important parameter that controls the yield and quality of biochar derived from a specific feedstock (Guo et al., 2012). The PL-derived biochar samples are hereafter referred to as C300, C350, C400, C450, C500, C550, and C600, respectively.
The PL and the derived biochars were ground to <0.15 mm and stored in brown glass vials. Total P (TP) and its constituents inorganic P (IP) and organic P (OP) of the samples were analyzed. The contents of TP were determined by acid digestion and colorimetric P measurement (Song and Guo, 2012). For each sample, duplicate measurements were conducted. All the laboratory wares were of glass or Teflon and were acid-washed. In operation, 0.20 g of a sample were transferred into a 45-mL Teflon digestion cylinder (Parr Instrument Company, Moline, IL), followed by addition of 5.0 mL Milli-Q water and 5.0 mL concentrated HNO3. Procedure blanks without sample addition were included. After gentle swirling for mixing, the cylinder was capped and placed into a digestion case and microwaved at 0.6 KW for 2.5 min. The digestion bomb was left in the microwave oven to cool to nearly room temperature, then opened, and the digestate in the cylinder was fully transferred into a 100-mL volumetric flask with several times of Milli-Q water rinsing. The digestate in the flask was brought to 100 mL by adding Milli-Q water, well-mixed, and passed through a 0.45-μm glass fiber filter to remove any particulates. The clear digestate was then analyzed for TP concentrations using the standard phosphomolybdate blue method (Murphy and Riley, 1962). The IP contents were determined by extracting the samples with 1 M HCl at 1:50 solid/solution ratio under room temperature rotary shaking for 24 h, centrifuging the extract at 4,080 × g for 20 min., passing the supernatant through a 0.2-μm syringe filter, and measuring the P content of the filtered extract using the phosphomolybdate blue method (de Jonge et al., 1993). The OP contents were computed as the difference between TP and IP of a sample. The method detection limit was 3.0 mg kg−1.
To examine the fractionation of TP in different pools showing distinct leachability and bioavailability, the PL and biochar samples in duplicates were sequentially extracted by Milli-Q water, 0.5 M NaHCO3, 0.1 M NaOH, and 1 M HCl, each at 1:50 solid/solution ratio (Qian and Jiang, 2014). At each sequential extraction step, the solid/solution slurry was continuously agitated by 30 rpm rotary shaking at room temperature for 24 h and centrifuged at 4080 × g for 20 min to separate the mixture into supernatant and pellet. The supernatant was passed through a 0.2-μm syringe filter and collected in a 50-mL volumetric flask; the pellet was washed twice each by 10 mL Milli-Q water and the rinsate was combined with the supernatant. The next sequential extraction step was then started by adding a new extractant solution and re-suspending the pellet. Any P remaining in the pellet after the sequential 1 M HCl extraction was treated as residual P (non-labile, non-bioavailable) of the original sample. The extract in the volumetric flask was brought to volume with Milli-Q water and analyzed for concentrations of total dissolved P (Pt), dissolved organic P (Po), dissolved inorganic P (Pi), dissolved inorganic orthophosphate P (Pr), and dissolved inorganic polyphosphate P (Px). Prior to bringing to the volume, the NaHCO3– and NaOH-extracts were adjusted to pH just below 8 by drop-adding concentrated HCl with phenolphthalein as the indicator. The Pt, Pi, and Pr concentrations of the sequential extracts were measured following the phosphomolybdate blue method after acidic K2S2O8 autoclave digestion, H2SO4 autoclave digestion, and no pretreatment of the extracts, respectively (Wang et al., 2015). Once again, procedure blanks without sample addition were included. All standard P solutions for establishing the calibration curves were subject to the same digestion processes as the extracts. The method detection limit was 0.91 mg kg−1. The Po was computed as the difference between Pt and Pi (Po = Pt – Pi) and Px as the difference between Pi and Pr (Px = Pi – Pr). For the HCl-extracts, only Pr was measured; no organic P or inorganic polyphosphate P was expected in this extract and therefore, Pr = Pi = Pt was assumed (Qian and Jiang, 2014).
To further estimate the bioavailability of P in the PL and biochar samples, the fractions of Mehlich-3 P, Bray-1 P, and Olsen P were measured by batch extraction methods (Pierzynski, 2000). Briefly, 0.5-g aliquots of the samples were extracted separately by 25 mL of Mehlich-3 extractant (0.2 M CH3COOH + 0.015 M NH4F + 0.013 M HNO3 + 0.001 M EDTA + 0.25 M NH4NO3), Bray-1 extractant (0.025 M HCl in 0.03 M NH4F), and Olsen extractant (0.5 M NaHCO3, pH adjusted to 8.5) at room temperature for 24 h under continuous rotary shaking and the extracts were analyzed for Pt concentrations following the phosphomolybdate blue method after acidic K2S2O8 autoclave digestion. For each sample, duplicate measurements were conducted. Procedure blanks without sample addition were included. The method detection limit was 0.61 mg kg−1.
To identify the existing species of P in PL-derived biochar, solid-state 31P single-pulse (SP) and 31P{1H} cross-polarization (CP) magic-angle spinning (MAS) nuclear magnetic resonance (NMR) spectra of the biochar samples were collected on a 400 MHz Varian Unity Inova spectrometer (Varian, Inc., Palo Alto, CA) at operating frequencies of 161.8 and 399.8 MHz for 31P and 1H, respectively. Spectra were collected using a Varian/Chemagnetics T3-type probe, with samples contained in 7.5 mm (o.d.) normal wall ZrO2 rotors. The 31P{1H} CP/MAS spectra were obtained at the spinning rate of 5 kHz with CP contact time of 1 ms using the same probe. The CP kinetic curves were measured at a spinning rate of 5 kHz with contact times varying from 0.3 to 5 ms and irradiation under the n = −1 sideband match condition. For all CP/MAS spectra, the transverse 1H field (γB1, H) was ramped over approximately 5 kHz, centered near the first sideband match at a 42 kHz 1H field. Proton decoupling (CW) was employed during acquisition of all 31P{1H} CP/MAS spectra. The 31P chemical shifts (δiso, P) were reported relative to external 85% H3PO4 solution, using hydroxyapatite as a secondary reference set to δiso, P = 2.65 ppm.
All chemical characterization data are expressed as means of duplicate measurements, with standard deviations showing the analytical precision. The results of analytical procedure blanks were incorporated in sample data processing. The relative abundance of various P forms is articulated as the percentage relative to the TP concentrations of the PL and biochar samples. Variations in speciation and bioavailability of P in PL and the derived biochars from different pyrolysis temperatures were statistically evaluated at the level of significance α = 0.05 following the analysis of variance (ANOVA) and the Fisher least significant difference (LSD) methods.
The PL contained 13.7 g kg−1 TP, of which 67.6% was IP and 32.4% belonged to OP. In the biochar products, the TP content was elevated to 22.7 g kg−1 for C300 and 30.5 g kg−1 for C600, increasing steadily as the pyrolysis temperature was raised (Table 1). The proportion of IP in the TP was also elevated to 87.0% for C300 and further to 99.7% for C450. When the pyrolysis temperature was increased to 500°C and above, the “nomial” proportion of IP (extractable by 1 M HCl) decreased slightly from the peak value of nearly 100 to 96.6% in C500 and to 89.2% in C600 (Table 1). Correspondingly, the OP proportion decreased from 32.4% in raw PL to 13.0% in C300 and further to nearly null (0.25%) in C450. As the pyrolysis temperature was elevated to 500°C and higher, the “nomial” OP proportion showed a slightly increasing trend in the resulting biochar products, reaching 10.8% in C600 (Table 1). The changes in IP and OP proportions between the biochars generated at 300–600°C with a 50°C interval were statistically significant, especially when the pyrolysis temperature was ≤450°C (Table 1).
Table 1. Contents of total phosphorus (TP) and its inorganic phosphorus (IP) and organic phosphorus (OP) fractions in poultry litter (PL) and the derived biochars.
In raw PL, water-soluble P accounted for nearly half (49.5%) of the TP, while the remaining half was shared by NaHCO3-extractable, NaOH-extractable, HCl-extractable, and residual P at 9.0, 5.5, 17.1, and 18.9%, respectively (Figure 1). In PL-derived biochars, the portion of water-soluble P decreased radically to 11.7% of TP in C300, 8.7% in C350, 5.5% in C400, and further to ~2.4% in biochars produced at ≥450°C pyrolysis temperatures. The proportion of NaHCO3-extractable P, on the contrary, increased significantly to 20.4% in C300, fluctuated between 17.7 and 19.2% in C350, C400, C450, and C500. The proportion then descended to 10.8% in C550 and 9.7% in C600. Similarly, the NaOH-extractable P increased from 5.5% of TP in raw PL to the peak proportion of 13.2% (of TP) in C300 and then declined gradually to 9.2% in C350, 7.5% in C400, 4.2% in C450, 1.6% in C500, and to <0.2% in biochars generated at ≥550°C pyrolysis temperatures (Figure 1). The sequentially HCl-extractable P increased its proportion from 17.1% of TP in raw PL to 33.9% in C300, 52.2% in C350, and further to 60–72% in biochars produced from ≥400°C pyrolysis. The residual P in C300 accounted for 20.8% of the TP, a percentage close to that of raw PL (18.9%). The proportion decreased to 10.8% in C350 and reached the bottom level of 5.4% in C450. Further elevation of the pyrolysis temperature caused back increases of the residual P proportion in the resulting biochar products, to 16.1% in C600 (Figure 1). The residual P proportion profile is consistent with that of “nominally” OP (Table 1). In general, converting to biochar through pyrolysis significantly reduced the highly labile, highly leachable, and highly bioavailable (primarily water-soluble) P in PL.
Figure 1. Fractionation of phosphorus (P) in poultry litter (PL) and the derived biochars into water-extractable, NaHCO3-extractable, NaOH-extractable, HCl-extractable, and residual forms. Data are means of duplicate measurements. The coefficients of variation of the duplicate measurements are within 3%.
Inorganic P (Pi, including orthophosphate-P (Pr) and polyphosphate-P (Px)) dominated the water-soluble P in PL and the derived biochars, accounting for >89% of the total dissolved P (Pt) extractable by water (Table 2). In PL, for example, the water-soluble P consisted of 84.5% Pr, 4.5% Px, and 11.0% Po (dissolved organic P). The proportion of Po in water-soluble P from C300 decreased to 2.9%, whereas the proportion of Px increased to 10.4% and Pr to 86.7% (Pi 97.1%). The Po proportion of water-soluble P in biochar products decreased gradually as the production temperature was elevated in the range of 300–600°C; the Px proportion also decreased steadily; yet the Pr proportion and the overall Pi proportion increased accordingly. Organic P disappeared from the C500 water extract; only Pr was present in water-soluble P of biochars produced at ≥550°C pyrolysis temperatures (Table 2). Sequential extraction of PL and the biochars by NaHCO3 after water showed the composition of 75.2% Pr, 6.9% Px, and 17.9% Po in the extractable P from PL and 86.7% Pr, 13.3% Px, and 0.0% Po in the extractable P from C300. Organic P completely disappeared from the sequential NaHCO3 extracts of biochars, while Px was only present in extracts from low-temperature biochars and decreased its proportion in products from higher temperature pyrolysis. In the sequential NaHCO3 extracts of C450, Px nulled and Pr accounted for 100% of Pi and Pt (Table 2). The composition of P in the sequential NaOH extracts demonstrated similar variation trends. The strongly alkaline NaOH solution is rather efficient for extracting organics from solid matrix. It was assumed that all remaining Po in the PL and biochars after the sequential NaHCO3 extraction would be recovered in the NaOH extracts. Consequently, Po was the principal form of P in the NaOH extracts from PL, accounting for 65.6% of the Pt (Table 2). In the NaOH extracts from C300, however, Po was merely 3.9% of the Pt, and proportion decreased to 0.0% in the extracts from C400. Meanwhile, Px decreased its proportion of 24.4% in the C300 extracts to 1.1% in the C400 extracts. In the NaOH extracts from C450, Pr became the single form of dissolved P (Table 2).
Table 2. Composition of water-soluble, NaHCO3-extractable, and NaOH-extractable phosphorus (P) fractions in poultry litter (PL) and the derived biochars.
Of the total extractable P from the four-stage sequential extraction (by water, NaHCO3, NaOH, and HCl), Pr, Px, and Po accounted for 82.0, 4.8, and 13.2%, respectively in PL. The composition changed to 89.9% Pr, 9.0% Px, and 1.1% Po in C300 (Figure 2). In biochars produced at higher pyrolysis temperatures, both the extractable Po and Px demonstrated a steadily declining trend in proportion. The extractable Po and Px disappeared and Pr was the only extractable P form in biochars prepared through ≥500°C pyrolysis (Figure 2). In consistent with Table 1, the results suggest transformation of OP to polyphosphate-P and further to orthophosphate-P during converting PL to biochar. The transformation was facilitated as the pyrolysis temperature was elevated.
Figure 2. Composition of total extractable phosphorus (P) in poultry litter (PL) and the derived biochars. Data are means of duplicate measurements. Superscript letters denote significance of difference between treatment levels.
Water was able to extract 2.4–11.7% of TP in PL-derived biochars, the proportion decreasing readily to the minimum as the pyrolysis temperature was elevated in the range of 300°C to 450°C and above (Figure 3). In comparison, water extracted 49.5% of TP from raw PL under the same conditions. Clearly, conversion to biochar dramatically reduced the water solubility of P in PL. The Olsen solution (0.5 M NaHCO3, pH 8.5) performed slightly better than water in recovering P from PL and the derived biochars. The Olsen P accounted for 53.3% of TP in raw PL and 13.8–30.0% in biochars. Nevertheless, the pyrolysis temperature effect on the proportion of Olsen P in PL-derived biochar was not straightforward. For C300, the proportion was 21.6%. The proportion increased to 30.0% for C350 yet then declined for biochars produced at higher pyrolysis temperatures, to 28.2% for C400 and 13.8% for C600 (Figure 3). The Bray-1 extractant (0.025 M HCl in 0.03 M NH4F) acted more efficiently than water and the Olsen extractant in recovering P from PL and the biochars. The solution was able to recover 73.3% of TP from raw PL and 17.0–57.8% of TP from the biochars. Furthermore, the proportion of Bray-1 extractable P in biochars decreased gradually as the pyrolysis temperature was elevated in the range of 300–600°C (Figure 3). Compared with the Bray-1 extractant, the more corrosive Mehlich-3 solution (0.2 M CH3COOH + 0.013 M HNO3 + 0.015 M NH4F + 0.001 M EDTA + 0.25 M NH4NO3) extracted 89.9% of TP from PL and 35.0–80.4% of TP from the biochars, among which C350 demonstrated the highest proportion (80.4%) of Mehlich-3 P while C550 and C600 possessed the drastically lower (35–50%) fractions (Figure 3). In contrast, the strongly acidic extractant 1 M HCl recovered 67.6% of TP from PL yet much higher proportions (87.0–99.8%) from the biochars. All IP but little OP in soils and soil amendments was assumed extractable by 1 M HCl. The high recovery of P by HCl extraction (Figure 3) implicated that inorganic phosphate was the predominant P form in biochars. Overall, water, Bray-1, and 1 M HCl extracted significantly different proportions of P from PL and the biochars especially those generated at ≤450°C, but the alkaline Olsen solution and the corrosive Mehlich-3 agent failed in this function (Figure 3).
Figure 3. Extractability (indicating leachability and potential bioavailability) of phosphorus (P) in poultry litter (PL) and the derived biochars by water, Olsen extractant, Bray-1 extractant, Mehlich-3 extractant, and 1 M HCl. Data are means of duplicate measurements. Letters above bars denote significance of difference between treatment levels.
The 31P SP/MAS NMR spectra of the PL-derived biochars are illustrated in Figure 4. A broad peak at the chemical shift δP−31 = 2.7 ppm with full width at half maximum (FWHM) of 6.8 ppm was observed for C300. The chemical shift is fully consistent with that of hydroxyapatite (Ca10(PO4)6(OH)2), but the FWHM is much broader than well-crystalline hydroxyapatite. This peak was therefore assigned to poorly crystalline hydroxyapatite (Jaeger et al., 2006; He et al., 2007; Vyalikh et al., 2017). The same peak was also observed for C350 and C400. As the pyrolysis temperature was elevated to 450°C an additional shoulder at δP−31 = −0.3 ppm was observed for the biochar product, suggesting some poorly crystalline hydroxyapatite was transformed to a new species. This shoulder became more and more pronounced in biochars produced from higher temperature pyrolysis. Meanwhile, the major peak at δP−31 = 2.7 turned to be narrower with increasing the pyrolysis temperature, indicating facilitated crystallization of hydroxyapatite under higher temperature heating. At 600°C, two well-separated NMR peaks at δP−31 = 2.7 and −0.3 ppm were identified (Figure 4). Assignment of the peak at δP−31 = 2.7 ppm to hydroxyapatite is further supported by the CP kinetics of C600 shown in the 31P{1H} CP/MAS NMR spectra (Figure 5), since only hydroxyapatite possesses such phosphate/proton environments that would yield stronger signal at a longer CP contact time (i.e., 5,000 μs) than at a shorter CP contact time (e.g., 300 and 1,000 μs). The assignment of the shoulder peak at δP−31 = −0.3 ppm was not straightforward. The compound from which the peak stemmed was most likely a dehydrated product of hydroxyapatite after heating. In the 31P{1H} CP/MAS spectra of C600, the peak at δP−31 = −0.3 ppm shows no CP signals (Figure 5), implicating there are no protons associated with the P species. Though the peak occurred at a chemical shift rather close to that of β-tricalcium phosphate (Ca3(PO4)2) (Sakka et al., 2013), formation of tetracalcium phosphate (Ca4(PO4)2O) and β-tricalcium phosphate (Ca3(PO4)2) from dehydrating hydroxyapatite required a much higher temperature (e.g., >1000°C) environment (Greenwood, 2014). At 600°C, however, hydroxyapatite is ready to be dehydrated to form oxyapatite: Ca10(PO4)6(OH)2 → Ca10(PO4)6O + H2O (Greenwood, 2014). Therefore, the δP−31 = −0.3 ppm peak was assigned to oxyapatite (Figure 4).
Figure 4. 31P SP/MAS NMR spectra of poultry litter-derived biochars from different pyrolysis temperatures. All spectra were collected at a spinning rate of 5 KHz and pulse delay of 120 s.
Figure 5. 31P{1H} CP/MAS spectra of the biochar C600 derived from poultry litter (PL) through 600°C pyrolysis. The spectra were collected at varied CP contact times, spinning rate of 5 KHz, and a pulse delay of 2 s.
Pyrolysis of PL enriched the inherent non-volatile elements including P in biochar products. The enrichment became more prominent at higher pyrolysis temperatures in response to the decreasing biochar yield (Song and Guo, 2012). The enrichment factor of P (the ratio of TP content between biochar and PL) for C300 was computed at 1.66. For C450 and C600, the factor increased to 1.94 and 2.23, respectively (Table 1). In addition to enrichment, speciation transformation of P was also evident. In PL, both inorganic P species (e.g., dicalcium phosphate (CaHPO4), amorphous tricalcium phosphate (Ca3(PO4)2, and octacalcium phosphate (Ca8H2(PO4)6·5H2O)) and organic P species (e.g., phytates, phospholipids, and nucleic acids) are significantly present (Hunger et al., 2004; Turner and Leytem, 2004; Toor et al., 2005; He et al., 2008; Li et al., 2014). Most of the OP in PL was converted to inorganic forms during pyrolysis, as indicated by the drastic decreases in the proportion of OP yet abrupt increases in the proportion of IP in the derived biochars relative to raw PL (Table 1). Transformation of OP to metal/mineral-associated inorganic species was widely observed when converting sewage sludge, manures, and other solid biowastes to biochar through pyrolysis (Huang et al., 2017). Decomposition of OP was promoted by increasing the pyrolysis temperature. At 450°C, nearly all OP in PL was converted to inorganic P species, with the product C450 showing an OP proportion close to 0% (Table 1). The null presence of OP in biochars generated from ≥450°C pyrolysis was validated by the sequential extraction analyses, in which OP was barely recovered from these biochars by the extractants water, NaHCO3, and NaOH (Table 2; Figure 2). In Table 1, the unexpected increasing OP proportion for biochars produced at higher pyrolysis temperatures (C500, C550, and C600) was calculated based on the difference between TP and IP. As the pyrolysis temperature was elevated to 450°C and above, however, IP in the biochar products became more recalcitrant and less extractable by 1 M HCl. This is supported by the sequential extraction results, showing increased proportions of residual P in biochars generated from ≥450°C pyrolysis (Figure 1). The “nominal” OP in C500, C550, and C600 (Table 1) was actually residual P (unextractable by 1 M HCl).
Indeed, transformation of OP to IP occurred during pyrolysis of PL to biochar. The transformation was initially to inorganic polyphosphates (i.e., condensed P forms including pyrophosphates, polyphosphates, and metaphosphates) and further to inorganic orthophosphates at higher pyrolysis temperature. Using solution 31P NMR spectroscopic techniques, polyphosphates were detected in raw PL (Turner and Leytem, 2004; Dou et al., 2009), sewage sludge (Qian and Jiang, 2014; Huang and Tang, 2015), and other solid biowastes (Huang et al., 2017). Relative to raw PL, biochars generated at low pyrolysis temperature (e.g., C300) showed notably reduced proportion of extractable OP and correspondingly increased proportion of polyphosphate-P (Figure 2), suggesting transformation of OP to initially inorganic polyphosphates during pyrolysis. Elevating the pyrolysis temperature resulted in biochar products possessing decreased proportions of extractable polyphosphate-P yet increased proportions of orthophosphate-P (Figure 2), implicating further conversion of polyphosphates to orthophospahtes. Raw PL is abundant in ash minerals, with the molar Ca/P ratio greater than 2.0 (Song and Guo, 2012). Seemingly, high temperature and sufficient metal ion supply facilitates transformation of polyphosphates to orthophosphates. Different forms of P vary in water solubility, mobility, and phytoavailability. Converting PL to biochar would substantially alter the environmental fate and transport of P following land application.
Conversion of PL to biochar greatly reduced the water-soluble P fraction (Figure 1), which is the most mobile and plant available P portion in PL. Runoff P losses are largely controlled by and directly correlated with the water-soluble P content of land-applied solid wastes (Shreve et al., 1995; Hart et al., 2004; White et al., 2010). Evidently, soil amendment with biochar instead of raw PL would minimize the highly-concerned P runoff risks. The sequentially-used extractants water, NaHCO3, NaOH, and HCl were designed to recover readily labile P, generally labile P (adsorbed on crystalline mineral surfaces), moderately labile P (associated with carbonates and Fe/Al oxides or in organic particulates), and low labile P (bound in Ca-minerals), respectively, in soils and solid residues (Hedley et al., 1982; Dou et al., 2000; He et al., 2006). Likely, the readily labile, water-soluble P in PL was transformed mainly to low labile and residual P (e.g., calcium phosphate minerals such as hydroxyapatite and oxyapatite) and marginally to generally/moderately labile P (e.g., amorphous Ca3(PO4)2 surface precipitate on calcium carbonate and phosphate surface complexes on Fe/Al oxides) (Figure 1). Phosphate surface precipitates and Fe/Al-oxide complexes were identified in PL using solid state MAS and CP-MAS 31P NMR techniques (Hunger et al., 2004). Transformation of these two phases of phosphate to Ca-bound phosphates (e.g., hydroxyapatite) and further to residual P (e.g., oxyapatite) occurred possibly at higher temperature in the presence of available calcium (Figure 1). Overall, the labile portion of P (sequentially extractable by water, NaHCO3, and NaOH; readily to moderately labile; and immediately to medium-term plant available) was significantly reduced during converting PL to biochar; and the reduction became greater as the pyrolysis temperature was elevated in the range of 300–600°C. Similar P transformation trends were observed for pyrolysis of sewage sludge to biochar at different temperatures ranging from 400°C to 800°C (Qian and Jiang, 2014).
The labile portion of P in PL and the biochars was dominated by orthophosphates, with polyphosphates and organic P as minor forms (Table 2; Figure 2). For PL, all the three forms of P (Pr, Px, and Po) were present in the water-soluble, NaHCO3-extractable, and NaOH-extractable fractions. Organic P was the principal form of P in the NaOH-extractable fraction, suggesting significant existence of P-containing particulate organic matter in PL (Table 2). Due to the thermal decomposition of OP to IP, Po was detected only in the water-soluble and NaOH-extractable fractions from the biochars generated at lower pyrolysis temperature (≤450°C). Polyphosphates existed predominantly in the water-soluble P pool of biochars and fully disappeared in products from ≥550°C pyrolysis. Using coupled sequential extraction and solution 31P NMR techniques, Qian and Jiang (2014) detected polyphosphates in sewage sludge and low pyrolysis temperature (400–600°C) biochar products but not in high temperature (800°C) biochars.
The bioavailability of P in soil is commonly estimated by batch extraction using a specific chemical extractant (Pierzynski, 2000). For example, the Olsen solution (0.5 M NaHCO3, pH 8.5) was introduced to extract phytoavailable P from neutral, alkaline, and calcareous soils by enhancing the dissolution of Ca-phosphates. The Bray-1 extractant was designed to recover water-soluble and adsorbed forms of P in pH <7.5 soils. The Mehlich-3 extractant were developed to remove adsorbed and Fe/Al oxides-complexed phosphates and other elements from acidic and neutral soils (Elrashidi, 2001). Lucero et al. (1998) found that both Bray-1 and Mehlich-3 extracts were effective to evaluate excess P in PL-fertilized soils. In the present study, the raw PL had a pH value of 7.1 and the biochars of 9.5–11.5, increasing with the pyrolysis temperature (Song and Guo, 2012). It is notable that all the chemical extractants recovered more P from PL and the biochars than water, and their effectiveness for extracting P from biochars followed the order: Olsen < Bray-1 < Mehlich-3 < HCl (Figure 3). The Mehlich-3 solution was more efficient than HCl in extracting P from raw PL, probably due to the abundance of OP (Table 1) that would be precipitated in 1 M HCl. The Bray-1 extractable P demonstrated a variation trend highly consistent with that of the labile P fraction (extractable by water, NaHCO3, and NaOH) among the PL and the derived-biochars (Figure 1), suggesting the solution is an appropriate extractant for evaluating the labile P content of PL-derived biochars. A large portion of HCl-extractable, Ca-bound P is low labile yet long-term available to plants (Qian and Jiang, 2014) and therefore, Mehlich-3 was considered a proper solution for recovering overall bioavailable P from biochar. The Mehlich-3 extraction was able to reflect the changes in residual P fraction and general P recalcitrance of biochars produced at different pyrolysis temperatures (Figure 1). It is noteworthy that chemical extraction cannot reflect the interactions among soil, biochar, and plants tht influence the P bioavailabity of field applied biochar and therefore, the lability and bioavailability of P in PL-derived biochars as evaluated by the present batch and sequential extraction methods need to be validated in soil-biochar-plant systems.
To date most phosphate compounds and organic P species in soil, solid biowastes, and biochars remain unknown due to the matrix complexity, P chemical diversity, and technology restriction. In addition to solid-state 31P NMR techniques, other methods such as liquid 31P NMR, X-ray absorption near edge structure (XANES) spectroscopy, Fourier transform infrared spectroscopy (FTIR), and X-ray diffraction (XRD) spectroscopy have been used to characterize P compounds in environmental samples (Toor et al., 2005; He et al., 2007; Dou et al., 2009; Li et al., 2014; Qian and Jiang, 2014; Huang and Tang, 2015; Huang et al., 2017). Identified P chemicals are limited to phytate, dicalcium phosphate, tricalcium phosphate, struvite (NH4MgPO4·6H2O), hydroxyapatite, and broadly pyrophosphates (Hunger et al., 2008; Qian and Jiang, 2014; Huang and Tang, 2015; Huang et al., 2017). Hydroxyapatite does not exist in raw PL (Hunger et al., 2004). Instead, octacalcium phosphate, a precursor of hydroxyapatite, is present in PL (Hunger et al., 2008). The present 31P NMR analysis indicates that hydroxyapatite was formed during pyrolysis of PL to biochar. Improved crystallization of hydroxyapatite occurred as the pyrolysis temperature was elevated. At 450°C and above, a portion of hydroxyapatite was dehydrated and transformed to possibly oxyapatite (Figure 4). Both hydroxyapatite and oxyapatite are water insoluble and belongs to the low labile P pool in sequential extraction. The speciation transformation supports the chemical extraction results that the solubility, lability, and bioavailability of P generally decreased in biochars produced from higher temperature pyrolysis.
Nearly all P in the feedstock was recovered during pyrolytic conversion of PL to biochar. Relative to raw PL, the derived biochar was substantially P-enriched and might demonstrate a total P content doubling that of the original feedstock. During pyrolysis, OP in PL was decomposed to IP, initially to polyphosphates and subsequently to orthophosphates at higher temperature. Orthophosphate-P was the predominant form of P in PL-derived biochars. In the products manufactured at ≥450°C pyrolysis temperature, OP fully disappeared and polyphosphate-P became barely detectable. Overall, pyrolysis transformed the P in raw PL to much less labile forms (e.g., hydroxyapatite and oxyapatite) in biochar. The lability of P in biochars decreased as the pyrolysis temperature increased in the range of 300–600°C. Among the biochars generated at different temperatures, C450 possessed the smallest pool of residual P, suggesting 450°C is the optimal temperature for converting PL to biochar with maximum P bioavailability. The Bray-1 extractant was an appropriate solution for recovering the immediately to medium-term available P in biochar, while the Mehlich-3 solution was suitable for evaluating the overall P bioavailability of soil-applied biochar. The bioavailability of P in PL-derived biochars needs to be further assessed in soil-biochar-plant systems. In addition to P lability and bioavailability, the remarkable N losses and potential stable C recovery should also be considered in selecting an optimal pyrolysis temperature for converting PL to biochar.
MG designed the experiments, synthesized the data, and drafted the paper. WL developed the research topic, participated in sample analysis, interpreted the spectroscopic results, and helped prepare the manuscript. XF carried out the spectroscopic analysis of the biochar samples, processed the spectral information, and reviewed the manuscript. WS conducted chemical characterization of the samples and reviewed the draft.
The authors declare that the research was conducted in the absence of any commercial or financial relationships that could be construed as a potential conflict of interest.
This research was financially supported by National Natural Science Foundation of China (through Grant No. 41571130061) and the Delmarva Land Grant Institution Cooperative Seed Grant program.
Bolan, N. S., Szogi, A. A., Chuasavathi, T., Seshadri, B., Rothrock, M. J., and Panneerselvam, P. (2010). Uses and management of poultry litter. Worlds. Poult. Sci. J. 66, 673–698. doi: 10.1017/S0043933910000656
Chan, K. Y., Van Zwieten, L., Meszaros, I., Downie, A., and Joseph, S. (2008). Using poultry litter biochars as soil amendments. Aust. J. Soil Res. 46, 437–444. doi: 10.1071/SR08036
Coker, C. (2017). Poultry litter digestion. Biocycle 58, 50–51. Available online at: https://www.biocycle.net/2017/09/07/poultry-litter-digestion/
Dai, L., Li, H., Tan, F., Zhu, N., He, M., and Hu, G. (2016). Biochar: a potential route for recycling of phosphorus in agricultural residues. Glob. Change Biol. Bioenergy 8, 852–858. doi: 10.1111/gcbb.12365
de Jonge, V. N., Engelkes, M. M., and Bakker, J. F. (1993). Bio-availability of phosphorus in sediments of the western Dutch Wadden Sea. Hydrobiologia 253, 151–163. doi: 10.1007/BF00050735
Dou, Z., Toth, J. D., Galligan, D. T., Ramberg, C. F., and Ferguson, J. D. (2000). Laboratory procedures for characterizing manure phosphorus. J. Environ. Qual. 29, 508–514. doi: 10.2134/jeq2000.00472425002900020019x
Dou, Z., Toth, J. D., Wang, Y., Sharpley, A. N., Boyd, S. E., Chen, C. R., et al. (2009). Phosphorus speciation and sorption-desorption characteristics in heavily manured soils. Soil Sci. Soc. Am. J. 73, 93–101. doi: 10.2136/sssaj2007.0416
Elrashidi, M. A. (2001). Selection of an Appropriate Phosphorus Test for Soils. Lincoln, NE: USDA-NRCS Soil Survey Laboratory.
Greenwood, C. (2014). Physicochemical Modifications to Bone Mineral. dissertation/Ph.D. thesis, Cranfield University, Cranfield.
Guo, M., He, Z., and Uchimiya, S. M. (2016). “Introduction to biochar as an agricultural and environmental amendment,” in Agricultural and Environmental Applications of Biochar: Advances and Barriers, ed M. Guo et al. (Madison, WI: Soil Science Society of America), 1–14.
Guo, M., Labreveux, M., and Song, W. (2009a). Nutrient release from bisulfate-amended phytase-diet poultry litter under simulated weathering conditions. Waste Manage. 29, 2151–2159. doi: 10.1016/j.wasman.2009.02.012
Guo, M., Shen, Y., and He, Z. (2012). “Poultry litter-based biochar: preparation, characterization, and utilization,” in Applied Research in Animal Manure Management: Challenges and Opportunities beyond the Adverse Environmental Impacts, ed Z. He (Hauppauge, NY: Nova Science Publishers), 171–202.
Guo, M., Tongtavee, N., and Labreveux, M. (2009b). Nutrient dynamics of field-weathered Delmarva poultry litter: implications for land application. Biol. Fertil. Soils 45, 829–838. doi: 10.1007/s00374-009-0397-4
Hart, M. R., Quin, B. F., and Nguyen, M. L. (2004). Phosphorus runoff from agricultural land and direct fertilizer effects: a review. J. Environ. Qual. 33, 1954–1972. doi: 10.2134/jeq2004.1954
He, Z., Honeycutt, C. W., Cade-Menun, B. J., Senwo, Z. N., and an d Tazisong, I. A. (2008). Phosphorus in poultry litter and soil: enzymatic and nuclear magnetic resonance characterization. Soil Sci. Soc. Am. J. 72, 1425–1433. doi: 10.2136/sssaj2007.0407
He, Z., Honeycutt, C. W., Xing, B., McDowell, R. W., Pellechia, P. J., and Zhang, T. (2007). Solid-state Fourier transform infrared and 31P nuclear magnetic resonance spectral features of phosphate compounds. Soil Sci. 172, 501–515. doi: 10.1097/SS.0b013e318053dba0
He, Z., Toor, G. S., Honeycutt, C. W., and Sims, J. T. (2006). An enzymatic hydrolysis approach for characterizing labile phosphorus forms in dairy manure under mild assay conditions. Bioresour. Technol. 97, 1660–1668. doi: 10.1016/j.biortech.2005.07.021
Hedley, M. J., White, R. E., and Nye, P. H. (1982). Plant-induced changes in he rhizosphere of rape (Brassica napus var. Emerald) seedlings: III. Changes in L value, soil phosphate fractions and phosphatase activity. New Phytol. 91, 45–56. doi: 10.1111/j.1469-8137.1982.tb03291.x
Huang, R., Fang, C., Lu, X., Jiang, R., and Tang, Y. (2017). Transformation of phosphorus during (hydro)thermal treatments of solid biowastes: reaction mechanisms and implications for P reclamation and recycling. Environ. Sci. Technol. 51, 10284–10298. doi: 10.1021/acs.est.7b02011
Huang, R., and Tang, Y. (2015). Speciation dynamics of phosphorus during (hydro)thermal treatments of sewage sludge. Environ. Sci. Technol. 49, 14466–14474. doi: 10.1021/acs.est.5b04140
Hunger, S., Cho, H., Sims, J. T., and Sparks, D. L. (2004). Direct speciation of phosphorus in alum-amended poultry litter: solid-state 31P NMR investigation. Environ. Sci. Technol. 38, 674–681. doi: 10.1021/es034755s
Hunger, S., Sims, J. T., and Sparks, D. L. (2008). Evidence for struvite in poultry litter: effect of storage and drying. J. Environ. Qual. 37, 1617–1625. doi: 10.2134/jeq2007.0331
Jaeger, C., Maltsev, S., and Karrasch, A. (2006). Progress of structural elucidation of amorphous calcium phosphate (ACP) and hydroxyapatite (HAp): disorder and surfaces as seen by solid state NMR. Key Eng. Mater. 309–311, 69–72. doi: 10.4028/www.scientific.net/KEM.309-311.69
Li, G., Li, H., Leffelaar, P. A., Shen, J., and Zhang, F. (2014). Characterization of phosphorus in animal manures collected from three (dairy, swine, and broiler) farms in China. PLoS ONE 9:e102698. doi: 10.1371/journal.pone.0102698
Lucero, D. W., Martens, D. C., McKenna, J. R., and Starner, D. E. (1998). Comparison of Mehlich 3- and bray 1-extractable phosphorus levels in a Starr clay loam amended with poultry litter. Commun. Soil Sci. Plant Anal. 29, 1133–1142. doi: 10.1080/00103629809370014
Mierzwa-Hersztek, M., Gondek, K., and Baran, A. (2016). Effect of poultry litter biochar on soil enzymatic activity, ecotoxicity and plant growth. Agric,. Ecosyst. Environ. Appl. Soil Ecol. 105, 144–150. doi: 10.1016/j.apsoil.2016.04.006
Murphy, J., and Riley, H. P. (1962). A modified single solution method for the determination of phosphate in natural waters. Anal. Chim. Acta 27, 31–36. doi: 10.1016/S0003-2670(00)88444-5
NASA (2016). How farms affect the Chesapeake Bay's water: image of the day. National Aeronautics and Space Administration. Washington, DC. Available online at: https://earthobservatory.nasa.gov/IOTD/view.php?id=88523
Pierzynski, G. (2000). Methods of Phosphorus Analysis for Soils, Sediments, Residuals, and Waters. Southern Cooperative Series Bulletin. Raleigh, NC: North Carolina State University.
Qian, T., and Jiang, H. (2014). Migration of phosphorus in sewage sludge during different thermal treatment processes. ACS Sustainable Chem. Eng. 2, 1411–1419. doi: 10.1021/sc400476j
Revell, K. T., Maguire, R. O., and Agblevor, F. A. (2012). Influence of poultry litter biochar on soil properties and plant growth. Soil Sci. 177, 402–408. doi: 10.1097/SS.0b013e3182564202
Sadras, V. O. (2005). The N:P stoichiometry of cereal, grain legume and oilseed crops. Field Crops Res. 95, 13–29. doi: 10.1016/j.fcr.2005.01.020
Sakka, S., Bouaziz, J., and Ayed, F. B. (2013). “Mechanical properties of biomaterials based on calcium phosphates and bioinert oxides for applications in biomedicine,” in Advances in Biomaterials Science and Biomedical Applications, ed R. Pignatello (London, UK: InTech Open, Ltd), 23–50.
Shreve, B. R., Moore, P. A., Daniel, T. C., Edwards, D. R., and Miller, D. M. (1995). Reduction of phosphorus in runoff from field-applied poultry litter using chemical amendments. J. Environ. Qual. 24, 106–111. doi: 10.2134/jeq1995.00472425002400010015x
Song, W., and Guo, M. (2012). Quality variations of poultry litter biochars generated at different pyrolysis temperatures. J. Anal. Appl. Pyrolysis 94, 138–145. doi: 10.1016/j.jaap.2011.11.018
Toor, G. S., Peak, J. D., and Sims, J. T. (2005). Phosphorus speciation in broiler litter and turkey manure produced from modified diets. J. Environ. Qual. 34, 687–697. doi: 10.2134/jeq2005.0687
Turner, B. L., and Leytem, A. B. (2004). Phosphorus compounds in sequential extracts of animal manures: chemical speciation and novel fractionation procedure. Environ. Sci. Technol. 38, 6101–6108. doi: 10.1021/es0493042
Vyalikh, A., Elschner, C., Schulz, M. C., Mai, R., and Scheler, U. (2017). Early stages of biomineral formation—A solid-state NMR investigation of the mandibles of minipigs. Magnetochemistry 3:39. doi: 10.3390/magnetochemistry3040039
Wang, Y., Lin, Y., Chiu, P., Imhoff, P. T., and Guo, M. (2015). Phosphorus release behaviors of poultry litter biochar as a soil amendment. Sci. Tot. Environ. 512–513, 454–463. doi: 10.1016/j.scitotenv.2015.01.093
Keywords: poultry litter biochar, phosphorus speciation, hydroxyapatite, lability, chemical extraction
Citation: Li W, Feng X, Song W and Guo M (2018) Transformation of Phosphorus in Speciation and Bioavailability During Converting Poultry Litter to Biochar. Front. Sustain. Food Syst. 2:20. doi: 10.3389/fsufs.2018.00020
Received: 14 March 2018; Accepted: 11 May 2018;
Published: 30 May 2018.
Edited by:
Airton Kunz, Embrapa Suínos e Aves, BrazilReviewed by:
Eduardo Moreno Jiménez, Universidad Autonoma de Madrid, SpainCopyright © 2018 Li, Feng, Song and Guo. This is an open-access article distributed under the terms of the Creative Commons Attribution License (CC BY). The use, distribution or reproduction in other forums is permitted, provided the original author(s) and the copyright owner are credited and that the original publication in this journal is cited, in accordance with accepted academic practice. No use, distribution or reproduction is permitted which does not comply with these terms.
*Correspondence: Mingxin Guo, bWd1b0BkZXN1LmVkdQ==
Disclaimer: All claims expressed in this article are solely those of the authors and do not necessarily represent those of their affiliated organizations, or those of the publisher, the editors and the reviewers. Any product that may be evaluated in this article or claim that may be made by its manufacturer is not guaranteed or endorsed by the publisher.
Research integrity at Frontiers
Learn more about the work of our research integrity team to safeguard the quality of each article we publish.