- 1Centro de Edafología y Biología Aplicada del Segura, Consejo Superior de Investigaciones Científicas (CSIC), Murcia, Spain
- 2LEAF, Instituto Superior de Agronomia, Universidade de Lisboa, Lisbon, Portugal
- 3EPS-Orihuela, Miguel Hernandez University, Alicante, Spain
Composts were prepared from the solid fraction of pig slurry (SPS) by mixing with cotton gin waste (CGW) in two different proportions. The aim was to assess the fertilizer value of the two composts and to evaluate potential pollution risks due to the heavy metal (Cu and Zn) concentrations in the composts and the nitrate leaching following their soil application. For this, a pot trial using Italian ryegrass (Lolium multiflorum Lam.) as a test plant was run in a glasshouse. Two composts, A and B, prepared with SPS:CGW ratios of 4:3 and 3:4 (v:v), respectively (OM 63 and 57%, TN 36.1 and 32.7%, and TP 15.3 and 10.8 g kg−1 in A and B, respectively), were applied to a sandy-loam soil at two doses based on the N supplied (D1: 360 mg TN pot−1, equivalent to 52 kg N ha−1, and D2: 720 mg TN pot−1). They were compared with a soluble mineral fertilizer (3.62 g pot−1 of N:P:K 10:10:10; providing 360 mg N pot−1); unfertilized control soil was also tested. The potential risk of nitrate-N leaching in the soil after two simulated intense rainfall events was evaluated. The experiment was carried out in double-layer pots, which allowed the collection of leachates. Both composts were able to increase plant biomass production and soil TN and available P with respect to the control; available Cu and Zn in the soil did not increase significantly with respect to the control soil and mineral fertilizer treatment, although N deficiency limited plant growth at harvests 2 and 3. The compost prepared with a higher proportion of SPS (A) was more efficient for N fertilization (relative agronomic efficiency: 38.1–47.6%; nitrogen use efficiency: 34.1–41.9% of TN), due to its greater inorganic-N concentration. To obtain high P and K efficiencies, application rates based on compost TN are recommended, which also prevent Cu and Zn soil enrichment. Nitrate leaching was very low in all treatments (<0.5% of TN applied). Therefore, both composts can be used as organic fertilizers in the partial substitution of mineral fertilizers, without a significant nitrate leaching risk.
Introduction
The current Circular Economy approach requires the treatment of wastes so that their organic matter (OM) and nutrients can be recovered and used in agriculture. Materials derived from organic residues, such as compost, can be considered as sources of plant nutrients, for partial substitution of synthetic fertilizers (Flotats et al., 2011; Brockmann et al., 2014), in accordance with the key principles of the Circular Economy (Bernal, 2017). Compost application to soil allows the recycling of nutrients in the soil–plant system (Tiquia et al., 2002; Bernal et al., 2009a; Flotats et al., 2011), increasing biomass production and soil fertility by improving the physicochemical and biological properties of the soil (Keener et al., 2000; Loecke et al., 2004; Cordovil et al., 2007; Bedada et al., 2014) as well as nutrient availability (Tejada and Gonzalez, 2006; Biau et al., 2012). Simultaneously, composting provides an efficient and cost-effective way of recycling these materials (Arcadis, EUNOMIA Research & Consulting, 2010; Burgos et al., 2006).
Composting has proved to be a feasible technology to treat the solid fraction of pig slurry (SPS) in areas with a high pig density, where there is excess slurry production with respect to the agricultural land available for its spreading. The stable final product is free of pathogens and rich in humified OM and nutrients (Vanotti et al., 2006; Brockmann et al., 2014; Santos et al., 2016; Sáez et al., 2017). Compared with other organic wastes (e.g., animal slurry, digestates, or untreated plant residues), qualitatively high-class composts show clear benefits as soil amendments/fertilizers: reliable sanitization, a high proportion of stable and humic-like substances, and high concentrations of nutrients (Bernal et al., 2009a, 2017; Moral et al., 2009), the latter allowing a reduction in mineral fertilization (Brockmann et al., 2014).
However, one of the main limitations to compost utilization as organic fertilizer is the fact that the exact amount of N available to plants is not clearly known (Bernal, 2017), which can induce an under- or over-application of N if the compost application rate is based on the total or mineral N content, respectively. Indeed, total N comprises both inorganic forms (mainly nitrate in mature compost; Bernal et al., 2009a), readily available to plants, and organic forms, which need to be mineralized in order to be available to plants (Bernal et al., 1998; Cordovil et al., 2006). So, when compost is applied on a total N basis, the fertilizer efficiency may be lower than that of mineral fertilizers. Furthermore, the rate of organic-N mineralization should be such that available N is produced when required by the crop (Cordovil et al., 2006); otherwise, it can be accumulated in the soil or lost to the environment (He et al., 2000).
Several parameters and criteria have been used for determining the efficiency of the nutrients present in fertilizers (Fixen et al., 2014). Nutrient use efficiency is an important concept in the evaluation of crop production systems and is commonly measured using several indicators (Gunnarsson et al., 2010; Fixen et al., 2014), such as agronomic efficiency and apparent recovery fraction (ARF) or efficiency. Modifications of the most common criteria also exist, to compare the efficiency of organic fertilizers with that of readily available mineral fertilizers. For instance, the relative agronomic efficiency (RAE) compares the agronomic efficiency of an organic fertilizer with that of a mineral fertilizer and also allows the extrapolation of the results to different total nutrient application rates (Brod et al., 2015). Much attention has been paid to N efficiency, due to the complicated N cycles in the soil, water, and atmosphere. The EU Nitrogen Expert Panel (2015) proposed an easy-to-use indicator for “nitrogen use efficiency” (NUE), applicable to agriculture and food production–consumption systems, based on the mass balance principle. The EU Nitrogen Expert Panel (2015) considered a range of NUE values, indicating >100, soil N mining; 90–100, risk of soil N mining; 70–90, balanced fertilization; 50–70, risk of N losses; <50, a high risk of N losses due to inefficient resource use.
Another limitation associated with the use of composted manures, and in particular SPS, is the presence of high concentrations of Cu and Zn (Sáez et al., 2017). Both elements are supplied to piglets in great quantities, to promote growth (Cu), and prevent diseases (Zn). However, almost 90% is excreted in the feces (Legros et al., 2010) and is later concentrated in the SPS and in the derived compost (Sáez et al., 2017). Thus, the systematic application of composted pig manure as organic fertilizer could promote the accumulation of these elements in the soil (Gräber et al., 2005).
The over-application of mineral and organic fertilizers to crops can lead to an excess of nutrients and their consequent loss, initially through leaching, to the surrounding environment (Cavanagh et al., 2011). In Mediterranean climates, the amount of rainfall frequently is not sufficient to wash the nitrate down into aquifers, and it accumulates in the vadose zone (Jorge-Mardomingo et al., 2015). However, heavy storms in short time periods do occur, which can constitute a risk regarding nitrate pollution by leaching. The use of fertilizers in nitrate-vulnerable zones is regulated by EU legislation (Directive 91/676/EEC; EEC, 1991) that limits the application to 170 kg N ha−1 year−1, to minimize the impacts of nitrate pollution. The potential contamination of groundwater by nitrate from N fertilization depends mainly on the stability of the amendment used and therefore on the mineralization rate of the organic N and on the water input into the soil system, upon which the transport of nitrate to aquifers depends (Jorge-Mardomingo et al., 2015). Also, some physical properties of the composts, such as water holding capacity (WHC), can affect the water fluxes in the soil and thus the N losses (Corrêa et al., 2005). In this sense, the use of stabilized composts could reduce the potential nitrate leaching.
We hypothesized that mature compost derived from the SPS could be considered a fertilizing material due to the concentration of inorganic N readily available to plants and the organic-N forms, which will mineralize later in the soil, and that the nutrient efficiency will be similar in composts from the same origin although prepared with different SPS:cotton gin waste (CGW) proportions. Also, we considered that mature compost may have a low risk of nitrate pollution by leaching, relative to mineral fertilizer, and the application of pig slurry compost at a rate adequate for nutrient supply will prevent Cu and Zn toxicity to plants and their soil accumulation.
Therefore, the main objective of this study was to assess the fertilizer value of two composts produced from the SPS and CGW and to evaluate potential pollution risks due to the heavy metal (Cu and Zn) concentrations in the composts and the nitrate leaching following their soil application.
Materials and Methods
Experimental Design
A 4-month pot trial with Italian ryegrass (Lolium multiflorum Lam.) was carried out in a greenhouse. The soil was collected from the 0- to 15-cm soil layer of an agricultural field at UTAD (Universidade de Trás-os-Montes e Alto Douro), Vila Real, Portugal, air-dried, and sieved to 4 mm for the pot experiment and to 2 mm for analysis. The soil is non-calcareous (CaCO3 < 0.5%), with a sandy-loam texture, a WHC of 32%, and very low OM, total nitrogen (TN), and extractable P (Olsen) concentrations (Table 1). Two composts (A and B) were prepared from the SPS and CGW, at proportions of 4:3 and 3:4 (by volume), respectively, by the static composting system (Rutgers) in a pilot plant (Santos et al., 2016; Table 1). The composts were applied to the soil at two doses and compared with a soluble N:P:K (10:10:10) commercial fertilizer (N as NH4NO3).
Six treatments were tested as follows:
– AD1 and BD1: compost A and compost B at dose 1 (D1), using 10 and 11 g pot−1 of composts A and B, respectively — providing 360 mg TN pot−1 (equivalent to 1.44 and 1.59 t ha−1 of composts A and B, respectively or 52 kg N ha−1), 350–272 mg P2O5 pot−1 and 402–439 mg K2O pot−1, respectively;
– AD2 and BD2: compost A and compost B at dose 2 (D2), adding double the amount of D1 (720 mg TN pot−1);
– F: mineral fertilizer (N:P:K 10:10:10) at a rate of 3.62 g pot−1 [360 mg N pot−1 (as in D1 of compost)], equivalent to 52 kg N ha−1 and 360 mg pot−1 of P2O5 and K2O; and
– C: control treatment without any fertilization.
A glasshouse experiment was run in 7-L double-layer polyethylene pots (System-Kick Brauckmann) which allow leachate collection from the bottom of the external part of the pot, through a polyethylene tube. The soil (9 kg of dry soil) was placed in each pot at a density similar to that in the field (1.3 g cm3). For the compost treatments, the composts were mixed with the soil at the beginning of the experiment, and for the F treatment, the mineral fertilizer was divided into two applications (1.8 g pot−1 each time): before sowing and after the first harvest. All treatments were replicated four times (the total number of pots = 24). The moisture content of the soils was adjusted to 70% of the WHC with distilled water at the beginning of the experiment; then, 0.5 g of ryegrass seeds was added per pot (equivalent to 717 g m−2). The soil moisture content was corrected weekly by weighing each pot and adding water to reach 70% of the WHC. Any leachate produced after watering was collected from the bottom of the external part of the pot, quantified, and frozen for further analysis. The pots were placed in mobile racks which allowed frequent changes in position (every 4 days) inside the glasshouse (without temperature control). During the experiment, the plant material was harvested three times—30, 60, and 120 days after emergence—by cutting the aerial parts 2 cm above the soil surface. The fresh and dry weights were determined before and after drying at 60°C, respectively.
The potential nitrate leaching risk in the soil was assessed after the first and second harvests. For this, the moisture content of the soil was first adjusted to 70% of WHC; then, a heavy rain event (equivalent to 25 mm) was simulated by adding 1.75 L of distilled water to the top of the pot (equivalent to 60% of WHC). The leachates produced were quantified and frozen for further analysis.
At the end of the experiment, after the third harvest, the soil was separated from the main roots, homogenized, and sampled for analysis. The roots were cleaned—first by removing most of the soil particles with tap water, then two times with abundant distilled water, and, finally, by ultrasound (5 min at 750 W)—to eliminate any remaining soil particles adhering to the roots. Plant material (aerial part and roots) was dried at 60°C for 48 h and milled for analysis.
Analytical Methods
The moisture content of the plant material was determined by drying the sample at 60°C for 48 h in an oven with forced aeration. Then, chemical analyses were performed on the dry and ground samples: for compost and plants, total organic carbon (TOC) and TN by automatic microanalysis (EuroVector EuroEA 3000 elemental analyzer); after H2O2/HNO3 microwave-assisted digestion, total P by a colorimetric method, K by atomic emission spectrophotometry (iCE 3000 series, Thermo Scientific), and Cu and Zn by atomic absorption spectroscopy (AAS) (iCE 3000 series, Thermo Scientific). The soil samples were analyzed for moisture content, by oven-drying the samples at 105°C for 12 h; WHC, gravimetrically, after allowing water-saturated soil to drain for 24 h; total CaCO3 by the calcimeter method; pH, in a saturated soil paste; electrical conductivity (EC), in a 1:5 water extract; TOC and TN, by automatic microanalysis (Navarro et al., 1993); OM concentration, calculated by multiplying TOC by 1.72 (Nelson and Sommers, 1982); –N, by a colorimetric method based on Berthelot’s reaction (Sommer et al., 1992), after 2 M KC1 extraction (1:20, w/v); –N, with a selective electrode (EPA 9210A) in a 1:20 water extract; available P, by a colorimetric method, after 0.5 M NaHCO3 extraction (1:10, w/v) (Olsen-P; Watanabe and Olsen, 1965); available-K, by EAS, after 1 M CH3COONH4 extraction (1:10, w/v) (Kundsen et al., 1982); water-soluble and exchangeable Cu and Zn, by AAS, after 0.1 M CaCl2 extraction (1:10, w/v) (McGrath and Cegarra, 1992). The leachates were analyzed for water-soluble nitrogen (Nw), using an automatic analyzer for liquid samples (TOC-V CSN Analyzer, Shimadzu), and nitrate, by ionic chromatography (850 Professional IC, Metrohm, Herisau, Switzerland). All the analyses were performed at least in duplicate, with analytical errors of <2%.
Calculations and Statistical Analysis
The plant N-utilization efficiency was calculated on the basis of the ARF, as suggested by Gunnarsson et al. (2010), and was reported as cumulative according to the following equation:
in which N uptake treatment is the total N uptake (mg pot−1) of a fertilizer treatment (AD1, AD2, BD1, BD2, and F) at time n (n = harvest 1, 2, and 3); N uptake control is the total N uptake (mg pot−1) of the unfertilized control at time n (n = harvest 1, 2, and 3); TNadded is the total N added to each pot (mg pot−1).
The RAE of N, P, and K in the compost treatments was calculated from the nutrient uptake in the aboveground biomass of the compost treatment in comparison with the mineral fertilizer, taking into account the input in the double-dose treatments (Brod et al., 2015):
The NUE of the compost and of the mineral fertilizer was calculated by expressing as a percentage the ratio of the Noutput (N uptake by plants) and Ninput (N applied in the treatment) of the soil (EU Nitrogen Expert Panel, 2015).
For statistical analysis, IBM SPSS Statistics 21.Ink software was used. The standard error of the means was calculated for the chemical and physicochemical characteristics of the soils and the plant biomass and nutrients; a one-way analysis of variance (ANOVA) was performed, and differences between means were determined using Tukey’s test. A two-way ANOVA was used to determine significance in the plant parameters according to the harvest and treatment and to the type of compost and application rate. The normality and homogeneity of the variances were checked using the Shapiro–Wilk and Levene tests, respectively, before ANOVA.
Results and Discussion
Biomass Production of L. multiflorum
The shoot biomass production of L. multiflorum (dry weight) increased in the compost treatments and with the compost application rate, in comparison with the control plants, at all harvests, without significant differences between the two composts (Figure 1). The root biomass production was highest in F and only treatment AD2 gave results statistically different from the control (Figure 1). The compost treatments at D1 gave shoot biomass values similar to that of F in the first harvest, with significantly greater values at D2, due to the greater supply of inorganic N (300 and 260 mg pot−1 in composts A and B, respectively) in comparison with D1 (150 and 130 mg pot−1 in composts A and B, respectively) and with the first application of F (180 mg pot−1). The pig manure composts had high inorganic N concentrations (Table 1), supplying adequate N for correct plant growth after soil application. Thus, this readily available N seems to be the main factor responsible for the augmented plant growth (Eghball and Power, 1999), demonstrated by the positive correlation (r = 0.98; p < 0.001) between the aerial biomass production (DM) and the TN supplied by the amendments. The dependence of DM yields of Italian ryegrass on the N supply was suggested by Douglas et al. (2003), who used different organic wastes in a field experiment. Treatment F led to the greatest plant DM production at harvests 2 and 3, as the mineral fertilizer was divided into two applications, at the beginning of the experiment and after the first harvest, providing available N for the subsequent plant growth.
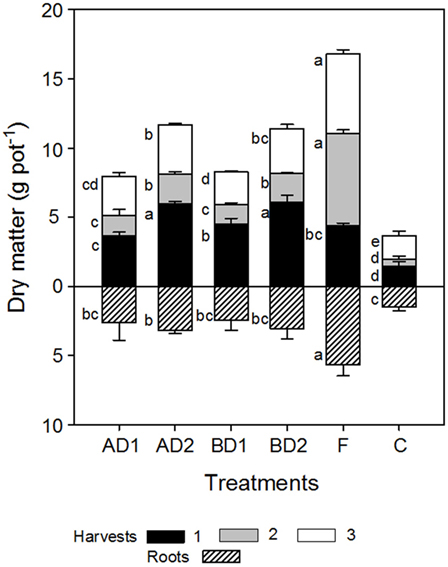
Figure 1. Shoot biomass production of Lolium multiflorum at each harvest and root biomass at the end of the experiment (dry matter) in the different treatments: AD1, compost A at dose 1; AD2, compost A at dose 2; BD1, compost B at dose 1; BD2, compost B at dose 2; F, mineral fertilizer; C, non-fertilized control. For each harvest, values (bars) with the same letter do not differ significantly according to the Tukey test (P < 0.05).
Inorganic N ( and ) in the composts, readily available to plants, was responsible for the effects seen at the first harvest, but organic N needed to be mineralized for plant uptake prior to the following harvests. The decreased yields at harvests 2 and 3 for the compost treatments may indicate scarce mineralization of the organic N in both composts, as observed in previous studies (Bernal et al., 1998; Moral et al., 2009), due to the microbial stability of the OM, with a low amount of degradable TOC (Bernal et al., 1998, 2009a). Indeed, the N mineralization of mature compost is usually slow and may not have been sufficient to provide enough inorganic N to the crop for the following harvests. Cordovil et al. (2006) reported a good relationship between the N supplied to ryegrass by different organic amendments and their potentially mineralizable N. Different studies revealed differences in the mineralizable N in compost; for instance, Moral et al. (2009) reported N-mineralization values ranging from 0 to 34% of TN in composts prepared from manures, but only from 1 to 4.7% of TN in those prepared from pig slurry.
Also, fresh organic wastes and immature or unstable compost can cause N immobilization in the soil after their application, mainly for high C/N ratios, reducing plant-available N and leading to low yields (Bernal et al., 1998; Douglas et al., 2003; García-Gómez et al., 2003). Increases in the biomass production of maize (12–15%) with the use of composted pig manure, in comparison with the fresh manure, were found by Loecke et al. (2004) during a 2-year experiment. Cordovil et al. (2007) also found a greater wheat production with composted pig manure than with pig slurry, and Bedada et al. (2014) observed an increase in crop production of 11%, in comparison with the control treatment, when compost prepared with cattle manure and agricultural wastes was applied. Thus, compost maturity guarantees the N mineralization after soil application, avoiding soil microbial N immobilization.
Nutrient Uptake and Accumulation by L. multiflorum
Compost application significantly increased the concentrations of the macronutrients N and K in the plants, compared with the control, at each harvest, without significant differences relative to treatment F (Table 2). Only treatment BD1, at the first harvest, reduced the N concentration in the plants with respect to F, due to the low inorganic-N concentration in compost B (Table 1). All compost treatments led to greater P concentrations in the plants than did treatment F at harvests 2 and 3, even at D1. This was probably due to a concentration effect associated with the low yields obtained at those harvests for the compost treatments (Ylivainio et al., 2008). So, both composts were able to substitute only partially for mineral fertilizer.
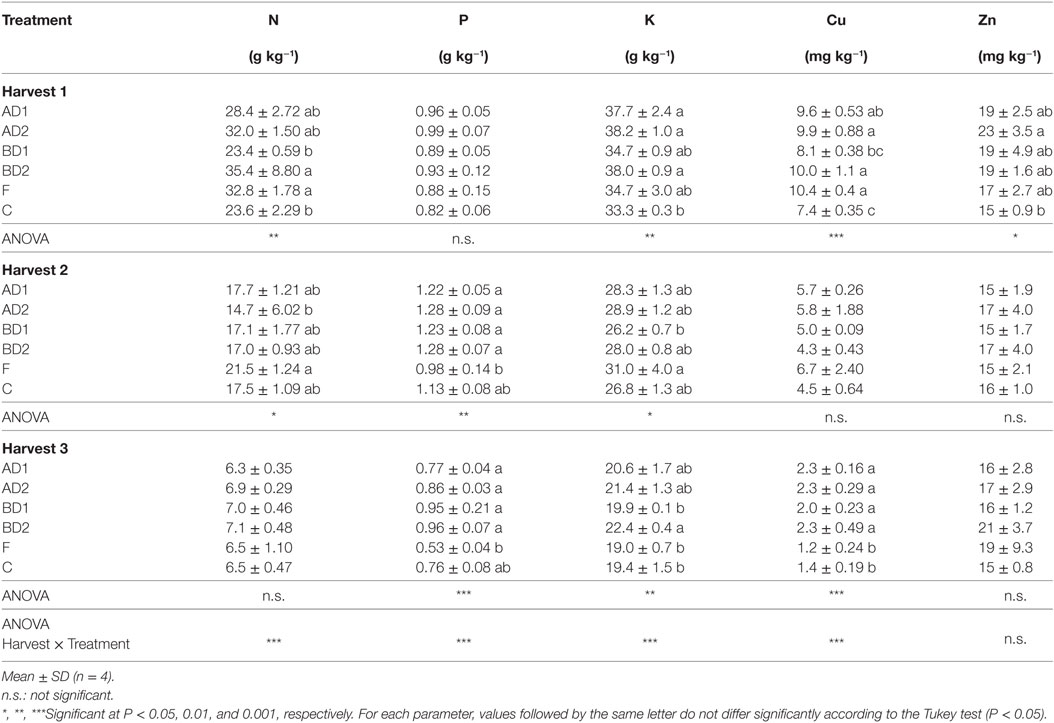
Table 2. Plant nutrient concentrations in the aerial parts of L. multiflorum at the three harvests, according to the different treatments applied to the soil: AD1, compost A at dose 1; AD2, compost A at dose 2; BD1, compost B at dose 1; BD2, compost B at dose 2; F, mineral fertilizer; C, non-fertilized control.
Besides the significant, positive responses of the N, P, and K concentrations in ryegrass to compost application, a significant decline in N with the harvesting cycle was observed, which may be due to the consumption of the easily available N and scarce N mineralization discussed previously, or to nutrient leaching after each “rain event” (discussed below). Despite the slightly higher N concentration in plants from treatment D2, relative to D1, in the first harvest, the values for the compost-based treatments did not differ significantly (P < 0.001), and only TN in BD2 was higher than the control values. Considering the optimal shoot N, P, and K concentrations in Italian ryegrass (30–42 g N kg−1 DM, 3.5–5 g P kg−1 DM, and 25–35 g K kg−1 DM; Bergmann, 1986), N and P might have limited the growth, particularly at harvests 2 and 3. The P concentration in the aboveground biomass was within the lower range found by Ylivainio et al. (2008), who suggested that a low P concentration can be due to a dilution effect caused by the enhanced growth of L. multiflorum—as shown at harvest 2, when the P concentration was lowest for plants of treatment F, which also gave the highest yield. The N:P ratio in the aerial parts of the plants can indicate the nutrient that is restricting plant growth (Güsewell, 2004). The N:P ratio of >20 found at the first harvest, for all treatments, suggests that plant growth was initially restricted by P availability, while, at harvest 3, the N:P ratio of <10 indicates that N limited plant growth at the end of the experiment for the compost treatments (Güsewell, 2004). At the second harvest, for all treatments, the N:P ratio was adequate. These results indicate that the plant-available N from compost and F was adequate immediately after soil addition, but the precipitation or fixation of P can occur shortly after addition to the soil (e.g., due to insoluble Ca-phosphate formation), being released later on during the growing session. Organic acids in the soil, produced from root exudates, may compete for the same binding sites as P, depressing the P sorption strength of the soil (Ylivainio et al., 2008). The exudation of organic anions by the roots has been found to occur in the rhizosphere, associated with –N nutrition in preference to –N (Marschner, 1995; Hinsinger et al., 2003); also, localized release of H+ by some portions of the roots has been reported to occur as a response to P deficiency, favoring P solubility in the soil (Hinsinger et al., 2003). Jones (1998) reported two mechanisms of P release in the soil rhizosphere: direct ligand exchange, whereby citrate directly replaces P on ligand exchange surfaces, and complexation with metal ions in the solid phase, which constitutes the exchange matrix holding P (such as Ca2+ in rock phosphate). According to Jorgensen et al. (2010), composting of the separated solids from animal slurry does not alter the P availability but P precipitation as Ca or Mg phosphate may occur during composting due to the pH increase. Ylivainio et al. (2008) indicated that the high proportion of inorganic P in manure compost makes it a convenient P fertilizer for L. multiflorum due to its high root-to-shoot ratio of P (as shown in the present experiment; Tables 2 and 3); these authors referred to it as a P-efficient species with relatively low external P requirements.
The nutrient uptake by the aerial parts of L. multiflorum during the pot trial is shown in Figure 2. Compost application, particularly at the high rate (D2), significantly increased the N, P, and K accumulation by the shoots, when compared with the control (Figure 2). The uptake of N, P, and K was greatest in treatment F (324, 13.5, and 467 mg pot−1, respectively), with a considerable difference from BD2 (270, 11.4, and 362 mg pot−1, respectively). Composts A and B did not show any significant differences at the same application rate, but nutrient uptake increased with the compost application rate. An increase in N uptake with the compost application rate was also found by Cordovil et al. (2006), but differences were not found when organic and inorganic fertilizations were combined, suggesting that the mineral N supplied could have stimulated not only N uptake but also that of other nutrients. Also, Ylivainio et al. (2008) reported a higher P uptake by L. multiflorum when composted manure was applied at a double rate and even greater uptake at a quadruple dose, at all harvests.
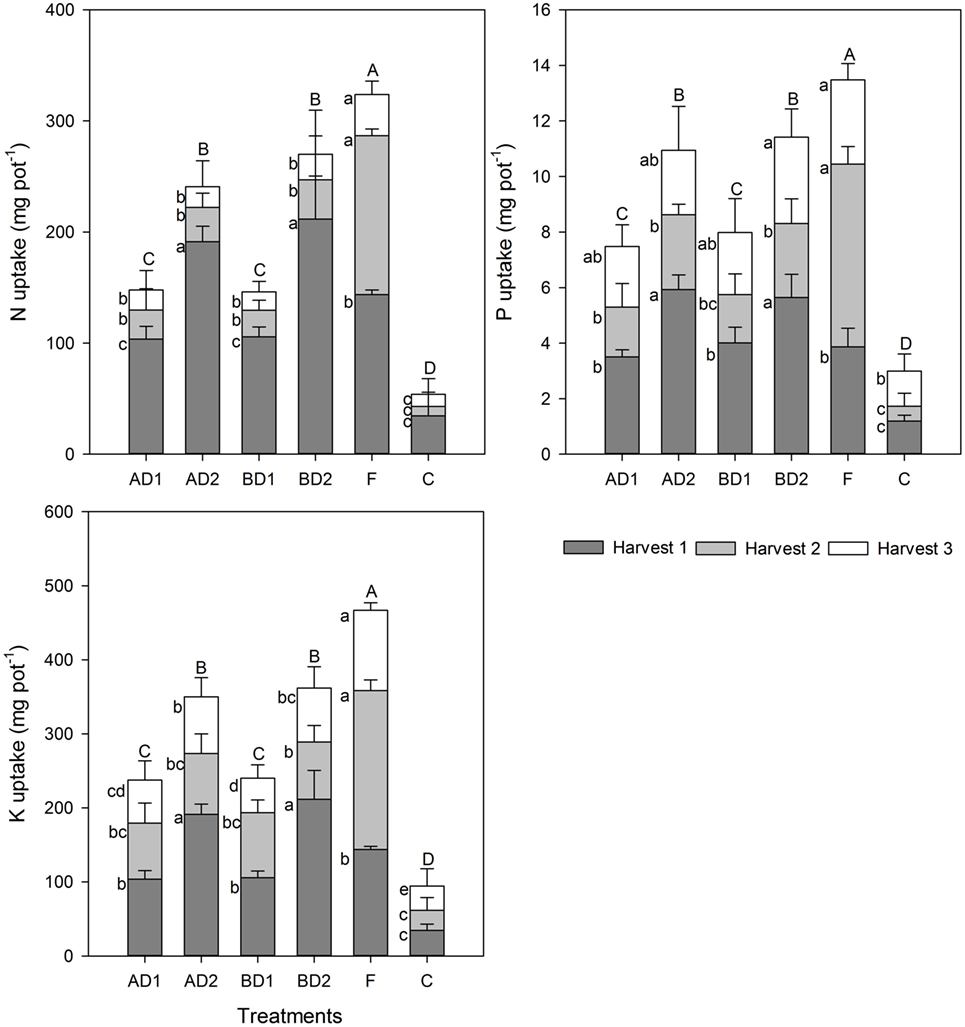
Figure 2. Nutrient uptake in the aerial parts of Lolium multiflorum at each harvest during the pot trial, according to the different treatments applied to the soil: AD1, compost A at dose 1; AD2, compost A at dose 2; BD1, compost B at dose 1; BD2, compost B at dose 2; F, mineral fertilizer; C, non-fertilized control. For each harvest, values (bars) with the same letter do not differ significantly according to the Tukey test (P < 0.05). Capital letters refer to the accumulated values (sum of harvests 1 + 2 + 3) and lower-case letters refer to each harvest.
High concentrations of Cu and Zn are usually found in pig slurry compost (Sáez et al., 2017). The Spanish legislation for fertilizing products (Ministerio de la Presidencia, 2013) classifies composts according to the total Cu and Zn concentrations. The composts in the present experiment belonged to class C, with Cu between 300 and 400 mg kg−1 and Zn between 500 and 1000 mg kg−1, limiting the use to 5 t ha−1 year−1 on agricultural soil. The concentrations of Zn and Cu in the plants were analyzed in order to determine their accumulation due to the use of pig slurry composts. At the first harvest, the shoot Cu and Zn concentrations were generally higher in the compost treatments than in control plants, but without statistically significant differences from treatment F. The concentrations of Zn measured in the aerial parts of the plants can be considered normal for plant nutrition (27–150 mg kg−1; Kabata-Pendias, 2001) and are within the range found for grasses (12–80 mg kg−1; Kabata-Pendias, 2001). Although the shoot Cu concentration remained higher in the compost treatments than in the control, the values are within the normal range for plants (5–30 mg kg−1; Kabata-Pendias, 2001) and the range for grasses (1.3–33.1 mg kg−1; Kabata-Pendias, 2001). The Cu concentration was decreased at the following harvests; this may be due to the ability of Cu to form chelates with the stable and humified OM (Bernal et al., 2009b). Therefore, both composts used here, A and B, did not present any risk regarding metal toxicity to plants.
Fertilizing Efficiency
The nutrient fertilizing efficiency of the compost was estimated as the ARF, calculated according to Eq. 1. With respect to the control, the N-ARF of the composts ranged from 24 to 29%, without significant differences between the compost types or doses (Table 4). Similar values (28%) were found by Thomsen and Kjellerup (1997) for manure without litter (cattle feces), being 8–14% for manure with litter (cattle feces + urine + straw), related to the inorganic-N concentration; lower values were found in winter, associated with N leaching. The mineral fertilizer treatment (F) reached the greatest N-ARF value, because all the N was in inorganic forms (NH4NO3), whereas in composts A and B, 26.7 and 19.0%, respectively, of the TN was in inorganic forms (Table 1). This indicates that the inorganic N present in compost A was assimilated by the plants, but partial mineralization of the organic N of compost B may have occurred, rendering it available to plants. Cordovil et al. (2006) compared the potentially mineralizable N of several organic materials (determined in incubation experiments) with the plant N uptake in a pot experiment. Their results showed that the plant N uptake from pig manure compost exceeded the potentially mineralizable N (33.3 and 25%, respectively), similar to the results found here, while compost from municipal solid waste led to very similar values of N uptake and potentially mineralizable N (19%). Also, Douglas et al. (2003) found a very low N-ARF of green compost, in spite of its high TN concentration (4.3–5.8 g kg−1).
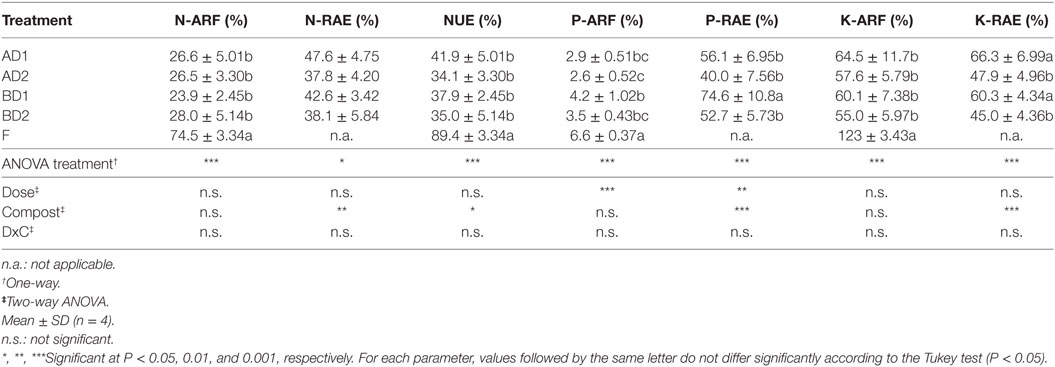
Table 4. Evaluation of the fertilizer efficiency by the Apparent Recovery Fraction (ARF) and Relative Agronomic Efficiency (RAE) for N, P, and K, and the Nitrogen Use Efficiency (NUE).
Compared with the efficiency of the mineral fertilizer, the values of RAE (Table 4) confirm that the mineralization of organic N in the mature composts occurred in the soil during the crop development, accounting for 10–20% of the TN in compost A and an average of 22% in compost B. According to Bernal et al. (1998), the potentially mineralizable N increases with composting time, representing about 9% of the TN in mature compost derived from sewage sludge. Although the NUE is considered applicable to agriculture and food production–consumption systems, this parameter also can be applied to small agronomic experiments (EU Nitrogen Expert Panel, 2015). Considering the inputs and outputs in the present system (pots), the maximum NUE occurred with F, its value decreasing with the compost application rate (Table 4). The NUE values may also indicate that N mineralization occurred in the system, representing 8–18% of TN, with greater values for compost B.
The ARF of P was low in all treatments, the highest value being in treatment F (6.6%; Table 4). This implies that this nutrient was applied in excess of crop requirements and accumulated in the soil. In the work of Ylivainio et al. (2008), the ARF of P for ryegrass in the first growing year was 20–26% for manure compost applied at different rates, with similar values in the treatments with mineral fertilizer (21.7–26.8%). Grigatti et al. (2017) found, in a 21-week pot experiment, P-ARF values for green waste compost of 10–40%, depending on the characteristics (P fractionation) of the compost. They used a P-application rate (15 mg kg−1) similar to that in the present experiment; the ARF was related to the H2O + NaHCO3−extractable P, which was mainly inorganic P. The solubility of the P from compost may depend on the degree of maturation. During composting of animal manures, Eneji et al. (2003) found that water-soluble P decreased during composting, shifting toward the more resistant HCl-soluble P (associated with Ca and Mg), which was the dominant phase at the end of composting. The high degree of maturation of the compost used in the present experiment (Santos et al., 2016) may have reduced the availability of P. The RAE of P in compost B (74.6 and 52.7%, respectively, for doses 1 and 2) was greater than in compost A (56.1 and 40.0%, respectively, in doses 1 and 2), due to the lower concentration of P in compost B, giving a greater efficiency of P uptake by the plants. Brod et al. (2015) determined the P-RAE of different organic waste materials for ryegrass: the greatest values occurred for dairy cattle manure, with a greater efficiency than the mineral fertilizer (Ca(H2PO4)2), followed by liquid digestate and chicken manure (72–78%), with lower values for fish sludge and solid digestate (47–70%), for one or up to four harvests in a soil of pH 6.9. These values were well predicted using the NaHCO3−extractable P (Grigatti et al., 2017).
A very different behavior was shown by K, with 100% of the K added to the soil in F assimilated by the plants while the ARF reached very high values for composts A and B, without differences according to the compost type and dose (Table 4). This is due to the highly available (mainly soluble) forms of K present in the composts; the fraction not taken up by plants can be retained in the soil as exchangeable and soluble forms, but can also be lost by leaching. The high EC of the leachates of the compost treatments (up to 630 μS cm−1 in the first rain event and up to 263 μS cm−1 in the second), with respect to the control (116 and 127 μS cm−1, rain event 1 and 2, respectively) and treatment F (219 and 170 μS cm−1, rain event 1 and 2, respectively), may indicate the leaching of soluble salts, including K.
Nitrogen Leaching and Residual Soil Nutrients Availability
Losses of N during the experiment could have occurred by leaching or denitrification (Hoffman and Van Cleemput, 2004; Burgos et al., 2006; Demuras et al., 2016). For leaching to occur, N must be in a water-soluble, mobile form, and the rainfall must be abundant enough to transport N through the soil. Nitrate is the N form most susceptible to leaching, since ammonium N can be retained in the soil cation exchange capacity. In this sense, water-soluble TN (Nw) present in the leachate from the first rain event occurred as nitrate (data not shown); then, only Nw was measured in the following leachates.
Considering the threshold of 50 mg l−1 for concentration in ground and surface water set by the European Legislation (European Union, 1998), all the leachate samples were far away from that value (maximum concentration 5.28 mg l−1). All the fertilizer treatments had higher N losses than the control during the experiment (1.7- to 2.1-times greater), without significant differences between treatments or rain events (Figure 3). The amount of N leached during the rain events did not differ statistically among the fertilizer treatments, and similar values also occurred during the irrigation in the soils treated with compost B (Figure 3). For TN, the total proportion lost (during irrigation and rain events) was lowest when each compost was applied at a high rate (Figure 3). The WHC of the composts may have had a strong influence, reducing N leaching (Fulekar, 2010), as compost can improve soil properties to achieve more efficient water utilization. In fact, compost B, prepared with a greater amount of CGW than compost A, produced a slightly lower volume of leachate during the rain events than compost A, especially at D2 (2,100 ± 395 and 1,695 ± 380 ml pot−1 in AD2 and BD2, respectively; 2,180 ± 212 ml pot−1 in F). In terms of the doses applied, no significant differences were found when comparing the amounts of N leached (Figure 3). A higher –N application might imply a greater N leaching risk, due to the excess of with respect to the plant requirements. However, the lack of a significant change in the loss of N by leaching (or accumulation in the soil, Table 5) indicates that the –N provided by the compost was used by the plants (as the ARF, RAE, and NUE also indicate). Burgos et al. (2006) pointed out that N losses by leaching tend to be higher when poorly stabilized organic materials rich in N are applied, but also stated that losses by leaching from compost-amended soils are usually very low. Demuras et al. (2016) concluded from a field experiment that the replacement of organic with mineral N-fertilization systems was not effective at mitigating leaching, although the residual N remained in the soil for further mineralization. In fact, they did not find any reduction in N leaching during winter, when mineral fertilizer was used instead of organic N.
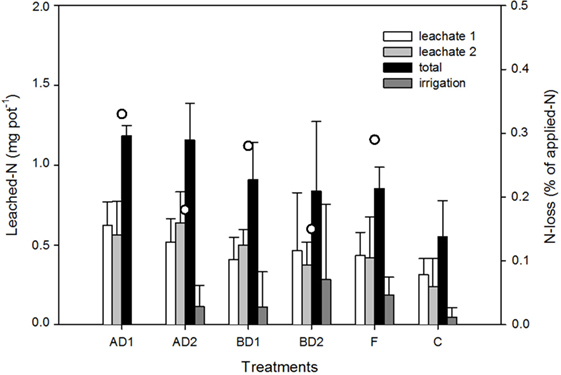
Figure 3. Nitrogen leached during each rain event simulated during the pot trial. No significant differences were found among the treatments. Dots indicate total N leached in each treatment as a percentage of TN added.
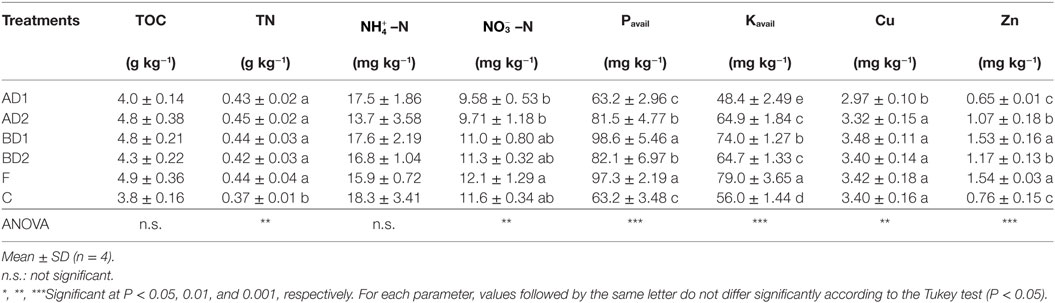
Table 5. Total organic carbon (TOC), total N (TN), inorganic-N forms, available P and K, and the soluble and exchangeable fraction of Cu and Zn (CaCl2-extractable) of the soils at the end of the experiment.
The chemical properties of the soils at the end of the experiment are shown in Table 5. The TOC in the soil showed small and non-significant differences between the treatments with compost and the control. Evanylo et al. (2008) found small differences in the TOC in soils amended with compost at low doses, indicating that a relevant proportion of the OM from the compost may have been mineralized during the experiment. All the treatments were able to increase TN in the soil, at the end of the experiment, with respect to the control soil, without differences among the fertilizing treatments. Increases in TN in the soil after application of composts produced from livestock manure or from municipal solid wastes have been found in pot studies (Soumaré et al., 2003; Evanylo et al., 2008; Karami et al., 2011)—indicating that the resistant fraction of the organic N in mature composts accumulated in the soil, being incorporated into the humified OM.
The lack of significant differences in the soil –N concentration among the treatments indicates its complete nitrification, in similar proportions in all treatments. The lowest concentrations of –N were observed in the soils amended with compost A, in spite of its high –N concentration (Table 1), but the RAE and NUE results indicate lower N mineralization than in compost B. No statistically significant differences occurred for the rest of the treatments, suggesting that the inorganic N supplied by the amendments may have been used by the plants or lost from the soil. The low N losses by leaching during the two rain events (Figure 3) indicate that the N applied in excess accumulated in the soil, as losses by denitrification may have been irrelevant at a soil moisture content of <70% WHC. According to Estavillo et al. (1996), N losses by denitrification from mineral fertilizers can be 9–17% during spring/autumn, reaching 2% from mature composts (Cambardella et al., 2003). In fact, the TN accumulation in the soil with the compost and mineral fertilizer treatments occurred as organic N (Table 5).
The concentrations of available P and K were lowest for treatment AD1 and greatest in treatment F (Table 5), which may be due to the excess P supplied by the treatments, giving a low ARF, as discussed previously, and the highly soluble nature of the K supplied by all treatments. However, a greater proportion of the excess P provided by the mineral fertilizer may have remained in the soil in the available fraction, in comparison with that provided by the compost, because P in well-matured compost can be found in less available forms (Eneji et al., 2003; Grigatti et al., 2017).
The elements Cu and Zn are essential plant micronutrients, but their accumulation in the soil can cause toxicity to plants (Kabata-Pendias, 2001). The use of compost from pig manure can lead to soil accumulation, this being one of the main environmental risks associated with compost use. In fact, limits for the Cu and Zn concentrations in compost for agricultural use have been set in several countries and in the EU for soil protection (Bernal et al., 2017). In the present experiment, the composts did not significantly increase the available Cu and Zn concentrations in the soil, with respect to the control (Table 5), and similar values were obtained for Zn in treatment F. These results, together with the plant concentrations found, indicate that these elements in the compost had a beneficial effect, as plant micronutrients. Considering the total concentrations of Cu and Zn in the compost and the application rate, the increases in their total levels in the soil were low (Cu < 0.4 and Zn < 1.0 mg kg−1 soil), with a scarce risk of soil accumulation at the compost rates applied. However, their pollution risk due to accumulation resulting from repeated applications over a long period needs to be tested in field experiments.
Conclusion
Both composts prepared from the SPS can be considered organic fertilizer products, since they efficiently increased the biomass yield of L. multiflorum, providing nutrients for plant growth; N was provided in readily available forms (inorganic N) and was slowly released by partial mineralization of the organic N during the crop growth. Differences in the timing of the supply of N and P to plants could have been responsible for plant growth restriction due to limited P or N supply in the early or later stages of the growing period, respectively, suggesting that the combined application of compost with mineral fertilizer during the growing season may ensure adequate plant growth prior to the subsequent harvests.
The RAE (47.6%) of N was highest for compost A (prepared with the greatest SPS:CGW ratio), due to the concentration of inorganic N directly available to plants. According to the average RAE values, 41% of the N from pig slurry compost can partially replace N from mineral fertilizer. The fertilizer efficiency of P was low for all treatments, but the P-RAE percentages reached 56 and 75% in composts A and B, respectively, at a low application rate.
Taking into consideration the limitation of the present experiment run in pots and under controlled conditions, the microbial stability of mature compost and an adequate C/N ratio for soil application seem to control the N supply to crops as a slow release fertilizer. Under these experimental conditions, the use of both composts as soil fertilizers did not show any threat in terms of nitrate leaching, even after a heavy rain event and with a double dose of compost. As a consequence, it can be concluded that the fertilizing value of both composts can partially substitute for mineral fertilizer.
An experiment under real field conditions may be needed to confirm the present results. To supply the nutrients required for efficient crop production at each particular stage of the growing session, the combination of mineral and organic fertilizers, using composts as a base fertilization before sowing and complementing with mineral fertilizer during the growing season, might be worth testing under real conditions. Thus, the results obtained here for NUE, ARF, and RAE can be used to calculate the compost application rate and mineral fertilizer requirement under a combined fertilization plan, with a partial mineral fertilizer substitution by compost to avoid over-fertilization.
Author Contributions
The author AS has performed the experimental work, statistical analysis, and written the first version of the manuscript; MB has designed the experiment and supervised the experimental work, corrected the paper, and written the final version; DF supervised the experimental work of AS and revised the paper; and RM revised the final version of the paper.
Conflict of Interest Statement
The authors declare that the research was conducted in the absence of any commercial or financial relationships that could be construed as a potential conflict of interest.
Acknowledgments
The research was funded by the People Program (Marie Curie Actions) of the European Union’s Seventh Framework Program FP7/2007–2013/under REA grant agreement no. 289887, and the results and conclusions achieved reflect only the author’s view, the European Union not being liable for any use that may be made of the information contained therein. The authors thank Dr. D. J. Walker for the English revision of the manuscript.
References
Arcadis, EUNOMIA Research & Consulting. (2010). Assessment of the Options to Improve the Management of Bio-Waste in the European Union. Study Contract NR 07.0307/2008/517621/ETU/G4. European Commission DG Environment. Available at: ec.europa.eu/environment/waste/compost/pdf/ia_biowaste%20-%20final%20report.pdf (Accessed: January 5, 2018).
Bedada, W., Karltun, E., Lemenih, M., and Tolera, M. (2014). Long-term addition of compost and NP fertilizer increases crop yield and improves soil quality in experiments on smallholder farms. Agric. Ecosyst. Environ. 195, 193–201. doi: 10.1016/j.agee.2014.06.017
Bergmann, W. (1986). Bemerkungen und Tabellen zur analytischen Pflanzendiagnose der Pflanzenoder Blattanalyse. Farbatlas Ermährngsstörungen bei Kulturpflanzen und Analytische Diagnose. Jena: VEG Gustav-Fischer-Verlag.
Bernal, M. P. (2017). Grand challenges in waste management in agroecosystems. Front. Sustain. Food Syst. 1:1. doi:10.3389/fsufs.2017.00001
Bernal, M. P., Alburquerque, J. A., and Moral, R. (2009a). Composting of animal manures and chemical criteria for compost maturity assessment. A review. Bioresour. Technol. 100, 5444–5453. doi:10.1016/j.biortech.2008.11.027
Bernal, M. P., Clemente, R., and Walker, D. J. (2009b). “Interactions of heavy metals with soil organic matter in relation to phytoremediation,” in Phytoremediation: The Green Salvation of the World, ed. J. P. Navarro-Aviñó (Kerala, India: Research Signpost), 109–129.
Bernal, M. P., Navarro, A. F., Sánchez-Monedero, M. A., Roig, A., and Cegarra, J. (1998). Influence of sewage sludge compost stability and maturity on carbon and nitrogen mineralization in soil. Soil Biol. Biochem. 30, 305–313. doi:10.1016/S0038-0717(97)00129-6
Bernal, M. P., Sommer, S. G., Chadwick, D., Qing, C., Guoxue, L., and Michel, F. C. Jr. (2017). Current approaches and future trends in compost quality criteria for agronomic, environmental, and human health benefits. Adv. Agron. 144, 143–233. doi:10.1016/bs.agron.2017.03.002
Biau, A., Santiveri, F., Mijangos, I., and Lloveras, J. (2012). The impact of organic and inorganic fertilizers on soil quality parameters and productivity of irrigated maize crops in semiarid regions. Eur. J. Soil Biol. 53, 56–61. doi:10.1016/j.ejsobi.2012.08.008
Brockmann, D., Hanhoun, M., Négri, O., and Hélias, A. (2014). Environmental assessment of nutrient recycling from biological pig slurry treatment—impact of fertilizer substitution and field emissions. Bioresour. Technol. 163, 270–279. doi:10.1016/j.biortech.2014.04.032
Brod, E., Øgaard, A. F., Haraldsen, T. K., and Krogstad, T. (2015). Waste products as alternative phosphorus fertilizers part II: predicting P fertilisation effects by chemical extraction. Nutr. Cycl. Agroecosyst. 103, 187–199. doi:10.1007/s10705-015-9731-4
Burgos, P., Madejón, E., and Cabrera, F. (2006). Nitrogen mineralization and nitrate leaching of a sandy soil amended with different organic wastes. Waste Manag. Res. 24, 175–182. doi:10.1177/0734242X06062876
Cambardella, C. A., Richard, T. L., and Russell, A. (2003). Compost mineralization in soil as function of composting process conditions. Eur. J. Soil Biol. 39, 117–127. doi:10.1016/S1164-5563(03)00027-X
Cavanagh, A., Gasser, M. O., and Labrecque, M. (2011). Pig slurry as fertilizer on willow plantation. Biomass Bioenerg. 35, 4165–4173. doi:10.1016/j.biombioe.2011.06.037
Cordovil, C. M. d. S., Cabral, F., and Coutinho, J. (2007). Potential mineralization of nitrogen from organic wastes to ryegrass and wheat crops. Bioresour. Technol. 98, 3265–3268. doi:10.1016/j.biortech.2006.07.014
Cordovil, C. M. d. S., Cabral, F., Coutinho, J., and Goss, M. (2006). Nitrogen uptake by ryegrass from organic wastes applied to a sandy loam soil. Soil Use Manag. 22, 320–322. doi:10.1111/j.1475-2743.2006.00031.x
Corrêa, R. S., White, R. E., and Weatherley, A. J. (2005). Modelling the risk of nitrate leaching from two soils amended with five different biosolids. Rev. Bras. Ciênc. Solo 29, 619–626. doi:10.1590/S0100-06832005000400014
Demuras, C. E., Seddaiu, G., Ledda, L., Cappai, C., Doro, L., Carletti, A., et al. (2016). Replacing organic N with mineral N fertilization does not reduce nitrate leaching in double crop forage systems under Mediterranean conditions. Agric. Ecosyst. Environ. 219, 83–92. doi:10.1016/j.agee.2015.12.010
Douglas, J. T., Aitken, M. N., and Smith, C. A. (2003). Effects of five non-agricultural organic wastes on soil composition, and on the yield and nitrogen recovery of Italian ryegrass. Soil Use Manag. 19, 135–138. doi:10.1079/SUM2003180
EEC. (1991). Council directive of 12 December 1991 concerning the protection of waters against pollution caused by nitrates from agricultural sources (91/676/EEC). Off. J. Eur. Commun. L375, 1–8.
Eghball, B., and Power, J. F. (1999). Phosphorus and nitrogen-based manure and compost applications: corn production and soil phosphorus. Soil Sci. Am. J. 63, 895–901. doi:10.2136/sssaj1999.634895x
Eneji, A. E., Honna, T., Yamamoto, S., Masuda, T., Endo, T., and Irshad, M. (2003). Changes in humic substances and phosphorus fractions during composting. Commun. Soil Sci. Plant Anal. 34, 2303–2314. doi:10.1081/CSS-120024065
Estavillo, J. M., Rodriguez, M., and Gonzalez-Murua, C. (1996). Nitrogen losses by denitrification and leaching in grassland: the effect of cow slurry application. Fert. Res. 43, 197–201. doi:10.1007/BF00747702
EU Nitrogen Expert Panel. (2015). Nitrogen Use Efficiency (NUE) – An Indicator for the Utilization of Nitrogen in Agriculture and Food Systems. Wageningen, Netherlands: Wageningen University.
European Union. (1998). Council directive 98/83/CE of 3 November 1998 on the quality of water intended for human consumption. Off. J. Eur. Commun. L330, 32–54.
Evanylo, G., Sherony, C., Spargo, J., Starner, D., Brosius, M., and Hearing, K. (2008). Soil and water environmental effects of fertilizer-, manure-, and compost-based fertility in an organic vegetable cropping system. Agric. Ecosyst. Environ. 127, 50–58. doi:10.1016/j.agee.2008.02.014
Fixen, P., Brentrup, F., Bruulsema, T., Garcia, F., Norton, R., and Zingore, S. (2014). “Nutrient/fertilizer use efficiency; measurement, current situation and trends,” in Managing Water and Fertilizer for Sustainable Agricultural Intensification, First Edn, Chap. 1, eds P. Drechsel, P. Heffer, H. Magen, R. Mikkelsen, and D. Wichelns (Paris, France: IFA, IWMI, IPNI and IPI). 1–30
Flotats, X., Foged, H. L., Bonmati Blasi, A., Palatsi, J., Magri, A., and Schelde, K. M. (2011). “Manure processing technologies,” Technical Report No. II Concerning “Manure Processing Activities in Europe” (European Commission, Directorate-General Environment). Available at: agro-technology-atlas.eu/docs/21010_technical_report_II_manure_processing_technologies.pdf. Accessed January 1, 2018.
Fulekar, M. H. (2010). Environmental Biotechnology: Chemical Benefits of Compost. Boca Raton, Florida: CRC Press LLC.
García-Gómez, A., Bernal, M. P., and Roig, A. (2003). Carbon mineralisation and plant growth in soil amended with compost samples at different degrees of maturity. Waste Manag. Res. 21, 161–171. doi:10.1177/0734242X0302100210
Gräber, I., Hansen, J. F., Olesen, S. E., Petersen, J., Østergaard, H. S., and Krogh, L. (2005). Accumulation of copper and zinc in Danish agricultural soils in intensive pig production areas. Danish J. Geogr. 105, 15–22. doi:10.1080/00167223.2005.10649536
Grigatti, M., Boanini, E., Mancarella, S., Simoni, A., Centemero, M., and Veeken, A. H. M. (2017). Phosphorous extractability and ryegrass availability from bio-waste composts in a calcareous soils. Chemosphere 174, 722–731. doi:10.1016/j.chemosphere.2017.02.039
Gunnarsson, A., Bengtsson, F., and Caspersen, S. (2010). Use efficiency of nitrogen from biodigested plant material by ryegrass. J. Plant Nutr. Soil Sci. 173, 113–119. doi:10.1002/jpln.200800250
Güsewell, S. (2004). N:P ratios in terrestrial plants: variation and functional significance. New Phytol. 164, 243–266. doi:10.1111/j.1469-8137.2004.01192.x
He, Z. L., Alva, A. K., Yan, P., Li, Y. C., Calvert, D. V., Stoffella, P. J., et al. (2000). Nitrogen mineralization and transformation from composts and biosolids during field incubation in a sandy soil. Soil Sci. 165, 161–169. doi:10.1097/00010694-200002000-00007
Hinsinger, P., Plassard, C., Tang, C., and Jaillard, B. (2003). Origins of root-mediated pH changes in the rhizosphere and their responses to environmental constraints: a review. Plant Soil 248, 43–59. doi:10.1023/A:1022371130939
Hoffman, G., and Van Cleemput, O. (2004). Soil and Plant Nitrogen. Paris, France: International Fertilizer Industry Association (IFA), 48.
Jones, D. L. (1998). Organic acids in the rhizosphere—a critical review. Plant Soil 205, 25–44. doi:10.1023/A:1004356007312
Jorge-Mardomingo, I., Jiménez-Hernández, M. E., Moreno, L., de la Losa, A., de la Cruz, M. T., and Casermeiro, M. A. (2015). Application of high doses of organic amendments in Mediterranean agricultural soil: an approach for assessing the risk of groundwater contamination. Catena 131, 74–83. doi:10.1016/j.catena.2015.03.013
Jorgensen, K., Magid, J., Luxhoi, J., and Jensen, L. S. (2010). Phosphorus distribution in untreated and composted solid fractions from pig slurry separation. J. Environ. Qual. 39, 393–401. doi:10.2134/jeq2009.0168
Karami, N., Clemente, R., Moreno-Jiménez, E., Lepp, N. W., and Beesley, L. (2011). Efficiency of green waste compost and biochar soil amendments for reducing lead and copper mobility and uptake to ryegrass. J. Hazard. Mater. 191, 41–48. doi:10.1016/j.jhazmat.2011.04.025
Keener, H. M., Dick, W. A., and Hoitink, H. A. J. (2000). “Composting and beneficial utilization of composted by-product materials,” in Land Application of Agricultural, Industrial, and Municipal By-Products, ed. W. A. Dick (Wisconsin: SSSA Inc), 315–341.
Kundsen, D., Peterson, G. A., and Pratt, P. F. (1982). “Lithium, sodium and potassium,” in Methods of Soil Analysis Agronomy Monograph 9, 2nd Edn, Part 2, eds A. L. Page, R. H. Miller, and D. H. Keeney (Madison, WI: ASA and SSSA), 225–246.
Legros, S., Chaurand, P., Rose, J., Masion, A., Briois, V., Ferrasse, J. H., et al. (2010). Investigation of copper speciation in pig slurry by a multitechnique approach. Environ. Sci. Technol. 44, 6926–6932. doi:10.1021/es101651w
Loecke, T. D., Liebman, M., Cambardella, C. A., and Richard, T. L. (2004). Corn growth responses to composted and fresh solid swine manures. Crop Sci. 44, 177–184. doi:10.2135/cropsci2004.1770b
McGrath, S. P., and Cegarra, J. (1992). Chemical extractability of heavy metals during and after long-term applications of sewage sludge to soil. J. Soil Sci. 43, 313–321. doi:10.1111/j.1365-2389.1992.tb00139.x
Ministerio de la Presidencia. (2013). Real Decreto 506/2013, de 28 de junio, sobre productos fertilizantes. Boletín Oficial del Estado 164, 51119–51207.
Moral, R., Paredes, C., Bustamante, M. A., Marhuenda-Egea, F., and Bernal, M. P. (2009). Utilisation of manure composts by high-value crops: safety and environmental challenges. Bioresour. Technol. 100, 5454–5460. doi:10.1016/j.biortech.2008.12.007
Navarro, A. F., Roig, A., Cegarra, J., and Bernal, M. P. (1993). Relationship between total organic carbon and oxidizable carbon in calcareous soils. Commun. Soil Sci. Plant Anal. 24, 2203–2212. doi:10.1080/00103629309368949
Nelson, D. W., and Sommers, L. E. (1982). “Total carbon, organic carbon and organic matter,” in Methods of Soil Analysis. Part 2. Chemical and Microbiological Properties, ed. A. L. Page (Madison, WI: Am. Soc. Agron. Soil Sci. Soc. Am), 539–579.
Sáez, J. A., Clemente, R., Bustamante, M. A., Yañez, D., and Bernal, M. P. (2017). Evaluation of the slurry management strategy and the integration of the composting technology in a pig farm—agronomical and environmental implications. J. Environ. Manag. 192, 57–67. doi:10.1016/j.jenvman.2017.01.040
Santos, A., Bustamante, M. A., Tortosa, G., Moral, R., and Bernal, M. P. (2016). Gaseous emissions and process development during composting of pig slurry: the influence of the proportion of cotton gin waste. J. Clean. Prod. 112, 81–90. doi:10.1016/j.jclepro.2015.08.084
Sommer, S. G., Kjellerup, V., and Kristjansen, O. (1992). Determination of total ammonium nitrogen in pig and cattle slurry: sample preparation and analysis. Acta Agric. Scand. B Soil Plant Sci. 42, 146–151.
Soumaré, F. M., Tack, F. M. G., and Verloo, M. G. (2003). Ryegrass response to mineral fertilization and organic amendment with municipal solid waste compost in two tropical agricultural soils of Mali. J. Plant Nutr. 26, 1169–1188. doi:10.1081/PLN-120020363
Tejada, M., and Gonzalez, J. L. (2006). Crushed cotton gin compost on soil biological properties and rice yield. Eur. J. Agron. 25, 22–29. doi:10.1016/j.eja.2006.01.007
Thomsen, I. K., and Kjellerup, V. (1997). Yields and N uptake of barley and ryegrass from soils with added animal manure differing in straw and urine content. Eur. J. Agron. 7, 285–292. doi:10.1016/S1161-0301(97)00005-1
Tiquia, S. M., Richard, T. L., and Honeyman, M. S. (2002). Carbon, nutrient, and mass loss during composting. Nutr. Cycl. Agroecosyst. 62, 15–24. doi:10.1023/A:1015137922816
Vanotti, M. B., Millner, P. D., Szogi, A. A., Campbell, C. R., and Fetterman, L. M. (2006). “Aerobic composting of swine manure solids mixed with cotton gin waste,” in ASABE Annual International Meeting (Portland, OR). Paper Number: 064061.
Watanabe, F. S., and Olsen, S. R. (1965). Test of an ascorbic acid method for determining phosphorus in water and NaHCO3 extracts from soil. Soil Sci. Soc. Am. J. 29, 677–678. doi:10.2136/sssaj1965.03615995002900060025x
Keywords: nitrate, organic fertilizer, plant nutrient uptake, ryegrass, phosphorus
Citation: Santos A, Fangueiro D, Moral R and Bernal MP (2018) Composts Produced From Pig Slurry Solids: Nutrient Efficiency and N-Leaching Risks in Amended Soils. Front. Sustain. Food Syst. 2:8. doi: 10.3389/fsufs.2018.00008
Received: 09 November 2017; Accepted: 14 March 2018;
Published: 29 March 2018
Edited by:
Maria Cruz Garcia Gonzalez, Instituto Tecnológico Agrario de Castilla y León, SpainReviewed by:
Fabrizio Adani, Università degli Studi di Milano, ItalyM. R. Morales-Corts, Universidad de Salamanca, Spain
Copyright: © 2018 Santos, Fangueiro, Moral and Bernal. This is an open-access article distributed under the terms of the Creative Commons Attribution License (CC BY). The use, distribution or reproduction in other forums is permitted, provided the original author(s) and the copyright owner are credited and that the original publication in this journal is cited, in accordance with accepted academic practice. No use, distribution or reproduction is permitted which does not comply with these terms.
*Correspondence: Maria Pilar Bernal, cGJlcm5hbEBjZWJhcy5jc2ljLmVz